- 1Research Center for Molecular Exercise Science, University of Physical Education, Budapest, Hungary
- 2Faculty of Sport Sciences, Waseda University, Tokorozawa, Japan
- 3Faculty of Health Sciences, The University of Western Ontario, London, ON, Canada
Trainability is an adaptive response to given exercise loads and must be localized to the targeted physiological function since exercise-induced acute and chronic adaptations are systemic. Lack of adaptation or moderate level of adaptation in one organ or one physiological function would not mean that other organs or functions would not benefit from exercise training. The most beneficial training load could easily be different for skeletal muscle, brain, the gastro-intestinal track, or the immune systems. Hence, the term of non-responders should be used with caution and just referred to a given organ, cell type, molecular signaling, or function. The present paper aims to highlight some, certainly not all, issues on trainability especially related to muscle and cardiovascular system. The specificity of trainability and the systemic nature of exercise-induced adaptation are discussed, and the paper aims to provide suggestions on how to improve performance when faced with non-responders.
Introduction
Trainability is an important issue not only in elite sport but also for recreational athletes. It is often observed that a repeated exercise load does not cause increased performance for some subjects and those are referred to as non-responders (Bouchard and Rankinen, 2001; McLaren et al., 2018; Hortobagyi et al., 2021; Hrubeniuk et al., 2021; Mattioni Maturana et al., 2021). It is known that exercise-induced adaptation is different from the general adaptation theory developed by Selye (1975) since exercise-induced adaptation is specific. This specificity guarantees the necessity of different types and loads of training in various sports and explains the different phenotypes of sprinters, long-distance runners, or body builders.
One can suggest that non-responders are probably non-responders to a given training load and they can be responders when a personalized training load is given. Let’s take an example of those who are referred to as non-responders to improve maximal oxygen uptake (VO2max) by endurance exercise with moderate intensity (about 70% of VO2max). VO2max is referred to as a cardiovascular fitness measure, but VO2max is dependent on heart and arteriovenous oxygen difference (a-v difference) and can be measured by incremental exercise protocols in the laboratory or can be apprised by a huge number of field activities (Radák, 2018b). VO2max in very well trained athletes can reach 80 ml/kg/min. A-V difference is very much dependent on the capillarization and mitochondrial number of the skeletal muscle. The appropriate training to enhance cardiac output and to a-v difference could be easily different.
Most published work uses either moderate-intensity exercise, around 70% of the VO2max or high-intensity interval training (HIIT) to test the trainability of endurance. Moderate intensity training mostly results in general adaptation including enhanced cardiovascular function, while HIIT causes metabolism-induced adaptation to skeletal muscle (Radák, 2018a). It has been reported in a recent study that the adaptation to moderate-intensity training varied to a greater degree than after HIIT and non-responders were not found in the HIIT group (Maturana et al., 2021). Moreover, it would be interesting to test how non-responders adapt to mixed training, which contains both moderate and high-intensity training. In the present review, we aim to point out some important issues on trainability, in order to help understand exercise-induced systemic adaptation and we also offer some suggestions on how to improve performance, when faced with non-responders. In addition, it is important to note that exercise-induced adaptation is systemic, and non-responders in one function or organ could be responders in other functions and organs.
Adaptation to a Single Bout of Exercise
Due to the nature of a single bout of exercise, the adaptive response is limited but it is systemic. One of the driving mechanisms that modulate adaptive response is the exercise-induced changes in metabolism and the generated metabolites. Since the degree of the elevation, the type of metabolism and the generated metabolites, and the main energy source of adenosine triphosphate (ATP) production are dependent upon the intensity of exercise, adaptation is dependent on intensity. In general, in the skeletal muscle, low-intensity exercise of long duration increases the resistance to fatigue, and high-intensity exercise leads to muscle strength and growth. At the cellular level, it can be measured by increased mRNA and short-lived protein levels. Exercise with a short duration and high intensity could elevate mRNA levels of enzymes involved in glycolytic metabolism because of the break-down of carbohydrate under anaerobic conditions, and the shortage of O2 delivery can activate hypoxia-inducible factor 1 (HIF1), and thus the inadequate availability of ATP can lead to activation of adenylate kinase, which can lead to phosphorylation of adenosine monophosphate-activated protein kinase (AMPK) and dependent cellular signaling and so forth. The decreased capacity to maintain the Na-K pump at the sarcolemma, the accumulation of metabolites such as lactate, ammonia, and inorganic phosphate, and the drop in creatine phosphate (CrP) levels could cause not only fatigue in the skeletal muscle, but they are also important initiators of adaptive response. This is very much true for reactive oxygen and nitrogen species (RONS) as well, which are produced at significant levels during high-intensity exercise (Radak et al., 2008a,2011, 2013). Single bouts of exercise with low and moderate intensity and long duration would cause increases in the mRNA expression of proteins involved in aerobic metabolism of sugars and fatty acids. Single bouts of exercise with low intensity can readily cause dehydration and increased body temperature. Blood glucose and muscle glycogen content could be significantly decreased. There are overlapping signaling pathways of high and low-intensity exercise bouts on various physiological processes, such as improving insulin sensitivity, regulation of mitochondrial network, etc. The release of microRNA-s from skeletal muscle to the circulation is also dependent on the intensity of exercise. Hence, resulting in different adaptive responses (Ramos et al., 2018; Torma et al., 2020).
It is very important to understand that exercise-induced adaptation is systemic. Lack of adaptation or moderate level of adaptation in one organ or one physiological function would not mean that other organs or functions would not benefit from exercise training. The most beneficial training load could be easily different for skeletal muscle, brain, the gastro-intestinal track, or the immune system. Hence, the term of non-responders should be used with caution and just referred to a given organ, cell type, molecular signaling, or function.
Physical exercise impacts the immune system via complex regulations, which involve proper adjustment of pro- and anti-inflammatory cytokine, and neopterin production (Scheffer and Latini, 2020). It is suggested that exercise-induced elevation of proinflammatory interleukin 6 (IL-6) even can block tumor necrosis factor alpha and attenuate IL-1β signaling, hence exhibiting anti-inflammatory effects (Pedersen, 2017). It also has been shown that exercise-induced modulation of metabolism alters proinflammatory responses in macrophages and the modulation can involve the energy sensor, AMPK (Nieman and Pence, 2020). Sirtuin 1 (SIRT1), the activity of which and its contents readily respond to exercise training (Radak et al., 2020) also promotes anti-inflammatory and tolerance programs in multiple immune cell types (Yoshizaki et al., 2010). Metabolites, which are the product of catabolic processes of proteins, carbohydrates, and fats are involved in immune defense and acute phase responses, complement activation, and humoral responses mediated by circulating immunoglobulins (Nieman and Pence, 2020). It is suggested that following a single bout of exercise, the immune system efficiency decreases, which provides an open window, which increases the chance of upper respiratory diseases (Kakanis et al., 2010). High-intensity exercise appears to increase the risk of upper respiratory track-related infections (Wang et al., 2012) and extreme exercise loads can also lead to the temporary weekend immune system (Simpson et al., 2020).
Indeed, the immune response is intensity dependent, since a single bout of high-intensity exercise is associated with a greater acute phase leukocyte count and redox response than aerobic exercise (Jamurtas et al., 2018). However, T lymphocyte and monocyte are important parts of the immune system, due to their angiogenic potential they are also important contributors of adaptive response by initiating to blood vessel growth and repair. It has been shown that a single bout of high-intensity exercise results in greater elevation of T lymphocyte and angiogenic Tie2-expressing monocytes than low-intensity acute exercise (O’Carroll et al., 2019).
Hormonal secretions can be changed by a single bout of exercise (Kraemer et al., 2020; Luse et al., 2020), which, due to the short time period, might not cause a long term adaptive response, but could be important to sharpen the sensitivity of receptors and initiate signaling processes. It has been shown that high-intensity exercise can increase the level of circulating anabolic hormones to a greater degree than high volume training (Wahl et al., 2013). Moreover, the effects of exercise on insulin sensitivity are also well described (DiMenna and Arad, 2021). A single bout of exercise, due to the altered regulation of blood supply, could readily cause ischemia in the liver, kidney, and gastro-intestinal track and affect the bacterial flora of the microbiome (Radak et al., 2019). Moreover, a single bout of exercise increases the levels of circulating microRNA (miR) (Denham and Prestes, 2016). These skeletal muscle-originated myo-miRs play a significant role in acute exercise-associated miR elevation. MiR(s) also provide a further control of translation, since they can readily lead to degradation of targeted mRNA (s) and thus prevent protein synthesis. Due to the systemic effects of exercise, oxygen and energy supply of the brain can be altered, which could cause modulation of neurotrophins, lactate uptake, etc. (Radak et al., 2019). Acute exercise can lead to increases in the level of circulating brain-derived neurotrophic factor (BDNF), and the results of animal studies have revealed that BDNF is increased following a single bout of exercise in the hippocampus of rats (Shahandeh et al., 2013).
When resistance exercise is done, acute resistance exercise with high muscle tension can cause damage to sarcomeres, which might be important in satellite cell proliferation, that can later on lead to myonuclear accretion (Damas et al., 2018). Moreover, high-intensity single exercise bouts activate phosphorylate transcription factors that, after repeated stimulation (which happens during chronic exercise), leads to increased muscle mass. When mouse skeletal muscle was treated with 50 high-intensity eccentric exercise, it turned out that serum response factor (SRF) activity is linked to a histone modification cascade starting with the phosphorylation of serine 10 on histone 3 (H3S10ph) (Solagna et al., 2020). The phosphorylation of histone can lead to increased protein synthesis which requires mitogen- and stress-activated kinase 1 (Solagna et al., 2020). The acute response of high-intensity exercise also causes phosphorylation of myocardin−related transcription factors on Ser66 (Solagna et al., 2020). The reversible modification of histone by acute exercise indicates that repeated exercise causes epigenetic modifications. Indeed, the effects of acute straight line running and running with 180-degree change of direction (mimicking ball game running) were studied on the DNA methylation of skeletal muscle and results revealed that overlapping methylation of many genes, and exercise specific methylation were also observed (Maasar et al., 2021). Acute exercise results decreased methylation of the whole genome, which could be an important step to initiate exercise-induced gene activation (Barres et al., 2012). It is important to note that the degree of the methylation of peroxisome proliferator-activated receptor gamma, coactivator 1 α (PGC-1 α), pyruvate dehydrogenase kinase, isoenzyme 4 (PDK4), and peroxisome proliferator-activated receptor δ (PPAR-δ), promoter regions were dependent on the intensity of a single bout of exercise (Barres et al., 2012), which further supports the idea that exercise-induced adaptation is dependent on the intensity of the exercise. The methylation of other organs is also modified by a single bout of exercise. It has been shown that acute restraint stress decreases global DNA methylation in the hippocampus, cortex, and periaqueductal gray in brain of rats, and this alteration was attenuated by a single bout of exercise, suggesting that exercise has the potential to modulate changes in DNA methylation and gene expression (Rodrigues et al., 2015).
The cellular and systemic responses to exercise with different intensities and durations are different. One of the well-known concepts of exercise-induced adaptation is shown in Figure 1. There is no reason to believe that a single bout of exercise, with over a certain intensity and duration, does not cause some of the biochemical, and physiological changes, which are the initiative of long-term adaptive responses. However, there is no guarantee that chronic training is always associated with enhanced performance.
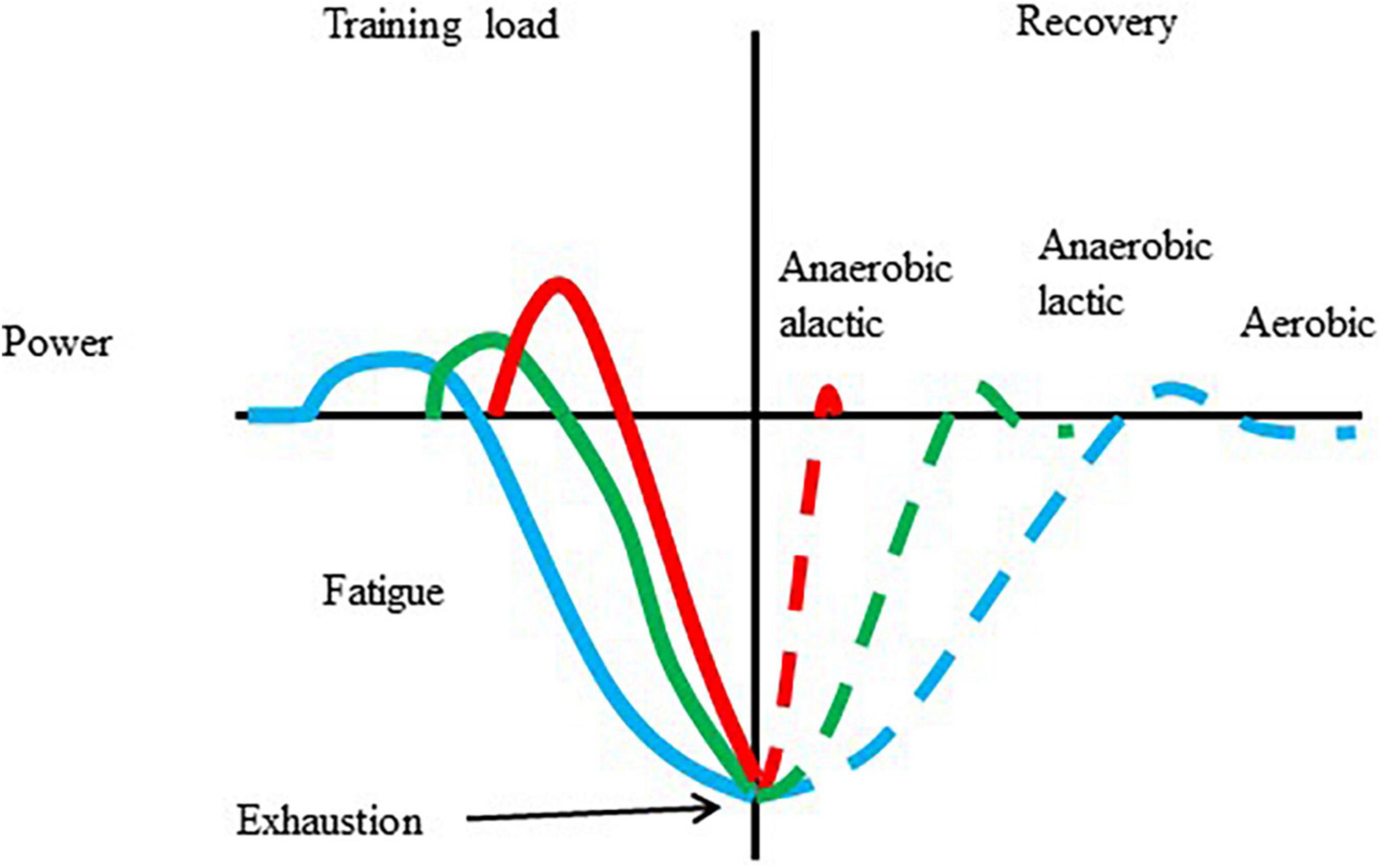
Figure 1. Adaptation to a single bout of exercise. Exercise results in increased metabolism, and power, which, after repeats can lead to fatigue and exhaustion. The length of recovery is dependent on the type of exercise that caused the fatigue and the level of fatigue. During the recovery periods overcompensation is possible.
Adaptation to Long Term Exercise
The adaptation to long-term exercise is much more complex than the biochemical, and physiological responses to a single bout of exercise. Depending on the level of physical fitness, 4–12 weeks of training, 3–20 training sessions a week, with 30 to 180 min duration, could be necessary to improve sport performance. In order to increase the performance from a high level of physical fitness, one has to work very hard. Even daily 4–8 h of training is not exceptional for certain sports. This period of repeated training sessions with rest periods can lead to the synthesis of training intensity/duration-dependent targeted proteins and related improvement of targeted biochemical and physiological processes, including metabolism, receptor sensitivity, regulation of autonomic nervous system, and so forth.
Overall, the targeted reversible changes in histone and DNA modifications, the activation of signaling pathways, microRNA, and mRNA expressions that are observed as a result of a single bout of exercise, turn into production of proteins, alteration of neuro-endocrine regulation, and the immune system leads to improved physiological function and altered phenotype following repeated cyclic training loads (Denham et al., 2014; Howlett and McGee, 2016; da Silva et al., 2017; Quan et al., 2020). Regular exercise is carried out to bring about functional changes although in a different manner in most of the organs.
On the other hand, it is also clear that long-term adaptation is not a linear process. Even with the best personalized training loads, there are periods with the absence of increased performance, which can last for weeks, months, or even for years (Figure 2). Plateaus are a normal part of the adaptive response, and the presence of a plateau does not mean that athletes are non-responders.
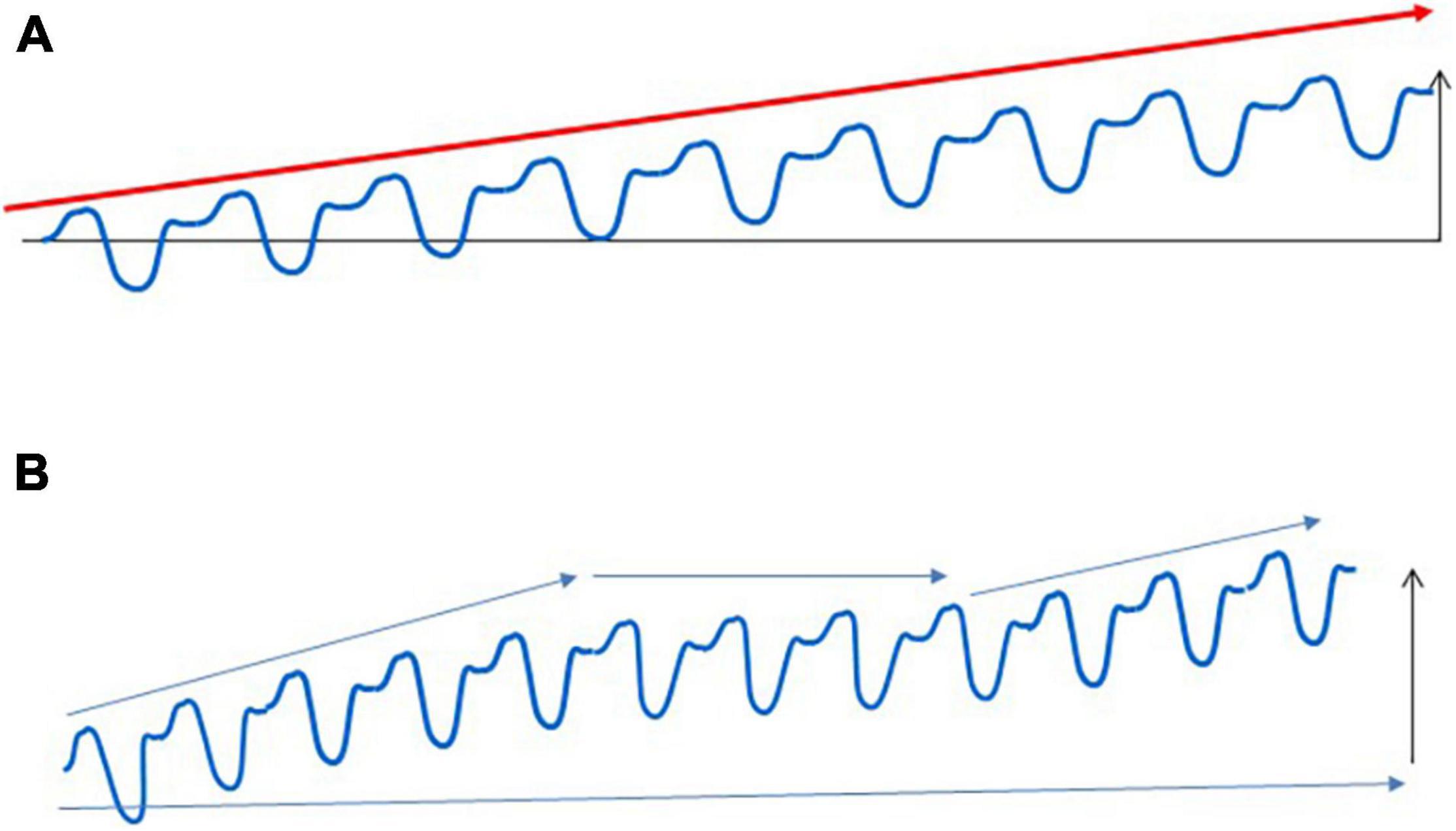
Figure 2. Adaptation to regular exercise bouts. Regular exercise does not cause a linear improvement in sport performance, which is shown in (A). Long-term exercise training generally leads to increased performance for the untrained, but the adaptive response of trained subjects contains many periods with unchanged, sometimes even decreased performance (B). These adaptive plateaus are a normal part of the adaptive response, and athletes whose preparation contains these plateaus cannot be regarded as non-responders.
Among other influencing factors, adaptation is mainly dependent on the appropriate loading and resting cycles. It is generally accepted, that the improvement of those performance influencing factors is most meaningful, and the most significantly limiting factor for the performance. If we take an example of the athlete with an 8 L/min cardiac output, and 70% type I fibers in the significantly working skeletal muscle groups of his/her event, and whose training plan contains only moderate-intensity exercise loads, he/she might easily plateau during preparation, while this kind of training could benefit the same size of athletes with 6 L/min cardiac output. Sprint interval training (SIT) sessions or HIIT, on the other hand, could be quite useful to the 8 L mentioned athlete above, since these training sessions have very significant effects on muscle metabolism. It is also known that, due to the different recruiting thresholds of type I and type IIB and IIx fibers, the mitochondrial mass is increased by intensity and duration-dependent manner (Bishop et al., 2014). Therefore, if the given training intensity/duration is not tailored to the person it is easy to accept that the training does not cause measurable increase in the performance, and we can call those individuals non-responders. Given the enormous resources available to sport science it is not very difficult to design individual tailored training programs, to minimize the duration of plateaus and avoid non-responding training periods. It is important to note that exercise-induced adaptation is systemic, yet the question arises whether non-responders are non-responders in a systemic sense or simply in some physiological function.
Trainability
It has been known for a long time that the same training program can result in different adaptive responses to different individuals and this observation was the basis for the development of personalized training loads. Interestingly, the training load which is used in most of the reported training studies is the same for all subjects. This can possibly explain the great variability of different training responses. Each subject participating in these studies could have a very different training experience, related to his/her inherited genetic setting, with different limits that influence training responses to the given load. As explained earlier, the ratio of fiber types could significantly influence the increase of mitochondrial mass to a given endurance training program, and the mitochondrial mass could influence VO2max, which often is used as a measure of trainability in endurance events. An animal model was recently introduced to study trainability (Koch et al., 2013). This model was set up using a genetically heterogeneous rat population (N/NIH stock) to develop lines named low response trainers (LRT) and high response trainers (HRT). Selection was based on the change in maximal running distance evaluated by a treadmill-running test to exhaustion. In the untrained condition, LRT and HRT rats were similar for exercise capacity. However, after receiving 9 weeks of a standard amount of endurance training, HRT rats improved, on average, by 200 meters for distance run whereas those bred as LRT failed to improve and, on average, declined in running capacity by 65 meters (Koch et al., 2013). We tested the different adaptive response of LRT and HRT rats after 3 month of endurance training at 70% of VO2max (Marton et al., 2015). We found that the alterations in the levels of VO2max, RONS, SIRT1, NAD (+)/NADH ratio, proteasome (R2 subunit), and mitochondrial network-related proteins such as mitochondrial fission protein 1 (Fis1) and mitochondrial fusion protein (Mfn1) were not related to trainability for these rats. However, data suggested that PGC1-α, nuclear respiratory factor 1 (NRF1), mitochondrial transcription factor A (TFAM), and Lon protease might be linked to trainability to the given exercise protocol. These results further suggest that HIIT training could possibly be more effective to train the LRT group, since HIIT more readily induced PGC1-α, NRF1, TFAM than aerobic exercise (Williams et al., 2019). The lack of non-personalized training, therefore, may be one of the reasons for the lack of a training response.
Nutritional habits could directly impact trainability. One interesting example is the possible effect of antioxidant supplementation on performance. The general belief is that antioxidants cannot directly improve exercise performance but could play an important role in preventing or attenuating exercise-induced muscle damage (Sureda et al., 2014; Decroix et al., 2018; Nocella et al., 2019). However, research-based opinions suggest pro and contra roles on the effects of antioxidant supplementation on adaptive responses. RONS are important signaling molecules of exercise-induced adaptation (Davies et al., 1982; Radak et al., 2008b; Torma et al., 2020), but is it possible that Vitamin C and E cocktails eliminate the systemic effects of exercise? This is certainly not the case. Antioxidant supplementation could down-regulate some cellular signaling processes (Gomez-Cabrera et al., 2008) and it has been shown that although the VO2max of the subjects increased from 41.2 to 45.6 ml/kg/min after 9 weeks exercise with daily 1 g of Vitamin C supplementation the increases were not significant, while untreated groups showed significant increases (Gomez-Cabrera et al., 2008). Therefore, the Vitamin C supplementation attenuated the effects of exercise, in a given group, but this did not eliminate or curb the beneficial effects. Indeed, it seems unlikely that the complex effects of exercise can be blocked by antioxidant supplementation (Higashida et al., 2011). The beneficial effect or the possible attenuation of exercise-induced adaptation of antioxidant supplementation could be dependent on the timing of supplementation and the level of physical fitness (Radak et al., 2017). A great number of elite athletes use antioxidant supplementation in order to support exercise performance. However, it seems improbable that antioxidant supplementation would increase or decrease exercise performance to a measurable degree (Reid, 2016; Bowtell and Kelly, 2019; Higgins et al., 2020; Arazi and Eghbali, 2021). According to our present knowledge, it seems unlikely that the antioxidant supplementation could create a non-responding group to exercise training.
What about trainability and resistance training? It is clear from the literature that protein uptake can directly influence the rate of muscle metabolism and, up to a degree, the development of muscle hypertrophy (Morton et al., 2018a). However, the intensity and duration of resistance training are the main factors of exercise-induced muscle plasticity. Results suggest that sensitivity of tension-mediated signaling pathways and the number of androgen receptors could make the difference between responders and non-responders (Morton et al., 2018b). Moreover, it has also been suggested that the difference in biogenesis of ribosomes (Figueiredo et al., 2015) and satellite cell proliferation capacity (Petrella et al., 2008) could be factors. Knocking out the paired box 7 (Pax7) gene, which is often used to identify satellite cells, results in muscle weakness and early death (Kuang et al., 2006). The difference in the expression patterns of miR (s) (Davidsen et al., 2011; Ogasawara et al., 2016) could also account for the different training responses to resistance training. All of these training adaptation limiting factors could be the result of a different genetic setting, in other words, the adaptation to training is very individual including epigenetic modifications that are due to exercise training, nutritional habits, and other lifestyle and environmental factors (Voisin et al., 2015).
One of the exercise-dependent controlling factors of adaptation is the methylation of DNA and post-translational modification of histone residues. Hypo-and hyper-methylation of CpG island of the promoter regions of genes can activate and silence transcription and directly alter adaptive responses. A single bout of exercise results in hypomethylation of whole DNA, and the promoter region of some genes which are important in exercise-induced adaptation (Barres et al., 2012), suggesting a regulatory role of methylation in trainability. Due to modulating role of exercise on methylation, the plasticity of this system makes it possible to convert non-responders to responders and vice versa. The adaptability or trainability to a given exercise program is initiated by methylation-controlled transcription, followed by translation. This notion is supported by the observation that the short-chain fatty acid, butyrate, which is produced at an elevated rate by the gut microbiome is an adaptive response to exercise training (Abraham et al., 2019) can readily change DNA methylation in fibroblasts (Parker et al., 1986). Although the direct evidence that methylation readily impacts trainability is rare, the health-associated consequences of exercise modulated DNA methylation are well known. It turns out that regular exercise hypermethylates the TRIM59 gene, which is a powerful oncogene, and KLF14 genes, which regulates inflammation (Spolnicka et al., 2018). The downregulation of these genes is associated with the anti-tumor and anti-inflammatory activities of regular exercise (Spolnicka et al., 2018).
Trainability is a complex phenomenon because exercise-induced adaptation is systemic (Figure 3). Most trainability studies focus on the adaptive response to the targeted training. However, adaptation in organs distant from skeletal muscle could significantly affect sport performance and health. The exercise-induced adaptation of liver directly affects exercise performance because liver controls lactate (Proia et al., 2016), carbohydrate, fat, and protein metabolism, partly by the generation of hepatokines such as Fibroblast Growth Factor 21, Fetuin-A, Angiopoietin-like protein 4, and Follistatin (Ennequin et al., 2019). Glomerular adaptation to exercise training, which involves filtration of lactate, Na+ and K+ and the adjustment of pH and hydration also directly alters exercise performance (Mallie et al., 2002). The adaptive response of training could lead to increased contractile response to sympathetic agonists of renal arteries as a result of a significantly reduced blood flow during exercise bouts (Kocer et al., 2011). Moreover, the systemic effects of adaptation to exercise training cover the adaptive response of the microbiome as well. It has been shown that endurance exercise resulted in elevation of the relative abundance of Veillonella atypica in the gut microbiome (Scheiman et al., 2019). This bacterium converts fatigue-inducing lactate into energy-providing propionate, and hence, supports endurance performance (Scheiman et al., 2019). At the moment we don’t have enough information on how the intensity of exercise training influences microbiome and the relative abundance of different bacterial strains. It is known that very long term exhaustive exercise can easily cause gut ischemia and gastrointestinal problems (de Oliveira and Burini, 2009), which most probably would differently alter the microbiome status of the gastrointestinal track. Using Zucker rats it was found that although HIIT was more effective to decrease epididymal fat mass than moderate-intensity exercise, the microbiome contents did not differ significantly (Maillard et al., 2019).
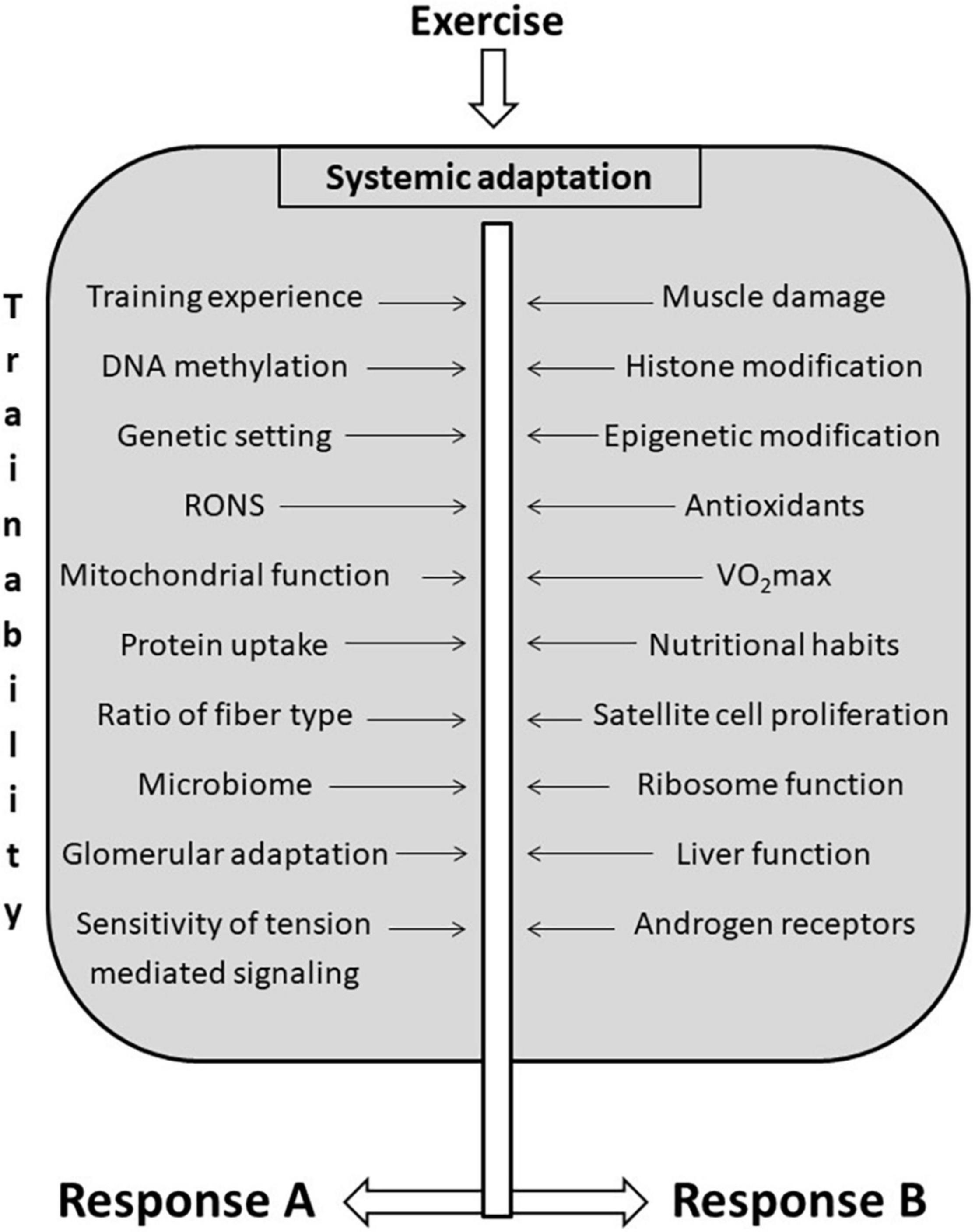
Figure 3. Factors that influencing trainability. There are a great number of factors that influence trainability of different organs. Exercise-induced adaptation is systemic, and the same training load could cause different adaptive responses in different organs indicated as (A,B), to different functions, and to different individuals.
The repeated exercise bouts associated with energy demands result in adaptive responses to liver, increasing fat oxidation, which serves a protective role against fatty liver diseases (Shephard and Johnson, 2015). Regular exercise beneficially affects the function of kidneys by maintaining mitochondrial function and suppressing inflammation (Radak et al., 2020). Regular exercise can easily lead to increased brain-derived neurotrophic factor (BDNF) concentration in the brain, and elevated BDNF beneficially affects brain plasticity, memory, mood, and viability of neurons (Gomez-Pinilla and Hillman, 2013; Kujach et al., 2019; Quan et al., 2020).
The present paper intends to show the complexity of exercise-induced systemic adaptation and point out how difficult it could be to divide the subjects into responders and non-responders, when the whole body is responding. Despite the systemic adaptation, the organ-dependent adaptation varies a lot at different intensities and loads, and the so-called optimal training load is different for different organs. Overall, trainability is considered to be the adaptive response of the targeted condition. Nonetheless, it must be kept in mind that the exercise performance is dependent on the response of many organs and a large range of influencing factors. The lack of or attenuated improvement of, the targeted condition to exercise training, might have many causes including adaptive plateau, improper loading, genetic limitation, epigenetic alteration, and so forth.
Limitation of the Study
One of the great limitations is the complexity of trainability, which appears to be very individual, and as of it, uniform approaches can provide limited information. Indeed, vast range of trainability studies used only one exercise protocol to all subjects without pointing out the individual limiting factor (s) of the targeted training goal. Therefore, the review of the results of these studies probably cannot provide realistic data on trainability. There is a huge limitation to gain functional results of heart, brain, liver, kidney, immune system, microbiome, and other organs especially in human studies, however, these elements can directly or indirectly affect trainability. The other limiting factor could be the possible plasticity of trainability, as we change one limiting factor the following limiting factor could be changed by very different training and the possible interactions are not well known. The present paper could just show a small part of trainability, which is part of an extremely complex adaptive response to exercise.
Future Perspectives
Appropriate testing of physiological functions of different systems and organs, like muscle and cardiovascular system and brain, before and after different training loads would be important to better understanding exercise-induced adaptive response. Moreover, using biomarkers to assess liver-, kidney-, and immune function along with the status of the microbiome would also add a lot to understanding the enigma of trainability.
Author Contributions
Both authors listed have made a substantial, direct, and intellectual contribution to the work, and approved it for publication.
Funding
This study was supported by the National Excellence Program (126823) and Scientific Excellence Program TKP2020-NKA-17 at the University of Physical Education, Innovation and Technology Ministry, Hungary (grants awarded to ZR).
Conflict of Interest
The authors declare that the research was conducted in the absence of any commercial or financial relationships that could be construed as a potential conflict of interest.
Publisher’s Note
All claims expressed in this article are solely those of the authors and do not necessarily represent those of their affiliated organizations, or those of the publisher, the editors and the reviewers. Any product that may be evaluated in this article, or claim that may be made by its manufacturer, is not guaranteed or endorsed by the publisher.
Acknowledgments
We are thankful to Tibor Hortobagyi, University Groningen, for his valuable comments.
References
Abraham, D., Feher, J., Scuderi, G. L., Szabo, D., Dobolyi, A., Cservenak, M., et al. (2019). Exercise and probiotics attenuate the development of Alzheimer’s disease in transgenic mice: role of microbiome. Exp. Gerontol. 115, 122–131. doi: 10.1016/j.exger.2018.12.005
Arazi, H., and Eghbali, E. (2021). Possible effects of beetroot supplementation on physical performance through metabolic, neuroendocrine, and antioxidant mechanisms: a narrative review of the literature. Front. Nutr. 8:660150. doi: 10.3389/fnut.2021.660150
Barres, R., Yan, J., Egan, B., Treebak, J. T., Rasmussen, M., Fritz, T., et al. (2012). Acute exercise remodels promoter methylation in human skeletal muscle. Cell Metab. 15, 405–411. doi: 10.1016/j.cmet.2012.01.001
Bishop, D. J., Granata, C., and Eynon, N. (2014). Can we optimise the exercise training prescription to maximise improvements in mitochondria function and content? Biochim. Biophys. Acta 1840, 1266–1275. doi: 10.1016/j.bbagen.2013.10.012
Bouchard, C., and Rankinen, T. (2001). Individual differences in response to regular physical activity. Med. Sci. Sports Exerc. 33, S446–S451. doi: 10.1097/00005768-200106001-00013
Bowtell, J., and Kelly, V. (2019). Fruit-derived polyphenol supplementation for athlete recovery and performance. Sports Med. 49, 3–23. doi: 10.1007/s40279-018-0998-x
da Silva, I. R. V., de Araujo, C. L. P., Dorneles, G. P., Peres, A., Bard, A. L., Reinaldo, G., et al. (2017). Exercise-modulated epigenetic markers and inflammatory response in COPD individuals: a pilot study. Respir. Physiol. Neurobiol. 242, 89–95. doi: 10.1016/j.resp.2017.04.004
Damas, F., Libardi, C. A., and Ugrinowitsch, C. (2018). The development of skeletal muscle hypertrophy through resistance training: the role of muscle damage and muscle protein synthesis. Eur. J. Appl. Physiol. 118, 485–500. doi: 10.1007/s00421-017-3792-9
Davidsen, P. K., Gallagher, I. J., Hartman, J. W., Tarnopolsky, M. A., Dela, F., Helge, J. W., et al. (2011). High responders to resistance exercise training demonstrate differential regulation of skeletal muscle microRNA expression. J. Appl. Physiol. (1985) 110, 309–317. doi: 10.1152/japplphysiol.00901.2010
Davies, K. J., Quintanilha, A. T., Brooks, G. A., and Packer, L. (1982). Free radicals and tissue damage produced by exercise. Biochem. Biophys. Res. Commun. 107, 1198–1205. doi: 10.1016/s0006-291x(82)80124-1
de Oliveira, E. P., and Burini, R. C. (2009). The impact of physical exercise on the gastrointestinal tract. Curr. Opin. Clin. Nutr. Metab. Care 12, 533–538. doi: 10.1097/MCO.0b013e32832e6776
Decroix, L., Soares, D. D., Meeusen, R., Heyman, E., and Tonoli, C. (2018). Cocoa flavanol supplementation and exercise: a systematic review. Sports Med. 48, 867–892. doi: 10.1007/s40279-017-0849-1
Denham, J., Marques, F. Z., O’Brien, B. J., and Charchar, F. J. (2014). Exercise: putting action into our epigenome. Sports Med. 44, 189–209. doi: 10.1007/s40279-013-0114-1
Denham, J., and Prestes, P. R. (2016). Muscle-enriched microRNAs isolated from whole blood are regulated by exercise and are potential biomarkers of cardiorespiratory fitness. Front. Genet. 7:196. doi: 10.3389/fgene.2016.00196
DiMenna, F. J., and Arad, A. D. (2021). The acute vs. chronic effect of exercise on insulin sensitivity: nothing lasts forever. Cardiovasc. Endocrinol. Metab. 10, 149–161. doi: 10.1097/XCE.0000000000000239
Ennequin, G., Sirvent, P., and Whitham, M. (2019). Role of exercise-induced hepatokines in metabolic disorders. Am. J. Physiol. Endocrinol. Metab. 317, E11–E24. doi: 10.1152/ajpendo.00433.2018
Figueiredo, V. C., Caldow, M. K., Massie, V., Markworth, J. F., Cameron-Smith, D., and Blazevich, A. J. (2015). Ribosome biogenesis adaptation in resistance training-induced human skeletal muscle hypertrophy. Am. J. Physiol. Endocrinol. Metab. 309, E72–E83. doi: 10.1152/ajpendo.00050.2015
Gomez-Cabrera, M. C., Domenech, E., Romagnoli, M., Arduini, A., Borras, C., Pallardo, F. V., et al. (2008). Oral administration of vitamin C decreases muscle mitochondrial biogenesis and hampers training-induced adaptations in endurance performance. Am. J. Clin. Nutr. 87, 142–149. doi: 10.1093/ajcn/87.1.142
Gomez-Pinilla, F., and Hillman, C. (2013). The influence of exercise on cognitive abilities. Compr. Physiol. 3, 403–428. doi: 10.1002/cphy.c110063
Higashida, K., Kim, S. H., Higuchi, M., Holloszy, J. O., and Han, D. H. (2011). Normal adaptations to exercise despite protection against oxidative stress. Am. J. Physiol. Endocrinol. Metab. 301, E779–E784. doi: 10.1152/ajpendo.00655.2010
Higgins, M. R., Izadi, A., and Kaviani, M. (2020). Antioxidants and exercise performance: with a focus on vitamin E and C supplementation. Int. J. Environ. Res. Public Health 17:8452. doi: 10.3390/ijerph17228452
Hortobagyi, T., Deak, D., Farkas, D., Blenyesi, E., Torok, K., Granacher, U., et al. (2021). Effects of exercise dose and detraining duration on mobility at late midlife: a randomized clinical trial. Gerontology 67, 403–414. doi: 10.1159/000513505
Howlett, K. F., and McGee, S. L. (2016). Epigenetic regulation of skeletal muscle metabolism. Clin. Sci. (Lond) 130, 1051–1063. doi: 10.1042/CS20160115
Hrubeniuk, T. J., Bouchard, D. R., Gurd, B. J., and Senechal, M. (2021). Can non-responders be ‘rescued’ by increasing exercise intensity? A quasi-experimental trial of individual responses among humans living with pre-diabetes or type 2 diabetes mellitus in Canada. BMJ Open 11:e044478. doi: 10.1136/bmjopen-2020-044478
Jamurtas, A. Z., Fatouros, I. G., Deli, C. K., Georgakouli, K., Poulios, A., Draganidis, D., et al. (2018). The effects of acute low-volume HIIT and aerobic exercise on leukocyte count and redox status. J. Sports Sci. Med. 17, 501–508.
Kakanis, M. W., Peake, J., Brenu, E. W., Simmonds, M., Gray, B., Hooper, S. L., et al. (2010). The open window of susceptibility to infection after acute exercise in healthy young male elite athletes. Exerc. Immunol. Rev. 16, 119–137.
Kocer, G., Kuru, O., Gunduz, F., Bayram, Z., Ozdem, S., Aksoy, D., et al. (2011). The effect of exercise training on the responsiveness of renal resistance arteries in rats. Ren. Fail 33, 587–592. doi: 10.3109/0886022X.2011.585000
Koch, L. G., Pollott, G. E., and Britton, S. L. (2013). Selectively bred rat model system for low and high response to exercise training. Physiol. Genomics 45, 606–614. doi: 10.1152/physiolgenomics.00021.2013
Kraemer, W. J., Ratamess, N. A., Hymer, W. C., Nindl, B. C., and Fragala, M. S. (2020). Growth hormone(s), testosterone, insulin-like growth factors, and cortisol: roles and integration for cellular development and growth with exercise. Front. Endocrinol. (Lausanne) 11:33. doi: 10.3389/fendo.2020.00033
Kuang, S., Charge, S. B., Seale, P., Huh, M., and Rudnicki, M. A. (2006). Distinct roles for Pax7 and Pax3 in adult regenerative myogenesis. J. Cell Biol. 172, 103–113. doi: 10.1083/jcb.200508001
Kujach, S., Olek, R. A., Byun, K., Suwabe, K., Sitek, E. J., Ziemann, E., et al. (2019). Acute sprint interval exercise increases both cognitive functions and peripheral neurotrophic factors in humans: the possible involvement of lactate. Front. Neurosci. 13:1455. doi: 10.3389/fnins.2019.01455
Luse, M. A., Heiston, E. M., Malin, S. K., and Isakson, B. E. (2020). Cellular and functional effects of insulin based therapies and exercise on endothelium. Curr. Pharm. Des. 26, 3760–3767. doi: 10.2174/1381612826666200721002735
Maasar, M. F., Turner, D. C., Gorski, P. P., Seaborne, R. A., Strauss, J. A., Shepherd, S. O., et al. (2021). The comparative methylome and transcriptome after change of direction compared to straight line running exercise in human skeletal muscle. Front. Physiol. 12:619447. doi: 10.3389/fphys.2021.619447
Maillard, F., Vazeille, E., Sauvanet, P., Sirvent, P., Combaret, L., Sourdrille, A., et al. (2019). High intensity interval training promotes total and visceral fat mass loss in obese Zucker rats without modulating gut microbiota. PLoS One 14:e0214660. doi: 10.1371/journal.pone.0214660
Mallie, J. P., Ait-Djafer, Z., Saunders, C., Pierrat, A., Caira, M. V., Courroy, O., et al. (2002). Renal handling of salt and water in humans during exercise with or without hydration. Eur. J. Appl. Physiol. 86, 196–202. doi: 10.1007/s00421-001-0524-x
Marton, O., Koltai, E., Takeda, M., Koch, L. G., Britton, S. L., Davies, K. J., et al. (2015). Mitochondrial biogenesis-associated factors underlie the magnitude of response to aerobic endurance training in rats. Pflugers Arch. 467, 779–788. doi: 10.1007/s00424-014-1554-7
Mattioni Maturana, F., Soares, R. N., Murias, J. M., Schellhorn, P., Erz, G., Burgstahler, C., et al. (2021). Responders and non-responders to aerobic exercise training: beyond the evaluation of VO2max. Physiol. Rep. 9:e14951. doi: 10.14814/phy2.14951
Maturana, F. M., Schellhorn, P., Erz, G., Burgstahler, C., Widmann, M., Munz, B., et al. (2021). Individual cardiovascular responsiveness to work-matched exercise within the moderate- and severe-intensity domains. Eur. J. Appl. Physiol. 121, 2039–2059. doi: 10.1007/s00421-021-04676-7
McLaren, S. J., Smith, A., Bartlett, J. D., Spears, I. R., and Weston, M. (2018). Differential training loads and individual fitness responses to pre-season in professional rugby union players. J. Sports Sci. 36, 2438–2446. doi: 10.1080/02640414.2018.1461449
Morton, R. W., Murphy, K. T., McKellar, S. R., Schoenfeld, B. J., Henselmans, M., Helms, E., et al. (2018a). A systematic review, meta-analysis and meta-regression of the effect of protein supplementation on resistance training-induced gains in muscle mass and strength in healthy adults. Br. J. Sports Med. 52, 376–384. doi: 10.1136/bjsports-2017-097608
Morton, R. W., Sato, K., Gallaugher, M. P. B., Oikawa, S. Y., McNicholas, P. D., Fujita, S., et al. (2018b). Muscle androgen receptor content but not systemic hormones is associated with resistance training-induced skeletal muscle hypertrophy in healthy, young men. Front. Physiol. 9:1373. doi: 10.3389/fphys.2018.01373
Nieman, D. C., and Pence, B. D. (2020). Exercise immunology: future directions. J. Sport Health Sci. 9, 432–445. doi: 10.1016/j.jshs.2019.12.003
Nocella, C., Cammisotto, V., Pigozzi, F., Borrione, P., Fossati, C., D’Amico, A., et al. (2019). Impairment between oxidant and antioxidant systems: short- and long-term implications for athletes’ health. Nutrients 11:1353. doi: 10.3390/nu11061353
O’Carroll, L., Wardrop, B., Murphy, R. P., Ross, M. D., and Harrison, M. (2019). Circulating angiogenic cell response to sprint interval and continuous exercise. Eur. J. Appl. Physiol. 119, 743–752. doi: 10.1007/s00421-018-04065-7
Ogasawara, R., Akimoto, T., Umeno, T., Sawada, S., Hamaoka, T., and Fujita, S. (2016). MicroRNA expression profiling in skeletal muscle reveals different regulatory patterns in high and low responders to resistance training. Physiol. Genomics 48, 320–324. doi: 10.1152/physiolgenomics.00124.2015
Parker, M. I., de Haan, J. B., and Gevers, W. (1986). DNA hypermethylation in sodium butyrate-treated WI-38 fibroblasts. J. Biol. Chem. 261, 2786–2790.
Pedersen, B. K. (2017). Anti-inflammatory effects of exercise: role in diabetes and cardiovascular disease. Eur. J. Clin. Invest. 47, 600–611. doi: 10.1111/eci.12781
Petrella, J. K., Kim, J. S., Mayhew, D. L., Cross, J. M., and Bamman, M. M. (2008). Potent myofiber hypertrophy during resistance training in humans is associated with satellite cell-mediated myonuclear addition: a cluster analysis. J. Appl. Physiol. (1985) 104, 1736–1742. doi: 10.1152/japplphysiol.01215.2007
Proia, P., Di Liegro, C. M., Schiera, G., Fricano, A., and Di Liegro, I. (2016). Lactate as a metabolite and a regulator in the central nervous system. Int. J. Mol. Sci. 17:1450. doi: 10.3390/ijms17091450
Quan, H., Koltai, E., Suzuki, K., Aguiar, A. S. Jr., Pinho, R., Boldogh, I., et al. (2020). Exercise, redox system and neurodegenerative diseases. Biochim. Biophys. Acta Mol. Basis Dis. 1866:165778. doi: 10.1016/j.bbadis.2020.165778
Radák, Z. (2018a). “Adaptation, phenotypic adaptation, fatigue, and overtraining,” in The Physiology of Physical Training, ed. Z. Radák (Cambrdige: Academic Press), 33–54.
Radak, Z., Chung, H. Y., and Goto, S. (2008a). Systemic adaptation to oxidative challenge induced by regular exercise. Free Radic. Biol. Med. 44, 153–159. doi: 10.1016/j.freeradbiomed.2007.01.029
Radak, Z., Chung, H. Y., Koltai, E., Taylor, A. W., and Goto, S. (2008b). Exercise, oxidative stress and hormesis. Ageing Res. Rev. 7, 34–42. doi: 10.1016/j.arr.2007.04.004
Radak, Z., Ishihara, K., Tekus, E., Varga, C., Posa, A., Balogh, L., et al. (2017). Exercise, oxidants, and antioxidants change the shape of the bell-shaped hormesis curve. Redox Biol. 12, 285–290. doi: 10.1016/j.redox.2017.02.015
Radak, Z., Koltai, E., Taylor, A. W., Higuchi, M., Kumagai, S., Ohno, H., et al. (2013). Redox-regulating sirtuins in aging, caloric restriction, and exercise. Free Radic Biol. Med. 58, 87–97. doi: 10.1016/j.freeradbiomed.2013.01.004
Radak, Z., Suzuki, K., Posa, A., Petrovszky, Z., Koltai, E., and Boldogh, I. (2020). The systemic role of SIRT1 in exercise mediated adaptation. Redox Biol. 35:101467. doi: 10.1016/j.redox.2020.101467
Radak, Z., Torma, F., Berkes, I., Goto, S., Mimura, T., Posa, A., et al. (2019). Exercise effects on physiological function during aging. Free Radic Biol. Med. 132, 33–41. doi: 10.1016/j.freeradbiomed.2018.10.444
Radak, Z., Zhao, Z., Goto, S., and Koltai, E. (2011). Age-associated neurodegeneration and oxidative damage to lipids, proteins and DNA. Mol. Aspects Med. 32, 305–315. doi: 10.1016/j.mam.2011.10.010
Ramos, A. E., Lo, C., Estephan, L. E., Tai, Y. Y., Tang, Y., Zhao, J., et al. (2018). Specific circulating microRNAs display dose-dependent responses to variable intensity and duration of endurance exercise. Am. J. Physiol. Heart Circ. Physiol. 315, H273–H283. doi: 10.1152/ajpheart.00741.2017
Reid, M. B. (2016). Redox interventions to increase exercise performance. J. Physiol. 594, 5125–5133. doi: 10.1113/JP270653
Rodrigues, G. M. Jr., Toffoli, L. V., Manfredo, M. H., Francis-Oliveira, J., Silva, A. S., Raquel, H. A., et al. (2015). Acute stress affects the global DNA methylation profile in rat brain: modulation by physical exercise. Behav. Brain Res. 279, 123–128. doi: 10.1016/j.bbr.2014.11.023
Scheffer, D. D. L., and Latini, A. (2020). Exercise-induced immune system response: anti-inflammatory status on peripheral and central organs. Biochim. Biophys. Acta Mol. Basis Dis. 1866:165823. doi: 10.1016/j.bbadis.2020.165823
Scheiman, J., Luber, J. M., Chavkin, T. A., MacDonald, T., Tung, A., Pham, L. D., et al. (2019). Meta-omics analysis of elite athletes identifies a performance-enhancing microbe that functions via lactate metabolism. Nat. Med. 25, 1104–1109. doi: 10.1038/s41591-019-0485-4
Shahandeh, M., Roshan, V. D., Hosseinzadeh, S., Mahjoub, S., and Sarkisian, V. (2013). Chronic exercise training versus acute endurance exercise in reducing neurotoxicity in rats exposed to lead acetate. Neural Regen. Res. 8, 714–722. doi: 10.3969/j.issn.1673-5374.2013.08.006
Shephard, R. J., and Johnson, N. (2015). Effects of physical activity upon the liver. Eur. J. Appl. Physiol. 115, 1–46. doi: 10.1007/s00421-014-3031-6
Simpson, R. J., Campbell, J. P., Gleeson, M., Kruger, K., Nieman, D. C., Pyne, D. B., et al. (2020). Can exercise affect immune function to increase susceptibility to infection? Exerc. Immunol. Rev. 26, 8–22.
Solagna, F., Nogara, L., Dyar, K. A., Greulich, F., Mir, A. A., Turk, C., et al. (2020). Exercise-dependent increases in protein synthesis are accompanied by chromatin modifications and increased MRTF-SRF signalling. Acta Physiol (Oxf) 230:e13496. doi: 10.1111/apha.13496
Spolnicka, M., Pospiech, E., Adamczyk, J. G., Freire-Aradas, A., Peplonska, B., Zbiec-Piekarska, R., et al. (2018). Modified aging of elite athletes revealed by analysis of epigenetic age markers. Aging (Albany NY) 10, 241–252. doi: 10.18632/aging.101385
Sureda, A., Tejada, S., Bibiloni Mdel, M., Tur, J. A., and Pons, A. (2014). Polyphenols: well beyond the antioxidant capacity: polyphenol supplementation and exercise-induced oxidative stress and inflammation. Curr. Pharm. Biotechnol. 15, 373–379. doi: 10.2174/1389201015666140813123843
Torma, F., Gombos, Z., Jokai, M., Berkes, I., Takeda, M., Mimura, T., et al. (2020). The roles of microRNA in redox metabolism and exercise-mediated adaptation. J. Sport Health Sci. 9, 405–414. doi: 10.1016/j.jshs.2020.03.004
Voisin, S., Eynon, N., Yan, X., and Bishop, D. J. (2015). Exercise training and DNA methylation in humans. Acta Physiol. (Oxf) 213, 39–59. doi: 10.1111/apha.12414
Wahl, P., Mathes, S., Kohler, K., Achtzehn, S., Bloch, W., and Mester, J. (2013). Acute metabolic, hormonal, and psychological responses to different endurance training protocols. Horm. Metab. Res. 45, 827–833. doi: 10.1055/s-0033-1347242
Wang, J., Song, H., Tang, X., Yang, Y., Vieira, V. J., Niu, Y., et al. (2012). Effect of exercise training intensity on murine T-regulatory cells and vaccination response. Scand. J. Med. Sci. Sports 22, 643–652. doi: 10.1111/j.1600-0838.2010.01288.x
Williams, C. J., Gurd, B. J., Bonafiglia, J. T., Voisin, S., Li, Z., Harvey, N., et al. (2019). A Multi-center comparison of O2peak trainability between interval training and moderate intensity continuous training. Front. Physiol. 10:19. doi: 10.3389/fphys.2019.00019
Keywords: responders, non-responders, VO2max, resistance training, systemic adaptation
Citation: Radak Z and Taylor AW (2022) Issues on Trainability. Front. Physiol. 12:790196. doi: 10.3389/fphys.2021.790196
Received: 06 October 2021; Accepted: 31 December 2021;
Published: 24 January 2022.
Edited by:
Ricardo Ferraz, University of Beira Interior, PortugalReviewed by:
Mitsuharu Okutsu, Nagoya City University, JapanFernanda M. Silva, University of Coimbra, Portugal
Mohammad Mehedi Hasan Khan, Sylhet Agricultural University, Bangladesh
Copyright © 2022 Radak and Taylor. This is an open-access article distributed under the terms of the Creative Commons Attribution License (CC BY). The use, distribution or reproduction in other forums is permitted, provided the original author(s) and the copyright owner(s) are credited and that the original publication in this journal is cited, in accordance with accepted academic practice. No use, distribution or reproduction is permitted which does not comply with these terms.
*Correspondence: Zsolt Radak, cmFkYWsuenNvbHRAdGYuaHU=