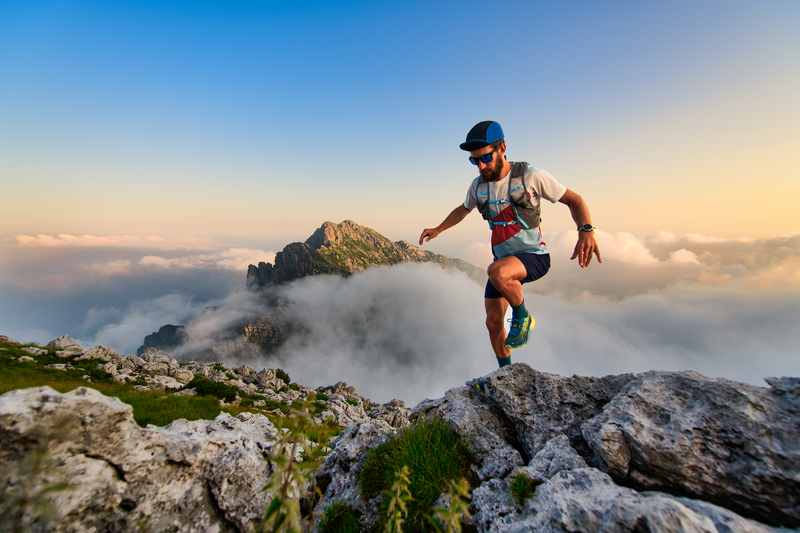
95% of researchers rate our articles as excellent or good
Learn more about the work of our research integrity team to safeguard the quality of each article we publish.
Find out more
REVIEW article
Front. Physiol. , 21 December 2021
Sec. Respiratory Physiology and Pathophysiology
Volume 12 - 2021 | https://doi.org/10.3389/fphys.2021.785014
This article is part of the Research Topic Protecting the Acutely Injured Lung: Physiologic, Mechanical, Inflammatory, and Translational Perspectives View all 17 articles
Mechanical ventilation (MV) is a lifesaving supportive intervention in the management of acute respiratory distress syndrome (ARDS), buying time while the primary precipitating cause is being corrected. However, MV can contribute to a worsening of the primary lung injury, known as ventilation-induced lung injury (VILI), which could have an important impact on outcome. The ARDS lung is characterized by diffuse and heterogeneous lung damage and is particularly prone to suffer the consequences of an excessive mechanical stress imposed by higher airway pressures and volumes during MV. Of major concern is cyclic overdistension, affecting those lung segments receiving a proportionally higher tidal volume in an overall reduced lung volume. Theoretically, healthier lung regions are submitted to a larger stress and cyclic deformation and thus at high risk for developing VILI. Clinicians have difficulties in detecting VILI, particularly cyclic overdistension at the bedside, since routine monitoring of gas exchange and lung mechanics are relatively insensitive to this mechanism of VILI. Expired CO2 kinetics integrates relevant pathophysiological information of high interest for monitoring. CO2 is produced by cell metabolism in large daily quantities. After diffusing to tissue capillaries, CO2 is transported first by the venous and then by pulmonary circulation to the lung. Thereafter diffusing from capillaries to lung alveoli, it is finally convectively transported by lung ventilation for its elimination to the atmosphere. Modern readily clinically available sensor technology integrates information related to pulmonary ventilation, perfusion, and gas exchange from the single analysis of expired CO2 kinetics measured at the airway opening. Current volumetric capnography (VCap), the representation of the volume of expired CO2 in one single breath, informs about pulmonary perfusion, end-expiratory lung volume, dead space, and pulmonary ventilation inhomogeneities, all intimately related to cyclic overdistension during MV. Additionally, the recently described capnodynamic method provides the possibility to continuously measure the end-expiratory lung volume and effective pulmonary blood flow. All this information is accessed non-invasively and breath-by-breath helping clinicians to personalize ventilatory settings at the bedside and minimize overdistension and cyclic deformation of lung tissue.
The acute respiratory distress syndrome (ARDS) is the most severe form of acute respiratory failure that affects the lungs in a heterogeneous way, profoundly impairing their mechanical properties and gas-exchange functions. Diffuse lung pan-endothelial inflammation, the hallmark of the syndrome, leads to the invasion of alveolar spaces by edema and inflammation reducing effective pulmonary lung volume (i.e., functional residual capacity, FRC). The lung becomes heavier exerting a superimposed pressure on the dependent parts of the lung, critically decreasing regional transpulmonary pressure. This further reduces FRC by promoting lung collapse, a common pathophysiological feature of ARDS (Gattinoni et al., 2006).
Mechanical ventilation (MV) is the principal life-support intervention in the management of patients with ARDS but at the risk to perpetuate or aggravate lung damage. Ventilation-induced lung injury (VILI) results from the need to use high transpulmonary pressures and frequently higher tidal volumes (VT) to oxygenate and ventilate the heterogeneously ARDS lung. The delivered VT is distributed in a much smaller lung volume with important regional differences, with three important consequences: (1) less diseased lung regions will receive a larger fraction of the VT causing an increased cyclic tissue deformation or strain, a mechanism that triggers lung inflammation, (2) more diseased but ventilated regions will be submitted to a higher transpulmonary pressure for any given delivered VT increasing the local mechanical stress also known as cyclic overdistension, and (3) collapsed regions eventually re-open and close with each tidal inflation and deflation causing cyclic recruitment–de-recruitment, which can be considered an extreme form of regional strain. These are three of the most important mechanisms by which MV damages lung parenchyma. Lung imaging techniques, such as CT or intravital microscopy, have confirmed the heterogeneity of lung injury in ARDS at different scales, revealing the coexistence of normal, collapsed, and overdistended alveoli in different lungs regions. The resulting non-uniform distribution of tidal ventilation can be visualized in real time at the bedside by electrical impedance tomography (EIT), whereas more sophisticated techniques, such as PET and single-photon emission tomography (SPECT) imaging, have located the main inflammatory response in normally ventilated areas but not in collapsed ones (Bellani et al., 2009, 2011). Thus, lung collapse acts as a stress-raiser since it contributes to lung heterogeneity creating areas receiving an excessive VT in relation to their regional volume.
The introduction of lung-protective ventilation strategies aimed at reducing the mechanical stress imposed by the ventilator has contributed to reducing morbidity and mortality of patients with ARDS (ARDS Network, 2000). Ideally, these strategies should be individualized, but this requires useful and directed bedside clinical monitoring. However, routine monitoring only includes basic lung mechanics, gas exchange and intermittently, lung imaging techniques and thus, the clinical detection of overdistension or lung strain, and VILI remains difficult. The analysis of expired gases, in particular CO2, is a well-established, robust, and clinically accessible monitoring option. Volumetric capnography (VCap), representing the volume of CO2 expired in one breath, has specific features regarding the analysis of body CO2 kinetics that can be of great value in detecting lung overdistension by providing continuous non-invasive information on lung perfusion, convective gas transport, lung diffusion, and dead space ventilation (VD), all intimately related and sensitive to the effects of lung overdistension. However, this source of highly relevant biological information is still largely underused in clinical practice. A progressive better understanding of the complex behavior and physiology of CO2 kinetics have led to recent new developments for advanced analysis of the volumetric capnogram and a derived capnodynamic method that hold great promise in overcoming the difficulties in adopting the expired CO2 monitoring in routine clinical practice.
The aim of this manuscript is to review the principles, uses, and physiological basis of expired CO2 kinetics monitoring. We will review new developments and value of VD monitoring using VCap in patients with ARDS and describe the new capnodynamic method that continuously monitors effective lung volume and perfusion in a non-invasive way.
The integration of an infrared CO2 sensor and a flow sensor in a mainstream configuration allows for the reconstruction of the volumetric capnogram at the airway opening (Figure 1). As the cardiorespiratory system has an open arrangement, VCap contains implicit information regarding these systems expressed not only in their derived variables and indexes but also in its shape that helps the interpretation of normality and diseases throughout its derived-parameters. As mentioned above, CO2 kinetics is context-sensitive, which means that VCap parameters must be interpreted when changes in metabolism, pulmonary perfusion, or ventilation occur one at a time (Sipmann et al., 2014). For example, an increase in VT improves CO2 elimination explained solely by a ventilatory change when metabolism and hemodynamics are constant.
Figure 1. Components of volumetric capnography. The volumetric capnogram is the plot of expired CO2 in one tidal breath measured by mainstream flow and CO2 sensors. The capnogram phases are shown in green: phase I is the portion of the expired gas without CO2 that represents pure airway dead space; phase II is the transition zone composed by the progressive emptying of lung units with different time-constants and spatial distribution; and phase III is the pure alveolar gas expired once airway dead space (VDaw) has been washed out. The relevant partial pressures of CO2 measured by VCap are presented in red: end-tidal (PETCO2), mean alveolar (PACO2), mixed expired (PCO2), and inspired (PICO2) pressure. The arterial PaCO2 is not directly measured, but it included for referencing of the other partial pressures. The volumes obtained by the VCap are presented in blue: volume of airway dead space (VDaw), alveolar tidal volume (VTalv), and volume of CO2 eliminated per breath or area under the VCap curve (VTCO2,br). The blue dot represents the airway–alveolar interface, the limit between convective and diffusive CO2 transport close to the entrance of lung acini and, therefore, it also morphologically separates the conducting from the gas-exchanging lung compartment.
Interpretation of CO2 kinetics can be done during steady and non-steady state conditions, although the non-steady state is of particular interest in clinical monitoring as it allows to interpret and react to changes in pulmonary perfusion, end-expiratory lung volume, and/or alveolar ventilation after adjusting the ventilatory settings or to changing clinical conditions.
Dead space ventilation is the wasted portion of ventilation not involved in gas exchange per se. It constitutes the evolutionary adaptive price paid by all mammals that depend on the bulk convective transport of ambient air to the gas–liquid gas-exchange interface, the alveolar–capillary membrane. By the end of the 19th century, Christian Bohr presented a method to estimate VD volume based on the mass balance of any gas breathed during the respiratory cycle (Figure 2) (Bohr, 1891). He brilliantly adapted his formula using CO2 as the tracer gas, which still constitutes the basis of the clinical measurement of dead space. Aitken and Clark-Kennedy (1928) were the first to propose to represent CO2 in relation to the expired volume, the VCap. Since then, the usefulness of this tool to calculate VD has been highlighted by many authors. Nowadays, major advances in CO2 and flow sensing technology together with powerful hardware and computation capabilities have made real-time breath-by-breath VD calculations possible. The key feature to calculate VD using VCap relies on the precise measurement of mixed expired (PCO2) and the mean alveolar (PACO2) partial pressures of CO2. The first necessary step was the simplification of the measurement of PCO2, which represents the dilution of CO2 within the lungs caused by the dead space effect. Initially, it could only be obtained by the cumbersome measurement using the Douglas’ bag. Currently, PCO2 can be measured by VCap in one single breath by calculating the mixed expired fraction of CO2 (FCO2), which is then transformed to partial pressure and expressed in BTPS as follows:
Figure 2. Differences between Bohr’s and Enghoff’s approaches. The main physiological difference between Bohr’s dead space (VDBohr) and Enghoff’s index is visualized in one volumetric capnogram. While VDBohr represents true dead space in non-perfused alveoli and main conducted airways, the Enghoff’s index includes also the effect of shunt and low V/Q in its calculation (gray area). PaCO2, PACO2, and PCO2 are the arterial, alveolar, and mixed expired partial pressure of carbon dioxide, respectively. VDphys, VDaw, and VDalv are physiological, airway, and alveolar dead spaces, respectively. VT is the tidal volume and VTalv is the alveolar tidal volume.
where VTCO2,br is the amount of CO2 expired in one VT, P is barometric pressure, and PH2O is water vapor pressure. The measurement of PCO2 by VCap has been validated by different research groups, all showing good agreement with the Douglas bag method (Lum et al., 1998; Sinha and Soni, 2012) or a metabolic monitor (Kallet et al., 2005; Siobal et al., 2013) in children (Lum et al., 1998) and adults (Sinha and Soni, 2012) also with ARDS (Kallet et al., 2005; Siobal et al., 2013). More recently, using a more advanced analysis of VCap, Doorduin et al. (2016) compared PCO2 measured by VCap versus the Douglas’ bag in patients with ARDS and found a bias of 0.2 mmHg and limits of agreement of − 3.0 to 4.5 mmHg (Doorduin et al., 2016). Our data in an animal model of ARDS using the multiple inert gas elimination technique (MIGET), the gold standard method for gas-exchange analysis supported the above findings. Our group found a close correlation of PCO2 measured by VCap with MIGET (r = 0.92; p < 0.0001), with a mean bias of −0.5 mmHg and limits of agreement between −2.5 and 1.5 mmHg (Tusman et al., 2011a).
The second, more recently introduced step, was the possibility to measure the mean alveolar partial pressure of CO2 (PACO2). This another essential component of the Bohr’s formula is difficult to measure and is still controversial parameter because PACO2 varies topographically and temporarily within inhomogeneous lungs along the respiratory cycle, even in healthy patients. This means that any single lung unit has its own PACO2 according to its respective V/Q ratio. Therefore, many controversies arose about what the “alveolar gas” really means and what is the proper definition and representative value of PACO2 (Rossier and Buhlmann, 1955). Two different approaches to describe the alveolar gas have been proposed in the past: the ideal and the expired alveolar gas. The ideal alveolar gas concept described by Riley and Cournand is based on the convenient and didactic assumption that the lung behaves as a perfect unit, where PACO2 equals capillary PCO2 (Riley and Cournand, 1949). However, this condition does not really exist even in healthy subjects due to the presence of airway dead space, anatomical shunt, stratified inhomogeneities, spatial and temporal V/Q mismatches, and incomplete gas mixing of inspired gases within the lungs (Fletcher et al., 1981; Crawford et al., 1985; Verbanck and Paiva, 2013). The impossibility to estimate PACO2 in the past was solved by Henrik Enghoff who suggested to use PCO2 in arterial blood (PaCO2) in the Bohr’s formula as a surrogate of PACO2 (Enghoff, 1938). This solution to calculate VD is still used today although, strictly speaking, it calculates not only dead space but also all the spectrum of V/Q mismatch present in the lung (Figure 2). The expired alveolar air concept offers a better and more realistic approximation to PACO2. Alveolar CO2 fluctuates during the respiratory cycle changing ∼4 mmHg between inspiration and expiration. DuBois described the “mean” PACO2 as the absolute value representing the whole lung (Dubois et al., 1952). This concept was confirmed decades later using complex mathematical models that included other aspects that affect alveolar gas composition such as pulmonary capillary pulsatile blood flow, capillary recruitment, solubility of CO2 in pulmonary tissue, and CO2 chemical reactions in blood (Hlastala, 1972). The alveolar gas must necessarily be measured during expiration due to the location of the CO2 sensor at the airway opening where inspiratory gases washes-out any remanent CO2. Mean PACO2 can conveniently be found at the midpoint of the phase III of the VCap. This phase is exclusively composed of alveolar gas where its slope represents the emptying of CO2 from all alveoli at different rates during the expiratory time (Fletcher et al., 1981; Tusman et al., 2012). We tested this hypothesis in an animal model of ARDS by comparing the VCap-based PACO2 with the one derived from the alveolar gas equation solved with data obtained from MIGET. We found a mean bias of − 0.1 mmHg with limits of agreement of −2.18 to 1.98 mmHg (Tusman et al., 2011a). This confirms the original DuBois’ description of mean PACO2, resolving the measurement of PACO2 at the bedside and allowing the non-invasive calculation of Bohr’s dead space breath by breath.
According to the previous discussion, it is clear that Bohr’s and Enghoff’s approaches measure different but complementary aspects of gas exchange (Figure 2) (Tusman et al., 2012; Sipmann et al., 2014). The Bohr’s equation measures “true” dead space because it uses parameters exclusively from the alveolar compartment, and thus is based on the expired alveolar air concept. The PA-CO2 represents the degree of CO2 dilution in naturally heterogeneous human lungs. This was confirmed in a model of ARDS where the Bohr’s dead space calculated by VCap was in an excellent agreement (bias 0.01 and LoA −0.04 to 0.06) with the value obtained with the MIGET analysis (Tusman et al., 2011a). The Enghoff’s approach measures not only dead space but also all the spectrum of V/Q mismatch present in the lung (Figure 2). This is because by using PaCO2 as a surrogate of PACO2 (the ideal alveolar gas concept), the effects of low V/Q and shunt are included in its estimation. The true shunt and low V/Q zones let high venous blood PCO2 pass through the alveoli increasing PaCO2 much above PACO2. Some authors called this effect “shunt” dead space (Fletcher et al., 1981), a misleading term as it mixes the two opposite extremes of V/Q mismatch. We observed a poor correlation between the Enghoff’s approach with MIGET dead space (r = 0.38; p = 0.0078) but a good one with MIGET shunt (r = 0.64; p < 0.0001) (Tusman et al., 2011a). This is why we think that calling the result of the Enghoff’s equation, a global index of gas exchange, as “dead space” is both physiologically and clinically incorrect!
The Bohr’s equation calculates the whole physiological dead space. It can be expressed as an absolute volume in one breath (VDphys in ml), as part of minute ventilation (VD in L) or, more commonly, as a fraction of VT (VD/VT) (Fletcher et al., 1981; Tusman et al., 2012). VCap is the only clinical monitoring tool that separates the volume of gas within conducting airways from the volume of gas in the alveolar compartment in one breath (Figure 2). The classical geometrical method to identify the midpoint of phase II of the capnogram, described by Fowler (1948), can be replaced by a more accurate mathematical analysis (Tusman et al., 2009). This is of great importance as the slope of phase II represents the emptying of lung units of different time constants and V/Q ratios and the midpoint the averaged interface between convective and diffusive intrapulmonary gas transport. We have named this point as the airway–alveolar interface (Figure 1), and it is needed to estimate airway dead space (VDaw) and alveolar VT (VTalv). Failure to correctly estimate this point can lead to interpretation errors of VCap and dead space components (Tusman et al., 2009). Finally, the alveolar dead space (VDalv) is easily obtained by subtracting VDaw from total VDphys (Fletcher et al., 1981). VDaw and VDalv are commonly expressed as a fraction of VT to allow comparisons among different breaths and sizes of patients. The alveolar component is better expressed as a fraction of VTalv (VDalv/VTalv) because it is an index that closely represents the inefficiency of gas exchange (Fletcher et al., 1981; Tusman et al., 2012).
Table 1 shows a summary of published Bohr’s and Enghoff’s values from healthy volunteers to patients with ARDS (Nunn and Hill, 1960; Larsson and Severinghaus, 1962; Blanch et al., 1999; Åström et al., 2000; Beydon et al., 2002; Tusman et al., 2013; Doorduin et al., 2016; Gogniat et al., 2018). Mechanical ventilation per se, apart from pulmonary diseases, increases dead space. In patients with ARDS, VD is elevated by many other factors including instrumental dead space (HME, elbows, close-suction systems), using low VT ventilation, high respiratory rates, pulmonary vascular involvement, or high positive end-expiratory pressure (PEEP), among others. Instrumental dead space may be of major relevance in patients with ARDS because it is an easily modifiable factor and can sum up to 90 to 100 ml in some unfortunate configurations.
As discussed above, cyclic overdistension is one of the major mechanisms of VILI. It is referred to in different terms depending on whether it denotes functional (hyperdistension) or morphological (hyperinflation) phenomena. Recently, a more conceptual and integrative framework adopted from bioengineering terminology has been introduced to describe the stress or lung deformation that the lung parenchyma suffers during inflation, especially at the end of inspiration when maximal airway pressures are reached. Lung stress is defined as the distribution of internal forces per area unit applied to an elastic material, and lung strain as the deformation or change in shape of an elastic material from a reference initial value when submitted to a force (Chiumello et al., 2008). Lung parenchyma is constituted by a network of elastic tissues that normally works within a certain range of normal stress and strain. The topographical distribution of stress and strain is heterogeneous due to the natural fractal configuration of the lungs and by the effects of gravity. Lung parenchyma is prone to damage if the normal limits of stress and strain for a particular lung region are exceeded (Protti et al., 2013; Hurtado et al., 2017).
Volumetric capnography informs about the occurrence of lung overdistension from a different but complementary perspective, as standard and transpulmonary lung mechanics. The Bohr’s dead space increases with positive pressure ventilation, especially in the diseased lungs. The effects of PEEP on VD are well described in the literature (Blanch et al., 1999; Beydon et al., 2002). More importantly, PEEP and the resulting end-inspiratory pressure also increase VDalv as it creates alveolar units with a high V/Q behavior. This is probably the most important parameter related to the risk of developing VILI as it reflects the phenomena occurring at the alveolar level, the most vulnerable part of the lung. Most reports describing the effects of PEEP or MV on VD and VDalv use the Enghoff’s approach, thereby introducing the confounder effect of low V/Q areas on its estimation. In their hallmark paper, Suter et al. (1975) defined the best PEEP as the one resulting in the highest compliance and the lowest VDalv. This level also resulted in a reduced shunt, suggesting that the reduction in VDalv could have been responsible in part to this effect. In an animal model of ARDS, we recently reproduced these results by applying 4 cmH2O of PEEP below and above the “best” PEEP using the VCap analysis. The Bohr’s derived VDalv/VTalv showed the lowest value at the open-lung PEEP and high values with 4 cmH2O of PEEP below or above this value (Figure 3) (Tusman et al., 2011b). Of note, by measuring the Bohr’s (i.e., true) dead space, we specifically analyzed the effects of PEEP on the high V/Q component, that is overdistension, eliminating the confounder of shunt or low V/Q regions, which is an important aspect to consider when aiming at monitoring overdistension.
Figure 3. Dead space at optimum PEEP. Comparison of the data obtained by Suter et al. (1975) using Enghoff’s approach with our more recent data using VCap using Bohr’s approach and multiple inert gas elimination technique (MIGET) (Tusman et al., 2011b). Physiological dead space measured by MIGET and VCap airway dead space increase proportional to PEEP, whereas shunt decreases with PEEP. An individualized level of PEEP (“Best” PEEP) corresponding to maximal respiratory system compliance, resulted in the lowest alveolar dead space and the highest elimination of CO2 measured by VCap. PEEP above and below this optimum value results in an increased alveolar dead space and decreased the elimination of CO2 per breath (VTCO2,br). (*) p < 0.05 compared to best PEEP.
Gogniat et al. (2018) analyzed the effects of PEEP on VD and VDalv in 15 patients with ARDS. They found a similar behavior, i.e., increased overdistension when PEEP was not only above but also below the optimum level set according to the best lung compliance (Table 2) (Gogniat et al., 2018). The authors could identify two clear responses to PEEP when patients were split into two groups according to changes of driving pressure (DP) from baseline ventilation. Patients with DP < 15% (i.e., with better compliance in response to PEEP) presented the lowest dead space and Enghoff’s index values at any PEEP, whereas those with DP > 15% responded exaggeratedly to an increased PEEP. Table 2 shows that the Bohr’s dead space increased proportional to PEEP, whereas Enghoff’s index remained unchanged. As Enghoff’s index includes all V/Q mismatches, it was affected by the opposite simultaneous effects of PEEP on true dead space (increased by overdistension) and shunt (decreased by alveolar recruitment), reducing the sensibility and specificity for detecting lung overdistension.
For examining the role of VD in detecting overdistension in more detail, the effects of increasing PEEP levels from 0 to 30 cmH2O were analyzed in an experimental model of ARDS (Tusman et al., 2020). The Bohr’s VD, with both its airway and alveolar components, increased in proportion when PEEP exceeded 15 cmH2O, reflecting clear global lung overdistension that was confirmed by a parallel decrease in CO2 elimination by the lungs, an increase in lung elastance, transpulmonary DP, and end-inspiratory transpulmonary pressure (Figure 4). However, at PEEP < 10 cmH2O, VDaw was minimal but VDalv increased. This increase was associated to a low VTCO2,br and high lung elastance and transpulmonary DP. End-inspiratory transpulmonary pressure remained stable but high (∼20 cmH2O). These findings could be interpreted as an increase in stress and strain in the alveolar compartment of the aerated lung, despite lower levels of PEEP, caused by the stressor-raiser role of atelectasis (Figures 4B,C).
Figure 4. Effects of PEEP in dead space and lung mechanics. Airway dead space increases in proportion to PEEP and has a direct impact on physiological Bohr’s VD. Alveolar dead space (VDalv), on the contrary, changes according to the balance between recruitment and overdistension in the alveolar compartment. Panel (A) shows the effect of PEEP on absolute dead space values. Panels (B,C) present the differences in alveolar dead space (ΔVDalv), elimination of CO2 per breath (ΔVTCO2,br), transpulmonary driving pressure (ΔDPtp), transpulmonary end-inspiratory pressure (ΔPtpinsp), and lung elastance (ΔEL) between certain PEEP level with the reference15-PEEP considered as the “Best” PEEP according to findings in gas exchange, lung ultrasound, and respiratory mechanics adapted from Tusman et al. (2020).
Recently, a new method for monitoring lung overdistension by directly measuring lung strain based on expired CO2 kinetics, the capnodynamic method, has been described (Suarez-Sipmann et al., 2019). Based on the principles of mass balance for CO2 in the lung and the differential Fick principle for CO2, the capnodynamic equation provides two highly relevant parameters for monitoring purposes: effective pulmonary blood flow (EPBFCO2) (i.e., the non-shunted portion of cardiac output) and end-expiratory lung volume (EELVCO2):
where FACO2n refers to the alveolar fraction of CO2 at the nth breath and FACO2n–1 for the preceding breath. Δtn is the duration of the nth respiratory cycle, CvCO2 and CcCO2n is the mixed venous and capillary CO2 content, VTCO2n is the volume of CO2 eliminated by the nth breath.
The mass balance occurs between the CO2 content in the lung (represented on the left side of the equation) equals the difference between the CO2 supplied to the lung by perfusion and the amount of CO2 eliminated by the lung (on the right side of the equation). To solve the equation for its three unknowns: EELVCO2, EPBFCO2, and CvCO2, a small modification in CO2 alveolar concentration must occur under the assumption that CvCO2 remains constant during the measurement cycle. All other parameters can be obtained non-invasively by VCap. The change in FACO2 is obtained by a minimal modification of the breathing pattern (three consecutive respiratory cycles in which a short expiratory hold is added are repeatedly interspersed between six normal cycles) in a passively breathing patient under MV. Applying a repetitive sequence of six normal and three prolonged breaths, iterative mathematics can solve the capnodynamic equation after a set of nine equations is obtained. From then on, any new breath is added to the sequence providing a new solution (i.e., a new value of EELVCO2 and EPBFCO2, which can be monitored continuously). A more in-depth description of the method is beyond the scope of this manuscript. The method has been submitted to extensive experimental validation in challenging pulmonary and circulatory conditions (Sander et al., 2014, 2015). Recently, the first validations of EPBFCO2 in patients on general anesthesia (Sigmundsson et al., 2021) and of EELVCO2 (Öhman et al., 2020) have been published. Both performed well in good agreement with clinical reference methods and excellent trending abilities.
The decrease in FRC is one of the major contributors to VILI during MV. A way to quantify this risk is to determine lung strain, a measure of lung tissue deformation during inflation. To calculate lung strain, VT needs to be normalized to lung volume (strain = VT/FRC) (Chiumello et al., 2008), which during MV is referred to as end-expiratory lung volume as resting volume is influenced by the level of PEEP applied. When a certain threshold of strain is exceeded, the potential for mechanical damage to the ARDS lung increases (Bellani et al., 2011; González-López et al., 2012). The capnodynamic method offers the unprecedented possibility not only to measure EELV at the bedside, which has been very difficult to date, but also to do it in a non-invasive continuous way, extending and complementing the possibilities of VCap to measure lung overdistension and strain.
The hemodynamic consequence of lung overdistension is crucial information in mechanically ventilated patients with ARDS. Volumetric capnography has the unique ability to describe lung overdistension both from the ventilatory and hemodynamics perspective. Volumetric capnography assesses pulmonary perfusion qualitatively through the parameters PETCO2 and VCO2 (Tusman et al., 2010) and quantitatively by calculating the effective capillary pulmonary blood flow (EPBFCO2) using equations based on the differential Fick’s formula and the above-described capnodynamic method (Sander et al., 2014, 2015). Lung overdistension induced by high alveolar pressure can collapse pulmonary capillaries, decrease pulmonary blood flow, and increase right ventricle afterload. The association of high dead space with low VCO2 or EPBFCO2 has been observed during high PEEP ventilation, resulting in a functional overdistension because CO2 exchange and elimination are impaired.
VCO2 or EPBFCO2 are decreased not only by lung overdistension but also by other hemodynamical causes like hypovolemia, embolism, arrhythmias, or heart failure. Therefore, physicians involved in the care of ventilated patients should first rule out any other hemodynamic problem when evaluating the hemodynamic consequence of lung overdistension. Again, the context-sensitive nature of CO2 kinetics is relevant to make differential diagnoses. Many questions arise when the operator observes changes in pulmonary perfusion in ventilated patients. Is any acute hemodynamic problem of extra-pulmonary origin responsible for the low elimination of CO2? Is the patient with normovolemia or has a preload-dependency? Have alveolar ventilation or body metabolism changed?
The role of VCap to determine the prognosis of patients with ARDS has been well established. Enghoff’s index has been found to be strongly associated with mortality in the early and late course of ARDS (Nuckton et al., 2002; Kallet et al., 2014). The same research group showed that the risk to death increased by 22% for every 0.05 increase in Enghoff’s index (OR = 1.22, 95% CI 1.11–1.35, p < 0.001) in patients with moderate and severe ARDS (Kallet et al., 2017). The magnitude of changes in the Enghoff’s index varied according to ARDS etiology although, in each ARDS subgroup, this variable was always higher in non-survivors than in survivors. Recently, surrogates of VD, such as the ventilatory ratio—an index calculated by the quotient between measured and predicted minute ventilation and PaCO2—was independently associated with mortality in patients with ARDS (Sinha et al., 2019). Why are these CO2-based indexes, such good predictors of survival, better than the usual oxygen-based index in ARDS? When PaCO2 is used to calculate dead space (according to the ideal alveolar gas concept) what it is measured is a global index of gas exchange including low V/Q and shunt. Lung physiology explains that shunt is represented not only by the PA-aO2 but also by the Pa-ACO2 difference. Therefore, these indexes based on PaCO2 reflect the severity of ARDS by assessing shunt in combination with dead space. The prognostic value of Bohr’s “true” dead space in a patient with ARDS is still unknown, but it is very likely that it also has an important role as a direct measure of lung ventilatory inefficiency and overdistension, both with likely strong influence on outcome.
Analysis of expired CO2 kinetics by VCap provides important non-invasive cardiorespiratory information for clinical assessment, monitoring, and management of ARDS mechanically ventilated patients. Dead space and the Enghoff’s index are calculated with high precision even in patients with very severe lung injury, as those with ARDS. The concept of VD is clinically useful not only to assess and adjust alveolar ventilation in the context of lung-protective MV but also to detect alveolar overdistension. Capnodynamic measurement of end-expiratory lung volume allows estimating strain that in combination with lung mechanics and lung perfusion can provide a more in-depth understanding of the lung condition and detect when this value exceeds safe limits. Real-time assessment of the elimination of CO2 and effective pulmonary capillary blood flow provides information about the hemodynamic consequences of positive pressure ventilation. The combination of an increase in VD and a decrease in pulmonary capillary blood flow characterizes a situation of functional lung overdistension. Further studies are needed to explore the precise cutoff value to define harmful functional overdistension with VD and to determine its role as a screening tool to predict the evolution in patients with ARDS.
All authors listed have made a substantial, direct, and intellectual contribution to the work, and approved it for publication.
This study has been funded by Instituto de Salud Carlos III through the project “PI20/01548” (Co-funded by European Regional Development Fund/European Social Fund) “A way to make Europe”/“Investing in your future.”
The authors declare that the research was conducted in the absence of any commercial or financial relationships that could be construed as a potential conflict of interest.
All claims expressed in this article are solely those of the authors and do not necessarily represent those of their affiliated organizations, or those of the publisher, the editors and the reviewers. Any product that may be evaluated in this article, or claim that may be made by its manufacturer, is not guaranteed or endorsed by the publisher.
Aitken, R. S., and Clark-Kennedy, A. E. (1928). On the fluctuation in the composition of the alveolar air during the respiratory cycle in muscular exercise. J. Physiol. 65, 389–411. doi: 10.1113/jphysiol.1928.sp002485
ARDS Network (2000). Ventilation with lower tidal volumes as compared with traditional tidal volumes for acute lung injury and the acute respiratory distress syndrome. N. Engl. J. Med. 342, 1301–1308. doi: 10.1056/nejm200005043421801
Åström, E., Niklason, L., Drefeldt, B., Bajc, M., and Jonson, B. (2000). Partitioning of dead space – a method and reference values in the awake human. Eur. Respir. J. 16, 659–664. doi: 10.1034/j.1399-3003.2000.16d16.x
Bellani, G., Guerra, L., Musch, G., Zanella, A., Patroniti, N., Mauri, T., et al. (2011). Lung regional metabolic activity and gas volume changes induced by tidal ventilation in patients with acute lung injury. Am. J. Respir. Crit. Care Med. 183, 1193–1199. doi: 10.1164/rccm.201008-1318oc
Bellani, G., Messa, C., Guerra, L., Spagnolli, E., Foti, G., Patroniti, N., et al. (2009). Lungs of patients with acute respiratory distress syndrome show diffuse inflammation in normally aerated regions: a [18F]-fluoro-2-deoxy-D-glucose PET/CT study. Crit. Care Med. 37, 2216–2222. doi: 10.1097/ccm.0b013e3181aab31f
Beydon, L., Uttman, L., Rawal, R., and Jonson, B. (2002). Effects of positive end-expiratory pressure on dead space and its partitions in acute lung injury. Intensive Care Med. 28, 1239–1245. doi: 10.1007/s00134-002-1419-y
Blanch, L., Lucangelo, U., Aguilar, J. L., Fernandez, R., and Romero, P. V. (1999). Volumetric capnography in patients with acute lung injury: effects of positive end-expiratory pressure. Eur. Respir. J. 13, 1048–1054.
Chiumello, D., Carlesso, E., Cadringher, P., Caironi, P., Valenza, F., Polli, F., et al. (2008). Lung stress and strain during mechanical ventilation for acute respiratory distress syndrome. Am. J. Respir. Crit. Care Med. 178, 346–355. doi: 10.1164/rccm.200710-1589oc
Crawford, A. B., Makowska, M., Paiva, M., and Engel, L. A. (1985). Convection- and diffusion-dependent ventilation maldistribution in normal subjects. J. Appl. Physiol. 59, 838–846. doi: 10.1152/jappl.1985.59.3.838
Doorduin, J., Nollet, J. L., Vugts, M. P. A. J., Roesthuis, L. H., Akankan, F., Hoeven, J. G., et al. (2016). Assessment of dead-space ventilation in patients with acute respiratory distress syndrome: a prospective observational study. Crit. Care 20:121. doi: 10.1186/s13054-016-1311-8
Dubois, A. B., Britt, A., and Fenn, W. (1952). Alveolar CO2 during the respiratory cycle. J. Appl. Physiol. 4, 535–548. doi: 10.1152/jappl.1952.4.7.535
Enghoff, H. (1938). Volumen inefficax. Bemerkungen zur frage des schädlichen raumes. Uppsala Läkareforen Forhandl 44, 191–218.
Fletcher, R., Jonson, B., Cumming, G., and Brew, J. (1981). The concept of deadspace with special reference to the single breath test for carbon dioxide. Br. J. Anaesth. 53, 77–88. doi: 10.1093/bja/53.1.77
Fowler, W. S. (1948). Lung function studies. II. The respiratory dead space. Am. J. Physiol. Leg. 154, 405–410. doi: 10.1152/ajplegacy.1948.154.3.405
Gattinoni, L., Caironi, P., Cressoni, M., Chiumello, D., Ranieri, V. M., Quintel, M., et al. (2006). Lung recruitment in patients with the acute respiratory distress syndrome. N. Engl. J. Med. 354, 1775–1786. doi: 10.1056/nejmoa052052
Gogniat, E., Ducrey, M., Dianti, J., Madorno, M., Roux, N., Midley, A., et al. (2018). Dead space analysis at different levels of positive end-expiratory pressure in acute respiratory distress syndrome patients. J. Crit. Care 45, 231–238. doi: 10.1016/j.jcrc.2018.01.005
González-López, A., García-Prieto, E., Batalla-Solís, E., Amado-Rodríguez, L., Avello, N., Blanch, L., et al. (2012). Lung strain and biological response in mechanically ventilated patients. Intensive Care Med. 38, 240–247. doi: 10.1007/s00134-011-2403-1
Hlastala, M. P. (1972). A model of fluctuating alveolar gas exchange during the respiratory cycle. Respir. Physiol. 15, 214–232. doi: 10.1016/0034-5687(72)90099-0
Hurtado, D. E., Villarroel, N., Andrade, C., Retamal, J., Bugedo, G., and Bruhn, A. (2017). Spatial patterns and frequency distributions of regional deformation in the healthy human lung. Biomech. Model. Mechanobiol. 16, 1413–1423. doi: 10.1007/s10237-017-0895-5
Kallet, R. H., Daniel, B. M., Garcia, O., and Matthay, M. A. (2005). Accuracy of physiologic dead space measurements in patients with acute respiratory distress syndrome using volumetric capnography: comparison with the metabolic monitor method. Respir. Care 50, 462–467.
Kallet, R. H., Zhuo, H., Ho, K., Lipnick, M. S., Gomez, A., and Matthay, M. A. (2017). Lung injury. and other factors influencing the relationship between dead space fraction and mortality in ARDS. Respir. Care 62, 1241–1248. doi: 10.4187/respcare.05589
Kallet, R. H., Zhuo, H., Liu, K. D., Calfee, C. S., Matthay, M. A., and National Heart Lung and Blood Institute ARDS Network Investigators (2014). The association between physiologic dead-space fraction and mortality in subjects with ARDS enrolled in a prospective multi-center clinical trial. Respir. Care 59, 1611–1618. doi: 10.4187/respcare.02593
Larsson, C. P., and Severinghaus, J. W. (1962). Postural variations in dead space and CO2 gradients breathing air and O2. J. Appl. Physiol. 17, 417–420. doi: 10.1152/jappl.1962.17.3.417
Lum, L., Saville, A., and Venkataraman, S. T. (1998). Accuracy of physiologic deadspace measurement in intubated pediatric patients using a metabolic monitor. Crit. Care Med. 26, 760–764. doi: 10.1097/00003246-199804000-00029
Nuckton, T. J., Alonso, J. A., Kallet, R. H., Daniel, B. M., Pittet, J.-F., Eisner, M. D., et al. (2002). Pulmonary dead-space fraction as a risk factor for death in the acute respiratory distress syndrome. N. Engl. J. Med. 346, 1281–1286. doi: 10.1056/nejmoa012835
Nunn, J. F., and Hill, D. W. (1960). Respiratory dead space and arterial to end-tidal CO2 difference in anesthetized man. J. Appl. Physiol. 15, 383–389. doi: 10.1152/jappl.1960.15.3.383
Öhman, T., Sigmundsson, T. S., Hallbäck, M., Sipmann, F. S., Wallin, M., Oldner, A., et al. (2020). Clinical and experimental validation of a capnodynamic method for end-expiratory lung volume assessment. Acta Anaesthesiol. Scand. 64, 670–676. doi: 10.1111/aas.13552
Protti, A., Andreis, D. T., Monti, M., Santini, A., Sparacino, C. C., Langer, T., et al. (2013). Lung stress and strain during mechanical ventilation. Crit. Care Med. 41, 1046–1055. doi: 10.1097/ccm.0b013e31827417a6
Riley, R. L., and Cournand, A. (1949). “Ideal”; alveolar air and the analysis of ventilation-perfusion relationships in the lungs. J. Appl. Physiol. 1, 825–847.
Rossier, P., and Buhlmann, A. (1955). The respiratory dead space. Physiol. Rev. 35, 860–876. doi: 10.1152/physrev.1955.35.4.860
Sander, C. H., Hallback, M., Wallin, M., Emtell, P., Oldner, A., and Bjorne, H. (2014). Novel continuous capnodynamic method for cardiac output assessment during mechanical ventilation. Br. J. Anaesth. 112, 824–831. doi: 10.1093/bja/aet486
Sander, C. H., Lönnqvist, P.-A., Hallbäck, M., Sipmann, F. S., Wallin, M., Oldner, A., et al. (2015). Capnodynamic assessment of effective lung volume during cardiac output manipulations in a porcine model. J. Clin. Monit. Comput. 30, 761–769. doi: 10.1007/s10877-015-9767-7
Sigmundsson, T. S., Öhman, T., Hallbäck, M., Suarez-Sipmann, F., Wallin, M., Oldner, A., et al. (2021). Comparison between capnodynamic and thermodilution method for cardiac output monitoring during major abdominal surgery: an observational study. Eur. J. Anaesth. doi: 10.1097/eja.0000000000001566 [Epub online ahead of print].
Sinha, P., Calfee, C. S., Beitler, J. R., Soni, N., Ho, K., Matthay, M. A., et al. (2019). Physiologic analysis and clinical performance of the ventilatory ratio in acute respiratory distress syndrome. Am. J. Respir. Crit. Care Med. 199, 333–341. doi: 10.1164/rccm.201804-0692oc
Sinha, P., and Soni, N. (2012). Comparison of volumetric capnography and mixed expired gas methods to calculate physiological dead space in mechanically ventilated ICU patients. Intensive Care Med. 38, 1712–1717. doi: 10.1007/s00134-012-2670-5
Siobal, M. S., Ong, H., Valdes, J., and Tang, J. (2013). Calculation of physiologic dead space: comparison of ventilator volumetric capnography to measurements by metabolic analyzer and volumetric CO2 monitor. Respir. Care 58, 1143–1151. doi: 10.4187/respcare.02116
Sipmann, F. S., Böhm, S. H., and Tusman, G. (2014). Volumetric capnography: the time has come. Curr. Opin. Crit. Care 20, 333–339. doi: 10.1097/mcc.0000000000000095
Suarez-Sipmann, F., Tusman, G., and Wallin, M. (2019). “Continuous non-invasive monitoring of cardiac output and lung volume based on CO2 kinetics,” in Annual Update in Intensive Care and Emergency Medicine 2019, ed. J.-L. Vincent (Cham: Springer), 215–229. doi: 10.1007/978-3-030-06067-1_16
Suter, P. M., Fairley, B., and Isenberg, M. D. (1975). Optimum end-expiratory airway pressure in patients with acute pulmonary failure. N. Engl. J. Med. 292, 284–289. doi: 10.1056/nejm197502062920604
Tusman, G. Böhm, S. H., Sipmann, F. S., Scandurra, A., and Hedenstierna, G. (2010). Lung recruitment and positive end-expiratory pressure have different effects on CO2 elimination in healthy and sick lungs. Anes. Anal. 111, 968–977. doi: 10.1213/ane.0b013e3181f0c2da
Tusman, G., Gogniat, E., Böhm, S. H., Scandurra, A., Sipmann, F. S., Torroba, A., et al. (2013). Reference values for volumetric capnography-derived non-invasive parameters in healthy individuals. J. Clin. Monit. Comput. 27, 281–288. doi: 10.1007/s10877-013-9433-x
Tusman, G., Gogniat, E., Madorno, M., Otero, P., Dianti, J., Ceballos, I. F., et al. (2020). Effect of PEEP on dead space in an experimental model of ARDS. Respir. Care 65, 11–20. doi: 10.4187/respcare.06843
Tusman, G., Scandurra, A., Böhm, S. H., Sipmann, F. S., and Clara, F. (2009). Model fitting of volumetric capnograms improves calculations of airway dead space and slope of phase III. J. Clin. Monit. Comput. 23, 197–206. doi: 10.1007/s10877-009-9182-z
Tusman, G., Sipmann, F. S., and Böhm, S. H. (2012). Rationale of dead space measurement by volumetric capnography. Anesth. Analg. 114, 866–874. doi: 10.1213/ane.0b013e318247f6cc
Tusman, G., Sipmann, F. S., Borges, J. B., Hedenstierna, G., and Böhm, S. H. (2011a). Validation of Bohr dead space measured by volumetric capnography. Intensive Care Med. 37, 870–874. doi: 10.1007/s00134-011-2164-x
Tusman, G., Suarez-Sipmann, F., Bohm, S. H., Borges, J. B., and Hedenstierna, G. (2011b). Capnography reflects ventilation/perfusion distribution in a model of acute lung injury. Acta Anaesthesiol. Scand. 55, 597–606. doi: 10.1111/j.1399-6576.2011.02404.x
Keywords: volumetric capnography, dead space, acute respiratory distress syndrome, ventilator-induced lung injury, mechanical ventilation
Citation: Suárez-Sipmann F, Villar J, Ferrando C, Sánchez-Giralt JA and Tusman G (2021) Monitoring Expired CO2 Kinetics to Individualize Lung-Protective Ventilation in Patients With the Acute Respiratory Distress Syndrome. Front. Physiol. 12:785014. doi: 10.3389/fphys.2021.785014
Received: 28 September 2021; Accepted: 18 October 2021;
Published: 21 December 2021.
Edited by:
Gary Frank Nieman, SUNY Upstate Medical University, United StatesReviewed by:
Penny Andrews, Upstate Medical University, United StatesCopyright © 2021 Suárez-Sipmann, Villar, Ferrando, Sánchez-Giralt and Tusman. This is an open-access article distributed under the terms of the Creative Commons Attribution License (CC BY). The use, distribution or reproduction in other forums is permitted, provided the original author(s) and the copyright owner(s) are credited and that the original publication in this journal is cited, in accordance with accepted academic practice. No use, distribution or reproduction is permitted which does not comply with these terms.
*Correspondence: Fernando Suárez-Sipmann, ZnN1YXJlenNpcG1hbm5AZ21haWwuY29t
†These authors have contributed equally to this work
Disclaimer: All claims expressed in this article are solely those of the authors and do not necessarily represent those of their affiliated organizations, or those of the publisher, the editors and the reviewers. Any product that may be evaluated in this article or claim that may be made by its manufacturer is not guaranteed or endorsed by the publisher.
Research integrity at Frontiers
Learn more about the work of our research integrity team to safeguard the quality of each article we publish.