- 1Department of Physiology, Radboud University Nijmegen Medical Center, Nijmegen, Netherlands
- 2Department of Pediatrics, Philipps-University Marburg, Marburg, Germany
- 3Department of Biomedicine, Aarhus University, Aarhus, Denmark
- 4Department of Biomedical Sciences, University of Copenhagen, Copenhagen, Denmark
- 5Department of Endocrinology and Nephrology, North Zealand Hospital, Hillerød, Denmark
- 6Department of Nephrology, Radboud University Nijmegen Medical Center, Nijmegen, Netherlands
- 7Department of Cardiovascular and Renal Research, Institute of Molecular Medicine, University of Southern Denmark, Odense, Denmark
Arginine vasopressin (AVP) stimulates the concentration of renal urine by increasing the principal cell expression of aquaporin-2 (AQP2) water channels. Prostaglandin E2 (PGE2) and prostaglandin2α (PGF2α) increase the water absorption of the principal cell without AVP, but PGE2 decreases it in the presence of AVP. The underlying mechanism of this paradoxical response was investigated here. Mouse cortical collecting duct (mkpCCDc14) cells mimic principal cells as they endogenously express AQP2 in response to AVP. PGE2 increased AQP2 abundance without desmopressin (dDAVP), while in the presence of dDAVP, PGE2, and PGF2α reduced AQP2 abundance. dDAVP increased the cellular PGD2 and PGE2 release and decreased the PGF2α release. MpkCCD cells expressed mRNAs for the receptors of PGE2 (EP1/EP4), PGF2 (FP), and TxB2 (TP). Incubation with dDAVP increased the expression of EP1 and FP but decreased the expression of EP4. In the absence of dDAVP, incubation of mpkCCD cells with an EP4, but not EP1/3, agonist increased AQP2 abundance, and the PGE2-induced increase in AQP2 was blocked with an EP4 antagonist. Moreover, in the presence of dDAVP, an EP1/3, but not EP4, agonist decreased the AQP2 abundance, and the addition of EP1 antagonists prevented the PGE2-mediated downregulation of AQP2. Our study shows that in mpkCCDc14 cells, reduced EP4 receptor and increased EP1/FP receptor expression by dDAVP explains the differential effects of PGE2 and PGF2α on AQP2 abundance with or without dDAVP. As the V2R and EP4 receptor, but not the EP1 and FP receptor, can couple to Gs and stimulate the cyclic adenosine monophosphate (cAMP) pathway, our data support a view that cells can desensitize themselves for receptors activating the same pathway and sensitize themselves for receptors of alternative pathways.
Introduction
To prevent dehydration, an adequate maintenance of water homeostasis is essential. In this process, the kidney plays a critical role. In response to hypernatremia or hypovolemia, arginine vasopressin (AVP) is released from the posterior pituitary gland. Subsequently, binding of AVP to the basolateral vasopressin type-2 receptor (V2R) in the connecting tubule and collecting duct principal cells in the kidney results in the redistribution of aquaporin-2 (AQP2) water channels from intracellular vesicles to the apical membrane, greatly increasing the osmotic water permeability, a prerequisite for forming concentrated urine (Knepper, 1997). In addition, AVP also increases the expression of AQP2 via phosphorylation of the cyclic adenosine monophosphate (cAMP)-responsive element binding protein, which activates transcription from the AQP2 promoter (Terris et al., 1996; Matsumura et al., 1997; Yasui et al., 1997).
Besides AVP, several other signaling molecules regulate the water balance by antagonizing the AVP-induced water transport (Boone and Deen, 2008). One such group of molecules is the prostaglandins (Figure 1). Prostaglandins can bind to their unique G-protein-coupled receptors (i.e., DP, FP, IP, and TP) or to one or more of four different PGE2 receptors (i.e., EP1, EP2, EP3, and EP4). Some of these receptors (i.e., DP, EP2, EP4, and IP) are Gs-coupled and thus increase intracellular cAMP levels when activated, whereas others are coupled to Gi (i.e., EP3 and FP), reducing the cAMP synthesis, and/or Gq (i.e., EP1, FP, and TP), inducing calcium mobilization (Breyer et al., 1998; Hebert et al., 2005; Hao and Breyer, 2008).
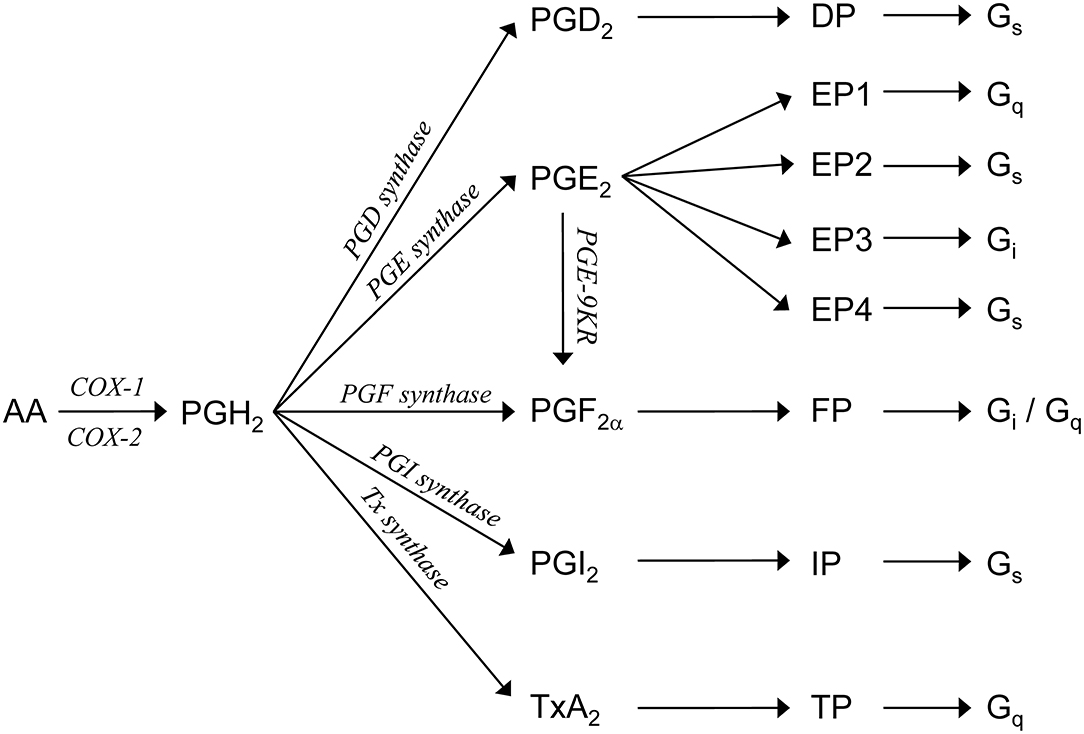
Figure 1. Prostaglandin synthesis. Arachidonic acid (AA) is metabolized by COX1 or COX2 to PGH2. PGH2 is enzymatically converted, by specific synthases [prostaglandin D (PGD) synthase, prostaglandin E (PGE) synthase, prostaglandin F (PGF) synthase, prostaglandin I (PGI) synthase, and thromboxane synthase] or prostaglandin E 9-ketoreductase (PGE-9KR), to one of five primary prostanoids, namely, PGI2, PGD2, PGE2, PGF2α, or TxA2. Each prostanoid interacts with distinct members of a subfamily of the G-protein-coupled receptors. PGI2 activates the IP receptor, PGD2 activates the DP receptor, PGF2α activates the FP receptor, and TxA2 activates the TP receptor. PGE2 interacts with one of four distinct EP receptors.
Of the different prostaglandins, PGE2 in particular has been shown to decrease AVP-stimulated water reabsorption in perfused collecting ducts (Hebert et al., 1990; Nadler et al., 1992; Sakairi et al., 1995). In addition, PGE2 is also involved in the pathological regulation of water reabsorption. PGE2 has been suggested to play an important role in the development of lithium-induced nephrogenic diabetes insipidus (NDI). This is based on the observation that the renal expression of the enzyme cyclooxygenase 2 (COX-2), involved in prostaglandin production, is markedly increased in lithium-treated mice, resulting in an increased excretion of urinary PGE2 (Rao et al., 2005). Also, treatment with a COX-2 inhibitor alleviated lithium-induced polyuria (Kim et al., 2008). Similarly, in the bilateral ureteral obstruction, associated with AQP2 downregulation, COX-2 protein abundance as well as the concentrations of PGE2 and other prostanoids are increased in the kidney inner medulla (Norregaard et al., 2010). Administration of COX-2 inhibitor prevents the increase of urinary PGE2 and the downregulation of AQP2 in inner medullary collecting ducts seen after the bilateral ureteral obstruction (Norregaard et al., 2005). In addition, PGE2 has recently been suggested to be instrumental in the increased free water reabsorption and volume expansion, leading to thiazide-induced hyponatremia (Ware et al., 2017). Besides PGE2, PGF2α can also inhibit AVP-stimulated water permeability in the collecting duct (Zook and Strandhoy, 1981; Hebert et al., 2005).
Paradoxically, PGE2 increases the osmotic water permeability in the absence of AVP (Hebert et al., 1990; Sakairi et al., 1995). The underlying mechanism of this switch in function, however, is still unclear. Therefore, in the present study, we utilized the cortical collecting duct (mpkCCDc14) cells of a mouse as a model system for the renal principal cell to delineate how prostaglandins can exert their diverse effects on the principal cell water reabsorption in the presence or absence of AVP.
Materials and Methods
Cell Culture
Mouse mpkCCDc14 cells were maintained essentially as described (Hasler et al., 2002). Cells were seeded at a density of 1.5 × 105 cells/cm2 on semipermeable filters (Transwell®, 0.4 μm pore size, Corning Costar, Cambridge, MA) and cultured for 8 days. Unless stated otherwise, the cells were exposed to 1 nM of the V2R agonist desmopressin (dDAVP) at the basolateral side during the last 96 h, to maximally induce the AQP2 expression (Li et al., 2006). Cells were incubated with 10 μM indomethacin, 1 μM PGE2 (both Sigma, St. Louis, MO, USA), 1 μM PGF2α (Calbiochem, San Diego, CA), 300 nM of EP1/EP3 agonists sulprostone (Sigma, St. Louis, MO, USA), 1 μM of EP4 agonists CAY10580, 0.5 μM of EP4 antagonist Gw627368, 2.5 nM of the EP4 antagonist L161982, 20 μM of EP1 antagonist Sc-51089, or 100 nM of EP1 antagonist Ono-8711 (all Cayman Chemical, Ann Arbor, Michigan, USA) during the last 48 h. The medium was replaced after 24 h, or in experiments using the EP agonists or antagonists, the medium was replaced every 12 h.
Immunoblotting
MpkCCDc14 cells grown on 1.13 cm2 filters were lysed using 200 μl Laemmli. Sodium dodecyl sulfate-polyacrylamide gel electrophoresis, blotting, and blocking of the polyvinylidene fluoride membranes were carried out as described previously (Kamsteeg et al., 1999). Membranes were incubated for 16 h with 1:3,000-diluted affinity-purified rabbit anti-AQP2 antibodies [R7 (Deen et al., 1994) or Novus Biologicals, Littleton, CO] in Tris-buffered saline Tween-20 (TBS-T) supplemented with 1% w/v nonfat dried milk. Blots were incubated for 1 h with 1:5,000-diluted goat anti-rabbit IgGs (Sigma, St. Louis, MO) as secondary antibodies coupled to horseradish peroxidase. Proteins were visualized using enhanced chemiluminescence (ECL, Pierce, Rockford, IL).
(Quantitative) Reverse-Transcriptase Polymerase Chain Reaction
MpkCCDc14 cells were grown as described above, and total RNA was isolated using the TriZol extraction reagent (Gibco, Life Technologies, Rockville, MD), according to the instructions of the manufacturer. To remove genomic DNA, total RNA was treated with DNase (Promega, Madison, WI) for 1 h at 37°C, extracted with phenol/chloroform, and precipitated. RNA was reverse-transcribed into cDNA using Moloney Murine Leukemia Virus reverse-transcriptase and random primers (Promega, Madison, WI). During cDNA production, a control reaction without the reverse-transcriptase enzyme was conducted to exclude genomic DNA amplification. Exon overlapping primers were designed for prostaglandin receptors (see Table 1). Amplification was performed using the cDNA equivalent of 5 ng RNA for 40 cycles (i.e., 95°C 45 s, 50°C 1 min, and 72°C 1.5 min). β-actin was used as a positive control for cDNA amplification. cDNA from the tissue reported to express the particular receptor was taken along as a positive control. The proper identity of products was confirmed using the restriction analysis.
SYBR Green real-time quantitative reverse-transcriptase polymerase chain reaction (RT-PCR) was performed on an iQ5 Real-Time PCR Detection System from Bio-Rad by utilizing the SYBR Green PCR Master Mix (Applied Biosystems Foster City, CA). Signals for the ribosomal 18S were used to normalize for differences in the amount of starting cDNA.
Prostanoid Analysis
Samples were prepared as described previously (Schweer et al., 1994) with minor modifications. In brief, cell culture supernatants were spiked with ~1 ng of deuterated internal standards, and the methoximes were obtained through the reaction with an O-methylhydroxylamine hydrochloride-acetate buffer. After acidification to pH 3.5, prostanoid derivatives were extracted, and the pentafluorobenzylesters were formed. Samples were purified by thin layer chromatography, and a broad zone with RF 0.03–0.4 was eluted. After withdrawal of the organic layer, trimethylsilyl ethers were prepared by the reaction with bis(trimethylsilyl)-trifluoroacetamide and thereafter, subjected to the gas chromatography-tandem mass spectrometry (GC/MS/MS) analysis on a Finnigan MAT TSQ700 GC/MS/MS (Thermo Electron Corp., Dreieich, Germany) equipped with a Varian 3400 gas chromatograph (Palo Alto, CA) and a CTC A200S autosampler (CTC Analytics, Zwingen, Switzerland).
Statistical Analysis
Student's t-test was applied to compare two groups with Gaussian distribution. Comparisons of more than two groups were performed using a one-way ANOVA followed by a Dunnett multiple comparison test. Levene's test was used to compare variances. P-values < 0.05 were considered significant. Immunoblotting signals were analyzed using the Bio-Rad software. Data are presented as mean ± standard error of the mean (SEM).
Results
In MpkCCD Cells, Regulation of AQP2 Expression by Prostanoids Is Modulated by AVP
To analyze the effect of PGE2 on the AQP2 expression, mpkCCDc14 cells were grown to confluence for 8 days, either with or without 1 nM of the V2R agonist dDAVP for the last 4 days and with or without 1 μM PGE2 during the last 48 h. PGE2 increased the AQP2 abundance in the absence of dDAVP but decreased it in the presence of dDAVP (Figure 2). In the presence of dDAVP, 1 μM PGF2α also decreased the AQP2 abundance.
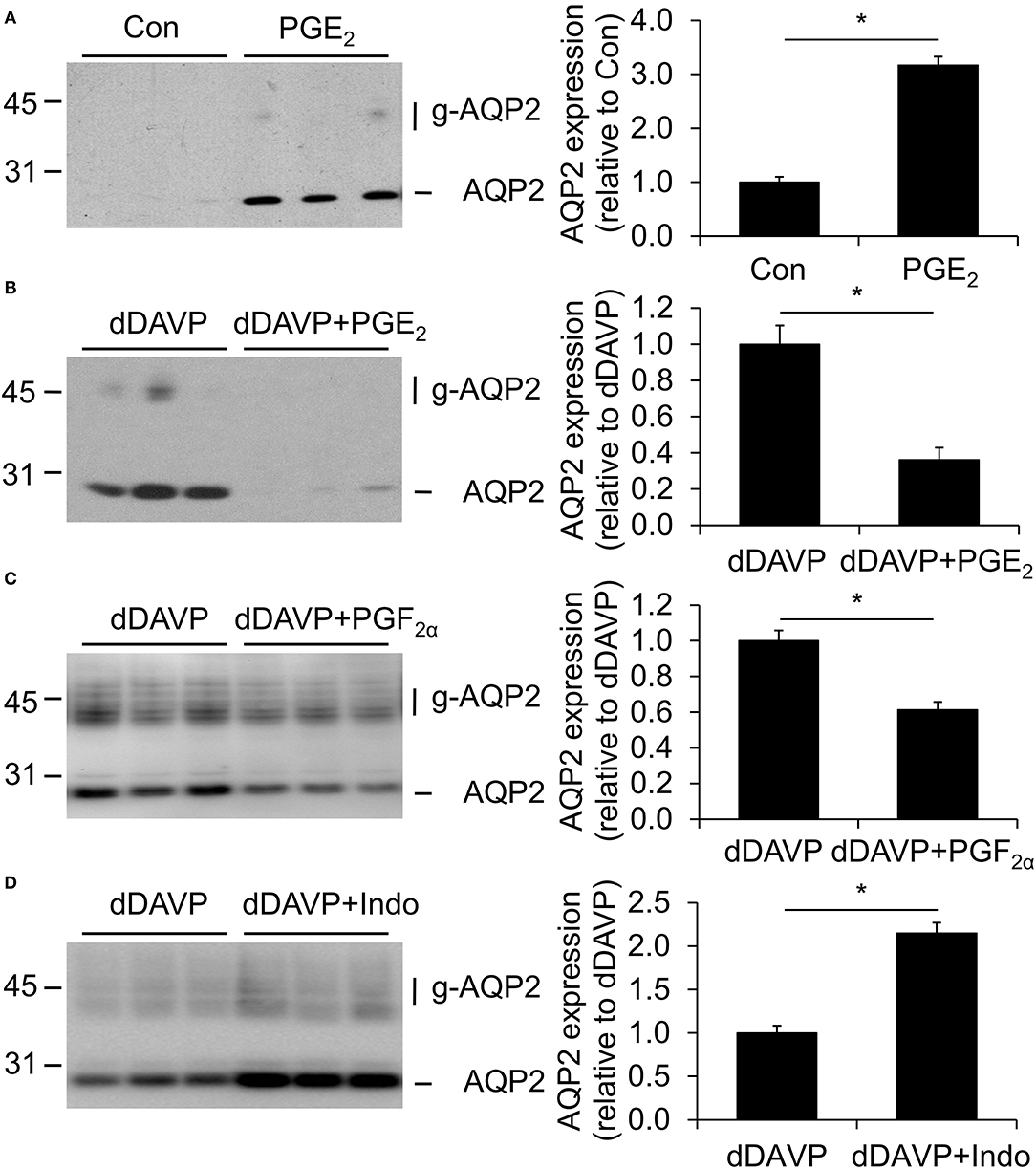
Figure 2. Effect of prostaglandins on aquaporin-2 (AQP2) expression. MpkCCDc14 cells were grown for 8 days, either with or without 1 nM desmopressin (dDAVP) stimulation for the last 4 days and with or without 1 μM PGE2 (A,B), 1 μM PGF2α (C), or 10 μM indomethacin (D) during the last 48 h. Cells were lysed and subjected to immunoblotting for AQP2. Molecular masses (in kDa) are indicated on the left. Nonglycosylated (AQP2) and complex-glycosylated (g-AQP2) forms of AQP2 are detected and densitometrically quantified. Significant differences from control or dDAVP alone (p < 0.05) are indicated by an asterisk. Bars are mean values of nine filters per condition (±SEM).
To test whether COX inhibition affects the dDAVP-induced AQP2 expression, cells were grown as described above, i.e., the last 4 days in the presence of dDAVP and the last 48 h in the presence of 10 μM indomethacin. Subsequent immunoblotting showed an increased AQP2 abundance with indomethacin (Figure 2), suggesting that dDAVP-treated mpkCCDc14 cells produce prostanoids, which decrease the AQP2 abundance.
dDAVP Changes Prostanoid Production in MpkCCD Cells
To determine whether mpkCCDc14 cells produce PGE2 or other prostanoids, and whether the presence of dDAVP affects the release of these prostanoids, cells were grown as above, i.e., with or without dDAVP for the last 4 days, after which the medium was collected and analyzed for the presence of prostanoids. Prostaglandin concentrations from the fresh medium were subtracted. The major prostanoids released from control cells were PGE2 and PGF2α, while levels of PGD2, 6-keto-PGF1α (i.e., a stable metabolite of PGI2), and TxB2 (i.e., a stable metabolite of TxA2) were lower and bordering on their detection limit (Figure 3). The dDAVP treatment significantly increased the production of PGD2 and PGE2, while PGF2α levels were decreased. No effect of dDAVP was observed on the release of 6-keto-PGF1α or TxB2.
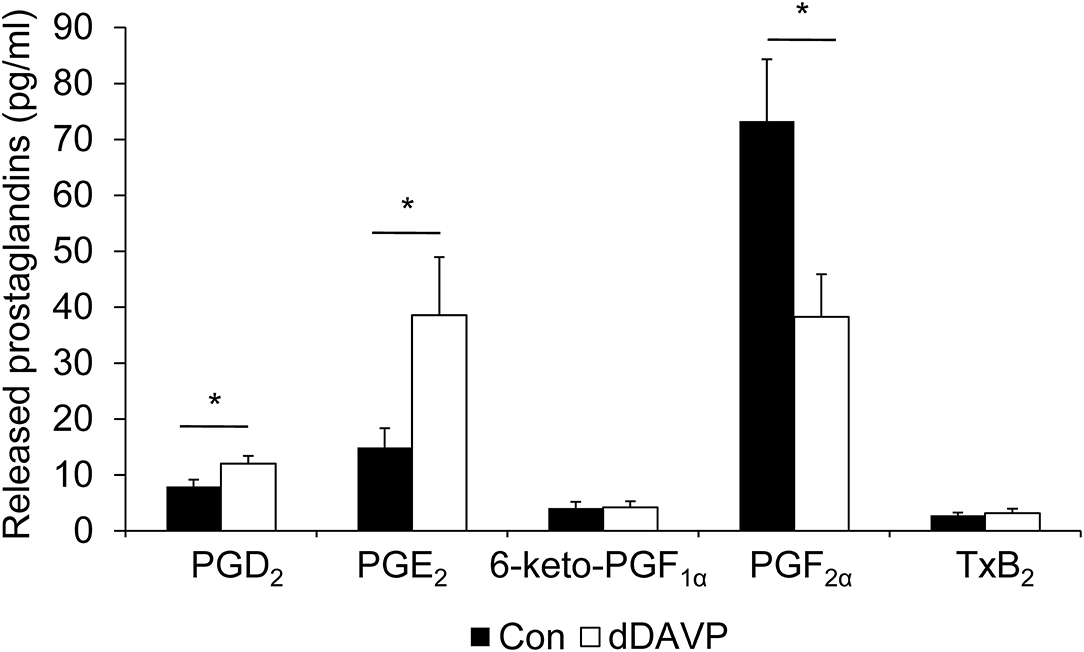
Figure 3. Effect of dDAVP on prostaglandin production. MpkCCDc14 cells were grown for 8 days and treated with or without (con) 1 nM dDAVP for the last 4 days. The medium from both sides, incubated with the cells for 24 h, was collected, and prostaglandin concentrations were determined. Bars are mean values of eight filters per condition (±SEM). Significant differences from control (p < 0.05) are indicated by an asterisk.
dDAVP Differentially Affects Prostanoid Receptor mRNA Expression in MpkCCD Cells
The effects of prostaglandins on the AQP2 expression are conferred by effects on their respective receptors. Immunoblotting was unsuitable to examine the expression of the individual prostaglandin receptors (not shown). Therefore, we determined the mRNA expression of the prostaglandin receptors in mpkCCD cells using the RT-PCR.
From unstimulated cells, cDNA products of the expected size were obtained for EP1, EP4, FP, and TP receptors (Figure 4A). While PCR products for EP2, EP3, or DP receptors were found in control tissues, no products were obtained in mpkCCDc14 cells, indicating that these receptors are not expressed. A detectable expression of the IP receptor was inconsistent. The same receptors were expressed in mpkCCD cells treated with dDAVP (not shown).
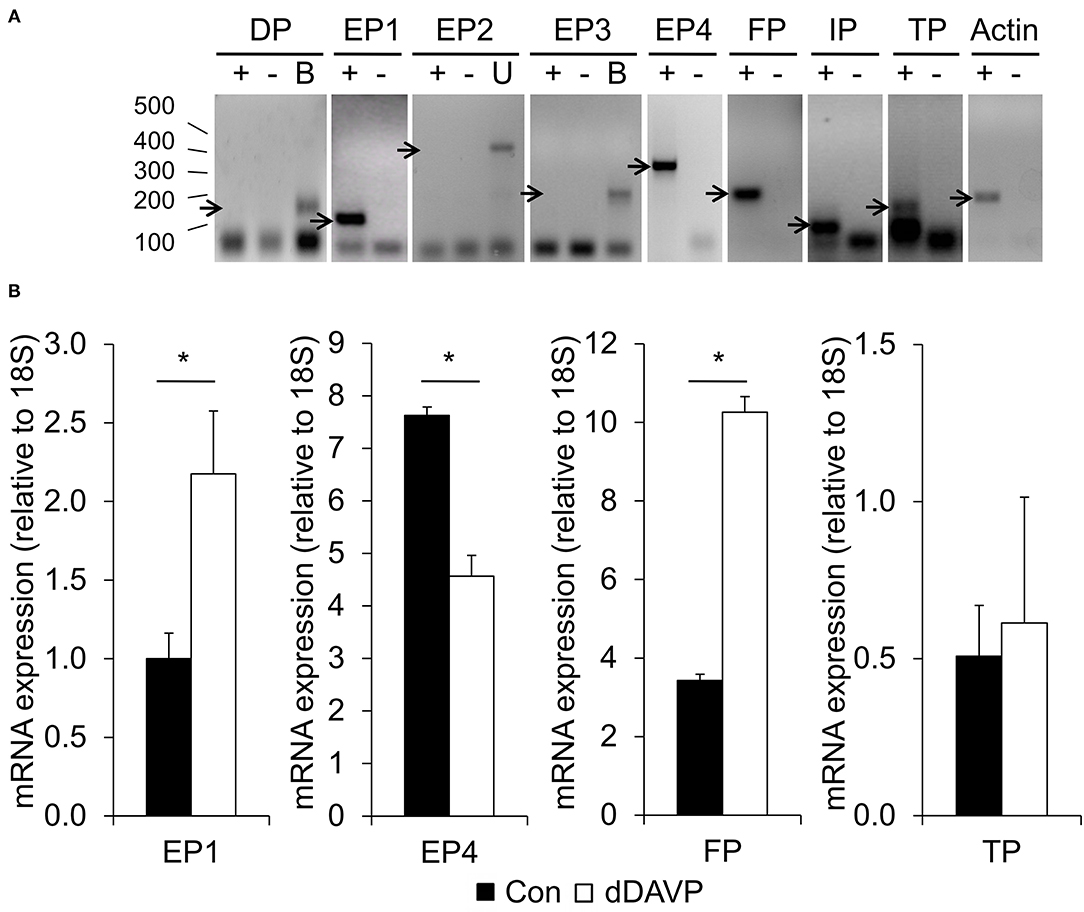
Figure 4. Prostaglandin receptor expression. (A) MpkCCDc14 cells were grown for 8 days. Cells were lysed, total RNA was isolated, and RNA was reverse-transcribed into cDNA. By using the reverse-transcriptase polymerase chain reaction (RT-PCR), the expression of the prostaglandin receptors was analyzed. β-actin was used as a positive control for cDNA amplification. ± = with or without reverse transcriptase during the cDNA production. B, brain, U, uterus. Sizes in bp are indicated on the left. Arrows point at product of expected size. (B) MpkCCDc14 cells were grown for 8 days and incubated with or without (con) 1 nM dDAVP for the last 4 days. Total RNA was isolated, RNA was reverse-transcribed into cDNA, and the relative expression of the prostaglandin receptors was analyzed by performing the quantitative (q)RT-PCR. The signals obtained from the house-keeping 18S were used to normalize for difference in the amount of starting cDNA. Bars are mean values of eight filters per condition (±SEM). Significant differences (p < 0.05) from control are indicated by an asterisk.
To test if the levels of the expressed prostanoid receptors were influenced by dDAVP, we determined their relative expression by using the qRT-PCR. dDAVP increased the expression of the EP1 and FP receptor, while the expression of the EP4 receptor was significantly decreased (Figure 4B). No difference was detected in the expression of the TP receptor.
Modulation of PGE2 Receptor Subtype Expression by dDAVP Explains the Differential Effect of Prostanoids on AQP2 Abundance
As the EP1/FP receptors and EP4 receptors are coupled to Gi/Gq and Gs (Figure 1), respectively, an altered activation of these receptors due to their changes in the expression with dDAVP could explain the differential effect of prostanoids on the AQP2 abundance. To further explore the roles of the different PGE2 receptor subtypes in mediating the effects of PGE2 on AQP2 levels, we used EP receptor-specific agonists and antagonists.
MpkCCD cells were grown as described above, i.e., stimulated with or without dDAVP, and incubated with the EP4 agonist CAY10580 or the EP1/EP3 agonist sulprostone (Kiriyama et al., 1997; Billot et al., 2003) during the last 48 h. As the EP3 receptor is not expressed in mpkCCD cells (Figure 4A), sulprostone will act as a specific EP1 agonist in these cells. Consistent with a contribution of EP4 to the prostanoid-stimulated AQP2 abundance in unstimulated cells, CAY10580 and PGE2 increased the AQP2 abundance as compared with unstimulated cells or cells incubated with sulprostone (Figure 5A). In cells stimulated with dDAVP, however, CAY10580 did not affect the AQP2 abundance, while both sulprostone and PGE2 decreased the AQP2 abundance, therewith, illustrating an important contribution of the EP1 receptor in reducing the AQP2 abundance in dDAVP-stimulated mpkCCD, cells (Figure 5B).
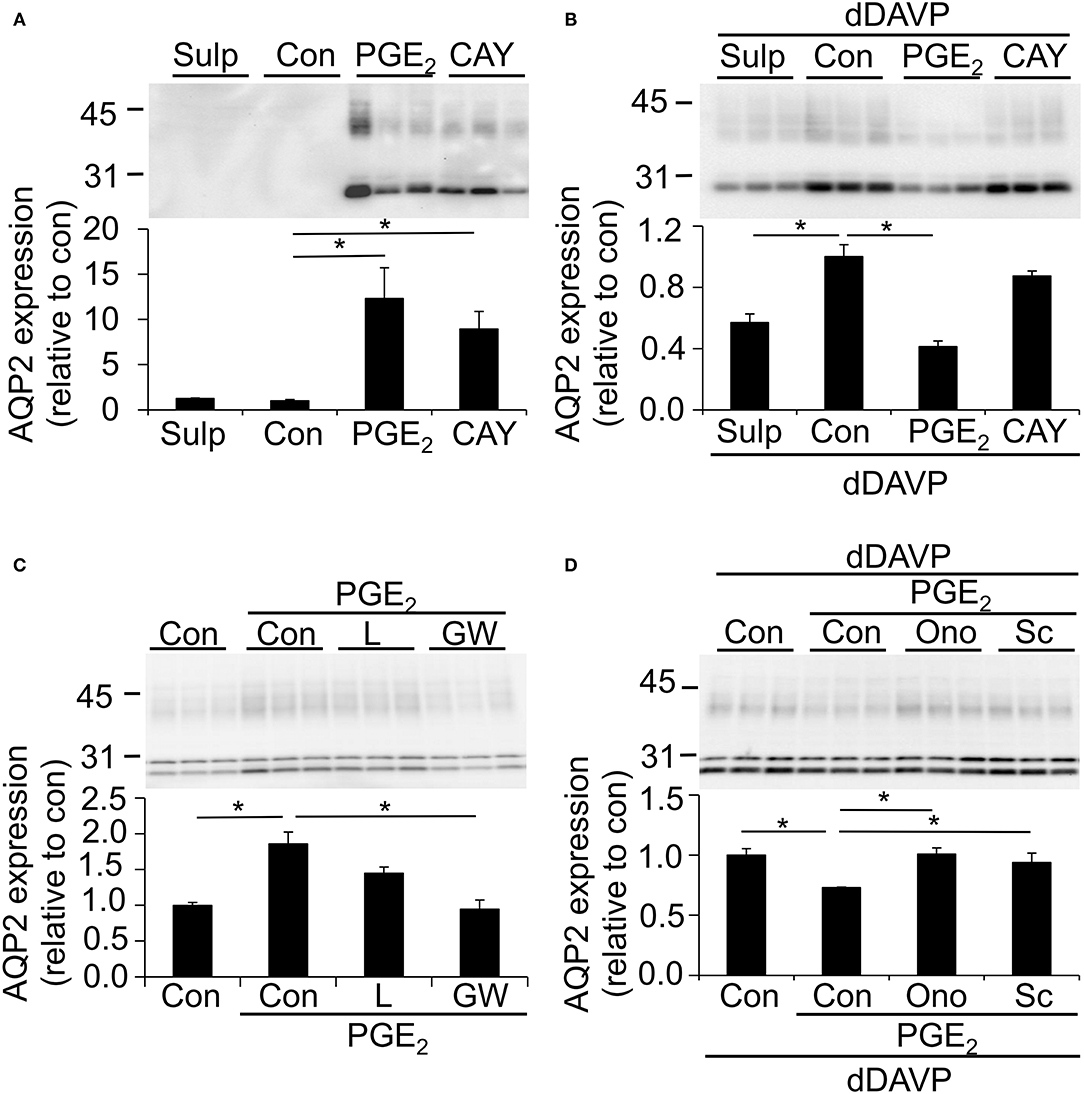
Figure 5. Effect of different PGE2 receptor agonists and antagonists on the AQP2 expression. MpkCCDc14 cells were grown for 8 days, either with our without 1 nM dDAVP stimulation for the last 4 days and with or without 1 μM PGE2 during the last 48 h. (A,B) Cells were incubated with1 μM sulprostone (Sulp) or 300 nM Cayman10580 (Cay) during the last 48 h. (C) Cells were incubated with 0.5 μM of EP4 antagonist Gw627368 (GW) or 2.5 nM of the EP4 antagonist L161982 (L) during the last 48 h. (D) Cells were incubated with 20 μM of EP1 antagonist Sc-51089 (Sc) or 100 nM of EP1 antagonist Ono-8711 (Ono) during the last 48 h. Cells were lysed and subjected to immunoblotting for AQP2. Molecular masses (in kDa) are indicated on the left. Nonglycosylated AQP2 (29 kDa) and complex-glycosylated (40–45 kDa) forms of AQP2 are detected and densitometrically quantified. Significant differences from control (con, p < 0.05) are indicated by an asterisk. Bars are mean values of 9 (A,B) or 6 (C,D) filters per condition (±SEM).
To further investigate the role of the EP4 receptor in the prostanoid-induced AQP2 abundance, mpkCCD cells were treated with PGE2 with or without the EP4 antagonists L161982 and Gw627368. While PGE2 alone again increased the AQP2 abundance significantly, Gw627368 completely blocked the PGE2-mediated AQP2 increase, whereas L161982 had a tendency to decrease the AQP2 expression relative to cells treated with PGE2 alone (Figure 5C).
To investigate the role of EP1 in the PGE2-mediated AQP2 decrease in dDAVP-treated cells, mpkCCD cells were stimulated with dDAVP and incubated with or without PGE2 and the specific EP1 antagonists Sc-51089 or Ono-8711. Both antagonists fully prevented the PGE2-mediated downregulation of AQP2 (Figure 5D), illustrating an important contribution of the EP1 receptor in the regulation of AQP2.
Discussion
Prostanoids Affect AQP2 Expression in MpkCCD Cells
Prostaglandin E2 reduce the AVP-stimulated water reabsorption in the collecting duct (Hebert et al., 1990; Nadler et al., 1992), while in the absence of AVP, ex vivo water permeability is increased by PGE2 (Sakairi et al., 1995). A short-term action of PGE2 is to alter the localization of AQP2 at the plasma membrane (Zelenina et al., 2000; Nejsum et al., 2005; Olesen et al., 2011). Here, we showed that long-term PGE2 affects the abundance of the AQP2 protein. PGE2 attenuated the dDAVP-induced AQP2 expression, while PGE2 stimulated the AQP2 abundance in the absence of dDAVP. In addition, dDAVP-stimulated AQP2 levels were decreased after the application of PGF2α, explaining the inhibition of water reabsorption in the collecting duct observed after the PGF2α treatment (Zook and Strandhoy, 1981; Hebert et al., 2005). Furthermore, blocking the prostaglandin production by indomethacin increased the AQP2 abundance, showing that the dDAVP-stimulated AQP2 abundance is likely reduced due to the effects of endogenously produced prostaglandins. The major prostaglandins produced in mpkCCD cells are PGE2 and PGF2α. The dDAVP stimulation significantly increased both the production of PGE2 and PGD2, while levels of PGF2α were decreased. In agreement with these findings, it has been shown that AVP stimulates the PGE2 synthesis in isolated collecting ducts (Schlondorff et al., 1985; Bonvalet et al., 1987).
In MpkCCD Cells, dDAVP-Induced Changes in PGE2 Receptor Expression and Activation Explain the Different Effects of PGE2 on AQP2 Abundance in the Presence or Absence of AVP
Consistent with previous studies, the PGE2 receptors expressed in mpkCCDc14 cells are EP1 and EP4 (Olesen et al., 2016). Our experiments using receptor antagonists and agonists show that it is the EP4 receptor that is involved in the stimulatory effect of PGE2 on the AQP2 expression in mpkCCD cells. The EP4 receptor can couple to Gs-stimulated cAMP generation, thereby activating the same pathway as AVP. Incubation with dDAVP increased the expression of the EP1 receptor in mpkCCD cells but decreased the expression of the EP4 receptor. Additionally, our experiments showed that the activation of EP1 is the pathway by which PGE2 inhibits the dDAVP-induced AQP2 expression in mpkCCD cells (Figure 6). The EP1 receptors can couple to Gq and increase cytosolic Ca2+ and activate protein kinase C (PKC; Funk et al., 1993; Watabe et al., 1993). In microperfused collecting ducts, the inhibitory effect of PGE2 on AVP-stimulated water permeability was dependent on the activity of PKC (Hebert et al., 1990; Nadler et al., 1992). PKC activation also promotes AQP2 endocytosis, similar to PGE2 (Zelenina et al., 2000; Van Balkom et al., 2002; Nejsum et al., 2005), and increases AQP2 ubiquitination, leading to lysosomal degradation (Kamsteeg et al., 2006). This suggests that the EP1 activation will decrease the AQP2 abundance by lysosomal degradation (Figure 6).
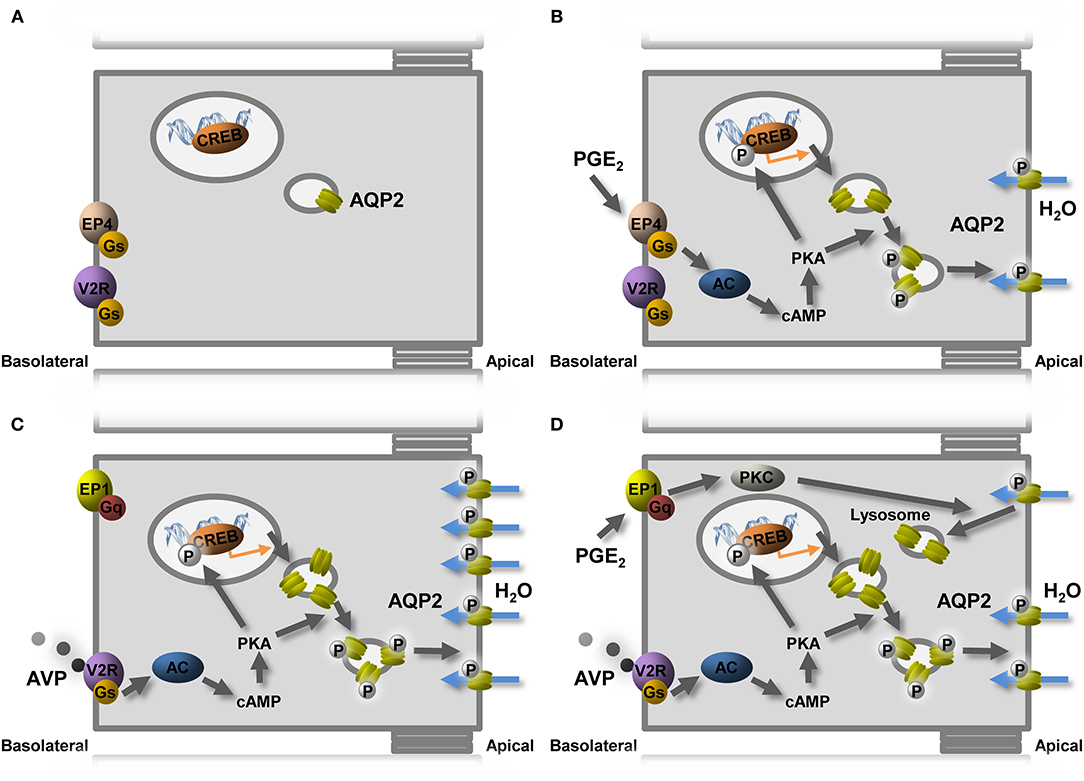
Figure 6. Model of PGE2-mediated regulation of AQP2-mediated water reabsorption. (A) In the absence of AVP, the AQP2 expression is low and present in intracellular vesicles. (B) PGE2 stimulates water reabsorption by binding to the EP4 receptor, coupling to the Gs protein, leading to cAMP generation, followed by AQP2 transcription and translocation. (C) AVP increases the expression of AQP2 but also induces the expression of the AVP-counteracting EP1 receptor and reduces EP4. (D) In the presence of AVP, PGE2 decreases the AQP2 expression by stimulating EP1. Indicated are AC, adenylate cyclase; AQP2, aquaporin-2; AVP, vasopressin; cAMP, cyclic adenosine monophosphate; PKA, protein kinase A; PKC, protein kinase C; V2R, vasopressin V2 receptor.
The expression of the FP receptor was increased by dDAVP incubation in mpkCCD cells. As the activation of the FP receptor inhibits water reabsorption, the increase in the FP expression might be a compensatory mechanism to counteract AVP stimulation, similar to the increase in the EP1 expression.
As no DP receptor was detected in mpkCCDc14 cells, the role of the dDAVP-stimulated increase in the PGD2 production after dDAVP incubation is unclear. However, PGD2 has been shown to bind to the FP receptor with an affinity close to that for the DP receptor, indicating that PGD2 may act on the FP receptor (Kiriyama et al., 1997). The increase in PGD2 might counteract the dDAVP-induced increase in the AQP2 expression, although levels are low compared with the PGE2 and PGF2α production.
While the TP receptor is expressed in mpkCCDc14 cells, the expression of the IP receptor is inconclusive. Both thromboxane and PGI2 were produced in very low amounts in mpkCCDc14 cells, and the production was not affected by dDAVP. Whether these prostanoids have any role in water reabsorption remains unclear.
Relation of the MpkCCD Cell System to the in vivo Situation
A limitation of our study is that all experiments are performed in mpkCCD cells. However, a problem with in vivo studies investigating the effect of prostaglandins on the collecting duct is that these studies are complicated by the effect of prostaglandins on AVP release and on medullary osmolality, both of which will influence the AQP2 expression (Yamamoto et al., 1976; Stoff et al., 1981; Hasler et al., 2005). To study the effect of prostaglandins directly on principal cells, experiments were performed in mpkCCD cells, shown to display the essential functionalities characteristic of principal cells like the AVP-regulated AQP2 expression and aldosterone-mediated sodium transport via the epithelial sodium channel (Bens et al., 1999; Hasler et al., 2002).
The major prostaglandins produced in our cell system were PGE2 and PGF2α, which is in agreement with in vivo findings, showing that PGE2 is the most abundant prostanoid in both the renal cortex and medulla, followed by PGI2 and PGF2α (Qi et al., 2006). The synthases involved in the production of PGD2, PGE2, and PGF2α are detected in the nephron (Vitzthum et al., 2002; Sakurai et al., 2005), where the production of PGE2 and PGF2α has been shown to occur mainly in the collecting ducts (Farman et al., 1987). Neither PGI synthase nor thromboxane synthase mRNA is detected in any tubular structure (Vitzthum et al., 2002).
The effects of prostaglandins on the AQP2 expression are conferred by PG receptors. In mpkCCDc14 cells, EP1, EP4, and FP receptors are found, in agreement with expression in the collecting duct (Breyer et al., 1998; Saito et al., 2003).
In line with our data showing the role of EP4 in the stimulatory effect of PGE2 on AQP2, a study by Gao et al. demonstrates that disruption of EP4 in the collecting duct impaired the urinary concentration by decreasing the AQP2 abundance and apical membrane targeting, providing evidence that EP4 can regulate the urine concentration in vivo (Gao et al., 2015). In addition, a selective EP4 agonist has been shown to increase the urine osmolality, decrease the urine volume, and increase the AQP2 expression in a mouse model for congenital NDI (Li et al., 2009).
In agreement with our findings that the activation of the EP1 receptor decreases the AVP-induced AQP2 expression, the stimulation of the EP1 receptor has been shown to decrease the vasotocin-induced osmotic water permeability of the frog urinary bladder, a model system of the collecting duct (Bachteeva et al., 2007). In addition, EP1-knockout mice have a urinary concentrating defect (Kennedy et al., 2007), and recent studies show that PGE2 does not decrease AVP-mediated water transport in isolated collecting ducts of these mice (Nasrallah et al., 2018). Taken together with the present data, this suggests that EP1 conveys both acute and long-term modulation of the V2R activity.
Furthermore, TP and IP receptors are mainly localized in the glomerulus and vasculature, respectively, but have also been located in the collecting duct (Takahashi et al., 1996; Komhoff et al., 1998), in agreement with the expression seen in mpkCCDc14 cells. Based on our mpkCCD data, however, we anticipated that the IP receptor does not have a major impact on the principal cell AQP2 expression in the presence or absence of AVP.
None of the receptors DP, EP2, and EP3 seems to be expressed in mpkCCDc14 cells. While DP is also not expressed in the kidney, the presence of EP2 along the nephron is a matter of considerable debate (Breyer and Breyer, 2000; Olesen and Fenton, 2013). However, a previous study has shown that functionally, the collecting duct can respond to the stimulation of the EP2 receptor (Olesen et al., 2011).
The inhibitory effects of PGE2 on AVP-induced water reabsorption have, besides via the activation of EP1, also been suggested to occur through the activation of EP3 (Hebert et al., 1993; Fleming et al., 1998). Our cell model does not express the EP3 receptor, which was found in vivo by the in situ hybridization and RT-PCR on microdissected tubules to be expressed in the collecting duct (Breyer et al., 1998). However, a study using single-cell RNA-Seq of intercalated and principal cells from the mouse kidney demonstrated that EP3 was selectively expressed in collecting duct-intercalated cells, while EP1 and EP4 were expressed in the principal cells (Chen et al., 2017). In addition, EP3-knockout mice exhibit a similar urine-concentrating ability during basal conditions as well as in response to AVP compared with wild-type mice, arguing against a role of EP3 in the AQP2 regulation (Fleming et al., 1998). The exact role of the EP3 receptor in the AQP2 regulation needs further investigation.
Central Mechanism for the Differential Effect of PGE2 on AQP2 Expression
It is interesting to note that, while dDAVP increases the PGE2 production and release, the mRNA expression of the EP4 receptor is reduced, whereas that of the EP1 receptor is increased. As both receptors are bound and activated by PGE2, these data suggest that it is not the agonist per se that determines the expression level of the receptors. Instead, our data indicate that the signaling cascade that is mainly activated exerts a negative feedback regulation on receptors stimulating the same pathway and a positive feedback on receptors activating an opposite pathway: dDAVP increases the cAMP-AQP2 pathway, which can be stimulated by EP4, whereas EPl activates a pathway that leads to a decreased AQP2 expression and water permeability.
The same antagonizing mechanism can be seen in response to endothelin, which counteracts the AVP-mediated water permeability (Edwards et al., 1993), and at the same time, leads to an increased expression of the vasopressin V2 receptor in the inner medullary collecting duct of the rat (Sonntag et al., 2004). Similar to this antagonizing mechanism, dDAVP increases the mRNA levels of the purinergic receptor subunit P2Y2 in mpkCCD cells and targets the subunits P2Y2 and P2X2 to the plasma membrane, where the activation of these receptors leads to the AQP2 internalization and a decrease in the water permeability (Wildman et al., 2009). A similar mechanism can be seen with the hormone angiotensin II, which increases renal proximal sodium reabsorption but at the same time increases expression of the D4 dopamine receptor in renal proximal tubule cells, which activation will decrease sodium reabsorption, thereby counteracting the direct effect of angiotensin II (Tang et al., 2017).
In conclusion, our study shows that in mpkCCDc14 cells, both PGE2 and PGF2α decrease the dDAVP-stimulated AQP2 abundance, while in the absence of dDAVP, PGE2 increases AQP2 levels. Furthermore, our study suggests that EP4 mediates the PGE2-induced increase in the AQP2 abundance in the absence of dDAVP, while the PGE2-mediated decrease in the AQP2 abundance in the presence of dDAVP is likely mediated via EP1. This paradoxical difference in response to PGE2 is likely explained by the different receptor subtype expression induced by the dDAVP treatment, leading to an increase in EP1 and a decrease in EP4.
Based on our data above that a negative feedback is mediated by the signaling pathways activated instead of the agonist, we hypothesized that in vivo AVP increases, besides AQP2, the expression of EP1 and decreases the expression of EP4 receptors. Consequently, in conditions with the increased PGE2 release, such as with lithium-NDI or bilateral uteral obstruction, the AVP-induced AQP2 expression would be reduced via the activation of these EP1 receptors.
Data Availability Statement
The raw data supporting the conclusions of this article will be made available by the authors, without undue reservation.
Author Contributions
MK, JW, RF, and PD designed experiments. MK, MB, HS, EO, and CC performed experiments. MK and PD wrote manuscript. All authors approved the final manuscript.
Funding
PD is a recipient of VICI grant 865.07.0h02 of the Netherlands Organization for Scientific research (NWO). This work was supported by grants from NWO (VICI grant 865.07.002) and RUNMC (2004.55) to PD and grants from the Independent Research Fund Denmark (Project No. 1333-00279 and 1331-00738B) and the Aarhus University Research Foundation to MK. RF was funded by the Independent Research Fund Denmark (Project No. 1026-00063B) and the Novo Nordisk Foundation.
Conflict of Interest
The authors declare that the research was conducted in the absence of any commercial or financial relationships that could be construed as a potential conflict of interest.
Publisher's Note
All claims expressed in this article are solely those of the authors and do not necessarily represent those of their affiliated organizations, or those of the publisher, the editors and the reviewers. Any product that may be evaluated in this article, or claim that may be made by its manufacturer, is not guaranteed or endorsed by the publisher.
Acknowledgments
We thank Johan van Burgsteden and Michiel van den Brand, Nijmegen, and Christian Westberg, Aarhus, for their excellent technical assistance.
References
Bachteeva, V., Fock, E., Lavrova, E., Nikolaeva, S., Gambaryan, S., and Parnova, R. (2007). Prostaglandin E2 inhibits vasotocin-induced osmotic water permeability in the frog urinary bladder by EP1-receptor-mediated activation of NO/cGMP pathway. Am. J. Physiol Regul. Integr. Comp. Physiol. 293, R528–R537. doi: 10.1152/ajpregu.00811.2006
Bens, M., Vallet, V., Cluzeaud, F., Pascual-Letallec, L., Kahn, A., Rafestin-Oblin, M. E., et al. (1999). Corticosteroid-dependent sodium transport in a novel immortalized mouse collecting duct principal cell line. J. Am. Soc. Nephrol. 10, 923–934. doi: 10.1681/ASN.V105923
Billot, X., Chateauneuf, A., Chauret, N., Denis, D., Greig, G., Mathieu, M. C., et al. (2003). Discovery of a potent and selective agonist of the prostaglandin EP4 receptor. Bioorg. Med. Chem. Lett 13, 1129–1132. doi: 10.1016/S0960-894X(03)00042-8
Bonvalet, J. P., Pradelles, P., and Farman, N. (1987). Segmental synthesis and actions of prostaglandins along the nephron. Am. J. Physiol. 253, F377–387. doi: 10.1152/ajprenal.1987.253.3.F377
Boone, M., and Deen, P. M. (2008). Physiology and pathophysiology of the vasopressin-regulated renal water reabsorption. Pflugers Arch. 456, 1005–1024. doi: 10.1007/s00424-008-0498-1
Breyer, M. D., and Breyer, R. M. (2000). Prostaglandin E receptors and the kidney. Am. J. Physiol. Renal. Physiol. 279, F12–F23. doi: 10.1152/ajprenal.2000.279.1.F12
Breyer, M. D., Zhang, Y., Guan, Y. F., Hao, C. M., Hebert, R. L., and Breyer, R. M. (1998). Regulation of renal function by prostaglandin E receptors. Kidney Int. Suppl. 67, S88–S94. doi: 10.1046/j.1523-1755.1998.06718.x
Chen, L., Lee, J. W., Chou, C. L., Nair, A. V., Battistone, M. A., Paunescu, T. G., et al. (2017). Transcriptomes of major renal collecting duct cell types in mouse identified by single-cell RNA-seq. Proc. Natl. Acad. Sci. U. S. A. 114, E9989–E9998. doi: 10.1073/pnas.1710964114
Deen, P. M. T., Verdijk, M. a. J., Knoers, N. V. a. M., Wieringa, B., Monnens, L. a. H., Van Os, C. H., et al. (1994). Requirement of human renal water channel aquaporin-2 for vasopressin-dependent concentration of urine. Science 264, 92–95. doi: 10.1126/science.8140421
Edwards, R. M., Stack, E. J., Pullen, M., and Nambi, P. (1993). Endothelin inhibits vasopressin action in rat inner medullary collecting duct via the ETB receptor. J. Pharmacol. Exp. Ther. 267, 1028–1033.
Farman, N., Pradelles, P., and Bonvalet, J. P. (1987). PGE2, PGF2 alpha, 6-keto-PGF1 alpha, and TxB2 synthesis along the rabbit nephron. Am. J. Physiol. 252, F53–F59. doi: 10.1152/ajprenal.1987.252.1.F53
Fleming, E. F., Athirakul, K., Oliverio, M. I., Key, M., Goulet, J., Koller, B. H., et al. (1998). Urinary concentrating function in mice lacking EP3 receptors for prostaglandin E2. Am. J. Physiol 275, F955–F961. doi: 10.1152/ajprenal.1998.275.6.F955
Funk, C. D., Furci, L., Fitzgerald, G. A., Grygorczyk, R., Rochette, C., Bayne, M. A., et al. (1993). Cloning and expression of a cDNA for the human prostaglandin E receptor EP1 subtype. J. Biol. Chem. 268, 26767–26772. doi: 10.1016/S0021-9258(19)74379-8
Gao, M., Cao, R., Du, S., Jia, X., Zheng, S., Huang, S., et al. (2015). Disruption of prostaglandin E2 receptor EP4 impairs urinary concentration via decreasing aquaporin 2 in renal collecting ducts. Proc. Natl. Acad. Sci. U. S. A. 112, 8397–8402. doi: 10.1073/pnas.1509565112
Hao, C. M., and Breyer, M. D. (2008). Physiological regulation of prostaglandins in the kidney. Annu. Rev. Physiol. 70, 357–377. doi: 10.1146/annurev.physiol.70.113006.100614
Hasler, U., Mordasini, D., Bens, M., Bianchi, M., Cluzeaud, F., Rousselot, M., et al. (2002). Long-term regulation of aquaporin-2 expression in vasopressin-responsive renal collecting duct principal cells. J. Biol. Chem. 277, 10379–10386. doi: 10.1074/jbc.M111880200
Hasler, U., Vinciguerra, M., Vandewalle, A., Martin, P. Y., and Feraille, E. (2005). Dual effects of hypertonicity on aquaporin-2 expression in cultured renal collecting duct principal cells. J. Am. Soc. Nephrol. 16, 1571–1582. doi: 10.1681/ASN.2004110930
Hebert, R. L., Carmosino, M., Saito, O., Yang, G., Jackson, C. A., Qi, Z., et al. (2005). Characterization of a rabbit kidney prostaglandin F(2{alpha}) receptor exhibiting G(i)-restricted signaling that inhibits water absorption in the collecting duct. J. Biol. Chem. 280, 35028–35037. doi: 10.1074/jbc.M505852200
Hebert, R. L., Jacobson, H. R., and Breyer, M. D. (1990). PGE2 inhibits AVP-induced water flow in cortical collecting ducts by protein kinase C activation. Am. J. Physiol. 259, F318–F325. doi: 10.1152/ajprenal.1990.259.2.F318
Hebert, R. L., Jacobson, H. R., Fredin, D., and Breyer, M. D. (1993). Evidence that separate PGE2 receptors modulate water and sodium transport in rabbit cortical collecting duct. Am. J. Physiol. 265, F643–F650. doi: 10.1152/ajprenal.1993.265.5.F643
Kamsteeg, E. J., Hendriks, G., Boone, M., Konings, I. B., Oorschot, V., Van Der, S. P., et al. (2006). Short-chain ubiquitination mediates the regulated endocytosis of the aquaporin-2 water channel. Proc. Natl. Acad. Sci. U. S. A. 103, 18344–18349. doi: 10.1073/pnas.0604073103
Kamsteeg, E. J., Wormhoudt, T. A., Rijss, J. P. L., Van Os, C. H., and Deen, P. M. T. (1999). An impaired routing of wild-type aquaporin-2 after tetramerization with an aquaporin-2 mutant explains dominant nephrogenic diabetes insipidus. EMBO J. 18, 2394–2400. doi: 10.1093/emboj/18.9.2394
Kennedy, C. R., Xiong, H., Rahal, S., Vanderluit, J., Slack, R. S., Zhang, Y., et al. (2007). Urine concentrating defect in prostaglandin EP1-deficient mice. Am. J. Physiol. Renal Physiol. 292, F868–F875. doi: 10.1152/ajprenal.00183.2005
Kim, G. H., Choi, N. W., Jung, J. Y., Song, J. H., Lee, C. H., Kang, C. M., et al. (2008). Treating lithium-induced nephrogenic diabetes insipidus with a COX-2 inhibitor improves polyuria via upregulation of AQP2 and NKCC2. Am. J. Physiol. Renal Physiol. 294, F702–F709. doi: 10.1152/ajprenal.00366.2007
Kiriyama, M., Ushikubi, F., Kobayashi, T., Hirata, M., Sugimoto, Y., and Narumiya, S. (1997). Ligand binding specificities of the eight types and subtypes of the mouse prostanoid receptors expressed in Chinese hamster ovary cells. Br. J. Pharmacol. 122, 217–224. doi: 10.1038/sj.bjp.0701367
Knepper, M. A. (1997). Molecular physiology of urinary concentrating mechanism: regulation of aquaporin water channels by vasopressin. Am. J. Physiol. 41, F3–F12. doi: 10.1152/ajprenal.1997.272.1.F3
Komhoff, M., Lesener, B., Nakao, K., Seyberth, H. W., and Nusing, R. M. (1998). Localization of the prostacyclin receptor in human kidney. Kidney Int. 54, 1899–1908. doi: 10.1046/j.1523-1755.1998.00213.x
Li, J. H., Chou, C. L., Li, B., Gavrilova, O., Eisner, C., Schnermann, J., et al. (2009). A selective EP4 PGE2 receptor agonist alleviates disease in a new mouse model of X-linked nephrogenic diabetes insipidus. J. Clin. Invest. 119, 3115–3126. doi: 10.1172/JCI39680
Li, Y., Shaw, S., Kamsteeg, E. J., Vandewalle, A., and Deen, P. M. (2006). Development of lithium-induced nephrogenic diabetes insipidus is dissociated from adenylyl cyclase activity. J. Am. Soc. Nephrol. 17, 1063–1072. doi: 10.1681/ASN.2005080884
Matsumura, Y., Uchida, S., Rai, T., Sasaki, S., and Marumo, F. (1997). Transcriptional regulation of aquaporin-2 water channel gene by cAMP. J. Am. Soc. Nephrol. 8, 861–867. doi: 10.1681/ASN.V86861
Nadler, S. P., Zimpelmann, J. A., and Hebert, R. L. (1992). PGE2 inhibits water permeability at a post-cAMP site in rat terminal inner medullary collecting duct. Am. J. Physiol. 262, F229–F235. doi: 10.1152/ajprenal.1992.262.2.F229
Nasrallah, R., Zimpelmann, J., Eckert, D., Ghossein, J., Geddes, S., Beique, J. C., et al. (2018). PGE2 EP1 receptor inhibits vasopressin-dependent water reabsorption and sodium transport in mouse collecting duct. Lab. Invest. 98, 360–370. doi: 10.1038/labinvest.2017.133
Nejsum, L. N., Zelenina, M., Aperia, A., Frokiaer, J., and Nielsen, S. (2005). Bidirectional regulation of AQP2 trafficking and recycling: involvement of AQP2-S256 phosphorylation. Am. J. Physiol. Renal. Physiol. 288, F930–F938. doi: 10.1152/ajprenal.00291.2004
Norregaard, R., Jensen, B. L., Li, C., Wang, W., Knepper, M. A., Nielsen, S., et al. (2005). COX-2 inhibition prevents downregulation of key renal water and sodium transport proteins in response to bilateral ureteral obstruction. Am. J. Physiol. Renal. Physiol. 289, F322–F333. doi: 10.1152/ajprenal.00061.2005
Norregaard, R., Jensen, B. L., Topcu, S. O., Wang, G., Schweer, H., Nielsen, S., et al. (2010). Urinary tract obstruction induces transient accumulation of COX-2-derived prostanoids in kidney tissue. Am. J. Physiol Regul. Integr. Comp Physiol 298, R1017–R1025. doi: 10.1152/ajpregu.00336.2009
Olesen, E. T., and Fenton, R. A. (2013). Is there a role for PGE2 in urinary concentration? J. Am. Soc. Nephrol. 24, 169–178. doi: 10.1681/ASN.2012020217
Olesen, E. T., Moeller, H. B., Assentoft, M., Macaulay, N., and Fenton, R. A. (2016). The vasopressin type 2 receptor and prostaglandin receptors EP2 and EP4 can increase aquaporin-2 plasma membrane targeting through a cAMP-independent pathway. Am. J. Physiol. Renal. Physiol. 311, F935–F944. doi: 10.1152/ajprenal.00559.2015
Olesen, E. T., Rutzler, M. R., Moeller, H. B., Praetorius, H. A., and Fenton, R. A. (2011). Vasopressin-independent targeting of aquaporin-2 by selective E-prostanoid receptor agonists alleviates nephrogenic diabetes insipidus. Proc. Natl. Acad. Sci. U. S. A. 108, 12949–12954. doi: 10.1073/pnas.1104691108
Qi, Z., Cai, H., Morrow, J. D., and Breyer, M. D. (2006). Differentiation of cyclooxygenase 1- and 2-derived prostanoids in mouse kidney and aorta. Hypertension 48, 323–328. doi: 10.1161/01.HYP.0000231934.67549.b7
Rao, R., Zhang, M. Z., Zhao, M., Cai, H., Harris, R. C., Breyer, M. D., et al. (2005). Lithium treatment inhibits renal GSK-3 activity and promotes cyclooxygenase 2-dependent polyuria. Am. J. Physiol. Renal. Physiol. 288, F642–F649. doi: 10.1152/ajprenal.00287.2004
Saito, O., Guan, Y., Qi, Z., Davis, L. S., Komhoff, M., Sugimoto, Y., et al. (2003). Expression of the prostaglandin F receptor (FP) gene along the mouse genitourinary tract. Am. J. Physiol Renal Physiol 284, F1164–F1170. doi: 10.1152/ajprenal.00441.2002
Sakairi, Y., Jacobson, H. R., Noland, T. D., and Breyer, M. D. (1995). Luminal prostaglandin E receptors regulate salt and water transport in rabbit cortical collecting duct. Am. J. Physiol. 269, F257–F265. doi: 10.1152/ajprenal.1995.269.2.F257
Sakurai, M., Oishi, K., and Watanabe, K. (2005). Localization of cyclooxygenases-1 and−2, and prostaglandin F synthase in human kidney and renal cell carcinoma. Biochem. Biophys. Res. Commun. 338, 82–86. doi: 10.1016/j.bbrc.2005.08.194
Schlondorff, D., Satriano, J. A., and Schwartz, G. J. (1985). Synthesis of prostaglandin E2 in different segments of isolated collecting tubules from adult and neonatal rabbits. Am. J. Physiol. 248, F134–F144. doi: 10.1152/ajprenal.1985.248.1.F134
Schweer, H., Watzer, B., and Seyberth, H. W. (1994). Determination of seven prostanoids in 1 ml of urine by gas chromatography-negative ion chemical ionization triple stage quadrupole mass spectrometry. J. Chromatogr. 652, 221–227. doi: 10.1016/0378-4347(93)E0408-I
Sonntag, M., Wang, M. H., Huang, M. H., and Wong, N. L. (2004). Endothelin upregulates the expression of vasopressin V2 mRNA in the inner medullary collecting duct of the rat. Metabolism 53, 1177–1183. doi: 10.1016/j.metabol.2004.02.022
Stoff, J. S., Rosa, R. M., Silva, P., and Epstein, F. H. (1981). Indomethacin impairs water diuresis in the DI rat: role of prostaglandins independent of ADH. Am. J. Physiol. 241, F231–F237. doi: 10.1152/ajprenal.1981.241.3.F231
Takahashi, N., Takeuchi, K., Abe, T., Sugawara, A., and Abe, K. (1996). Immunolocalization of rat thromboxane receptor in the kidney. Endocrinology 137, 5170–5173. doi: 10.1210/endo.137.11.8895394
Tang, L., Zheng, S., Ren, H., He, D., Zeng, C., and Wang, W. E. (2017). Activation of angiotensin II type 1 receptors increases D4 dopamine receptor expression in rat renal proximal tubule cells. Hypertens. Res. 40, 652–657. doi: 10.1038/hr.2017.13
Terris, J., Ecelbarger, C. A., Nielsen, S., and Knepper, M. A. (1996). Long-term regulation of four renal aquaporins in rats. Am. J. Physiol. 40, F414–F422. doi: 10.1152/ajprenal.1996.271.2.F414
Van Balkom, B. W. M., Savelkoul, P. J., Markovich, D., Hofman, E., Nielsen, S., Van Der Sluijs, P., et al. (2002). The role of putative phosphorylation sites in the targeting and shuttling of the aquaporin-2 water channel. J. Biol. Chem. 277, 41473–41479. doi: 10.1074/jbc.M207525200
Vitzthum, H., Abt, I., Einhellig, S., and Kurtz, A. (2002). Gene expression of prostanoid forming enzymes along the rat nephron. Kidney Int. 62, 1570–1581. doi: 10.1046/j.1523-1755.2002.00615.x
Ware, J. S., Wain, L. V., Channavajjhala, S. K., Jackson, V. E., Edwards, E., Lu, R., et al. (2017). Phenotypic and pharmacogenetic evaluation of patients with thiazide-induced hyponatremia. J. Clin. Invest. 127, 3367–3374. doi: 10.1172/JCI89812
Watabe, A., Sugimoto, Y., Honda, A., Irie, A., Namba, T., Negishi, M., et al. (1993). Cloning and expression of cDNA for a mouse EP1 subtype of prostaglandin E receptor. J. Biol. Chem. 268, 20175–20178. doi: 10.1016/S0021-9258(20)80710-8
Wildman, S. S., Boone, M., Peppiatt-Wildman, C. M., Contreras-Sanz, A., King, B. F., Shirley, D. G., et al. (2009). Nucleotides downregulate aquaporin 2 via activation of apical P2 receptors. J. Am. Soc. Nephrol. 20, 1480–1490. doi: 10.1681/ASN.2008070686
Yamamoto, M., Share, L., and Shade, R. E. (1976). Vasopressin release during ventriculo-cisternal perfusion with prostaglandin E2 in the dog. J. Endocrinol. 71, 325–331. doi: 10.1677/joe.0.0710325
Yasui, M., Zelenin, S. M., Celsi, G., and Aperia, A. (1997). Adenylate cyclase-coupled vasopressin receptor activates AQP2 promoter via a dual effect on CRE and AP1 elements. Am. J. Physiol. 41, F443–F450. doi: 10.1152/ajprenal.1997.272.4.F443
Zelenina, M., Christensen, B. M., Palmer, J., Nairn, A. C., Nielsen, S., and Aperia, A. (2000). Prostaglandin E(2) interaction with AVP: effects on AQP2 phosphorylation and distribution. Am. J. Physiol. Renal. Physiol. 278, F388–F394. doi: 10.1152/ajprenal.2000.278.3.F388
Keywords: water transport, AQP2, vasopressin, prostaglandin, mpkCCD, PGE2, EP1, EP4
Citation: Deen PMT, Boone M, Schweer H, Olesen ETB, Carmone C, Wetzels JFM, Fenton RA and Kortenoeven MLA (2022) A Vasopressin-Induced Change in Prostaglandin Receptor Subtype Expression Explains the Differential Effect of PGE2 on AQP2 Expression. Front. Physiol. 12:787598. doi: 10.3389/fphys.2021.787598
Received: 30 September 2021; Accepted: 07 December 2021;
Published: 21 January 2022.
Edited by:
Hyun Jun Jung, Johns Hopkins Medicine, United StatesReviewed by:
Tae-Hwan Kwon, Kyungpook National University, South KoreaVladimir T. Todorov, Technical University Dresden, Germany
Copyright © 2022 Deen, Boone, Schweer, Olesen, Carmone, Wetzels, Fenton and Kortenoeven. This is an open-access article distributed under the terms of the Creative Commons Attribution License (CC BY). The use, distribution or reproduction in other forums is permitted, provided the original author(s) and the copyright owner(s) are credited and that the original publication in this journal is cited, in accordance with accepted academic practice. No use, distribution or reproduction is permitted which does not comply with these terms.
*Correspondence: Marleen L. A. Kortenoeven, a29ydGVub2V2ZW5AaGVhbHRoLnNkdS5kaw==