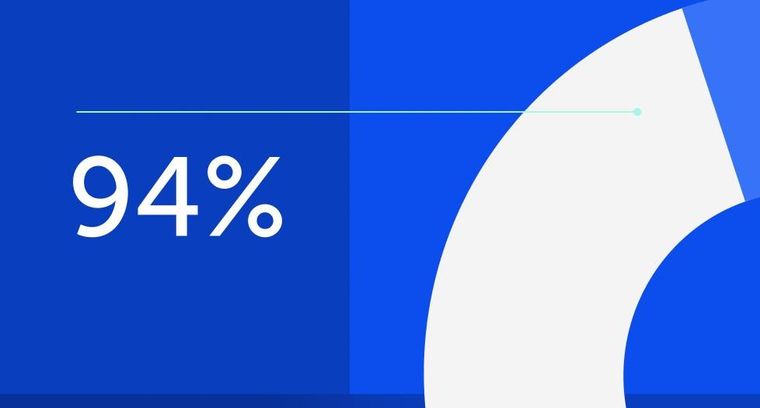
94% of researchers rate our articles as excellent or good
Learn more about the work of our research integrity team to safeguard the quality of each article we publish.
Find out more
REVIEW article
Front. Physiol., 20 January 2022
Sec. Lipid and Fatty Acid Research
Volume 12 - 2021 | https://doi.org/10.3389/fphys.2021.787848
During pregnancy, maternal plasma fatty acids are critically required for cell growth and development, cell signaling, and the development of critical structural and functional aspects of the feto-placental unit. In addition, the fatty acids modulate the early stages of placental development by regulating angiogenesis in the first-trimester human placenta. Preferential transport of maternal plasma long-chain polyunsaturated fatty acids during the third trimester is critical for optimal fetal brain development. Maternal status such as obesity, diabetes, and dietary intakes may affect the functional changes in lipid metabolic processes in maternal-fetal lipid transport and metabolism. Fatty acids traverse the placental membranes via several plasma membrane fatty acid transport/binding proteins (FAT, FATP, p-FABPpm, and FFARs) and cytoplasmic fatty acid-binding proteins (FABPs). This review discusses the maternal metabolism of fatty acids and their effects on early placentation, placental fatty acid transport and metabolism, and their roles in feto-placental growth and development. The review also highlights how maternal fat metabolism modulates lipid processing, including transportation, esterification, and oxidation of fatty acids.
Fatty acids have vital functions on energy metabolism and storage as they participate in cell enlargement, promote cell functions, regulate gene expression, coordinate intra- and extracellular communications, regulate the supply of energy substrates, and control cellular responses to the metabolic environment (Crabtree et al., 1998; Mallick et al., 2021). Fatty acids modulate membrane architecture, dynamics, and functionalities (Brash, 2001; Nakamura et al., 2001). Besides, they are functionally involved in regulating membrane ion channels and receptors and cell signaling (Kang and Leaf, 1996; Kim and Pleumsamran, 2000; Bazan, 2003; Carattino et al., 2003; Danthi et al., 2005). Linoleic acid, 18:2n-6 (LA), and alpha-linolenic acid 18:3n-3 (ALA) are the two essential fatty acids (EFAs) that belong to the n-6 and n-3 families of fatty acids. Both n-3 and n-6 fatty acids and their long-chain polyunsaturated fatty acids (LCPUFAs) play critical roles that include the structure and function of cell membranes as signaling molecules, act ligands for gene expression and maintain cellular metabolic homeostasis (Smith, 1989; Innis, 1991; Dutta-Roy, 1994). The metabolism of n-6 and n-3 EFAs is tightly regulated by the interplay of several enzymes such as desaturases, elongases, cyclooxygenases (COXs), lipooxygenases (LOXs), and cytochrome P450 system. N-3 and n-6 fatty acids metabolically produce compounds with diverse physiological and pathological functions. Eicosanoids are synthesized from n-3, and n-6 LCPUFAs include prostaglandins (PGs), thromboxanes (TXs), leukotrienes (LTs), and hydroxyeicosatetraenoic acids (HETEs). The source and metabolism of LCFAs and their various metabolites are summarized in Figures 1A,B. ARA, dihomo-γ-linolenic, or 20:3(n-6), EPA, and DHA are obtained from the diet or produced in the body from these EFA precursors. Multiple stimuli lead to the release of membrane-bound LCPUFAs such as ARA, dihomo-γ-linolenic acid,20:3n-6 (DGLA), EPA, and DHA via activation of cellular phospholipases. Cycloxygenase (COX) and lipoxygenase (LOXs) are involved in producing LCPUFA-metabolites, eicosanoids. Several LOXs act upon ARA, EPA, DGLA, and DHA positions. The docosanoids, such as protectins, resolvins, and maresins or “specialized pro-resolving mediators,” are derived from DHA. E-series resolvins are produced from EPA. The cytochrome P-450 epoxygenase pathway produces hydroxyeicosatetraenoic acids (HETEs) and epoxides (EETs). While many of the requisite enzymes, precursors, and products are specific to particular types of cells, the proximity of some cell types can facilitate the transfer of eicosanoids between cells for further metabolism, and for example, some of the leukotrienes are produced by trans-cellular mechanisms.
Figure 1. Metabolites of EPA, DHA, and ARA in the body. (A) Arachidonic acid (ARA), and eicosapentaenoic acid (EPA) are metabolized by enzymes such as cytochrome P450 (CYP), lipoxygenase (LOX), or cyclooxygenase (COX). (B) Metabolism of docosahexaenoic acid (DHA) to docosanoids and other derivatives. DHET, dihydroxyeicosatrienoic acids; HETE, hydroxyeicosatetraenoic acid; diHETE, dihydroxy eicosatetraenoic acid, PG, prostaglandin: LT, leukotrienes; diHDPA; dihydroxydocosapentaenoic acid.
Eicosanoids are involved in controlling various physiological and pathological processes in the body. Eicosanoids produced from n-6 LCPUFAs promote inflammation, tumor growth, and angiogenesis (Smith, 1989; Nie et al., 2000; Hoagland et al., 2001; Pai et al., 2001; Bagga et al., 2003; Pola et al., 2004; Kamiyama et al., 2006; Jin et al., 2009). In contrast, n-3 derived eicosanoids usually have anti-inflammatory, anti-cancer, and anti-angiogenic properties. LCPUFAs such as arachidonic acid,20:4n-6 (ARA) and docosahexaenoic acid,22:6n-3 (DHA) are involved in the development of the central nervous system, visual acuity, and cognitive functions (Budowski and Crawford, 1985; Innis, 1991; Jeffrey et al., 2001; Uauy et al., 2001). These fatty acids also regulate the expression of genes involved in fatty acids biosynthesis and oxidation, lipogenesis, glucose utilization, and insulin sensitivity, thermoregulation, energy partitioning, cholesterol transport, cholesterol synthesis, and cell growth and differentiation (Long and Pekala, 1996; Jump, 2002; Wahle et al., 2003; Wilke and Clandinin, 2005; Mallick and Duttaroy, 2021).
The fundamental roles of fatty acids as structural components and functional modulators (Duttaroy and Basak, 2020) define maternal metabolism of fatty acids is an essential factor for feto-placental development. The brain and the retina are rich in LCPUFAs such as ARA and DHA. An abundant supply of these LCPUFAs during pregnancy and the neonatal period is critical to ensure optimal brain development and functionalities (Basak et al., 2020; Dutta-Roy, 2000b). Pre-term babies have significantly lower EFA and LCPUFAs statuses than do full-term neonates. The dietary EFA deficiency has become a pertinent issue for pre-term infants’ nutrition because they do not receive the third-trimester intrauterine supply of DHA and ARA. Supplementation of pregnant mothers with LCPUFAs has improved neonatal LCPUFAs status (Judge, 2018; Middleton et al., 2018). It is concluded that LCPUFAs levels are higher in fetal than in maternal circulation via different mechanisms such as maternal lipid metabolism and preferential placental transport (Zamai et al., 2020; Basak et al., 2021). Several proteins such as cytoplasmic fatty acid-binding proteins (FABPs), plasma membrane fatty acid-binding protein (FABPpm), fatty acid translocase (FAT/CD36), fatty acid transporter proteins (FATPs), and free fatty acid receptors (FFARs) bind FFAs, whenever these are available in the extracellular medium, the cytosol, or the nuclear matrix (Duttaroy, 2009; Hara et al., 2013; Mallick et al., 2021). FFAs are taken up by cells by their membrane transport/binding proteins such as FABPpm, FAT/CD36, and FATPs. At the plasma membrane, FFAs can also activate FFARs, known as G protein-coupled receptors (GPRs) (Hara et al., 2013) while they bind FABPs in the cytosolic compartment and are processed to subcellular structures or metabolic pathways. Another essential lipid metabolic regulator is peroxisome proliferator-activated receptors (PPARs), ligand-activated transcription factors, which mediate the regulation of fatty acids in the nucleus (Zolezzi and Inestrosa, 2013). Currently, the regulatory and signaling roles of FFAs are gaining importance in physiological and pathological processes as these receptors are better characterized (Mallick et al., 2021). Figure 2 describes the schematic diagram showing proteins involved in transporting maternal fatty acids across the human placental trophoblast.
Figure 2. Schematic diagram showing the cellular proteins and their involvement in the transport of maternal fatty acids across the human placental trophoblast. The schematic overview of proteins involved in fatty acid uptake and transport in last trimester trophoblast cells. The location of FAT and FATP on both sides of the bipolar placental cells and the lack of specificity for particular types of FFAs allow transport by all fatty acids bi-directionally, i.e., from the mother to the fetus and vice versa. However, by virtue of its exclusive location on both sides and preference for ARA and DHA, p-FABPpm is thought to be involved in sequestering these maternal plasma LCPUFAs to the placenta. In addition, cytoplasmic FABPs may be responsible for the trans cytoplasmic movement of FFAs to their sites of esterification, β-oxidation, or fetal circulation via placental basal membranes. FATP, Fatty acid transporter protein; pFABPpm, Plasma membrane fatty acid-binding protein; ACBP, Acyl-CoA binding protein.
This review brings together recent developments on the metabolism of fatty acids in mothers and their impacts on fetoplacental development.
Pregnancy is generally marked with hyperlipidemia involving all lipid classes such as lipoproteins, triacylglycerols (TAGs), and free fatty acids (FFAs) (Punnonen, 1977; Darmady and Postle, 1982; Jimenez et al., 1988). The elevated lipid levels facilitate fetal access to the available fatty acids (Warth et al., 1975). The plasma levels of phospholipids increase by approximately 65% during the third trimester as compared first trimester of pregnancy (Punnonen, 1977). The fat deposition in mothers is primarily aimed to meet the lipid demand of the developing fetus. Maternal hyperlipidemia during late pregnancy plays a crucial role in maternal metabolic adaptations that are geared to benefit fetal growth. The fat mass in adipose tissue increases during pregnancy, even in malnourished mothers (Prentice and Goldberg, 2000; Herrera, 2002; Herrera et al., 2006). During fat deposition, the levels of different lipid fractions in plasma, such as lipoproteins, TAGs, phospholipids, FFAs, are increased in maternal circulation. Lowered insulin-dependent decrease in lipoprotein lipase (LPL) activity in adipose tissue and subsequent insulin resistance are observed during the pregnancy. Maternal lipolysis during gestation is regulated by insulin, glucose, progesterone, cortisol, prolactin, estrogen, adipokine, and leptin (Cousins, 1991; Catov et al., 2007). They inhibit hepatic LPL activity while increasing intestinal absorption of dietary fats (Cetin et al., 2009). These dynamic physiological changes increase the levels of circulating FFAs and glycerol, which are substrates for hepatic synthesis of very-low-density lipoproteins (VLDL). Adipose mass and hyperlipidemia are elevated in pregnant mothers, while the requirement of LCPUFAs is accelerated for fetal growth and development. The rapid increase in adipose mass is the result of both hyperphagia (Murphy and Abrams, 1993) and increased lipid synthesis driven by the enhanced adipose tissue insulin sensitivity during early pregnancy (Ramos et al., 2003). During early pregnancy, there is unchanged or even increased adipose tissue LPL activity. The LPL enzyme is extrahepatic, mainly active in adipose tissue, and catalyzes the hydrolysis of circulating TAGs in TAG-rich lipoproteins such as chylomicrons and VLDLs (Mead et al., 2002). The hydrolytic products, FFAs and 2-monoacylglycerol or glycerol, are taken up by the subjacent tissue for re-synthesis of TAGs. These changes facilitate the accumulation of circulating lipids in maternal fat depots. However, the increased accumulation of maternal adipose depot stops during the third trimester of pregnancy due to decreased adipose fatty acid synthesis (Ramos et al., 2003), and reduced LPL activity. The reduced activity of these enzymes causes a reduction in the hydrolysis and tissue uptake of TAGs in TAG-rich lipoproteins and contributes to the development of maternal hypertriglyceridemia; and increased adipose lipolytic activity (Elliott, 1975). The insulin resistance in the last trimester of pregnancy (Dahlgren, 2006; Barbour et al., 2007) contributes to these changes (Sivan et al., 1999). Pregnancy-specific hormones have insulin-antagonistic and lipolytic effects (Ryan and Enns, 1988). Increased blood levels of human placental lactogen, placentally derived human growth hormone, human chorionic gonadotropin, progesterone, cortisol, prolactin, and other hormones increase insulin resistance in peripheral tissues such as adipocytes and skeletal muscle (Newbern and Freemark, 2011). Increased levels of progesterone, cortisol and other hormones markedly decreased insulin sensitivity during the second trimester onward, with the highest insulin resistance noted at third trimester (Di Cianni et al., 2003). In addition, changes in the production of inflammatory mediators by the placenta and cytokines produced by adipose decrease insulin sensitivity (Di Cianni et al., 2003; Desoye and Hauguel-De Mouzon, 2007; Lain and Catalano, 2007). Central leptin resistance leads to an increased body weight despite the catabolic state in late gestation. During early pregnancy, maternal adipose fat accumulation may represent a store of essential lipid components derived from the maternal diet.
At first, TAG in the form of both liver-derived VLDL and chylomicrons must be hydrolyzed to FFA for placental supply to the fetus. The two primary human placental lipases, placental lipoprotein lipase and endothelial lipase release FFAs from lipoproteins. In maternal adipose, LPL is the major TAG hydrolase, and it likely plays a significant role in the placenta (Waterman et al., 1998, 2000; Magnusson-Olsson et al., 2006). Adipose lipoprotein lipase hydrolyses TAGs from VLDL and chylomicrons to FFA for adipose’s lipid uptake in the early stage of pregnancy when mothers are relatively insulin sensitive. However, during late gestation specific insulin resistance, activity reduces to support maternal energy requirements while glucose is diverted to the growing fetus (Kim et al., 2014; Herrera and Desoye, 2016). In the placenta, hydrolysis of TAG to FFA by placental lipases allows them to be taken up by the syncytiotrophoblast, where they can be metabolized and transported into fetal circulation (Waterman et al., 2000; Barrett et al., 2014; Heerwagen et al., 2018). As the liver is the site of LCPUFA synthesis, it is suggested that VLDL may play an important role in transporting plasma LCPUFAs in pregnancy. However, a ratio of HDL lipoprotein of ARA and DHA to HDL cholesterol and HDL- apoAI, enrichment of plasma HDL with ARA and DHA, suggest DHA is primarily carried by HDL during pregnancy (Zamai et al., 2020). Several mechanisms ensure adequate transport of these LCPUFAs to the fetus (Duttaroy, 2009). The fetal brain has its growth spurt in the third trimester of pregnancy and early childhood. The central nervous system is particularly rich in ARA and DHA, and the cerebral accretion of these LCPUFAs is essential (McNamara, 2010; Parellada et al., 2017; Basak et al., 2021). Therefore, an appropriate pre-and postnatal supply of these LCPUFAs is necessary for optimal fetal and neonatal growth, neurologic development and function (Innis, 1991; Makrides et al., 2001), learning and behavior (Stevens et al., 1995; Basak et al., 2021).
The plasma levels of phospholipid-containing ARA and DHA increase on average by ∼40% from the first trimester (10 weeks) to the time of parturition. However, non-essential unsaturated fatty acids increase considerably with the progression of gestation (>65%). Therefore, maternal EFA supply is continuously required for fetal growth and development, as the fetus depends on the maternal supply of LCPUFAs such as ARA, EPA, and DHA. A deficiency of these essential fatty acids may critically affect embryonic organogenesis, particularly in neurological development. LCPUFAs derived from the diet are stored in the maternal adipose depot during early pregnancy. The maternal body fat accumulation allows an essential store of LCPUFAs derived from the maternal diet and metabolism. Since the conversion of EFAs to DHA and ARA is relatively low in humans, maternal availability and supply of LCPUFA are essential for the developing fetus. Thus, the diet of pregnant women must contain sufficient LCPUFAs to meet the metabolic requirements of the mother and the developing fetus.
Early placentation requires the invasive properties of the extravillous trophoblasts derived from the outer trophectoderm layer of the blastocysts (Moser and Huppertz, 2017). Insufficient trophoblast invasion of the uterine wall and resultant reduced blood flow can complicate the pregnancy outcome, as observed in preeclampsia. Therefore, invasive extravillous trophoblasts of the human placenta are critically factored in a successful pregnancy. These trophoblasts remodel the uterine spiral arteries to increase blood flow and oxygen delivery to the developing fetus. This invasive behavior of trophoblasts follows a precise chronology of vascular events during early gestation. The development of a placental vascular network is essential for the growth and maintenance of the developing fetus (Chung et al., 2000; Chaiworapongsa et al., 2011; Basak et al., 2013). Defective invasion of trophoblasts in the uterine wall is directly involved in the development of preeclampsia, a major and frequent complication of human pregnancy with detrimental consequences on fetal and maternal health. The trophoblastic invasion of the uterine wall is precisely regulated. It is transiently restricted to early pregnancy, and it is spatially confined to the endometrium, the first-third of the myometrium, and the associated spiral arteries. The remodeling of spiral arteries mimics the process of angiogenesis and is critical for the early placentation process. The angiogenesis process involves several factors such as vascular endothelial growth factor (VEGF), angiopoietin-like protein 4 (ANGPTL4), platelet-derived growth factor (PDGF), and platelet-activating factor (PAF) (Chung et al., 2000; Hill, 2001; Chaiworapongsa et al., 2011; Basak et al., 2013). EFAs and LCPUFAs metabolites modulate angiogenesis processes (Hardman, 2004; Spencer et al., 2009; Salvado et al., 2012). Eicosanoids produced from ARA stimulate angiogenesis, whereas those produced from EPA and DHA inhibit angiogenesis and tumorigenesis (Hardman, 2004; Spencer et al., 2009; Salvado et al., 2012). Both n-3 and n-6 LCPUFAs and their eicosanoids regulate several angiogenic growth factors, cell migration, proliferation, and angiogenesis. N-3 fatty acids and their eicosanoid derivatives attenuate excess vascularization by reducing angiogenesis through decreased production of proangiogenic ARA-derived eicosanoids, membrane receptor-ligand interactions, and via their intrinsic anti-tumor activities (Nie et al., 2000; Pai et al., 2001; Bagga et al., 2003; Pola et al., 2004; Kamiyama et al., 2006). However, the angiogenic response is more complex and may involve several other factors (Dimitriadis et al., 2005; Spencer et al., 2009). Several n-6 eicosanoids promote tumor growth and angiogenesis. PGE2, produced from ARA, can induce angiogenesis by producing various proangiogenic factors in different cells (Spencer et al., 2009; Tobin et al., 2009; Zhang and Daaka, 2011; Basak et al., 2013). PGE2 increased the synthesis of VEGF, basic fibroblast growth factor (bFGF), or CXCL1 that, in turn, act on endothelial cells to promote angiogenesis (Weedon-Fekjaer et al., 2005; Wang et al., 2006; Spencer et al., 2009; Zhang and Daaka, 2011). The PGE2-induced angiogenic response is mediated via PGE2 receptor-mediated signaling systems. Since PGE2 is produced from ARA by COX-2, thus COX-2 is regarded as an angiogenesis mediator. Overexpression of COX-2 is significantly correlated to tumor invasiveness, prognosis, and survival in some cancers (Fu et al., 2004). Thus, COX-2 inhibitors can effectively prevent n-6 eicosanoids mediated inflammation, proliferation, angiogenesis, and apoptosis (Fu et al., 2004). In contrast, n-3 fatty acids reduce the expression of VEGF, PDGF, IL-6, and MMP-2 (Kang and Weylandt, 2008; Spencer et al., 2009). In marked contrast to the effects observed with the n-3 LCPUFAs, the n-6 LCPUFAs have a stimulatory or neutral effect on angiogenic processes, cell migration, or cell proliferation (Hardman, 2004; Szymczak et al., 2008). During the initiation and progression of tumors, several enzymes involved in the biosynthesis of eicosanoids, such as COX-2, 5-LOX, and 12-LOX, are upregulated. Overexpression of COX-2 in carcinoma cells increases angiogenesis, as evidenced by an increased migration and tube formation of the endothelial cells via producing eicosanoids and angiogenic factors, VEGF and bFGF. Besides, inflammatory cells in the tumors may release pro-inflammatory cytokines such as IL1β or TNFα that can induce angiogenesis. The anti-angiogenic activity of n-3 LCPUFAs is mediated by inhibiting the synthesis of ARA-derived eicosanoids (Spencer et al., 2009). VEGF or its receptors are upregulated in several tumors (Takahashi, 2011). N-3 fatty acids affect the expression of proangiogenic factors (Massaro et al., 2010; Scoditti et al., 2010). Both EPA and DHA significantly inhibit cell proliferation, migration, and tubule formation in endothelial cells (Kanayasu et al., 1991; Yang et al., 1998; Murota et al., 2000; Kim et al., 2005; Spencer et al., 2009). VEGF receptors are down-regulated by EPA by inhibiting activation of NFκB (Ghosh-Choudhury et al., 2009; Spencer et al., 2009). Moreover, EPA down-regulates Flk-1 receptors expression, whereas it upregulates the expression of Flt-1 receptors (Yang et al., 1998). N-3 LCPUFAs suppressed the VEGF-induced tube formation via down-regulation of VEGFR-2 in the endothelial cells (Tsuji et al., 2003; Calviello et al., 2004; Szymczak et al., 2008; Spencer et al., 2009). EPA and DHA reduce angiogenesis in tumors by inhibiting the synthesis of angiogenic factors such as VEGF, PDGF, COX-2, PGE2, nitric oxide (NO) (Spencer et al., 2009). 4-hydroxy-DHA, a 5-Lipoxygenase product of DHA, inhibits endothelial cell proliferation and angiogenesis mediated via PPARγ (Abdelwahab et al., 2003; Sapieha et al., 2011). Increased NO levels by n-3 fatty acids may decrease VEGFR-mediated angiogenesis (Matesanz et al., 2010). A higher ratio of O2–/NO contribute to disturbing angiogenesis (Matesanz et al., 2010; Hamed et al., 2011).
Since n-6 fatty acids are involved in tumor progression and metastasis via stimulated angiogenesis, reducing the ratio of n-6/n-3 fatty acids in tissue may impart benefit in cancers (Clarke, 2000; Price et al., 2000; Nakamura et al., 2001; Wahle et al., 2003). N-3 LCPUFAs reduce angiogenesis via several mechanisms, including modulation in the expression of VEGF, ANGPTL4, eicosanoids, COX-2, fatty acid-binding proteins (FABPs), and nitric oxide (NO) (Spencer et al., 2009). DHA, in contrast, increased tube formation to the greatest extent compared with EPA and ARA in extravillous trophoblasts, HTR8/SVneo cells. Table 1 shows the effects of fatty acids on the expression of mRNAs of angiogenic growth factors in these cells. DHA stimulated tube formation by stimulating the expression and secretion of VEGF in extravillous trophoblast cells (Johnsen et al., 2011). Thus, DHA can help the early placentation process by stimulating angiogenesis. This contrasts with the inhibitory effects of DHA on angiogenesis in many cell types, including tumors (Spencer et al., 2009). The mRNA expression of VEGF by DHA is unique as various growth factors and cytokines induce it but not by any other fatty acids. However, DHA stimulates VEGF expression and secretion, whereas all long-chain fatty acids stimulate ANGPTL4 secretion. In addition to stimulating the expression of major angiogenic factors such as VEGF and ANGPTL4, fatty acids also stimulate the expression of intracellular FABP-4 and FABP-3, which are known to modulate angiogenesis directly. Emerging data indicate that FABPs may be involved in the angiogenesis process. This indicates that differential mode of action of DHA compared with other fatty acids in the angiogenic process (Johnsen et al., 2011). Effects of these fatty acids were quite the opposite to what was observed in tumors (Basak et al., 2013; Kang and Liu, 2013). With the recent spate of studies on regulators of angiogenesis, these observations on the regulation of placental angiogenesis could be exploited for ensuring positive outcomes for most pregnancies.
Table 1. Shows the effects of fatty acids on the expression of mRNAs of angiogenic growth factors in these cells.
FABPs have a role in angiogenesis, and their expression is modulated by various angiogenic mediators (Mousiolis et al., 2012). Both VEGF and bFGF increase the mRNA and protein levels of FABP4 in endothelial cells. FABP4 promotes the proliferation of endothelial cells. First-trimester trophoblast cells express and secrete VEGF considerably in the presence of fatty acids and FABP4 via VEGF, ANGPTL4, and FABP4. FABP4-induced VEGF expression is inhibited by siRNA-mediated knockdown of VEGFR2, whereas the VEGFR1 agonists, PIGF1 and 2, had no such effects. FABP4 is a novel target of the VEGF/VEGFR2 pathway and acts as a positive regulator of angiogenesis in endothelial cells (Elmasri et al., 2009, 2012; Cataltepe et al., 2011; Ghelfi et al., 2013). Expression of FABP4 is demonstrated in an angiogenesis-dependent pathology, infantile hemangioma, the most common tumor of infancy, and endothelial cells (Fishman and Mulliken, 1993). FABP4 has a role in activating mitogenic pathways and the expression of several key mediators of angiogenesis. Leptin, EPA, and DHA- stimulated FABP4 expression possibly target VEGF signaling in trophoblast HTR8/SVneo cells (Basak and Duttaroy, 2012; Basak et al., 2013).
Despite the similarity in ligand binding property and tertiary structures, FABPs have a tissue-specific function (Storch and Thumser, 2010). Maternal plasma FABP4 is independently related to the development of preeclampsia (Yan et al., 2016). FABP4 involves preeclampsia’s pathogenesis via different pathways associated with insulin resistance, inflammation, and dyslipidemia. Increasing evidence suggests the angiogenic function of fatty acid transport/binding proteins in various cell systems (Akbal et al., 2013; Ghelfi et al., 2013). In placental trophoblasts, angiogenesis was maximally blocked in the presence of FABP4 inhibitor compared with other VEGF signaling inhibitors such as P38/MAPK, L-NAME. However, DHA- and VEGF- induced tube formation was maximally reduced by p38 MAP kinase inhibitor. All these inhibitors could not inhibit the ANGPTL4 and oleic acid-induced tube formation in placental trophoblasts. FABP4, therefore, may be involved in part in the basal level and stimulated tube formation by VEGF, DHA, and leptin, whereas it has little or no effect in ANGPTL4- and OA-induced tube formation in these cells (Pandya et al., 2016). FABP4 may play a differential role in fatty acids, and angiogenic growth factors mediated tube formation in the first-trimester trophoblast cells. Figure 3 describes the fatty acid-induced angiogenesis in the human placental first-trimester trophoblasts.
Figure 3. Fatty acid-induced angiogenesis in the human placental first-trimester trophoblasts. Proangiogenic growth factors such as ANGPTL4, VEGF, and FABP4 are involved in placental angiogenesis. ANGPTL4 and FABP4 are stimulated by all other long-chain fatty acids, whereas DHA stimulates VEGF. DHA, Docosahexaenoic acid; FABP4, Fatty acid-binding protein 4; VEGF, vascular endothelial growth factor; ANGPTL4, angiopoietin-like protein 4; LCFAs, long-chain fatty acids.
Fatty acids provide cellular energy, constitute an essential structural element of cellular membranes, and are important for developing specific tissues. The fetal source of fatty acids is either TAG-rich maternal lipoproteins, such as chylomicrons and VLDL, or FFAs bound to albumin. Albumin–FFAs complexes interact with fatty acid uptake/transport proteins in the microvillous plasma membrane (MVM), taking FFAs into the syncytiotrophoblast cells (Duttaroy, 2009). TAG in maternal lipoproteins, particularly very-low-density lipoproteins (VLDL), is hydrolyzed into FFAs by lipoprotein lipase (LPL) expressed in the MVM. FFA is subsequently transferred across the MVM. Alternatively, maternal lipoproteins interact with LDL/VLDL receptors in MVM, resulting in endocytosis and intracellular hydrolysis, which releases FFA. Intracellularly, FFAs are transported bound to FABPs (Duttaroy, 2009). During pregnancy, placental LPL activity increases, and plasma FFA concentrations rise rapidly during the third trimester, thus representing the main class of lipids crossing the placenta (Magnusson-Olsson et al., 2006). In diabetic pregnancy, a wide range of disturbances in lipid metabolism have been described, and maternal lipids seem to be the strongest determinants of fetal growth in GDM newborns (Schaefer-Graf et al., 2011). Both maternal TAGs and FFAs are positively correlated with neonatal weight and fat mass in GDM pregnancy, indicating that maternal dyslipidemia may enhance the availability of lipids to the fetus (Schaefer-Graf et al., 2011). Maternal diet and metabolic status alter placental lipid transport and biology (Lewis et al., 2018). First, the dietary composition may directly change the pool of fatty acids available for uptake by the placenta; however, quality and quantity of fats may alter placental lipid transfer indirectly, such as n-3 fatty acids, which is believed to modulate placental oxidant stress and inflammatory status, and lipid transport (Leghi and Muhlhausler, 2016). Maternal GDM and obesity have been reported to alter placental fatty acid transfer. However, further work is required in understanding the mechanisms of specific placental alterations on lipid transport and metabolism (Lewis et al., 2018).
The critical requirement of these fatty acids in feto-placental unit demands an efficient transport of these LCPUFAs to the fetus by the placenta. The human placenta plays a crucial role in mobilizing the fat stores of maternal adipose and actively concentrating and channeling important LCPUFAs to the fetus via multiple mechanisms, including selective uptake by the trophoblast, intracellular metabolic transfer, and selective supply to the fetal circulation. Several membrane proteins are thought to be responsible for the tissue fatty acid uptake of the placenta (Dutta-Roy, 2000b; Duttaroy, 2009). These include the 40-kDa plasma membrane-associated fatty acid-binding protein (FABPpm), the heavily glycosylated 88-kDa fatty acid translocase (FAT), also known as CD36, and a family of 63–70-kDa fatty acid transport proteins (FATP 1–6) (Duttaroy, 2009). FABPpm binds to long-chain fatty acids were isolated and purified from human placental membranes (Duttaroy, 2009). The human placental p-FABPpm, located exclusively on the maternal-facing membranes of the placenta, may be involved in the sequestration of maternal LCPUFAs (Dutta-Roy, 2000b). Radiolabeled fatty acid-binding revealed that p-FABPpm had higher affinities (Kd) and binding capacities (Vmax) for LCPUFAs than other fatty acids. Thus, the presence of p-FABPpm in the placental membrane facing maternal circulation may enforce the uni-directional flow of the LCPUFA from the mother to the fetus. In contrast, FAT and FATPs are present on both microvillous and basal membranes of the human placenta (Dutta-Roy, 2000b). Location of FAT and FATPs on both sides of the bipolar placental trophoblast cells may favor the transport of general FFA pool in both directions, i.e., from the mother to the fetus and vice versa.
FAT/CD36 is heavily glycosylated fatty acid translocase (FAT/CD36), the sequence of which is 85% homologous with that of glycoprotein IV (CD36), is an integral membrane protein (23). This 472-amino-acid (53 kDa) protein is substantially glycosylated (10 predicted N-linked glycosylated sites). Unlike FABPpm, FATP, FAT is a multifunctional protein with several putative ligands, including FFAs, collagen, thrombospondin, and oxidized LDL. FAT/CD36 was demonstrated in the human placenta using both pure trophoblast cells and placental membrane preparations. FAT is present in the placental membranes, microvillous, and basal membranes. Little is known about the regulation of FAT/CD36 function in placental cells, but several lines point toward a translocational mechanism for increasing LCFA uptake by different cells. Caveolin-1 may control FAT/CD36 mediated fatty acid uptake by increasing surface availability.
FATP is the family of integral transmembrane proteins consisting of six (FATP 1–6) isoforms that show different tissue expression patterns. FATP-1 was first identified in human placental membranes. Later other FATP isoforms were detected in the human placenta. Consistent with the role of FATP-1 in fatty acid internalization, a significant portion of FATP-1 is localized at the plasma membrane. FATPs are classified as fatty acid transport proteins because, when overexpressed, they increase the rate of fatty acid internalization, most notably at low concentrations when diffusion may not be sufficient. FATP is suggested to act in concert with fatty acyl-CoA synthetase, an enzyme that prevents efflux of the incorporated fatty acids by their conversion into acyl-CoA derivatives and hence rendering fatty acid uptake uni-directional. These long-chain fatty acyl-CoA esters act both as substrates and intermediates in various intracellular functions. FATP-mediated uptake of long-chain fatty acids was diminished in the face of cellular depletion of ATP. Thus, FATP is likely responsible for the increased uptake of long-chain fatty acids necessary to sustain increased β-oxidation. Therefore, the tissue-selective effects of various PPARs and their ligands produced by FATP and ACS may provide insight into the relationship between FFA uptake, triglyceride synthesis, and β-oxidation of fatty acids. Additionally, FATP-1 possesses ACS activity toward long-chain fatty acids (16–22 carbons), although ACS activity of FATP-1 is very low compared to ACSL1. FATP-1 has one membrane-spanning region and several membrane-associated regions. Since this arrangement does not typically support a channel or transporter, FATP may, therefore, increase the fatty acid internalization by increasing the rate of “flip-flop,” trapping the fatty acids in the inner leaflet of the plasma membrane or activating the fatty acid to its CoA formation. Disruption of the FATP-4 gene in mice demonstrated its essential function in normal mouse development. However, little is known about the specificity of FATPs for different fatty acids (Herrmann et al., 2003).
A transporter major facilitator superfamily domain-containing 2A (MFSD2a) is also reported in the human placenta (Basak et al., 2020). The precise roles of MFSD2a in the placenta is yet to be deciphered. Placental MFSD2a transporter expression decreased and correlated to decreased DHA in cord blood of women with gestational diabetes, indicating its contributiong to materno-fetal DHA transport (Sanchez-Campillo et al., 2020). Not much is known about the cellular localization of MFSD2a in the human placenta yet, although its expression is altered in the placenta of gestational diabetes placenta and preeclampsia (Prieto-Sanchez et al., 2017). Human placental MFSD2a (the major facilitator superfamily domain-containing 2a) also contributes to fetal DHA accumulation by transporting DHA-containing lysophospholipids (Prieto-Sanchez et al., 2017).
PPAR-γ and RXR regulate fatty acid transport in primary human trophoblasts (Schaiff et al., 2005). The incubation of human trophoblast cells with both PPAR-γ and RXR agonists resulted in elevated mRNA expression of FATP-1 and FATP-4 but not FATP-2, -3, and -6 in these cells (Schaiff et al., 2005). Similar results were reported in vivo using PPAR-γ agonist that increased the placental expression of FABPpm and FAT/CD36 (Schaiff et al., 2005).
Several FABPs are involved in the fatty acid trafficking of human placental trophoblasts. The complex interactions of these proteins may be essential for active fatty acid transport, metabolism, and gene expression in the placenta. Also, the involvement of several nuclear transcription factors (PPARs, LXR, RXR, and SREBP-1) in the expression of genes responsible for fatty acids uptake, placental trophoblast differentiation, and human chorionic gonadotropin (hCG) production indicates regulatory roles of fatty acid-activated transcriptions factors in placenta biology (Weedon-Fekjaer et al., 2005). The uptake of individual fatty acids was almost equally inhibited by triacsin C (an inhibitor of CoA formation), indicating that the CoA formation step may be involved in the uptake of these fatty acids in the first-trimester human trophoblast cells, HTR8/SVneo (Basak and Duttaroy, 2013). This is again in contrast to what was observed in triacsin C-induced reduction of DHA uptake by the epithelial placental trophoblasts where DHA uptake was inhibited least by triacsin C compared with other fatty acids (Tobin et al., 2009). DHA, by avoiding CoA formation in the last trimester, trophoblasts may allow preferential transport across these cells. In contrast, the first-trimester trophoblast cells are not fatty acid transporting cells like last trimester placental trophoblast cells.
The maternal, fetal, and neonatal EFA/LCPUFAs status is an important determinant of feto-placental growth and development (Innis, 1991; Uauy et al., 2001). As a structural component, fatty acids are involved in the function of the neuronal membrane. A change in the fatty acid composition of the synaptic membranes can affect neuronal functions in terms of membrane receptors, ion channels, and enzymes and the transmission of intra- and inter-cellular signals generated by fatty acid-derived second messengers. Nervous tissue has the second-highest concentration of fatty acids after adipose tissue, and LCPUFA levels are particularly high in the retina and cerebral cortex. Most LCPUFAs accumulate during brain development at a period of intense cell division, synaptogenesis (Innis, 2008). The importance of DHA during pregnancy in fetal cognitive development and its postnatal spill-over effect has been studied (Anderson et al., 1990; Innis, 2008). The critical role of DHA rests on its participation in maintaining membrane fluidity, impulse propagation, synaptic transmission, and its function as a cytosolic signal-transducing factor for gene expressions during brain development (Anderson et al., 1990; Abdelwahab et al., 2003). In humans, this accumulation starts at the beginning of the trimester and continues until 2 years of age (Clandinin et al., 1980; Innis, 2008).
Maternal LCPUFAs are provided to the offspring via placental transfer during gestation and via lactation after birth. Consequently, brain LCPUFA in the offspring much depend on maternal PUFA intake (Elias and Innis, 2001). Adequate DHA supply during the perinatal period is essential for optimal CNS development and function. In contrast, dietary n-3 LCPUFA deficiency impairs neuronal plasticity. Animal studies have demonstrated that DHA deficiency during gestation and soon after birth could not be fully corrected later in life (Krabbendam et al., 2007). At 33 weeks, the hypothalamus glycerophospholipids of young pups had significantly reduced DHA compared with controls (that had received ALA for 3 weeks after birth) even after dietary correction with ALA for 30 weeks.
During the third trimester of pregnancy, DHA requirements increase to support fetal growth, particularly of the brain and eyes (Innis, 1991). DHA can account for up to 50% of phospholipid fatty acids in these tissues, suggesting that it is heavily involved in neuronal and visual functions (Simopoulos, 1989; Neuringer, 2000). N-3 LCPUFAs play a well-documented role in the brain development of the fetus and child (Gibson and Makrides, 2000; Uauy and Hoffman, 2000). Premature infants require particular attention, as they have no reserve adipose tissue. These reserves are not generally built up until the third trimester. Premature infants are, therefore, directly dependent on maternal dietary intake of LCPUFAs (Gibson and Makrides, 2000). DHA also showed significant effects on photoreceptor membranes and neurotransmitters involved in the signal transduction, rhodopsin activation, rod and cone development, neuronal dendritic connectivity, and functional maturation of the central nervous system (Neuringer, 2000; Uauy et al., 2001).
DHA deposition in the brain takes place during intrauterine and lactation (Carver et al., 2001; Basak et al., 2021). Accretion of DHA in the fetal brain is the highest in the third trimester of pregnancy (Innis, 2008). DHA incorporation in the neuronal membrane in early life solely depends on placental transfer (Dutta-Roy, 2000a), breastfeeding, and endogenous synthesis of DHA (Cetin et al., 2009; Duttaroy, 2009). Maternal dietary intakes of DHA influence the long-chain fatty acid composition of breast milk and plasma levels of lactating women and their infants (Sherry et al., 2015). A robust linear relationship between maternal DHA level and umbilical cord plasma phospholipid contents was reported (Calder, 2016).
The high-affinity placental plasma membrane fatty acid-binding protein (p-FABPpm) is thought to be involved in maternal transport of DHA to the fetus (Campbell and Dutta-Roy, 1995; Basak et al., 2020). Since the rapid deposition of DHA into the brain occurs during the last trimester and subsequently in lactation, the maternal DHA status must be maintained well during the critical window of brain development. The dietary intake and maternal stores of DHA are the determinants of neonatal blood DHA concentrations at birth (Bourre, 2007). Blood LCPUFA in breastfed infants remain higher than maternal levels for some time postnatally (Koletzko et al., 1989; Jorgensen et al., 2006). Dietary supplementation of DHA to pregnant and nursing mothers dose-dependently increases the DHA level in breast milk, which causes higher tissue accretion of DHA in breastfed infants with improved outcomes of mental performance (Innis, 2005; Oddy et al., 2010; Hibbeln et al., 2019). Comprehensive studies have shown that dietary supplementation during pregnancy with marine oil sources of n-3 LCPUFAs results in increased blood levels of DHA and an associated improvement in visual and cognitive function in infants and children (Table 2).
Table 2. Effects of maternal long-chain polyunsaturated fatty acids during pregnancy on brain development and growth in infant and children: clinical trials.
Based on established guidelines, it is emphasized that maternal dietary DHA requirements should be increased during pregnancy and lactation. Precisely, a minimum of 200 mg of DHA per day is recommended during these periods (Van Elswyk and Kuratko, 2009). In both full-term and pre-term, the evidence is compelling that breastfeeding is vital for an infant’s neurodevelopment. Worldwide, mean DHA and ARA levels in breast milk are found 0⋅37 and 0⋅55% of total fatty acids, respectively.
Children’s intelligence quotient (I.Q) was increased by 0.8–1.8 points when their mothers were supplemented with DHA for a more extended period from pregnancy to lactation period and beyond (Cohen et al., 2005; Dunstan et al., 2008). Several prospective studies indicated that breastfed infants had a significant neurocognitive advantage compared with formula-fed infants (Agostoni et al., 1995; Kramer et al., 2008; Oddy et al., 2011), possibly due to the higher incorporation of DHA and ARA in breast milk relative to formula milk. The association between breastfeeding and child IQ concerning the FADS2 genetic profile, specifically in SNP rs174575b, established a genetic variability in the fatty acid metabolism (Caspi et al., 2007). Breastfed infants with rs174575 C-dominant carriers achieved higher scores on standardized IQ tests than non-breastfed C-carriers infants. However, observational data are confounded by the heterogeneous composition of breast milk and environmental factors that influence infant mental development.
Mother supplies both ARA and DHA to the growing and developing brain via placenta and breastfeeding. ARA uptake was higher in early trimester trophoblast cells than in EPA and DHA (Basak and Duttaroy, 2013; Basak et al., 2021). ARA may be required in a higher amount to support growth-promoting placental activities and the production of prostaglandins and eicosanoids. In human milk, the amount of ARA typically exceeds the DHA levels (Lauritzen and Carlson, 2011). Milk ARA content is also less varied than DHA, and, unlike DHA, ARA does not seem to be linked to maternal intake (Basak et al., 2021). There has been much discussion in recent years about the proportional requirement of ARA and DHA in infant formula. Studies clearly show the requirement for both ARA and DHA in addition to the essential fatty acids [linoleic acid, 18:2n-6 (LA), and alpha-linolenic acid, 18:3n-3 (ALA)] to support the optimal body and brain growth, and its function. ARA is quantitatively the most predominant LCPUFAs in the brain after the DHA (Contreras et al., 2000). Although DHA’s neuroprotective properties are documented, the roles of ARA in brain development and functions have not been highlighted to a greater extent. Given that ARA and its precursor, LA, contribute significantly to the Western diet and its pleiotropic biological effects and interactions with DHA make this n-6 LCPUFA a crucial modifiable factor in brain development and preventive strategies of brain diseases. ARA corresponds to around 20% of neuronal fatty acids and is mainly esterified in membrane phospholipids.
Several studies have suggested that the structure-function and metabolism of the brain depend on levels of ARA, DHA and interactions of their metabolites (Contreras and Rapoport, 2002). ARA must either be consumed in the diet or synthesized from its precursor LA in the liver. The brain contains relatively low LA levels, and its conversion into ARA is minimum in the brain. Thus, the brain depends on a steady supply of ARA from the circulation. Although lipoproteins and lysophospholipids of plasma may contribute to brain ARA levels, their quantitative contribution is unknown. Plasma unesterified ARA deposits in the brain at the rate of 17.8 mg/day in the whole brain of adult humans. Upon its entry into the brain, ARA is activated by a long-chain acyl-CoA synthetase and can be esterified into the sn-2 position of phospholipids. During neurotransmission, the brain ARA cascade is initiated when it is released from synaptic membrane phospholipid by neuroreceptor-initiated activation of cPLA2. PLA2 is activated by dopaminergic, cholinergic, glutamatergic, and serotonergic stimulation via G-proteins or calcium (Sun et al., 2010). Several PLA2 are activated via serotonergic (5-hydroxytryptaminergic), glutamatergic, dopaminergic, and cholinergic receptors (Bazinet, 2009; Sun et al., 2010). Usually, calcium-dependent cytosolic PLA2 (cPLA2) resides at the postsynaptic terminals, selective for releasing ARA, whereas calcium-independent PLA2 is believed to remove the DHA sn-2 position phospholipids [13,14]. Upon its release, a portion of the unesterified ARA is converted to prostaglandins, leukotrienes, and lipoxins, a portion oxidized via β-oxidation, and the remainder (approx. 97% under basal conditions) is activated by ACSL and ultimately recycled and re-esterified into the sn-2 position of phospholipids (Rapoport, 2008). An additional ARA is released by activated cytokine and glutamatergic N-methyl-d-aspartate receptors in conditions such as neuroinflammation and excitotoxicity. Although ARA and its derivatives relay signals are not entirely understood, they regulate cellular growth and differentiation, blood flow, neuroinflammation, excitotoxicity, the sleep/wake cycle, and neurogenesis (Duncan and Bazinet, 2010).
ARA itself is also directly involved in synaptic functions. The level of intracellular free ARA and the balance between the releasing and incorporating enzymes in membrane phospholipids may play critical roles in neuroinflammation and synaptic dysfunction. The substrate binding and trafficking of ARA and DHA are mediated by FABPs (Mallick et al., 2021). For example, FABP5 and FABP7 are more selective for DHA, whereas FABP3 binds ARA with much higher affinity (Boneva et al., 2011; Pan et al., 2015). Furthermore, regarding fatty acid metabolizing enzymes, DHA is preferentially used as a substrate by ACSL6 (Marszalek et al., 2005) and calcium-independent group VI PLA2 (Green et al., 2008), whereas ACSL4 and group IV cPLA2 used ARA as preferred substrate.
Both ARA and DHA are sources of phospholipids in the membrane fluidity of synapse that controls the functions of receptors, transporters, and membrane-bound proteins. ARA and DHA are required to replenish brain injury, vascular regulation, and brain development, and maturity in pre-term babies. ARA and its metabolites are needed to support endothelial cells during brain injury. In contrast, DHA and its metabolites are required to support membrane fluidity for the receptors, linear growth and network of neuronal cells. Neuroprotective actions of DHA in pre-term are manifested with anti-inflammatory initiation and resolutions by its own and its signaling lipid mediators in particular. ARA and DHA produced different sets of pro-resolving lipid bioactives. Lipoxins are derived from ARA, while resolving and maresins are derived from EPA and DHA. LipoxinA4 (LXA4) receptor staining during brain injury indicates that LXA4 lowers neuroinflammation and brain edema (Luo et al., 2013; Basak et al., 2020). The anti-inflammatory effects of LXA4 acts as an endogenous allosteric modulator of the cannabinoid receptor (Pamplona et al., 2012). Thus, in contrast to PGE2, which shows pro-inflammatory actions, lipoxins offer an inflammation resolution process (Basak et al., 2020).
The essentiality of both DHA and ARA in feto-placental development is corroborated by the fact that the mother is required to supply both preferentially during the critical window of brain development via the placenta and breastfeeding postnatally. Both ARA and DHA are necessary for fetal neurodevelopment, and a deficiency may compromise the optimum growth of the babies. Moreover, early placental angiogenesis is critical for establishing the placental size and vascularization and, thus, average fetal growth and development. LCPUFAs favor placental growth by increasing angiogenesis in the first-trimester placenta cells. The dietary fatty acids stimulated the expression of major proangiogenic growth factors such as VEGF and ANGPTL4, FABP4, FABP3, which modulate angiogenesis directly. The human placental trophoblasts play a crucial role in mobilizing the maternal fatty acids and channeling important LCPUFAs to the fetus via multiple mechanisms, including their selective uptake by the trophoblast, intracellular metabolic trafficking, and selective export to the fetal circulation. Among the fatty acid-binding/transport proteins, p-FABPpm, located exclusively on the maternal-facing membranes of the placenta, may be involved in the sequestration of maternal LCPUFAs by the placenta. A better understanding of the physiology of fatty acid transport of the fetoplacental unit is needed in order to secure optimum fetoplacental growth and development.
AD: writing—original draft preparation and writing—review and editing. SB: contributed to editing and revision of the work. Both have approved the final version for submission.
This study was supported by a grant from the Thune Holst Foundation.
The authors declare that the research was conducted in the absence of any commercial or financial relationships that could be construed as a potential conflict of interest.
All claims expressed in this article are solely those of the authors and do not necessarily represent those of their affiliated organizations, or those of the publisher, the editors and the reviewers. Any product that may be evaluated in this article, or claim that may be made by its manufacturer, is not guaranteed or endorsed by the publisher.
ARA, Arachidonic acid,20:4n-6; ALA, α-linolenic acids 18:3n-3; COX, Cyclooxygenase; DHA, Docosahexaenoic acid,22:6n-3; EPA, Eicosapentaenoic acid, 20:5n-3; LCPUFAs, Long-chain polyunsaturated fatty acids; EFA, Essential fatty acids; LA, Linoleic acid, 18:2n-6; FABPs, Fatty acid-binding proteins; FAT, fatty acid translocase; FATP, Fatty acid transporter protein; FABPpm, plasma membrane-fatty acid-binding protein; LOX, Lipoxygenase; VEGF, Vascular endothelial growth factor; ANGPTL4, Angiopoietin-like protein 4.
Abdelwahab, S. A., Owada, Y., Kitanaka, N., Iwasa, H., Sakagami, H., and Kondo, H. (2003). Localization of brain-type fatty acid-binding protein in Kupffer cells of mice and its transient decrease in response to lipopolysaccharide. Histochem. Cell Biol. 119, 469–475.
Agostoni, C., Trojan, S., Bellu, R., Riva, E., and Giovannini, M. (1995). Neurodevelopmental quotient of healthy term infants at 4 months and feeding practice: the role of long-chain polyunsaturated fatty acids. Pediatr. Res. 38, 262–266. doi: 10.1203/00006450-199508000-00021
Akbal, E., Koklu, S., Kocak, E., Cakal, B., Gunes, F., Basar, O., et al. (2013). Liver fatty acid-binding protein is a diagnostic marker to detect liver injury due to chronic hepatitis C infection. Arch. Med. Res. 44, 34–38. doi: 10.1016/j.arcmed.2012.11.007
Anderson, G. J., Connor, W. E., and Corliss, J. D. (1990). Docosahexaenoic acid is the preferred dietary n-3 fatty acid for the development of the brain and retina. Pediatr. Res. 27, 89–97. doi: 10.1203/00006450-199001000-00023
Bagga, D., Wang, L., Farias-Eisner, R., Glaspy, J. A., and Reddy, S. T. (2003). Differential effects of prostaglandin derived from omega-6 and omega-3 polyunsaturated fatty acids on COX-2 expression and IL-6 secretion. Proc. Natl. Acad. Sci. U.S.A. 100, 1751–1756. doi: 10.1073/pnas.0334211100
Barbour, L. A., Mccurdy, C. E., Hernandez, T. L., Kirwan, J. P., Catalano, P. M., and Friedman, J. E. (2007). Cellular mechanisms for insulin resistance in normal pregnancy and gestational diabetes. Diabetes Care 30(Suppl. 2), S112–S119. doi: 10.2337/dc07-s202
Barrett, H. L., Kubala, M. H., Scholz Romero, K., Denny, K. J., Woodruff, T. M., Mcintyre, H. D., et al. (2014). Placental lipases in pregnancies complicated by gestational diabetes mellitus (GDM). PLoS One 9:e104826. doi: 10.1371/journal.pone.0104826
Basak, S., and Duttaroy, A. K. (2012). Leptin induces tube formation in first-trimester extravillous trophoblast cells. Eur. J. Obstet. Gynecol. Reprod. Biol. 164, 24–29. doi: 10.1016/j.ejogrb.2012.05.033
Basak, S., and Duttaroy, A. K. (2013). Effects of fatty acids on angiogenic activity in the placental extravillious trophoblast cells. Prostaglandins Leukot. Essent. Fatty Acids 88, 155–162. doi: 10.1016/j.plefa.2012.10.001
Basak, S., Das, M. K., and Duttaroy, A. K. (2013). Fatty acid-induced angiogenesis in first trimester placental trophoblast cells: possible roles of cellular fatty acid-binding proteins. Life Sci. 93, 755–762. doi: 10.1016/j.lfs.2013.09.024
Basak, S., Mallick, R., and Duttaroy, A. K. (2020). Maternal docosahexaenoic acid status during pregnancy and its impact on infant neurodevelopment. Nutrients 12:3615. doi: 10.3390/nu12123615
Basak, S., Mallick, R., Banerjee, A., Pathak, S., and Duttaroy, A. K. (2021). Maternal supply of both arachidonic and docosahexaenoic acids is required for optimal neurodevelopment. Nutrients 13:2061. doi: 10.3390/nu13062061
Bazan, N. G. (2003). Synaptic lipid signaling: significance of polyunsaturated fatty acids and platelet-activating factor. J. Lipid Res. 44, 2221–2233. doi: 10.1194/jlr.R300013-JLR200
Bazinet, R. P. (2009). Is the brain arachidonic acid cascade a common target of drugs used to manage bipolar disorder? Biochem. Soc. Trans. 37, 1104–1109. doi: 10.1042/BST0371104
Boneva, N. B., Kikuchi, M., Minabe, Y., and Yamashima, T. (2011). Neuroprotective and ameliorative actions of polyunsaturated fatty acids against neuronal diseases: implication of fatty acid-binding proteins (FABP) and G protein-coupled receptor 40 (GPR40) in adult neurogenesis. J. Pharmacol. Sci. 116, 163–172. doi: 10.1254/jphs.10r34fm
Bourre, J. M. (2007). Dietary omega-3 fatty acids for women. Biomed. Pharmacother. 61, 105–112. doi: 10.1016/j.biopha.2006.09.015
Brash, A. R. (2001). Arachidonic acid as a bioactive molecule. J. Clin. Invest. 107, 1339–1345. doi: 10.1172/JCI13210
Budowski, P., and Crawford, M. A. (1985). A-Linolenic acid as a regulator of the metabolism of arachidonic acid: dietary implications of the ratio, n-6:n-3 fatty acids. Proc. Nutr. Soc. 44, 221–229. doi: 10.1079/pns19850041
Calder, P. C. (2016). The DHA content of a cell membrane can have a significant influence on cellular behaviour and responsiveness to signals. Ann. Nutr. Metab. 69, 8–21.
Calviello, G., Di Nicuolo, F., Gragnoli, S., Piccioni, E., Serini, S., Maggiano, N., et al. (2004). N-3 PUFAs reduce VEGF expression in human colon cancer cells modulating the COX-2/PGE2 induced ERK-1 and -2 and HIF-1alpha induction pathway. Carcinogenesis 25, 2303–2310. doi: 10.1093/carcin/bgh265
Campbell, F. M., and Dutta-Roy, A. K. (1995). Plasma membrane fatty acid-binding protein (FABPpm) is exclusively located in the maternal facing membranes of the human placenta. FEBS Lett. 375, 227–230. doi: 10.1016/0014-5793(95)01216-2
Campoy, C., Escolano-Margarit, M. V., Ramos, R., Parrilla-Roure, M., Csábi, G., Beyer, J., et al. (2011). Effects of prenatal fish-oil and 5-methyltetrahydrofolate supplementation on cognitive development of children at 6.5 y of age. Am. J. Clin. Nutr. 94, 1880s–1888s. doi: 10.3945/ajcn.110.001107
Carattino, M. D., Hill, W. G., and Kleyman, T. R. (2003). Arachidonic acid regulates surface expression of epithelial sodium channels. J. Biol. Chem. 278, 36202–36213. doi: 10.1074/jbc.M300312200
Carlson, S. E., Colombo, J., Gajewski, B. J., Gustafson, K. M., Mundy, D., Yeast, J., et al. (2013). DHA supplementation and pregnancy outcomes. Am. J. Clin. Nutr. 97, 808–815.
Carver, J. D., Benford, V. J., Han, B., and Cantor, A. B. (2001). The relationship between age and the fatty acid composition of cerebral cortex and erythrocytes in human subjects. Brain Res. Bull. 56, 79–85. doi: 10.1016/s0361-9230(01)00551-2
Caspi, A., Williams, B., Kim-Cohen, J., Craig, I. W., Milne, B. J., Poulton, R., et al. (2007). Moderation of breastfeeding effects on the IQ by genetic variation in fatty acid metabolism. Proc. Natl. Acad. Sci. U.S.A. 104, 18860–18865. doi: 10.1073/pnas.0704292104
Cataltepe, O., Arikan, M. C., Ghelfi, E., Karaaslan, C., Ozsurekci, Y., Dresser, K., et al. (2011). Fatty acid binding protein 4 is expressed in distinct endothelial and non-endothelial cell populations in glioblastoma. Neuropathol. Appl. Neurobiol. 38, 400–410. doi: 10.1111/j.1365-2990.2011.01237.x
Catov, J. M., Patrick, T. E., Powers, R. W., Ness, R. B., Harger, G., and Roberts, J. M. (2007). Maternal leptin across pregnancy in women with small-for-gestational-age infants. Am. J. Obstet. Gynecol. 196, 558.e1–558.e8. doi: 10.1016/j.ajog.2007.01.032
Cetin, I., Alvino, G., and Cardellicchio, M. (2009). Long chain fatty acids and dietary fats in fetal nutrition. J. Physiol. 587(Pt 14), 3441–3451. doi: 10.1113/jphysiol.2009.173062
Chaiworapongsa, T., Romero, R., Savasan, Z. A., Kusanovic, J. P., Ogge, G., Soto, E., et al. (2011). Maternal plasma concentrations of angiogenic/anti-angiogenic factors are of prognostic value in patients presenting to the obstetrical triage area with the suspicion of preeclampsia. J. Matern. Fetal Neonatal. Med. 24, 1187–1207. doi: 10.3109/14767058.2011.589932
Chung, I. B., Yelian, F. D., Zaher, F. M., Gonik, B., Evans, M. I., Diamond, M. P., et al. (2000). Expression and regulation of vascular endothelial growth factor in a first trimester trophoblast cell line. Placenta 21, 320–324. doi: 10.1053/plac.1999.0481
Clandinin, M. T., Chappell, J. E., Leong, S., Heim, T., Swyer, P. R., and Chance, G. W. (1980). Extrauterine fatty acid accretion in infant brain: implications for fatty acid requirements. Early Hum. Dev. 4, 131–138. doi: 10.1016/0378-3782(80)90016-x
Clarke, S. D. (2000). Polyunsaturated fatty acid regulation of gene transcription: a mechanism to improve energy balance and insulin resistance. Br. J. Nutr. 83(Suppl. 1), S59–S66. doi: 10.1017/s0007114500000969
Cohen, J. T., Bellinger, D. C., Connor, W. E., and Shaywitz, B. A. (2005). A quantitative analysis of prenatal intake of n-3 polyunsaturated fatty acids and cognitive development. Am. J. Prev. Med. 29, 366–374. doi: 10.1016/j.amepre.2005.06.008
Colombo, J., Shaddy, D. J., Gustafson, K., Gajewski, B. J., Thodosoff, J. M., Kerling, E., et al. (2019). The kansas university DHA outcomes study (kudos) clinical trial: long-term behavioral follow-up of the effects of prenatal DHA supplementation. Am. J. Clin. Nutr. 109, 1380–1392. doi: 10.1093/ajcn/nqz018
Contreras, M. A., and Rapoport, S. I. (2002). Recent studies on interactions between n-3 and n-6 polyunsaturated fatty acids in brain and other tissues. Curr. Opin. Lipidol. 13, 267–272. doi: 10.1097/00041433-200206000-00006
Contreras, M. A., Greiner, R. S., Chang, M. C., Myers, C. S., Salem, N. Jr., and Rapoport, S. I. (2000). Nutritional deprivation of alpha-linolenic acid decreases but does not abolish turnover and availability of unacylated docosahexaenoic acid and docosahexaenoyl-CoA in rat brain. J. Neurochem. 75, 2392–2400. doi: 10.1046/j.1471-4159.2000.0752392.x
Cousins, L. (1991). Insulin sensitivity in pregnancy. Diabetes 40, 39–43. doi: 10.2337/diab.40.2.s39
Crabtree, J. T., Gordon, M. J., Campbell, F. M., and Dutta-Roy, A. K. (1998). Differential distribution and metabolism of arachidonic acid and docosahexaenoic acid by human placental choriocarcinoma (BeWo) cells. Mol. Cell. Biochem. 185, 191–198. doi: 10.1023/a:1006852230337
Danthi, S. J., Enyeart, J. A., and Enyeart, J. J. (2005). Modulation of native T-type calcium channels by omega-3 fatty acids. Biochem. Biophys. Res. Commun. 327, 485–493.
Darmady, J. M., and Postle, A. D. (1982). Lipid metabolism in pregnancy. Br. J. Obstet. Gynaecol. 89, 211–215.
Desoye, G., and Hauguel-De Mouzon, S. (2007). The human placenta in gestational diabetes mellitus. The insulin and cytokine network. Diabetes Care 30(Suppl. 2), S120–S126. doi: 10.2337/dc07-s203
Di Cianni, G., Miccoli, R., Volpe, L., Lencioni, C., and Del Prato, S. (2003). Intermediate metabolism in normal pregnancy and in gestational diabetes. Diabetes Metab. Res. Rev. 19, 259–270.
Dimitriadis, E., White, C. A., Jones, R. L., and Salamonsen, L. A. (2005). Cytokines, chemokines and growth factors in endometrium related to implantation. Hum. Reprod. Update 11, 613–630. doi: 10.1093/humupd/dmi023
Duncan, R. E., and Bazinet, R. P. (2010). Brain arachidonic acid uptake and turnover: implications for signaling and bipolar disorder. Curr. Opin. Clin. Nutr. Metab. Care 13, 130–138. doi: 10.1097/MCO.0b013e328336b615
Dunstan, J. A., Simmer, K., Dixon, G., and Prescott, S. L. (2008). Cognitive assessment of children at age 2(1/2) years after maternal fish oil supplementation in pregnancy: a randomised controlled trial. Arch. Dis. Child. Fetal Neonatal Ed. 93, F45–F50. doi: 10.1136/adc.2006.099085
Dutta-Roy, A. K. (1994). Insulin mediated processes in platelets, erythrocytes and monocytes/macrophages: effects of essential fatty acid metabolism. Prostaglandins Leukot. Essent. Fatty Acids 51, 385–399. doi: 10.1016/0952-3278(94)90054-x
Dutta-Roy, A. K. (2000a). Cellular uptake of long-chain fatty acids: role of membrane-associated fatty-acid-binding/transport proteins. Cell. Mol. Life Sci. 57, 1360–1372. doi: 10.1007/PL00000621
Dutta-Roy, A. K. (2000b). Transport mechanisms for long-chain polyunsaturated fatty acids in the human placenta. Am. J. Clin. Nutr. 71, 315S–322S. doi: 10.1093/ajcn/71.1.315s
Duttaroy, A. K. (2009). Transport of fatty acids across the human placenta: a review. Prog. Lipid Res. 48, 52–61.
Duttaroy, A. K., and Basak, S. (2020). Maternal dietary fatty acids and their roles in human placental development. Prostaglandins Leukot. Essent. Fatty Acids 155:102080. doi: 10.1016/j.plefa.2020.102080
Elias, S. L., and Innis, S. M. (2001). Infant plasma trans, n-6, and n-3 fatty acids and conjugated linoleic acids are related to maternal plasma fatty acids, length of gestation, and birth weight and length. Am. J. Clin. Nutr. 73, 807–814. doi: 10.1093/ajcn/73.4.807
Elliott, J. A. (1975). The effect of pregnancy on the control of lipolysis in fat cells isolated from human adipose tissue. Eur. J. Clin. Invest. 5, 159–163. doi: 10.1111/j.1365-2362.1975.tb00442.x
Elmasri, H., Ghelfi, E., Yu, C. W., Traphagen, S., Cernadas, M., Cao, H., et al. (2012). Endothelial cell-fatty acid binding protein 4 promotes angiogenesis: role of stem cell factor/c-kit pathway. Angiogenesis 15, 457–468. doi: 10.1007/s10456-012-9274-0
Elmasri, H., Karaaslan, C., Teper, Y., Ghelfi, E., Weng, M., Ince, T. A., et al. (2009). Fatty acid binding protein 4 is a target of VEGF and a regulator of cell proliferation in endothelial cells. FASEB J. 23, 3865–3873. doi: 10.1096/fj.09-134882
Escolano-Margarit, M. V., Ramos, R., Beyer, J., Csábi, G., Parrilla-Roure, M., Cruz, F., et al. (2011). Prenatal DHA status and neurological outcome in children at age 5.5 years are positively associated. J. Nutr. 141, 1216–1223. doi: 10.3945/jn.110.129635
Fishman, S. J., and Mulliken, J. B. (1993). Hemangiomas and vascular malformations of infancy and childhood. Pediatr. Clin. North Am. 40, 1177–1200. doi: 10.1016/s0031-3955(16)38656-4
Fu, S. L., Wu, Y. L., Zhang, Y. P., Qiao, M. M., and Chen, Y. (2004). Anti-cancer effects of COX-2 inhibitors and their correlation with angiogenesis and invasion in gastric cancer. World J. Gastroenterol. 10, 1971–1974. doi: 10.3748/wjg.v10.i13.1971
Ghelfi, E., Yu, C. W., Elmasri, H., Terwelp, M., Lee, C. G., Bhandari, V., et al. (2013). Fatty acid binding protein 4 regulates VEGF-induced airway angiogenesis and inflammation in a transgenic mouse model: implications for asthma. Am. J. Pathol. 182, 1425–1433. doi: 10.1016/j.ajpath.2012.12.009
Ghosh-Choudhury, T., Mandal, C. C., Woodruff, K., St Clair, P., Fernandes, G., Choudhury, G. G., et al. (2009). Fish oil targets PTEN to regulate NFkappaB for downregulation of anti-apoptotic genes in breast tumor growth. Breast Cancer Res. Treat. 118, 213–228. doi: 10.1007/s10549-008-0227-7
Gibson, R. A., and Makrides, M. (2000). N-3 polyunsaturated fatty acid requirements of term infants. Am. J. Clin. Nutr. 71, 251S–255S. doi: 10.1093/ajcn/71.1.251s
Green, J. T., Orr, S. K., and Bazinet, R. P. (2008). The emerging role of group VI calcium-independent phospholipase A2 in releasing docosahexaenoic acid from brain phospholipids. J. Lipid Res. 49, 939–944. doi: 10.1194/jlr.R700017-JLR200
Hamed, E. A., Zakary, M. M., Abdelal, R. M., and Abdel Moneim, E. M. (2011). Vasculopathy in type 2 diabetes mellitus: role of specific angiogenic modulators. J. Physiol. Biochem. 67, 339–349. doi: 10.1007/s13105-011-0080-8
Hara, T., Kimura, I., Inoue, D., Ichimura, A., and Hirasawa, A. (2013). Free fatty acid receptors and their role in regulation of energy metabolism. Rev. Physiol. Biochem. Pharmacol. 164, 77–116.
Heerwagen, M. J. R., Gumina, D. L., Hernandez, T. L., Van Pelt, R. E., Kramer, A. W., Janssen, R. C., et al. (2018). Placental lipoprotein lipase activity is positively associated with newborn adiposity. Placenta 64, 53–60. doi: 10.1016/j.placenta.2018.03.001
Helland, I. B., Smith, L., Saarem, K., Saugstad, O. D., and Drevon, C. A. (2003). Maternal supplementation with very-long-chain n-3 fatty acids during pregnancy and lactation augments children’s iq at 4 years of age. Pediatrics 111, e39–e44. doi: 10.1542/peds.111.1.e39
Herrera, E. (2002). Implications of dietary fatty acids during pregnancy on placental, fetal and postnatal development–a review. Placenta 23(Suppl. A), S9–S19. doi: 10.1053/plac.2002.0771
Herrera, E., Amusquivar, E., Lopez-Soldado, I., and Ortega, H. (2006). Maternal lipid metabolism and placental lipid transfer. Horm. Res. 65(Suppl. 3), 59–64. doi: 10.1159/000091507
Herrera, E., and Desoye, G. (2016). Maternal and fetal lipid metabolism under normal and gestational diabetic conditions. Horm. Mol. Biol. Clin. Investig. 26, 109–127. doi: 10.1515/hmbci-2015-0025
Herrmann, T., Van Der Hoeven, F., Grone, H. J., Stewart, A. F., Langbein, L., Kaiser, I., et al. (2003). Mice with targeted disruption of the fatty acid transport protein 4 (Fatp 4. Slc27a4) gene show features of lethal restrictive dermopathy. J. Cell. Biol. 161, 1105–1115. doi: 10.1083/jcb.200207080
Hibbeln, J. R., Spiller, P., Brenna, J. T., Golding, J., Holub, B. J., Harris, W. S., et al. (2019). Relationships between seafood consumption during pregnancy and childhood and neurocognitive development: two systematic reviews. Prostaglandins Leukot. Essent. Fatty Acids 151, 14–36. doi: 10.1016/j.plefa.2019.10.002
Hill, J. A. (2001). Maternal-embryonic cross-talk. Ann. N. Y. Acad. Sci. 943, 17–25. doi: 10.1111/j.1749-6632.2001.tb03786.x
Hoagland, K. M., Maier, K. G., Moreno, C., Yu, M., and Roman, R. J. (2001). Cytochrome P450 metabolites of arachidonic acid: novel regulators of renal function. Nephrol. Dial. Transplant. 16, 2283–2285. doi: 10.1093/ndt/16.12.2283
Innis, S. M. (2005). Chapter 10 essential fatty acid metabolism during early development. Biol. Grow. Anim. 3, 235–274.
Innis, S. M. (2008). Dietary omega 3 fatty acids and the developing brain. Brain Res. 1237, 35–43. doi: 10.1016/j.brainres.2008.08.078
Jeffrey, B. G., Weisinger, H. S., Neuringer, M., and Mitchell, D. C. (2001). The role of docosahexaenoic acid in retinal function. Lipids 36, 859–871. doi: 10.1007/s11745-001-0796-3
Jimenez, D. M., Pocovi, M., Ramon-Cajal, J., Romero, M. A., Martinez, H., and Grande, F. (1988). Longitudinal study of plasma lipids and lipoprotein cholesterol in normal pregnancy and puerperium. Gynecol. Obstet. Invest. 25, 158–164. doi: 10.1159/000293765
Jin, Y., Arita, M., Zhang, Q., Saban, D. R., Chauhan, S. K., Chiang, N., et al. (2009). Anti-angiogenesis effect of the novel anti-inflammatory and pro-resolving lipid mediators. Invest. Ophthalmol. Vis. Sci. 50, 4743–4752. doi: 10.1167/iovs.08-2462
Johnsen, G. M., Basak, S., Weedon-Fekjaer, M. S., Staff, A. C., and Duttaroy, A. K. (2011). Docosahexaenoic acid stimulates tube formation in first trimester trophoblast cells, HTR8/SVneo. Placenta 32, 626–632. doi: 10.1016/j.placenta.2011.06.009
Jorgensen, M. H., Nielsen, P. K., Michaelsen, K. F., Lund, P., and Lauritzen, L. (2006). The composition of polyunsaturated fatty acids in erythrocytes of lactating mothers and their infants. Matern. Child. Nutr. 2, 29–39. doi: 10.1111/j.1740-8709.2006.00039.x
Judge, M. P. (2018). Omega-3 consumption during pregnancy to support optimal outcomes. J. Obstet. Gynecol. Neonatal. Nurs. 47, 429–437. doi: 10.1016/j.jogn.2017.06.004
Jump, D. B. (2002). Dietary polyunsaturated fatty acids and regulation of gene transcription. Curr. Opin. Lipidol. 13, 155–164. doi: 10.1097/00041433-200204000-00007
Kamiyama, M., Pozzi, A., Yang, L., Debusk, L. M., Breyer, R. M., and Lin, P. C. (2006). EP2, a receptor for PGE2, regulates tumor angiogenesis through direct effects on endothelial cell motility and survival. Oncogene 25, 7019–7028. doi: 10.1038/sj.onc.1209694
Kanayasu, T., Morita, I., Nakao-Hayashi, J., Asuwa, N., Fujisawa, C., Ishii, T., et al. (1991). Eicosapentaenoic acid inhibits tube formation of vascular endothelial cells in vitro. Lipids 26, 271–276. doi: 10.1007/BF02537136
Kang, J. X., and Leaf, A. (1996). Evidence that free polyunsaturated fatty acids modify Na+ channels by directly binding to the channel proteins. Proc. Natl. Acad. Sci. U.S.A. 93, 3542–3546. doi: 10.1073/pnas.93.8.3542
Kang, J. X., and Liu, A. (2013). The role of the tissue omega-6/omega-3 fatty acid ratio in regulating tumor angiogenesis. Cancer Metastasis Rev 32, 201–210. doi: 10.1007/s10555-012-9401-9
Kang, J. X., and Weylandt, K. H. (2008). Modulation of inflammatory cytokines by omega-3 fatty acids. Subcell Biochem. 49, 133–143. doi: 10.1007/978-1-4020-8831-5_5
Kim, D., and Pleumsamran, A. (2000). Cytoplasmic unsaturated free fatty acids inhibit ATP-dependent gating of the G protein-gated K(+) channel. J. Gen. Physiol. 115, 287–304. doi: 10.1085/jgp.115.3.287
Kim, H. J., Vosseler, C. A., Weber, P. C., and Erl, W. (2005). Docosahexaenoic acid induces apoptosis in proliferating human endothelial cells. J. Cell. Physiol. 204, 881–888. doi: 10.1002/jcp.20351
Kim, S. R., Kubo, T., Kuroda, Y., Hojyo, M., Matsuo, T., Miyajima, A., et al. (2014). Comparative metabolome analysis of cultured fetal and adult hepatocytes in humans. J. Toxicol. Sci. 39, 717–723. doi: 10.2131/jts.39.717
Koletzko, B., Schmidt, E., Bremer, H. J., Haug, M., and Harzer, G. (1989). Effects of dietary long-chain polyunsaturated fatty acids on the essential fatty acid status of premature infants. Eur. J. Pediatr. 148, 669–675. doi: 10.1007/BF00441531
Krabbendam, L., Bakker, E., Hornstra, G., and Van Os, J. (2007). Relationship between DHA status at birth and child problem behaviour at 7 years of age. Prostaglandins Leukot. Essent. Fatty Acids 76, 29–34. doi: 10.1016/j.plefa.2006.09.004
Kramer, M. S., Aboud, F., Mironova, E., Vanilovich, I., Platt, R. W., Matush, L., et al. (2008). Breastfeeding and child cognitive development: new evidence from a large randomized trial. Arch. Gen. Psychiatry 65, 578–584. doi: 10.1001/archpsyc.65.5.578
Lain, K. Y., and Catalano, P. M. (2007). Metabolic changes in pregnancy. Clin. Obstet. Gynecol. 50, 938–948.
Lauritzen, L., and Carlson, S. E. (2011). Maternal fatty acid status during pregnancy and lactation and relation to newborn and infant status. Matern. Child. Nutr. 7(Suppl. 2), 41–58. doi: 10.1111/j.1740-8709.2011.00303.x
Leghi, G. E., and Muhlhausler, B. S. (2016). The effect of n-3 LCPUFA supplementation on oxidative stress and inflammation in the placenta and maternal plasma during pregnancy. Prostaglandins Leukot. Essent. Fatty Acids 113, 33–39. doi: 10.1016/j.plefa.2016.08.010
Lewis, R. M., Wadsack, C., and Desoye, G. (2018). Placental fatty acid transfer. Curr. Opin. Clin. Nutr. Metab. Care 21, 78–82. doi: 10.1097/mco.0000000000000443
Long, S. D., and Pekala, P. H. (1996). Regulation of GLUT4 gene expression by arachidonic acid. Evidence for multiple pathways, one of which requires oxidation to prostaglandin E2. J. Biol. Chem. 271, 1138–1144. doi: 10.1074/jbc.271.2.1138
Luo, C. L., Li, Q. Q., Chen, X. P., Zhang, X. M., Li, L. L., Li, B. X., et al. (2013). Lipoxin A4 attenuates brain damage and downregulates the production of pro-inflammatory cytokines and phosphorylated mitogen-activated protein kinases in a mouse model of traumatic brain injury. Brain Res. 1502, 1–10. doi: 10.1016/j.brainres.2013.01.037
Magnusson-Olsson, A. L., Hamark, B., Ericsson, A., Wennergren, M., Jansson, T., and Powell, T. L. (2006). Gestational and hormonal regulation of human placental lipoprotein lipase. J. Lipid Res. 47, 2551–2561. doi: 10.1194/jlr.M600098-JLR200
Makrides, M., Gibson, R. A., Mcphee, A. J., Yelland, L., Quinlivan, J., and Ryan, P. (2010). Effect of DHA supplementation during pregnancy on maternal depression and neurodevelopment of young children: a randomized controlled trial. JAMA 304, 1675–1683. doi: 10.1001/jama.2010.1507
Makrides, M., Neumann, M. A., and Gibson, R. A. (2001). Perinatal characteristics may influence the outcome of visual acuity. Lipids 36, 897–900. doi: 10.1007/s11745-001-0799-0
Mallick, R., and Duttaroy, A. K. (2021). Modulation of endothelium function by fatty acids. Mol. Cell. Biochem. doi: 10.1007/s11010-021-04260-9 [Epub ahead of print].
Mallick, R., Basak, S., and Duttaroy, A. K. (2021). Fatty acids and evolving roles of their proteins in neurological, cardiovascular disorders and cancers. Prog. Lipid. Res. 83:101116. doi: 10.1016/j.plipres.2021.101116
Marszalek, J. R., Kitidis, C., Dirusso, C. C., and Lodish, H. F. (2005). Long-chain acyl-CoA synthetase 6 preferentially promotes DHA metabolism. J. Biol. Chem. 280, 10817–10826. doi: 10.1074/jbc.M411750200
Massaro, M., Scoditti, E., Carluccio, M. A., Campana, M. C., and De Caterina, R. (2010). Omega-3 fatty acids, inflammation and angiogenesis: basic mechanisms behind the cardioprotective effects of fish and fish oils. Cell. Mol. Biol. 56, 59–82.
Matesanz, N., Park, G., Mcallister, H., Leahey, W., Devine, A., Mcveigh, G. E., et al. (2010). Docosahexaenoic acid improves the nitroso-redox balance and reduces VEGF-mediated angiogenic signaling in microvascular endothelial cells. Invest. Ophthal. Vis. Sci. 51, 6815–6825. doi: 10.1167/iovs.10-5339
McNamara, R. K. (2010). DHA deficiency and prefrontal cortex neuropathology in recurrent affective disorders. J. Nutr. 140, 864–868. doi: 10.3945/jn.109.113233
Mead, J. R., Irvine, S. A., and Ramji, D. P. (2002). Lipoprotein lipase: structure, function, regulation, and role in disease. J. Mol. Med. 80, 753–769. doi: 10.1007/s00109-002-0384-9
Middleton, P., Gomersall, J. C., Gould, J. F., Shepherd, E., Olsen, S. F., and Makrides, M. (2018). Omega-3 fatty acid addition during pregnancy. Cochrane Database Syst. Rev. 11:CD003402.
Moser, G., and Huppertz, B. (2017). Implantation and extravillous trophoblast invasion: from rare archival specimens to modern biobanking. Placenta 56, 19–26. doi: 10.1016/j.placenta.2017.02.007
Mousiolis, A. V., Kollia, P., Skentou, C., and Messinis, I. E. (2012). Effects of leptin on the expression of fatty acid-binding proteins in human placental cell cultures. Mol. Med. Rep. 5, 497–502. doi: 10.3892/mmr.2011.686
Mulder, K. A., Elango, R., and Innis, S. M. (2018). Fetal DHA inadequacy and the impact on child neurodevelopment: a follow-up of a randomised trial of maternal DHA supplementation in pregnancy. Br. J. Nutr. 119, 271–279. doi: 10.1017/S0007114517003531
Murota, S. I., Onodera, M., and Morita, I. (2000). Regulation of angiogenesis by controlling VEGF receptor. Ann. N. Y. Acad. Sci. 902, 208–212. doi: 10.1111/j.1749-6632.2000.tb06315.x
Murphy, S. P., and Abrams, B. F. (1993). Changes in energy intakes during pregnancy and lactation in a national sample of US women. Am. J. Public Health 83, 1161–1163. doi: 10.2105/ajph.83.8.1161
Nakamura, M. T., Cho, H. P., Xu, J., Tang, Z., and Clarke, S. D. (2001). Metabolism and functions of highly unsaturated fatty acids: an update. Lipids 36, 961–964. doi: 10.1007/s11745-001-0806-5
Neuringer, M. (2000). Infant vision and retinal function in studies of dietary long-chain polyunsaturated fatty acids: methods, results, and implications. Am. J. Clin. Nutr. 71, 256S–267S. doi: 10.1093/ajcn/71.1.256S
Newbern, D., and Freemark, M. (2011). Placental hormones and the control of maternal metabolism and fetal growth. Curr. Opin. Endocrinol. Diabetes Obes. 18, 409–416. doi: 10.1097/MED.0b013e32834c800d
Nie, D., Lamberti, M., Zacharek, A., Li, L., Szekeres, K., Tang, K., et al. (2000). Thromboxane A(2) regulation of endothelial cell migration, angiogenesis, and tumor metastasis. Biochem. Biophys. Res. Commun. 267, 245–251. doi: 10.1006/bbrc.1999.1840
Oddy, W. H., Kendall, G. E., Li, J., Jacoby, P., Robinson, M., De Klerk, N. H., et al. (2010). The long-term effects of breastfeeding on child and adolescent mental health: a pregnancy cohort study followed for 14 years. J. Pediatr. 156, 568–574. doi: 10.1016/j.jpeds.2009.10.020
Oddy, W. H., Li, J., Whitehouse, A. J., Zubrick, S. R., and Malacova, E. (2011). Breastfeeding duration and academic achievement at 10 years. Pediatrics 127, e137–e145. doi: 10.1542/peds.2009-3489
Ogundipe, E., Tusor, N., Wang, Y., Johnson, M. R., Edwards, A. D., and Crawford, M. A. (2018). Randomized controlled trial of brain specific fatty acid supplementation in pregnant women increases brain volumes on MRI scans of their newborn infants. Prostaglandins Leukot. Essent. Fatty Acids 138, 6–13. doi: 10.1016/j.plefa.2018.09.001
Ostadrahimi, A., Salehi-Pourmehr, H., Mohammad-Alizadeh-Charandabi, S., Heidarabady, S., and Farshbaf-Khalili, A. (2018). The effect of perinatal fish oil supplementation on neurodevelopment and growth of infants: a randomized controlled trial. Eur. J. Nutr. 57, 2387–2397. doi: 10.1007/s00394-017-1512-1
Pai, R., Szabo, I. L., Soreghan, B. A., Atay, S., Kawanaka, H., and Tarnawski, A. S. (2001). PGE(2) stimulates VEGF expression in endothelial cells via ERK2/JNK1 signaling pathways. Biochem. Biophys. Res. Commun. 286, 923–928. doi: 10.1006/bbrc.2001.5494
Pamplona, F. A., Ferreira, J., Menezes De Lima, O., Jr, Duarte, F. S., Bento, A. F., Forner, S., et al. (2012). Anti-inflammatory lipoxin A4 is an endogenous allosteric enhancer of CB1 cannabinoid receptor. Proc. Natl. Acad. Sci. U.S.A. 109, 21134–21139. doi: 10.1073/pnas.1202906109
Pan, Y., Scanlon, M. J., Owada, Y., Yamamoto, Y., Porter, C. J., and Nicolazzo, J. A. (2015). Fatty acid-binding protein 5 facilitates the blood-brain barrier transport of docosahexaenoic acid. Mol. Pharm. 12, 4375–4385. doi: 10.1021/acs.molpharmaceut.5b00580
Pandya, A. D., Das, M. K., Sarkar, A., Vilasagaram, S., Basak, S., and Duttaroy, A. K. (2016). Tube formation in the first trimester placental trophoblast cells: differential effects of angiogenic growth factors and fatty acids. Cell Biol. Int. 40, 652–661. doi: 10.1002/cbin.10601
Parellada, M., Llorente, C., Calvo, R., Gutierrez, S., Lázaro, L., Graell, M., et al. (2017). Randomized trial of omega-3 for autism spectrum disorders: effect on cell membrane composition and behavior. Eur. Neuropsychopharmacol. 27, 1319–1330. doi: 10.1016/j.euroneuro.2017.08.426
Pola, R., Gaetani, E., Flex, A., Aprahamian, T. R., Bosch-Marce, M., Losordo, D. W., et al. (2004). Comparative analysis of the in vivo angiogenic properties of stable prostacyclin analogs: a possible role for peroxisome proliferator-activated receptors. J. Mol. Cell. Cardiol. 36, 363–370. doi: 10.1016/j.yjmcc.2003.10.016
Prentice, A. M., and Goldberg, G. R. (2000). Energy adaptations in human pregnancy: limits and long-term consequences. Am. J. Clin. Nutr. 71(Suppl. 5), 1226S–1232S. doi: 10.1093/ajcn/71.5.1226s
Price, P. T., Nelson, C. M., and Clarke, S. D. (2000). Omega-3 polyunsaturated fatty acid regulation of gene expression. Curr. Opin. Lipidol. 11, 3–7. doi: 10.1097/00041433-200002000-00002
Prieto-Sanchez, M. T., Ruiz-Palacios, M., Blanco-Carnero, J. E., Pagan, A., Hellmuth, C., Uhl, O., et al. (2017). Placental MFSD2a transporter is related to decreased DHA in cord blood of women with treated gestational diabetes. Clin. Nutr. 36, 513–521. doi: 10.1016/j.clnu.2016.01.014
Punnonen, R. (1977). The relationship between serum oestradiol levels and serum triglyceride, cholesterol and phospholipid levels in normal human pregnancy. Br. J. Obstet. Gynaecol. 84, 838–845. doi: 10.1111/j.1471-0528.1977.tb12505.x
Ramakrishnan, U., Gonzalez-Casanova, I., Schnaas, L., Digirolamo, A., Quezada, A. D., Pallo, B. C., et al. (2016). Prenatal supplementation with DHA improves attention at 5 y of age: a randomized controlled trial. Am. J. Clin. Nutr. 104, 1075–1082. doi: 10.3945/ajcn.114.101071
Ramakrishnan, U., Stein, A. D., Parra-Cabrera, S., Wang, M., Imhoff-Kunsch, B., Juarez-Marquez, S., et al. (2010). Effects of docosahexaenoic acid supplementation during pregnancy on gestational age and size at birth: randomized, double-blind, placebo-controlled trial in Mexico. Food Nutr. Bull. 31, S108–S116. doi: 10.1177/15648265100312S203
Ramos, M. P., Crespo-Solans, M. D., Del Campo, S., Cacho, J., and Herrera, E. (2003). Fat accumulation in the rat during early pregnancy is modulated by enhanced insulin responsiveness. Am. J. Physiol. Endocrinol. Metab. 285, E318–E328. doi: 10.1152/ajpendo.00456.2002
Ryan, E. A., and Enns, L. (1988). Role of gestational hormones in the induction of insulin resistance. J. Clin. Endocrinol. Metab. 67, 341–347. doi: 10.1210/jcem-67-2-341
Salvado, M. D., Alfranca, A., Haeggstrom, J. Z., and Redondo, J. M. (2012). Prostanoids in tumor angiogenesis: therapeutic intervention beyond COX-2. Trends Mol. Med. 18, 233–243. doi: 10.1016/j.molmed.2012.02.002
Sanchez-Campillo, M., Ruiz-Palacios, M., Ruiz-Alcaraz, A. J., Prieto-Sanchez, M. T., Blanco-Carnero, J. E., Zornoza, M., et al. (2020). Child head circumference and placental MFSD2a expression are associated to the level of MFSD2a in maternal blood during pregnancy. Front. Endocrinol. (Lausanne) 11:38. doi: 10.3389/fendo.2020.00038
Sapieha, P., Stahl, A., Chen, J., Seaward, M. R., Willett, K. L., Krah, N. M., et al. (2011). 5-Lipoxygenase metabolite 4-HDHA is a mediator of the antiangiogenic effect of omega-3 polyunsaturated fatty acids. Sci. Transl. Med. 3:69ra12. doi: 10.1126/scitranslmed.3001571
Schaefer-Graf, U. M., Meitzner, K., Ortega-Senovilla, H., Graf, K., Vetter, K., Abou-Dakn, M., et al. (2011). Differences in the implications of maternal lipids on fetal metabolism and growth between gestational diabetes mellitus and control pregnancies. Diabet. Med. 28, 1053–1059. doi: 10.1111/j.1464-5491.2011.03346.x
Schaiff, W. T., Bildirici, I., Cheong, M., Chern, P. L., Nelson, D. M., and Sadovsky, Y. (2005). Peroxisome proliferator-activated receptor-gamma and retinoid X receptor signaling regulate fatty acid uptake by primary human placental trophoblasts. J. Clin. Endocrinol. Metab. 90, 4267–4275. doi: 10.1210/jc.2004-2265
Scoditti, E., Massaro, M., Carluccio, M. A., Distante, A., Storelli, C., and De Caterina, R. (2010). PPARgamma agonists inhibit angiogenesis by suppressing PKCalpha- and CREB-mediated COX-2 expression in the human endothelium. Cardiovasc. Res. 86, 302–310. doi: 10.1093/cvr/cvp400
Sherry, C. L., Oliver, J. S., and Marriage, B. J. (2015). Docosahexaenoic acid supplementation in lactating women increases breast milk and plasma docosahexaenoic acid concentrations and alters infant omega 6:3 fatty acid ratio. Prostaglandins Leukot. Essent. Fatty Acids 95, 63–69. doi: 10.1016/j.plefa.2015.01.005
Simopoulos, A. P. (1989). Summary of the NATO advanced research workshop on dietary omega 3 and omega 6 fatty acids: biological effects and nutritional essentiality. J. Nutr. 119, 521–528. doi: 10.1093/jn/119.4.521
Sivan, E., Homko, C. J., Chen, X., Reece, E. A., and Boden, G. (1999). Effect of insulin on fat metabolism during and after normal pregnancy. Diabetes 48, 834–838. doi: 10.2337/diabetes.48.4.834
Smith, W. L. (1989). The eicosanoids and their biochemical mechanisms of action. Biochem. J. 259, 315–324. doi: 10.1042/bj2590315
Spencer, L., Mann, C., Metcalfe, M., Webb, M., Pollard, C., Spencer, D., et al. (2009). The effect of omega-3 FAs on tumour angiogenesis and their therapeutic potential. Eur. J. Cancer 45, 2077–2086. doi: 10.1016/j.ejca.2009.04.026
Stevens, L. J., Zentall, S. S., Deck, J. L., Abate, M. L., Watkins, B. A., Lipp, S. R., et al. (1995). Essential fatty acid metabolism in boys with attention-deficit hyperactivity disorder. Am. J. Clin. Nutr. 62, 761–768. doi: 10.1093/ajcn/62.4.761
Storch, J., and Thumser, A. E. (2010). Tissue-specific functions in the fatty acid-binding protein family. J. Biol. Chem. 285, 32679–32683. doi: 10.1074/jbc.R110.135210
Sun, G. Y., Shelat, P. B., Jensen, M. B., He, Y., Sun, A. Y., and Simonyi, A. (2010). Phospholipases A2 and inflammatory responses in the central nervous system. Neuromol. Med. 12, 133–148. doi: 10.1007/s12017-009-8092-z
Szymczak, M., Murray, M., and Petrovic, N. (2008). Modulation of angiogenesis by omega-3 polyunsaturated fatty acids is mediated by cyclooxygenases. Blood 111, 3514–3521. doi: 10.1182/blood-2007-08-109934
Takahashi, S. (2011). Vascular endothelial growth factor (VEGF). VEGF receptors and their inhibitors for antiangiogenic tumor therapy. Biol. Pharm. Bull. 34, 1785–1788. doi: 10.1248/bpb.34.1785
Tobin, K. A., Johnsen, G. M., Staff, A. C., and Duttaroy, A. K. (2009). Long-chain polyunsaturated fatty acid transport across human placental choriocarcinoma (BeWo) cells. Placenta 30, 41–47. doi: 10.1016/j.placenta.2008.10.007
Tsuji, M., Murota, S. I., and Morita, I. (2003). Docosapentaenoic acid (22:5, n-3) suppressed tube-forming activity in endothelial cells induced by vascular endothelial growth factor. Prostaglandins Leukot. Essent. Fatty Acids 68, 337–342. doi: 10.1016/s0952-3278(03)00025-5
Uauy, R., and Hoffman, D. R. (2000). Essential fat requirements of preterm infants. Am. J. Clin. Nutr. 71, 245S–250S. doi: 10.1093/ajcn/71.1.245S
Uauy, R., Hoffman, D. R., Peirano, P., Birch, D. G., and Birch, E. E. (2001). Essential fatty acids in visual and brain development. Lipids 36, 885–895. doi: 10.1007/s11745-001-0798-1
Van Elswyk, M. E., and Kuratko, C. (2009). Achieving adequate DHA in maternal and infant diets. J. Am. Diet Assoc. 109, 403–404. doi: 10.1016/j.jada.2009.01.004
Wahle, K. W., Rotondo, D., and Heys, S. D. (2003). Polyunsaturated fatty acids and gene expression in mammalian systems. Proc. Nutr. Soc. 62, 349–360. doi: 10.1079/pns2003249
Wang, D., Wang, H., Brown, J., Daikoku, T., Ning, W., Shi, Q., et al. (2006). CXCL1 induced by prostaglandin E2 promotes angiogenesis in colorectal cancer. J. Exp. Med. 203, 941–951. doi: 10.1084/jem.20052124
Warth, M. R., Arky, R. A., and Knopp, R. H. (1975). Lipid metabolism in pregnancy. II. Altered lipid composition in intermediage, very low, low and high-density lipoprotein fractions. J. Clin. Endocrinol. Metab. 41, 649–655. doi: 10.1210/jcem-41-4-649
Waterman, I. J., Emmison, N., and Dutta-Roy, A. K. (1998). Characterisation of triacylglycerol hydrolase activities in human placenta. Biochim. Biophys. Acta1394, 169–176. doi: 10.1016/s0005-2760(98)00105-2
Waterman, I. J., Emmison, N., Sattar, N., and Dutta-Roy, A. K. (2000). Further characterization of a novel triacylglycerol hydrolase activity (pH 6.0 optimum) from microvillous membranes from human term placenta. Placenta 21, 813–823. doi: 10.1053/plac.2000.0572
Weedon-Fekjaer, M. S., Duttaroy, A. K., and Nebb, H. I. (2005). Liver X receptors mediate inhibition of hCG secretion in a human placental trophoblast cell line. Placenta 26, 721–728. doi: 10.1016/j.placenta.2004.10.005
Wilke, M. S., and Clandinin, M. T. (2005). Influence of dietary saturated fatty acids on the regulation of plasma cholesterol concentration. Lipids 40, 1207–1213. doi: 10.1007/s11745-005-1487-9
Yan, Y., Peng, H., Wang, P., Wang, H., and Dong, M. (2016). Increased expression of fatty acid binding protein 4 in preeclamptic Placenta and its relevance to preeclampsia. Placenta 39, 94–100. doi: 10.1016/j.placenta.2016.01.014
Yang, S. P., Morita, I., and Murota, S. I. (1998). Eicosapentaenoic acid attenuates vascular endothelial growth factor-induced proliferation via inhibiting Flk-1 receptor expression in bovine carotid artery endothelial cells. J. Cell. Physiol. 176, 342–349. doi: 10.1002/(SICI)1097-4652(199808)176:2<342::AID-JCP12>3.0.CO;2-5
Zamai, N., Cortie, C. H., Jarvie, E. M., Onyiaodike, C. C., Alrehalli, A., Francois, M., et al. (2020). In pregnancy, maternal HDL is specifically enriched in, and carries the highest proportion of, DHA in plasma. Prostaglandins Leukot. Essent. Fatty Acids 163:102209. doi: 10.1016/j.plefa.2020.102209
Zhang, Y., and Daaka, Y. (2011). PGE2 promotes angiogenesis through EP4 and PKA Cgamma pathway. Blood 118, 5355–5364. doi: 10.1182/blood-2011-04-350587
Keywords: arachidonic acid, docosahexaenoic acid, placenta, fetus, FATP, FABP, angiogenesis, fetal development
Citation: Duttaroy AK and Basak S (2022) Maternal Fatty Acid Metabolism in Pregnancy and Its Consequences in the Feto-Placental Development. Front. Physiol. 12:787848. doi: 10.3389/fphys.2021.787848
Received: 01 October 2021; Accepted: 30 December 2021;
Published: 20 January 2022.
Edited by:
Vincenza Cifarelli, Washington University in St. Louis, United StatesReviewed by:
Marisol Castillo-Castrejon, University of Oklahoma Health Sciences Center, United StatesCopyright © 2022 Duttaroy and Basak. This is an open-access article distributed under the terms of the Creative Commons Attribution License (CC BY). The use, distribution or reproduction in other forums is permitted, provided the original author(s) and the copyright owner(s) are credited and that the original publication in this journal is cited, in accordance with accepted academic practice. No use, distribution or reproduction is permitted which does not comply with these terms.
*Correspondence: Asim K. Duttaroy, YS5rLmR1dHRhcm95QG1lZGlzaW4udWlvLm5v
Disclaimer: All claims expressed in this article are solely those of the authors and do not necessarily represent those of their affiliated organizations, or those of the publisher, the editors and the reviewers. Any product that may be evaluated in this article or claim that may be made by its manufacturer is not guaranteed or endorsed by the publisher.
Research integrity at Frontiers
Learn more about the work of our research integrity team to safeguard the quality of each article we publish.