- Department of Pathology, Dunedin School of Medicine, University of Otago, Dunedin, New Zealand
Polycystic kidney disease (PKD) is a significant cause of end-stage kidney failure and there are few effective drugs for treating this inherited condition. Numerous aberrantly expressed non-coding RNAs (ncRNAs), particularly microRNAs (miRNAs), may contribute to PKD pathogenesis by participating in multiple intracellular and intercellular functions through post-transcriptional regulation of protein-encoding genes. Insights into the mechanisms of miRNAs and other ncRNAs in the development of PKD may provide novel therapeutic strategies. In this review, we discuss the current knowledge about the roles of dysregulated miRNAs and other ncRNAs in PKD. These roles involve multiple aspects of cellular function including mitochondrial metabolism, proliferation, cell death, fibrosis and cell-to-cell communication. We also summarize the potential application of miRNAs as biomarkers or therapeutic targets in PKD, and briefly describe strategies to overcome the challenges of delivering RNA to the kidney, providing a better understanding of the fundamental advances in utilizing miRNAs and other non-coding RNAs to treat PKD.
1 Introduction
Polycystic kidney disease (PKD) is an inherited disorder in which fluid-filled cysts enlarge the kidney, resulting in kidney failure. Autosomal dominant polycystic kidney disease (ADPKD) and autosomal recessive polycystic kidney disease (ARPKD) are the two main types of PKD, with the more common adult form ADPKD having an estimated prevalence of between 1:1,000 and 1:2,500 (Torres et al., 2007; Willey et al., 2017). PKD1 mutations are responsible for 80% of ADPKD cases, while around 15% of cases are attributed to PKD2 mutations. The remaining cases are due to rare or unknown genetic abnormalities in other loci (Vasileva et al., 2021). ARPKD is less common than ADPKD with a reported incidence of 1 in 26,500 live births (Bergmann et al., 2018). ARPKD usually develops perinatally or in childhood, and most commonly results from missense or truncating mutations in PKHD1. The vasopressin 2 receptor antagonist tolvaptan is the only Food and Drug Administration (FDA)-approved drug for use in ADPKD, and can delay the progression of ADPKD but exhibits side effects like polyuria and liver damage (Muller et al., 2021). No drug has been approved for ARPKD treatment, however several potential drugs for ADPKD have been in clinical trials.
In the last 2 decades, increasing evidence supports key roles for non-coding RNAs (ncRNAs) in ADPKD pathogenesis. NcRNAs are classified into long ncRNAs (lncRNAs) and small ncRNAs, based on a size cut-off of 200 nucleotides. LncRNAs can be further divided into two types, linear lncRNAs and circular RNAs (circRNAs), and are characterized by the absence of a long open reading frame. Small ncRNAs comprise microRNA (miRNA), small interfering RNA (siRNA), piwi-interacting RNA (piRNA), transfer RNA-derived stress-induced small siRNA (tiRNA), small nuclear ribonucleic acid (snRNA), small nucleolar RNA (snoRNA), repeat-associated small interfering RNA (rasiRNA), small cajal body-specific RNA (scaRNA) and others (Zhou and Li, 2021). Among them, miRNAs are small single strand non-coding RNAs containing 19–25 nucleotides, of which a seed sequence, usually 2 to 8 bases, can perfectly or imperfectly bind to the 3’ untranslated region of complementary target mRNAs to mediate gene expression by translational repression or mRNA cleavage. MiRNAs are the most extensively investigated ncRNAs in a variety of diseases such as cancer, diabetes and kidney diseases, and their therapeutic application is the nearest to clinical development. Recent evidence has shown that ncRNAs, especially miRNAs, regulate cyst growth through diverse mechanisms, and could serve as potential biomarkers and therapeutic targets or tools. Thus, this review focuses on the current understanding of the molecular mechanisms by which ncRNAs and especially miRNAs contribute to the pathogenesis of PKD. We discuss advances in the understanding of ncRNAs as biomarkers as well as therapeutic targets or tools in PKD, and methods for delivery of these compounds to the kidney.
2 MicroRNAs in polycystic kidney disease pathogenesis
MiRNAs have been found to be expressed aberrantly in PKD patients and also in multiple PKD models by miRNA microarray, miRNA sequencing or RT-qPCR (Choi et al., 2021) (Dweep et al., 2013). Individual members of the miR-17–92 cluster represent differentially expressed miRNAs (DE-miRNAs) in both human and mouse ADPKD kidneys. Overexpression of the miR-17–92 cluster promoted tubular cysts and glomerular cysts by both directly targeting Pkd1 and Pkd2 via miR-17-5p and indirectly through inhibiting Pkd2 and Pkhd1 by miR-92a-3p targeting the transcription factor hepatocyte nuclear factor-1β (Hnf-1β) (Hajarnis et al., 2015).
Several aberrantly expressed factors have been shown to contribute to DE-miRNAs in PKD. Inactivation of Dicer, which is a necessary RNase III endonuclease that processes pre-miRNAs into mature miRNAs, resulted in downregulation of the miR-200 cluster and formation of tubular and glomerular cysts (Patel et al., 2012). RNA helicase p68, which forms a key ternary complex with Drosha for miRNA biogenesis, was elevated in ADPKD and its knockdown reduced levels of mature miR-17-5p, miR-200c-3p, and miR-182-5p. On the other hand, changes in regulation of miRNAs by transcription factors overexpressed in PKD has also been reported, including HNF-1β regulating the miR-200 cluster (Hajarnis et al., 2015), c-Myc regulating miR-17-5p (Hajarnis et al., 2017), and CREB regulating miR-21-5p (Lakhia et al., 2016). The proposed roles of significant DE-miRNAs in PKD are discussed below and summarized in Table 1.
2.1 MicroRNAs modulate oxidative stress in mitochondrial metabolism
Although Pkd1 and Pkd2 are direct miR-17-5p targets, miR-17-5p upregulated by c-Myc was also able to enhance proliferation in cystic kidneys from Pkd1 knockout (KO) ADPKD models, at least in part, by inhibiting mitochondrial oxidative phosphorylation through repressing Pparα (Hajarnis et al., 2017). Increased miR-132-3p in ADPKD was shown to increase mitochondrial superoxide levels by directly repressing its target gene Foxo3a. This transcription factor regulates the expression of Gatm to induce reactive oxygen species accumulation in ADPKD (Ishimoto et al., 2017), indicating that oxidative stress might be one of the key factors in the pathogenesis of ADPKD.
2.2 MicroRNAs dysregulate cell proliferation
Like development of tumour cells, cyst progression also requires sustained proliferative signaling (Lantinga-van Leeuwen et al., 2007). Over-activation of mTOR-regulated pathways is one of the critical pathogenic processes for hyper-proliferation in PKD (Ibraghimov-Beskrovnaya and Natoli, 2011). Upregulation of miR-501-5p in ADPKD cells and tissues activates mTOR by repressing PTEN and TSC1, resulting in cell proliferation (De Stephanis et al., 2018). Though mTOR is a key pathway for cyst growth, mTOR inhibitors are not recommended for treating ADPKD because of various side effects noted in clinical trials, including interfering with normal recovery from injury (Torres et al., 2010). Nevertheless, an mTOR inhibitor used at low doses or in combination with other effective drugs could be further investigated.
Normal cells have many genes that strictly control proliferation, but in tumours, as well as in cysts, these genetic suppressors are often inactivated, leading to accelerated cell proliferation. Downregulation of several miRNAs targeting classical proliferative signaling pathways such as EGFR/ErbB1 has been demonstrated in association with ADPKD (Harskamp et al., 2016). MiR-193a-3p which contributes as a tumor suppressor miRNA in many cancer types (Khordadmehr et al., 2019) was reported to be downregulated in cystic cell lines derived from ADPKD human kidneys. Reduced miR-193a-3p led to increased expression of its target, the EGF/ErbB family receptor ErbB4, in human and mouse ADPKD cysts, resulting in cyst proliferation (Streets et al., 2017).
2.3 MicroRNAs respond to cell damage
Cellular mechanisms that protect against cell damage, such as autophagy and apoptosis, also play a distinct role in cell survival in PKD. Genes positively regulating autophagy were highly expressed in ADPKD patients samples in an online public dataset (GSE35831) (Liu et al., 2019), and inhibition of an autophagy suppressor in cysts indicated that autophagy is a key component in the development of PKD. MiR-25-3p was reported to be increased in Pkd1-KO mice. Inhibition of miR-25-3p augmented autophagy, while silencing its target, ATG14, abolished the effect of miR-25-3p inhibition in a model of PKD (Liu et al., 2020).
Apoptosis is another crucial mechanism in PKD where miRNAs participate. For example, miR-199a-5p has been reported to be highly expressed, both in human ADPKD tissues and in an ADPKD rat model. Antisense inhibition of miR-199a-5p induced apoptosis, which was suggested by the authors as likely due to the action of its target gene, CDKN1C/p57, an important cell cycle regulator (Sun et al., 2015). Additionally, increased expression of a conserved onco-microRNA, miR-21-5p, has been found in cyst epithelial cells from various PKD mouse models and human ADPKD patients (Lakhia et al., 2016). Specifically, miR-21-5p was shown to be associated with cyst expansion through apoptosis suppression, rather than cyst initiation.
2.4 MicroRNAs play a role in inflammation
Proinflammatory cytokines such as IL-1β, TNF-alpha, and IL-2 are present in the cyst microenvironment of human ADPKD kidneys (Gardner et al., 1991), and macrophages accumulate in cystic areas of the kidney (Karihaloo et al., 2011). High expression of interstitial miR-214-3p and its host lncRNA Dnm3os, derived from stromal cells rather than cyst epithelial cells, were validated by both in situ hybridization and single-cell RNA-Seq (Lakhia et al., 2020). Interestingly, this study also revealed that the proinflammatory TLR4/IFN-γ/STAT1 pathway activated miR-214-3p transcription and in turn, miR-214-3p directly inhibited Tlr4. Deletion of miR-214-3p resulted in elevated Tlr4 mRNA expression, peri-cystic macrophage accumulation, and aggravated cyst growth, consistent with previous reports that macrophages promote cyst growth in ADPKD (Lakhia et al., 2020). These findings suggest that miR-214-3p generates a compensatory protective response in the cyst environment that restrains cyst inflammation.
2.5 MicroRNAs participate in Fibrosis
PKD is invariably associated with not only the expansion of cysts, but also the excessive deposition of extracellular matrix (ECM), resulting in ongoing fibrosis and loss of renal function leading to end-stage kidney disease (Xue and Mei, 2019). In PKD, the mechanisms for kidney fibrosis include chronic inflammation, epithelial-mesenchymal transition (EMT), up-regulation of transforming growth factor-β1 (TGF-β1) and increased expression of interstitial ECM proteins (Xue and Mei, 2019).
Previous profiling of end-stage ADPKD kidneys has shown increased expression of EMT-associated genes. Additionally, cystic epithelial cells express mesenchymal markers in the genotypic ADPKD Pck rat model, supporting a role for EMT in the pathogenesis of PKD (Norman, 2011). MiR-192-5p and -194-5p are both enriched in normal kidney but were found at decreased levels in the cysts of end-stage ADPKD human and mouse tissues (Kim et al., 2019). When miR-192-5p and -194-5p precursors were introduced into cysts, they were found to retard cyst growth by reducing expression of the EMT-associated genes ZEB2 and CDH2. Another miRNA, miR-182-5p, was also positively correlated with fibrosis and elevated the expression of collagen I, collagen IV and fibronectin in cystic epithelial cells (Sun et al., 2020). These findings indicate that dysfunctional miRNA expression in cystic epithelial cells contributes to fibrosis and might represent novel prognostic biomarkers or therapeutic targets for the progression of PKD.
The sources of ECM production in ADPKD are likely to be a heterogeneous population and are not limited to cystic epithelial cells. Myofibroblasts, which exhibit features of fibroblasts and smooth muscle cells, are generally considered to be the major source of ECM, leading to renal scarring and fibrosis. Transition of different cell types into activated interstitial myofibroblasts might also occur during renal fibrosis (Meng et al., 2016). In pericyte-derived myofibroblasts, silencing miR-132 via TGF-β signaling (Smad2/Smad3) attenuated the progression of renal fibrosis by selectively decreasing myofibroblast proliferation (Bijkerk et al., 2016). Crosstalk signaling, including via miRNAs within diverse cells types, potentially participates in the progression of cystic epithelial cells and accumulated fibrosis.
2.6 MicroRNAs are associated with cell-to-cell contacts via extracellular vesicles and exosomes
Bioinformatic analysis of two public GEO datasets (GSE7869 and GSE35831) of human ADPKD revealed that differentially expressed genes were enriched in extracellular exosomes (Liu et al., 2019). Cystic cell derived extracellular vesicles (EVs) and urinary exosomes derived from ADPKD patients promoted cyst growth in Pkd1 mutant kidneys and in 3D cultures (Ding et al., 2021). This function was partially achieved by microRNAs which are a part of the diverse array of biomolecules contained within EVs/exosomes. Increased levels of miR-200b-3p, miR-200c-3p, and miR-429-3p were secreted in EVs/exosomes derived from Pkd1-null renal epithelial cells (Ding et al., 2021). Inhibition of exosome biogenesis/release with GW4869 significantly delayed cyst growth in both aggressive and milder ADPKD mouse models (Ding et al., 2021). These findings suggest that EVs/exosome-derived biomolecules (including miRNAs) crucially influence cell-to-cell contacts. Therefore, exosomes and their miRNA cargoes have both diagnostic and therapeutic potential for ADPKD, and their significance in PKD will likely expand in the future.
3 LncRNAs and polycystic kidney disease
LncRNAs have diverse functions, including as guides, scaffolds, or activators for transcription factors and histone modifiers. They influence posttranscriptional regulation by affecting mRNA activity and stability, as well as translational efficiency. LncRNAs have been implicated in a range of diseases including various types of cancer, and recently their involvement in ADPKD has also been reported. Makorin1-p1 is a lncRNA, downregulation of which induced polycystic kidney and bone deformity in transgene-insertion-induced Makorin1-p1 mutant mice (Ivanova et al., 2003). This lncRNA shares a homologous 5’ region with the protein-encoding gene Makorin-1 and potentially protects this mRNA by competitive binding to a trans-acting RNA-destabilizing factor, whose binding site is within the homologous region of Makorin-1, increasing Makorin-1 mRNA stability (Ivanova et al., 2003). As mentioned previously, Dnm3os, the host lncRNA of miR-214-3p, was upregulated in orthologous ADPKD mouse models and cystic kidneys from ADPKD patients (Lakhia et al., 2020). Although its function has not been studied further in a PKD context, overexpression of Dnm3os in macrophages alters global histone modification and enhances inflammation (Das et al., 2018), indicating a possible role for Dnm3os in ADPKD. Similarly, HNF-1β, which directly regulates Pkd2 and Pkhd1, also binds to the promoter of a 28 kb lncRNA that encodes the miR-200b-3p/200a-3p/429-3p cluster, to regulate the expression of Zeb2 and Pkd1 (two target genes of miR-200b-3p), indirectly producing cysts (Hajarnis et al., 2015). RNA-seq has identified a kidney-specific, evolutionarily conversed lncRNA called Hoxb3os that was highly expressed in renal tubules in adult wild-type mice but down-regulated in cystic kidneys from Pkd1 and Pkd2 mutant mice. The human ortholog HOXB3-AS1 was also down-regulated in cystic kidneys from ADPKD patients (Aboudehen et al., 2018). Knock-out of Hoxb3os increased phosphorylation of mTOR and its down-stream targets, namely p70 S6 kinase, ribosomal protein S6, and the translation repressor 4E-BP1. Through activating mTOR signaling, Hoxb3os-KO cells displayed increased mitochondrial respiration. Exactly how Hoxb3os regulates the mTOR signaling is still unclear, but these findings indicate functional roles for lncRNAs in PKD.
In addition, lncRNAs that mediate expression of PKD1 in other diseases might be implicated in PKD and require further investigation. For example, lncRNA H19 can recruit CCCTC-binding factor to the promoter of PKD1, and inhibit the transcription of PKD1 in atherosclerotic vulnerable plaques (Yang et al., 2019). Furthermore, through bioinformatic analysis the PKD1–miR-20b-5p–AP000797 axis was predicted to regulate intervertebral disc degeneration (Cao et al., 2021).
4 Prospective applications of non-coding RNAs in polycystic kidney disease
4.1 Emerging biomarkers as liquid biopsy
Due to the relative stability of cell-free circulating miRNAs (as well as miRNAs and lncRNAs found in exosomes) in multiple body fluids, ncRNAs (and especially miRNAs) have become candidate biomarkers in liquid biopsy for various diseases. Compared with healthy subjects, serum from ADPKD patients contained increased levels of miR-3907-3p, miR-92a-3p, miR-25-3p and miR-21-5p, and decreased levels of miR-1587-5p and miR-3911-5p as renal function declined. Among these, an increasing level of miR-3907-3p had a potential predictive value for disease progression (Kocyigit et al., 2017). Profiling revealed that another four miRNAs are dysregulated in ADPKD urine specimens, compared to specimens of other chronic kidney diseases (Ben-Dov et al., 2014). In an initial discovery cohort of ADPKD patients with early or late disease, compared with healthy controls, profiling of human urinary exosome miRNA demonstrated that miR-192-5p, miR-194-5p, miR-30a-5p, miR-30d-5p and miR-30e-5p were significantly downregulated in patient urine exosomes, in both human PKD1 cystic kidney tissue, and in murine Pkd1 cystic kidneys. All five miRNAs showed significant correlation with baseline eGFR and ultrasound-determined mean kidney length, and improved the diagnostic performance (area under the curve) of mean kidney length for the rate of disease progression (Magayr et al., 2020).
In addition to biomarkers for disease progression, miRNAs can also serve as predictive factors for complications of ADPKD. For example, plasma levels of miR-16-5p were reported to be a predictive factor for intracranial aneurysms in renal transplant recipients with ADPKD, and may become a tool to identify high-risk groups who should undergo magnetic resonance angiography for intracranial aneurysm screening (Kulesza et al., 2021).
4.2 Potential therapeutic targets for polycystic kidney disease
The only ncRNA-based therapeutic approach for ADPKD to reach clinical trial to date is the use of antisense oligonucleotides to inhibit miR-17-5p. Preclinical results showed that the anti-miR-17-5p oligonucleotide RGLS4326 attenuated cyst growth in multiple PKD models (Lee et al., 2019). Interestingly, a recent publication demonstrated de-repression of Pkd1 and Pkd2 as a result of CRISPR/Cas9-mediated deletion of 3’-UTR miR-17-5p binding sites. This significantly alleviated cyst growth with decreased kidney-weight-to-body-weight ratio, decreased serum blood urea nitrogen levels, improved mitochondrial membrane potential, and inhibition of the pro-cystogenic effect of cAMP. These results were observed in cellular, ex vivo, and mouse ADPKD models (Lakhia et al., 2022). Moreover, blockade by RGLS4326 of Pkd1/2 cis-inhibition prevented cyst onset and stabilized existing cysts in mice (Lakhia et al., 2022). However, dose-limiting central nervous system toxicity was observed in both mice and monkeys receiving high doses of RGLS4326 due to the off-target inhibition of the neuroreceptor α-amino-3-hydroxy-5-methyl-4-isoxazolepropionic acid receptor (AMPA-R) by RGLS4326 (Edmund Lee, 2022). To overcome this toxicity, a next-generation anti-miR-17-5p oligonucleotide RGLS8429 with similar efficacy as RGLS4326, but no affinity to AMPA-R, has been developed and is currently being evaluated in a Phase 1 clinical trial (NCT05429073).
To complement sequence-based recognition of oligonucleotides, structure-binding small molecules also have huge potential for clinical application. Primary miRNAs and lncRNAs have specific hairpin structures which are favorable regions for structure-specific ligands to target. A ligand that targets the primary miR-17-92 cluster reduced its expression in PKD, prostate cancer, and breast cancer and was shown to rescue disease-associated phenotypes in the latter two (Liu et al., 2020b). Application of this ligand and its chemical modification, or optimized conjugation to an endogenous nuclease or a ribonuclease targeting chimera as an RNA-degradation targeting treatment for PKD warrants further investigation.
5 Delivery of non-coding RNAs to the kidney
Although single-stranded, chemically modified, antisense molecules such as RGLS4326 can be administered without a carrier (Lee et al., 2019), replacing suppressed microRNAs (using double-stranded RNA precursors) is more difficult. Although few miRNA mimics or siRNA-based therapies in PKD have been studied, research on RNA delivery in other kidney diseases might be informative and can be divided into pharmacological modification or use of delivery vehicles or carriers (Figure 1). Pharmacological modification of RNAs is an effective way to overcome poor stability, immune responses and to change the tissue-specific uptake. Due to the limitations of viral vectors, non-viral vehicle platforms to deliver RNAs to kidney such as nanoparticles and engineered exosomes have been advanced to stabilize RNAs and overcome poor cellular uptake in kidney disease. Other novel technologies, such as combinations of focused ultrasound and gas-filled microbubbles (Wonnacott et al., 2022) or virus-like particles (Segel et al., 2021) might also be applied to kidney and PKD treatment in the near future. A hurdle may be non-specific liver accumulation, although systemically administered carrier-free short RNAs do appear to preferentially accumulate in the kidney (Denby et al., 2014; Lee et al., 2019). This is possibly due to their hydrophilic nature, size and negative charge which could affect glomerular filtration and absorption, and release into cyst-related cells such as cyst-lining tubular epithelial cells, and cyst-surrounding macrophages (Fernandez-Pineiro et al., 2017).
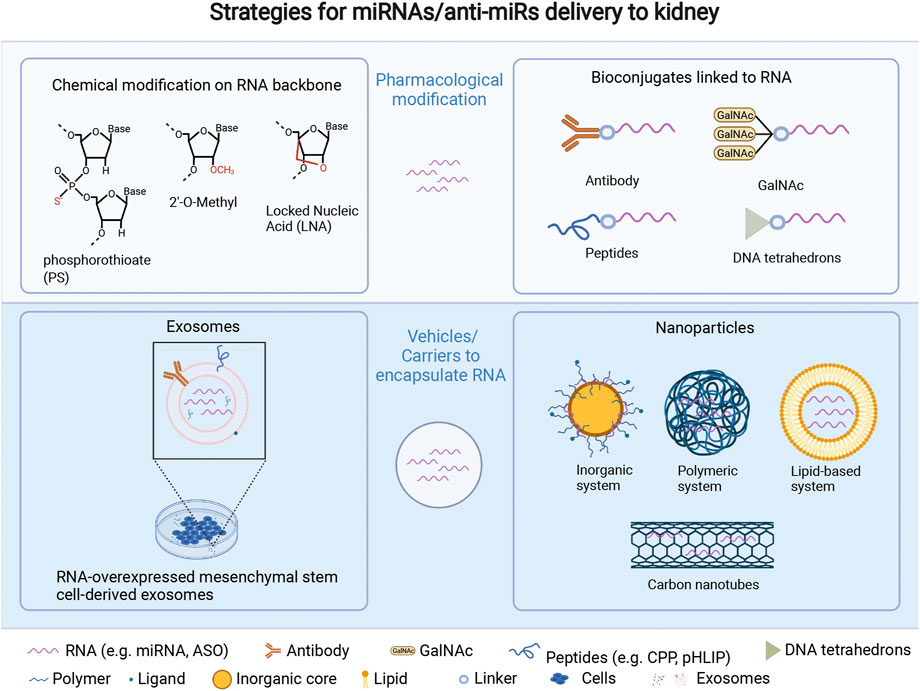
FIGURE 1. Strategies for miRNA/anti-miR delivery to kidney, which include pharmacological modification of miRNAs/anti-miRs, and vehicles/carriers embedding miRNAs/anti-miRs. Pharmacological modification: 1) chemical modifications such as phosphorothioate (PS) (Yheskel et al., 2019), 2ʹ-O-methyl (Straarup et al., 2010), and locked nucleic acid (Lendvai et al., 2005) all result in a high kidney uptake through intravenous injection. 2) Bioconjugates can address some oligonucleotide delivery challenges, such as improving biodistribution to a specific region or cell type (e.g., antibodies, DNA tetrahedrons) (Benizri et al., 2019), promoting endosomal escape (e.g., CPP) (Miguel et al., 2020), increasing receptor-mediated transport (e.g., GalNAc), and/or improving ability to cross cell and endosomal membranes (e.g., pHLIP). (Rohde et al., 2015; Wagner, 2015). Delivery vehicles or carriers comprise two main methods. 1) exosomes derived from various cell types, like mesenchymal stem cells, have the potential to be drug carriers for ncRNAs or oligonucleotides due to their stability, minimal immune response, and editable surface with various RNAs loading strategies including diffusion via a concentration gradient, transfection, or physical treatments like electroporation, which can also expand the cargo loading capability of exosomes (Jin et al., 2021). 2) Nanoparticles, which refer to a stable structure with a protective layer that can encapsulate and protect inner agents, and be modified with ligands or antibodies on their surface for kidney-specific targeting at a nano scale, have the potential to improve the pharmacokinetics, biodistribution, toxicity, and efficacy of encapsulated drugs: 1) inorganic system like pSi and magnetic particles (Tsai et al., 2019); 2) polymeric system including synthetic polymerics like polymeric CXCR4 inhibitors (Tang et al., 2022) and natural polymers such as chitosan (Chen et al., 2019); 3) lipid-based system (Su et al., 2022); 4) carbon-nanotubes (Alidori et al., 2016). LNA = locked nucleic acid; CPP = cell penetrating peptide; GalNAc = N-acetylgalactosamine; pHLIP = pH (low) insertion peptides. Created with Biorender.com.
6 Conclusion
MiRNAs and lncRNAs are emerging as indispensable regulators in the development of PKD. The discovery of aberrantly expressed ncRNAs in both PKD models and patient samples, provide clues for new molecular mechanisms of PKD pathogenesis and potential applications as biomarkers or therapeutic targets. Nevertheless, there are significant gaps, such as observed differences in efficacy between PKD animal models and clinical trials, issues with kidney-specificity of ncRNA delivery, and also off-target effects observed between ncRNA preclinical studies and clinical trials for PKD. The preclinical and clinical outcomes of RGLS4326 and RGLS8429 may provide valuable knowledge for ncRNA-based therapeutics in PKD. Moreover, precise ncRNA profiling of human specimens are still challenging and require further optimization. Importantly, the potential roles of other ncRNAs (in addition to miRNAs and lncRNAs) in PKD remain uninvestigated. Further studies are needed to support the emerging implementation of ncRNAs as therapeutic biomarkers and targets for patients with PKD.
Author contributions
The manuscript was written by QZ, and revised and edited by GR, ME, and CS.
Funding
QZ is supported by the Chinese Scholarship Council–New Zealand-China Research Collaboration Centres (CSC-NZ CRCC) joint funding programme and the New Zealand-China Non-Communicable Diseases Research Collaboration Centre (NCD CRCC), who also provided the publication fee for this article.
Conflict of interest
The authors declare that the research was conducted in the absence of any commercial or financial relationships that could be construed as a potential conflict of interest.
Publisher’s note
All claims expressed in this article are solely those of the authors and do not necessarily represent those of their affiliated organizations, or those of the publisher, the editors and the reviewers. Any product that may be evaluated in this article, or claim that may be made by its manufacturer, is not guaranteed or endorsed by the publisher.
References
Aboudehen K., Farahani S., Kanchwala M., Chan S. C., Avdulov S., Mickelson A. (2018). Long noncoding RNA Hoxb3os is dysregulated in autosomal dominant polycystic kidney disease and regulates mTOR signaling. J. Biol. Chem. 293 (24), 9388–9398. doi:10.1074/jbc.RA118.001723
Alidori S., Akhavein N., Thorek D. L., Behling K., Romin Y., Queen D., Beattie B. J., Manova-Todorova K., Bergkvist M., Scheinberg D. A., McDevitt M. R. (2016). Targeted fibrillar nanocarbon RNAi treatment of acute kidney injury. Sci. Transl. Med. 8 (331), 331ra339. doi:10.1126/scitranslmed.aac9647
Ben-Dov I. Z., Tan Y. C., Morozov P., Wilson P. D., Rennert H., Blumenfeld J. D., Tuschl T. (2014). Urine microRNA as potential biomarkers of autosomal dominant polycystic kidney disease progression: Description of miRNA profiles at baseline. PLoS One 9 (1). doi:10.1371/journal.pone.0086856doi:e86856
Benizri S., Gissot A., Martin A., Vialet B., Grinstaff M. W., Barthelemy P. (2019). Bioconjugated oligonucleotides: Recent developments and therapeutic applications. Bioconjug Chem. 30 (2), 366–383. doi:10.1021/acs.bioconjchem.8b00761
Bergmann C., Guay-Woodford L. M., Harris P. C., Horie S., Peters D. J. M., Torres V. E. (2018). Polycystic kidney disease. Nat. Rev. Dis. Prim. 4 (1), 50. doi:10.1038/s41572-018-0047-y
Bijkerk R., de Bruin R. G., van Solingen C., van Gils J. M., Duijs J. M., van der Veer E. P., Rabelink T. J., Humphreys B. D., van Zonneveld A. J. (2016). Silencing of microRNA-132 reduces renal fibrosis by selectively inhibiting myofibroblast proliferation. Kidney Int. 89 (6), 1268–1280. doi:10.1016/j.kint.2016.01.029
Cao S., Liu H., Fan J., Yang K., Yang B., Wang J., Li J., Meng L., Li H. (2021). An oxidative stress-related gene pair (CCNB1/PKD1), competitive endogenous RNAs, and immune-infiltration patterns potentially regulate intervertebral disc degeneration development. Front. Immunol. 12. doi:10.3389/fimmu.2021.765382doi:765382
Chen Y., Tang W., Yu F., Xie Y., Jaramillo L., Jang H. S., Li J., Padanilam B. J., Oupický D. (2019). Determinants of preferential renal accumulation of synthetic polymers in acute kidney injury. Int. J. Pharm. 568. doi:10.1016/j.ijpharm.2019.118555doi:118555
Choi S., Kim D. Y., Ahn Y., Lee E. J., Park J. H. (2021). Suppression of foxo3-gatm by miR-132-3p accelerates cyst formation by up-regulating ROS in autosomal dominant polycystic kidney disease. Biomol. Ther. Seoul. doi:10.4062/biomolther.2020.197
Das S., Reddy M. A., Senapati P., Stapleton K., Lanting L., Wang M., Amaram V., Ganguly R., Zhang L., Devaraj S., Schones D. E. (2018). Diabetes mellitus-induced long noncoding RNA Dnm3os regulates macrophage functions and inflammation via nuclear mechanisms. Arterioscler. Thromb. Vasc. Biol. 38 (8), 1806–1820. doi:10.1161/ATVBAHA.117.310663
de Stephanis L., Mangolini A., Servello M., Harris P. C., Dell'Atti L., Pinton P., Aguiari G. (2018). MicroRNA501-5p induces p53 proteasome degradation through the activation of the mTOR/MDM2 pathway in ADPKD cells. J. Cell Physiol. 233 (9), 6911–6924. doi:10.1002/jcp.26473
Denby L., Ramdas V., Lu R., Conway B. R., Grant J. S., Dickinson B., Aurora A. B. (2014). MicroRNA-214 antagonism protects against renal fibrosis. J. Am. Soc. Nephrol. 25 (1), 65–80. doi:10.1681/ASN.2013010072
Ding H., Li L. X., Harris P. C., Yang J., Li X. (2021). Extracellular vesicles and exosomes generated from cystic renal epithelial cells promote cyst growth in autosomal dominant polycystic kidney disease. Nat. Commun. 12 (1), 4548. doi:10.1038/s41467-021-24799-x
Dweep H., Sticht C., Kharkar A., Pandey P., Gretz N. (2013). Parallel analysis of mRNA and microRNA microarray profiles to explore functional regulatory patterns in polycystic kidney disease: Using PKD/mhm rat model. PLoS One 8 (1). doi:10.1371/journal.pone.0053780doi:e53780
Edmund Lee T. V., Owen Tate, Ramalingam Harini, Lakhia Ronak, Patel Vishal, Varrone Francesca, Wallace D. P., Lee E. C., Patel V. (2022). Discovery of next-generation anti-miR-17 oligonucleotide RGLS8429 for treatment of autosomal dominant polycystic kidney disease (ADPKD) [online]. Regulus therapeutics. Available: https://www.regulusrx.com/wp-content/uploads/FASEB-PKD-2022_RegulusPoster_FINAL-1.pdf [Accessed August 26 2022].
Fernandez-Pineiro I., Badiola I., Sanchez A. (2017). Nanocarriers for microRNA delivery in cancer medicine. Biotechnol. Adv. 35 (3), 350–360. doi:10.1016/j.biotechadv.2017.03.002
Gardner K. D., Burnside J. S., Elzinga L. W., Locksley R. M. (1991). Cytokines in fluids from polycystic kidneys. Kidney Int. 39(4), 718–724. doi:10.1038/ki.1991.87
Hajarnis S., Lakhia R., Yheskel M., Williams D., Sorourian M., Liu X., Aboudehen K., Zhang S., Kersjes K., Galasso R., Li J. (2017). microRNA-17 family promotes polycystic kidney disease progression through modulation of mitochondrial metabolism. Nat. Commun. 8. doi:10.1038/ncomms14395doi:14395
Hajarnis S. S., Patel V., Aboudehen K., Attanasio M., Cobo-Stark P., Pontoglio M., et al. (2015). Transcription factor hepatocyte nuclear factor-1beta (HNF-1beta) regulates MicroRNA-200 expression through a long noncoding RNA. J. Biol. Chem. 290 (41), 24793–24805. doi:10.1074/jbc.M115.670646
Harskamp L. R., Gansevoort R. T., van Goor H., Meijer E. (2016). The epidermal growth factor receptor pathway in chronic kidney diseases. Nat. Rev. Nephrol. 12 (8), 496–506. doi:10.1038/nrneph.2016.91
Ibraghimov-Beskrovnaya O., Natoli T. A. (2011). mTOR signaling in polycystic kidney disease. Trends Mol. Med. 17 (11), 625–633. doi:10.1016/j.molmed.2011.06.003
Ishimoto Y., Inagi R., Yoshihara D., Kugita M., Nagao S., Shimizu A., et al. (2017). Mitochondrial abnormality facilitates cyst formation in autosomal dominant polycystic kidney disease. Mol. Cell Biol. 37 (24). doi:10.1128/MCB.00337-17
Ivanova N., Sorokin A., Anderson I., Galleron N., Candelon B., Kapatral V., et al. (2003). An expressed pseudogene regulates the messenger-RNA stability of its homologous coding gene. Nature 423 (6935), 87–91. doi:10.1038/nature01582
Jin C., Wu P., Li L., Xu W., Qian H. (2021). Exosomes: Emerging therapy delivery tools and biomarkers for kidney diseases. Stem Cells Int. 2021. doi:10.1155/2021/7844455doi:7844455
Karihaloo A., Koraishy F., Huen S. C., Lee Y., Merrick D., Caplan M. J., et al. (2011). Macrophages promote cyst growth in polycystic kidney disease. J. Am. Soc. Nephrol. 22 (10), 1809–1814. doi:10.1681/ASN.2011010084
Khordadmehr M., Shahbazi R., Sadreddini S., Baradaran B. (2019). miR-193: A new weapon against cancer. J. Cell Physiol. 234 (10), 16861–16872. doi:10.1002/jcp.28368
Kim D. Y., Woo Y. M., Lee S., Oh S., Shin Y., Shin J. O., et al. (2019). Impact of miR-192 and miR-194 on cyst enlargement through EMT in autosomal dominant polycystic kidney disease. Faseb J. 33 (2), 2870–2884. doi:10.1096/fj.201800563RR
Kocyigit I., Taheri S., Sener E. F., Eroglu E., Ozturk F., Unal A., et al. (2017). Serum micro-rna profiles in patients with autosomal dominant polycystic kidney disease according to hypertension and renal function. BMC Nephrol. 18 (1), 179. doi:10.1186/s12882-017-0600-z
Kulesza A., Kulesza A., Fliszkiewicz M., Łabuś A., Pączek L., Niemczyk M. (2021). miRNA-16 as a predictive factor for intracranial aneurysms in autosomal dominant polycystic kidney disease. Neurol. Neurochir. Pol. doi:10.5603/PJNNS.a2021.0026
Lakhia R., Hajarnis S., Williams D., Aboudehen K., Yheskel M., Xing C., et al. (2016). MicroRNA-21 aggravates cyst growth in a model of polycystic kidney disease. J. Am. Soc. Nephrol. 27 (8), 2319–2330. doi:10.1681/asn.2015060634
Lakhia R., Ramalingam H., Chang C. M., Cobo-Stark P., Biggers L., Flaten A., et al. (2022). PKD1 and PKD2 mRNA cis-inhibition drives polycystic kidney disease progression. Nat. Commun. 13 (1), 4765. doi:10.1038/s41467-022-32543-2
Lakhia R., Yheskel M., Flaten A., Ramalingam H., Aboudehen K., Ferrè S., et al. (2020). Interstitial microRNA miR-214 attenuates inflammation and polycystic kidney disease progression. JCI Insight 5 (7). doi:10.1172/jci.insight.133785
Lantinga-van Leeuwen I. S., Leonhard W. N., van der Wal A., Breuning M. H., de Heer E., Peters D. J. (2007). Kidney-specific inactivation of the Pkd1 gene induces rapid cyst formation in developing kidneys and a slow onset of disease in adult mice. Hum. Mol. Genet. 16 (24), 3188–3196. doi:10.1093/hmg/ddm299
Lee E. C., Valencia T., Allerson C., Schairer A., Flaten A., Yheskel M., et al. (2019). Discovery and preclinical evaluation of anti-miR-17 oligonucleotide RGLS4326 for the treatment of polycystic kidney disease. Nat. Commun. 10 (1), 4148. doi:10.1038/s41467-019-11918-y
Lendvai G., Velikyan I., Bergstrom M., Estrada S., Laryea D., Valila M., et al. (2005). Biodistribution of 68Ga-labelled phosphodiester, phosphorothioate, and 2'-O-methyl phosphodiester oligonucleotides in normal rats. Eur. J. Pharm. Sci. 26 (1), 26–38. doi:10.1016/j.ejps.2005.04.017
Liu D., Huo Y., Chen S., Xu D., Yang B., Xue C., et al. (2019). Identification of key genes and candidated pathways in human autosomal dominant polycystic kidney disease by bioinformatics analysis. Kidney Blood Press Res. 44 (4), 533–552. doi:10.1159/000500458
Liu G., Kang X., Guo P., Shang Y., Du R., Wang X., et al. (2020a). miR-25-3p promotes proliferation and inhibits autophagy of renal cells in polycystic kidney mice by regulating ATG14-Beclin 1. Ren. Fail 42 (1), 333–342. doi:10.1080/0886022x.2020.1745236
Magayr T. A., Song X., Streets A. J., Vergoz L., Chang L., Valluru M. K., et al. (2020). Global microRNA profiling in human urinary exosomes reveals novel disease biomarkers and cellular pathways for autosomal dominant polycystic kidney disease. Kidney Int. 98 (2), 420–435. doi:10.1016/j.kint.2020.02.008
Meng X. M., Nikolic-Paterson D. J., Lan H. Y. (2016). TGF-Beta: The master regulator of fibrosis. Nat. Rev. Nephrol. 12 (6), 325–338. doi:10.1038/nrneph.2016.48
Miguel V., Rey C., Aceña J. L., Maqueda F., Fernández-Hernando C., Rodríguez-Puyol D., et al. (2020). The pHLIP system as a vehicle for microRNAs in the kidney. Nefrol. Engl. Ed. 40 (5), 491–498. doi:10.1016/j.nefroe.2020.05.003
Muller R. U., Messchendorp A. L., Birn H., Capasso G., Gall E. C., Devuyst O., et al. (2021). An update on the use of tolvaptan for ADPKD: Consensus statement on behalf of the ERA working group on inherited kidney disorders (WGIKD), the European rare kidney disease reference network (ERKNet) and polycystic kidney disease international (PKD-International). Nephrol. Dial. Transpl. doi:10.1093/ndt/gfab312
Norman J. (2011). Fibrosis and progression of autosomal dominant polycystic kidney disease (ADPKD). Biochim. Biophys. Acta 1812 (10), 1327–1336. doi:10.1016/j.bbadis.2011.06.012
Patel V., Hajarnis S., Williams D., Hunter R., Huynh D., Igarashi P. (2012). MicroRNAs regulate renal tubule maturation through modulation of Pkd1. J. Am. Soc. Nephrol. 23 (12), 1941–1948. doi:10.1681/ASN.2012030321
Patel V., Williams D., Hajarnis S., Hunter R., Pontoglio M., Somlo S., et al. (2013). miR-17∼92 miRNA cluster promotes kidney cyst growth in polycystic kidney disease. Proc. Natl. Acad. Sci. U. S. A. 110 (26), 10765–10770. doi:10.1073/pnas.1301693110
Patil A., Sweeney W. E., Pan C. G., Avner E. D. (2018). Unique interstitial miRNA signature drives fibrosis in a murine model of autosomal dominant polycystic kidney disease. World J. Nephrol. 7(5), 108–116. doi:10.5527/wjn.v7.i5.108
Rohde J. H., Weigand J. E., Suess B., Dimmeler S. (2015). A universal aptamer chimera for the delivery of functional microRNA-126. Nucleic Acid. Ther. 25 (3), 141–151. doi:10.1089/nat.2014.0501
Segel M., Lash B., Song J., Ladha A., Liu C. C., Jin X., et al. (2021). Mammalian retrovirus-like protein PEG10 packages its own mRNA and can be pseudotyped for mRNA delivery. Science 373 (6557), 882–889. doi:10.1126/science.abg6155
Shin Y., Kim D. Y., Ko J. Y., Woo Y. M., Park J. H. (2018). Regulation of KLF12 by microRNA-20b and microRNA-106a in cystogenesis. Faseb J. 32 (7), 3574–3582. doi:10.1096/fj.201700923R
Straarup E. M., Fisker N., Hedtjarn M., Lindholm M. W., Rosenbohm C., Aarup V., et al. (2010). Short locked nucleic acid antisense oligonucleotides potently reduce apolipoprotein B mRNA and serum cholesterol in mice and non-human primates. Nucleic Acids Res. 38 (20), 7100–7111. doi:10.1093/nar/gkq457
Streets A. J., Magayr T. A., Huang L., Vergoz L., Rossetti S., Simms R. J., et al. (2017). Parallel microarray profiling identifies ErbB4 as a determinant of cyst growth in ADPKD and a prognostic biomarker for disease progression. Am. J. Physiol. Ren. Physiol. 312 (4), F577–f588. doi:10.1152/ajprenal.00607.2016
Su C. T., See D. H. W., Huang J. W. (2022). Lipid-based nanocarriers in renal RNA therapy. Biomedicines 10 (2). doi:10.3390/biomedicines10020283
Sun L., Hu C., Wang Z., Zhang X. (2020). MiR-182 inhibits kidney fibrosis by regulating transforming growth factor β1/Smad3 pathway in autosomal dominant polycystic kidney disease. IUBMB Life 72 (7), 1340–1348. doi:10.1002/iub.2255
Sun L., Zhu J., Wu M., Sun H., Zhou C., Fu L., et al. (2015). Inhibition of MiR-199a-5p reduced cell proliferation in autosomal dominant polycystic kidney disease through targeting CDKN1C. Med. Sci. Monit. 21, 195–200. doi:10.12659/msm.892141
Tang W., Chen Y., Jang H. S., Hang Y., Jogdeo C. M., Li J., et al. (2022). Preferential siRNA delivery to injured kidneys for combination treatment of acute kidney injury. J. Control Release 341, 300–313. doi:10.1016/j.jconrel.2021.11.029
Torres V. E., Boletta A., Chapman A., Gattone V., Pei Y., Qian Q., et al. (2010). Prospects for mTOR inhibitor use in patients with polycystic kidney disease and hamartomatous diseases. Clin. J. Am. Soc. Nephrol. 5 (7), 1312–1329. doi:10.2215/CJN.01360210
Torres V. E., Harris P. C., Pirson Y. (2007). Autosomal dominant polycystic kidney disease. Lancet 369 (9569), 1287–1301. doi:10.1016/s0140-6736(07)60601-1
Tsai Y. C., Teng I. L., Jiang S. T., Lee Y. C., Chiou Y. Y., Cheng F. Y. (2019). Safe nanocomposite-mediated efficient delivery of MicroRNA plasmids for autosomal dominant polycystic kidney disease (ADPKD) therapy. Adv. Healthc. Mater 8 (5). doi:10.1002/adhm.201801358doi:e1801358
Vasileva V. Y., Sultanova R. F., Sudarikova A. V., Ilatovskaya D. V. (2021). Insights into the molecular mechanisms of polycystic kidney diseases. Front. Physiol. 12. doi:10.3389/fphys.2021.693130doi:693130
Wagner E. (2015). Tumor-targeted delivery of anti-microRNA for cancer therapy: pHLIP is key. Angew. Chem. Int. Ed. Engl. 54 (20), 5824–5826. doi:10.1002/anie.201502146
Willey C. J., Blais J. D., Hall A. K., Krasa H. B., Makin A. J., Czerwiec F. S. (2017). Prevalence of autosomal dominant polycystic kidney disease in the European Union. Nephrol. Dial. Transpl. 32 (8), 1356–1363. doi:10.1093/ndt/gfw240
Wonnacott A., Denby L., Coward R. J. M., Fraser D. J., Bowen T. (2022). MicroRNAs and their delivery in diabetic fibrosis. Adv. Drug Deliv. Rev. 182. doi:10.1016/j.addr.2021.114045doi:114045
Woo Y. M., Kim D. Y., Koo N. J., Kim Y. M., Lee S., Ko J. Y., et al. (2017). Profiling of miRNAs and target genes related to cystogenesis in ADPKD mouse models. Sci. Rep. 7 (1). doi:10.1038/s41598-017-14083-8doi:14151
Xue C., Mei C. L. (2019). Polycystic kidney disease and renal fibrosis. Adv. Exp. Med. Biol. 1165, 81–100. doi:10.1007/978-981-13-8871-2_5
Yang Y., Tang F., Wei F., Yang L., Kuang C., Zhang H., et al. (2019). Silencing of long non-coding RNA H19 downregulates CTCF to protect against atherosclerosis by upregulating PKD1 expression in ApoE knockout mice. AGING 11 (22), 15. doi:10.18632/aging.102388
Yheskel M., Lakhia R., Cobo-Stark P., Flaten A., Patel V. (2019). Anti-microRNA screen uncovers miR-17 family within miR-17∼92 cluster as the primary driver of kidney cyst growth. Sci. Rep. 9 (1), 1920. doi:10.1038/s41598-019-38566-y
Zhou J. X., Li X. (2021). Non-coding RNAs in hereditary kidney disorders. Int. J. Mol. Sci. 22 (6). doi:10.3390/ijms22063014
Keywords: non-coding RNAs, miRNAs, long non-coding RNAs, polycystic kidney disease, autosomal dominant polycystic kidney disease
Citation: Zheng Q, Reid G, Eccles MR and Stayner C (2022) Non-coding RNAs as potential biomarkers and therapeutic targets in polycystic kidney disease. Front. Physiol. 13:1006427. doi: 10.3389/fphys.2022.1006427
Received: 29 July 2022; Accepted: 31 August 2022;
Published: 20 September 2022.
Edited by:
Jennifer Hollywood, The University of Auckland, New ZealandReviewed by:
Shin-ichi Makino, University Hospital, Chiba University, JapanVeronika Sander, The University of Auckland, New Zealand
Copyright © 2022 Zheng, Reid, Eccles and Stayner. This is an open-access article distributed under the terms of the Creative Commons Attribution License (CC BY). The use, distribution or reproduction in other forums is permitted, provided the original author(s) and the copyright owner(s) are credited and that the original publication in this journal is cited, in accordance with accepted academic practice. No use, distribution or reproduction is permitted which does not comply with these terms.
*Correspondence: Cherie Stayner, Y2hlcmllLnN0YXluZXJAb3RhZ28uYWMubno=