- 1Key Laboratory of Mariculture, Ocean University of China, Ministry of Education, Qingdao, China
- 2Quality and Safety Center of Agricultural and Livestock Products, Bayannaoer, China
Heat shock proteins (HSPs) are a large class of highly conserved chaperons, which play important roles in response to elevated temperature and other environmental stressors. In the present study, 5 HSP90 genes and 17 HSP70 genes were systematically characterized in spotted seabass (Lateolabrax maculatus). The evolutionary footprint of HSP genes was revealed via the analysis of phylogeny, chromosome location, and gene copy numbers. In addition, the gene structure features and the putative distribution of heat shock elements (HSEs) and hypoxia response elements (HREs) in the promoter regions were analyzed. The protein-protein interaction (PPI) network analyses results indicated the potential transcriptional regulation between the heat shock factor 1 (HSF1) and HSPs and a wide range of interactions among HSPs. Furthermore, quantitative (q)PCR was performed to detect the expression profiles of HSP90 and HSP70 genes in gill, liver, and muscle tissues after heat stress, meanwhile, the expression patterns in gills under alkalinity and hypoxia stresses were determined by analyzing RNA-Seq datasets. Results showed that after heat stress, most of the examined HSP genes were significantly upregulated in a tissue-specific and time-dependent manners, and hsp90aa1.1, hsp90aa1.2, hsp70.1, and hsp70.2 were the most intense responsive genes in all three tissues. In response to alkalinity stress, 11 out of 13 significantly regulated HSP genes exhibited suppressed expression patterns. Alternatively, among the 12 hypoxia-responsive-expressed HSP genes, 7 genes showed induced expressions, while hsp90aa1.2, hsp70.1, and hsp70.2 had more significant upregulated changes after hypoxic challenge. Our findings provide the essential basis for further functional studies of HSP genes in response to abiotic stresses in spotted seabass.
Introduction
Heat shock proteins (HSPs) are a large group of molecular chaperones with highly conserved sequence structures, which are classified into five families, namely, HSP90, HSP70, HSP60/HSP10, HSP40, and small heat shock protein (sHSP) based on their molecular weights (MWs). The expressions of HSPs are constitutive as housekeeping genes in unstressed cells or induced significantly by abiotic and biotic stressors (Feder and Hofmann, 1999). Since the first discovery of HSPs in the salivary gland cells of the fruit fly (Drosophila busckii) (Ritossa, 1962; Tissiéres et al., 1974), the identification and function studies of HSPs have been widely reported in a broad range of vertebrates and invertebrates. For aquaculture species, HSP90 and HSP70 are the most widely studied HSPs in the very large HSP family, which are essential for the maintenance of protein homeostasis and cellular recovery after various environmental stresses (Hangzo et al., 2016; Umam et al., 2016; Gupta et al., 2020).
Heat shock proteins 90 and HSP70 genes are evolutionarily conserved molecular chaperones that are important for the folding and regulation of a variety of cellular proteins in an adenosine triphosphate (ATP)-dependent manner (Sib Sankar et al., 2014). In humans, 6 HSP90 genes (HSP90AA1, HSP90AA2, HSP90AB1, HSP90B1, TRAP1, and HSP90N) and 17 HSP70 genes (HSPA6, HSPA7, HSPA4L, HSPA9B, HSPA4, HSPA1L, HSPA1A, HSPA1B, HSPA5, HSPA12A, HSPA14, HSPA8, HYOU1, HSPH1, HSPA2, HSPA12B, and STCH) were identified (Chen et al., 2005; Brocchieri et al., 2008). Functional HSP90 is a homodimer with each monomer composed of three major domains: an ATP binding N-terminal domain (N-domain) (Prodromou et al., 1997), a protein-binding middle domain (M-domain), and a C-terminal domain (C-domain) that interacts with co-chaperones (Street et al., 2011; Xie et al., 2015). The three domains that are linked via flexible linkers can homodimerize to create an HSP90 active unit, which has a conserved function in the reconfiguration of abnormally folded proteins to their normal state through ATP hydrolysis and structural rearrangement (Zuehlke and Johnson, 2010). HSP70s are the most highly conserved HSPs, which contain two major domains: an N-domain that controls the interaction with the client protein, and a C-domain, which is the substrate-binding domain that identifies the hydrophobic regions in the client during its initial folding stage. The two domains are also connected by a flexible linker (Bukau et al., 2006; Gupta et al., 2020). When HSP70 is not bound to a cellular protein, its ATPase activity is lower than average. The co-chaperon, J-domain protein family, assists client protein to bind with HSP70, vitalizing its ATPase activity, and facilitating protein folding and transport (Gupta et al., 2020). HSP70 transforms to its apo form by liberating ADP from it after the J-domain protein leaves this, which makes the N-domain engage ATP, leading to native conformation of client protein to release (Schlecht et al., 2011; Gupta et al., 2020). In addition to carrying out chaperone activities independently, HSP90 and HSP70 were identified to interact directly and collaborate to assist in numerous cellular progresses (Wegele et al., 2006; Zuehlke and Johnson, 2010; Doyle et al., 2019; Gupta et al., 2020).
Fish are frequently subjected to varieties of environmental pressures, such as high or low temperature, low oxygen, osmotic stress, or other challenges caused by poor water quality. Owing to the important roles of HSP90 and HSP70 genes in response to abiotic stress factors, it is of great significance to understand their molecular structures and functional mechanism in teleosts. As the increasing number of available genomic resources, more and more HSP90 genes and HSP70 genes have been discovered in teleost species, and their potential involvement under environmental stresses have been investigated by examining the gene expression changes. For example, a total of 8 HSP90 and 16 HSP70 genes were identified in rainbow trout (Oncorhynchus mykiss), respectively (Ma et al., 2020; Ma and Luo, 2020). The significantly differential expressions of six HSP90 genes and four HSP70 genes in the liver, and six HSP90 genes and six HSP70 genes in the head kidney were reported after heat stress treatment, indicating they may take part in heat stress response in rainbow trout (Ma et al., 2020). In large yellow croaker (Larimichthys crocea), systematic gene characterization has been conducted for the HSP70 family and 17 genes have been identified. By examining RNA-seq data, six HSP70 genes were significantly upregulated or downregulated in the liver after cold or heat challenge, indicating their involvement in defending against thermal stresses (Xu et al., 2018). Moreover, the complete gene sets of HSP90 and/or HSP70 have been identified for channel catfish (Ictalurus punctatus) and mudskipper (Boleophthalmus pectinirostris) (Song et al., 2014; Xie et al., 2015; Deng et al., 2019). In addition, differentially expression of HSP90 and HSP70 genes after the hypoxia stress (Shi et al., 2020), hypotonic stress (Umam et al., 2016), and ammonia stress (Hangzo et al., 2016) has been demonstrated in fishes. These studies suggest that HSP90 and HSP70 genes are potential modulators participating in the heat and other abiotic stress, and the functions of HSPs genes may vary among different teleost species.
Spotted seabass (Lateolabrax maculatus; L. maculatus) is an economically important aquaculture fish species in China of its high yield, high nutritional value, and pleasant taste (Liu et al., 2020; Yu et al., 2020) with the annual production exceeding 190,000 tons in recent years (China Fishery Statistical Yearbook, 2021; Li et al., 2021). Nevertheless, it is facing various environmental threatens, such as heat stress caused by global warming, salinity stress derived from tidal water flow, and the oxidative stress along with the development of intensive aquaculture. These stressors lead to a reduction of the fitness of fish or even death, severely affecting its economic benefits. However, little is known about the roles of HSP molecular chaperones under environmental stresses in spotted seabass. Therefore, in this study, systematic identification and characterization of HSP90 and HSP70 genes were conducted in spotted seabass. Through phylogenetic and homology analyses, their annotation was determined, and their evolutionary relationship was clarified. Furthermore, the presence of conserved Cis-regulatory elements in the promoter region and the potential protein interaction network dominated by HSP90 and HSP70 were predicted. To provide insight into the function of the HSP90 and HSP70 genes of spotted seabass in response to various environmental stresses, their expression profiles in target tissues were detected after being challenged by different abiotic stress conditions, such as heat, alkalinity, and hypoxia stresses. Our results will reveal the molecular characteristics of the HSP90 and HSP70 gene family in spotted seabass and provide a theoretical basis for the in-depth study of their biological functions under abiotic stresses.
Materials and Methods
Identification of Heat Shock Protein Genes in Spotted Seabass
To identify HSP90 and HSP70 genes in L. maculatus, the reference genome (BioProject: PRJNA408177) and transcriptomic databases (SRR4409341, SRR4409397) were searched using TBLASTN based on the query sequences of HSP90 and HSP70 genes from human, mouse (Mus musculus), chicken (Gallus gallus), tropical clawed frog (Xenopus tropicalis), zebrafish, spotted gar (Lepisosteus oculatus), Mexican tetra (Astyanax mexicanus), tongue sole (Cynoglossus semilaevis), Atlantic salmon (Salmo salar), and channel catfish (Ictalurus punctatus) retrieved from the National Center for Biotechnology Information (NCBI)1 and previous research (Song et al., 2014) (The query sequence identifiers are listed in Supplementary Table 1), with a cutoff E-value of 1e––5. The open reading frames (ORFs) were predicted, and the retrieved sequences were translated by ORF Finder.2 Predicted ORFs were validated by BLASTP against NCBI non-redundant protein database.
The gene copy numbers of HSP90 and HSP70 family were compared based on the genome databases of spotted seabass and several selected vertebrates, such as human, mouse, chicken, zebrafish, channel catfish, Japanese medaka (Oryzias latipes), fugu (Takifugu rubripes), spotted gar, large yellow croaker, and Nile tilapia (Oreochromis niloticus). The chromosomal location of each HSP gene was shown according to the coordinates of each HSP gene on the spotted seabass genome.
Phylogenetic and Syntenic Analysis
Phylogenetic analysis was conducted using the amino acid sequences of HSP90 and HSP70 genes from several representative vertebrates retrieved from NCBI and Ensembl3 databases, such as human, mouse, chicken, zebrafish, spotted gar, Atlantic salmon, channel catfish, Nile tilapia, large yellow croaker, fugu, and Japanese medaka (Supplementary Table 1). After conducting multiple alignments of HSP sequences by MUSCLE with default parameters, the phylogenetic tree was constructed using MEGA 7 with the neighbor-joining method. Jones-Taylor-Thornton (JTT) + invariant sites (I) + gamma distribution for modeling rate heterogeneity (G) model was selected and bootstrapping with 1,000 replications was conducted to evaluate the phylogenetic tree. The tree was further modified using Interactive Tree of Life 6 (iTOL 64).
The syntenic analysis was performed to provide additional evidence for the annotation of the duplicated HSP90 and HSP70 genes. The neighboring genes of hsp90aa1, hspa8a, hyou1, hsp70.1, and hsp70.2 were identified from spotted seabass reference genome and further compared with the genome regions of selected representative vertebrates, which were obtained from NCBI and Genomicus databases 100.015.
Sequence Analysis of HSP90 and HSP70 Genes
Protein characteristics (MW and isoelectric points, pIs) of HSP genes in spotted seabass were predicted by the Prot Param tool6 using the deduced amino acids. The homologous domain was surveyed by the SMART 7.0 program7 and the NCBI conserved domain database.8 The gene structures of HSPs were obtained from the L. maculatus reference genome database and were visualized using the GSDS 2.0 software.9 The conserved motifs of HSPs were observed with the MEME online tool10 and were displayed using TBtools 1.068.
For the prediction of heat shock elements (HSEs) and hypoxia response elements (HREs), 2-kb upstream regions from the transcription start site (TSS) of each HSP gene were extracted from the spotted seabass reference genome and analyzed using JASPAR 2018 server.11
Protein-Protein Interaction Network Prediction
The Protein-Protein Interaction (PPI) relationships of the HSPs were predicted by constructing a zebrafish association model using STRING 11.0 software12 with deduced amino acid sequences of spotted seabass.
Heat Stress Experiment
The heat stress experiment was conducted at Shuangying Aquaculture Company, Dongying, Shandong, China. Sixty spotted seabass juveniles (body length: 13.33 ± 0.24 cm, body weight: 38.96 ± 2.01 g) were collected and acclimated for 2 weeks in a tank [5 m × 5 m × 1.5 m (L × W × H)]. During acclimation period, the experimental conditions were kept constant, such as water temperature (25.0 ± 1.0oC), pH (7.5 ± 0.4), salinity (31 ± 1.0 ppt), and dissolved oxygen (DO) (7.0 ± 0.5 mg/L).
After acclimation, 60 individuals were transferred to three tanks at the density of 20 per tank. The temperature was settled as 25oC, and fish were acclimated for 48 h. After that, the water temperature was increased at a constant rate of 1oC/h until it reached 32°C, and thereafter, the temperature was maintained. Three individuals per tank were sampled at each time point, such as 0 h (H0), 10 h (3 h after heat stress, H3), 19 h (12 h after heat stress, H12), and 31 h (24 h after heat stress, H24) after heat stress (Supplementary Figure 1). Sampled fish were anesthetized with tricaine methanesulfonate (MS-222, 200 mg/L), and gill, liver, and muscle tissues were dissected and flash-frozen in liquid nitrogen for RNA extraction.
Expression of HSP90 and HSP70 Genes Under Heat Stress by Quantitative Real-Time PCR Analysis
Total RNA of gill, liver, and muscle tissues in heat stress experiment was extracted using TRIzol reagent (Invitrogen, Waltham, CA, United States) according to the instructions of manufacturer. RNA concentration and integrity were measured by using the Biodropsis BD-1000 nucleic acid analyzer (Beijing Oriental Science & Technology Development Ltd., Beijing, China) and 1.5% agarose gel electrophoresis, respectively. cDNA was synthesized using PrimeScriptTM RT reagent Kit (Takara, Otsu, Japan) following the protocol of the manufacturer. qPCR was performed using the StepOne Plus Real-Time polymerase chain reaction (PCR) system (Applied Biosystems, Foster City, CA, United States) to detect the expression patterns of HSP90 and HSP70 mRNA. Primers of HSP90 and HSP70 genes were designed by Primer 6 software (Table 1). 18S rRNA was used as the internal control to correct the qPCR veracity, and the samples were run in triplicate (Wang et al., 2018). The 20 μl qPCR reaction volume included 2 μl template cDNA, 0.4 μl each forward/reverse primer, 10 μl 2 × ChamQ SYBR Color qPCR Master Mix, and 7.2 μl of nuclease-free water. The qPCR amplification was carried out using the following procedures: 95oC for 30 s and 40 cycles of 95oC for 5 s, 55oC for 30 s, and 72oC for 30 s. The relative mRNA expression levels of HSP90 and HSP70 genes were calculated according to the 2–ΔΔCT method.
ANOVA and Duncan’s multiple tests were applied to assess the means of the relative mRNA expression level using SPSS 26.0 software. The differences were considered as statistically significant when the P-value < 0.05. The graphs were depicted by the software of GraphPad Prism.8.0.2.
Expression Profiles of HSP90 and HSP70 Genes Following Alkalinity and Hypoxia Treatments by Analysis of RNA-Seq Datasets
To investigate the responsiveness of HSP90 and HSP70 genes to different abiotic stress conditions, RNA-Seq datasets generated by our previous challenge experiments of alkalinity and hypoxia were used to determine the gene expression pattern.
Briefly, for the alkalinity challenge experiment, 1-year-old spotted seabass individuals (body weight: 140.32 ± 2.56 g) were firstly acclimated in fresh water (pH: 7.8 ± 0.4) for 30 days. Carbonate-alkalinity solution was prepared by adding NaHCO3 (12.8 mmol/L) and Na2CO3 (2.6 mmol/L) to fresh water and aerated for 24 h before the experiment. The carbonate alkalinity (mmol/L) was monitored every day during the exposure period using acidimetric titrations. After acclimation, 45 spotted seabass individuals were immediately transferred to three replicated 100 L square tanks with alkaline water (carbonate alkalinity: 18 ± 0.2 mmol/L), and the dissolved oxygen concentration, temperature, and pH were maintained at 7.1 ± 0.4 mg/L, 22 ± 1oC and pH: 9.0 ± 0.2, respectively. The fish were not fed during the stress experiment. Gill tissues of three fish individuals in each tank were sampled at several time points, such as 0, 12, 24, and 72 h after alkalinity stress. The samples were frozen in liquid nitrogen and stored at − 80oC for RNA extraction. RNA of the three individuals from the same tank was pooled as one sample, and 12 sequencing libraries (3 replicated samples × 4 time points) were generated. Total 150 bp paired-end reads (PRJNA611641) were obtained by the Illumina HiSeq X Ten platform.
For hypoxic treatment experiment, spotted seabass individuals (body weight: 178.25 ± 18.56 g) were randomly divided into two groups: normoxic group (6.89 ± 0.25mg/L) and hypoxic group (1.1 ± 0.14 mg O2/L) in triplicate tanks at the density of 20 fish per tank. The threshold DO level was set as 1.1 ± 0.14 mg O2/L, which was determined by preliminary experiments. The oxygen level of the hypoxia group was reduced to 1.1 ± 0.14 mg/L by bubbling nitrogen gas for 30 min. During the experiment period, the dissolved oxygen levels were maintained constant by a mixture of air and nitrogen gas. Gill tissues of three fish per tank (a total of nine individuals for each time point) were sampled at 0, 3, 6, and 12 h after the hypoxia challenge. A total of 12 sequencing libraries (3 replicated samples X 4 time points) were generated. Total 150 bp paired-end reads (unpublished data) were generated using the Illumina HiSeq 4000 platform.
For each RNA-seq project, the high-quality clean reads from each library were generated using Trimmomatic 0.36 and then mapped to the reference genome of spotted seabass (PRJNA408177) using HISAT 2 (-p 4 –dta -t –phred33) with no mismatch. The mapped reads from alignments were counted and then normalized to determine expected number of Fragment Per Kilobase of transcript sequence per Million base pairs sequenced (FPKM). Differential expression in fold change of each HSP gene was determined based on the ratio of the expression value. Differential expression statistical analysis was performed using the DESeq 2 R package with P-value < 0.05.
Results
Identification and Characteristics of HSP90 and HSP70 Genes in Spotted Seabass
A total of 5 HSP90 (hsp90aa1.1, hsp90aa1.2, hsp90ab1, hsp90b1, and trap1) and 17 HSP70 (hspa8a.1, hspa8a.2, hspa8b, hsc70, hspa1b, hsp70.1, hsp70.2, hspa5, hspa9, hspa13, hyou1, hspa4a, hspa4b, hspa4l, hspa14, hspa12a, and hspa12b) genes were identified in spotted seabass. Their detailed information is summarized in Table 2. The ORF of HSP90 genes was ranged from 1,944 to 2,241 bp, encoding protein lengths different from 647 to 744 amino acid (aa). Their predicted MW was varied from 74.22 to 85.31 kDa, and pI were from 4.65 to 5.99. HSP70 genes contained ORFs varying from 1,329 to 2,982 bp in length and encoded proteins with 442 to 993 aa. MWs of HSP70 genes were distinct from 48.10 to 110.39 kDa, and pIs were from 4.95 to 7.22. All HSPs sequences of spotted seabass had been submitted to GenBank databases, and their accession numbers are listed in Table 2.
Phylogenetic and Syntenic Analysis
As shown in Figure 1A, HSP90 genes of spotted seabass were clustered with respective counterparts and four clades were generated, namely, HSP90AA1, HSP90AB1, HSP90B1, and TRAP1, which were consistent with their annotation. Two copies of hsp90aa1 (hsp90aa1.1 and hsp90aa1.2) in spotted seabass were orthologous to teleost hsp90aa1.1 and hsp90aa1.2, respectively.
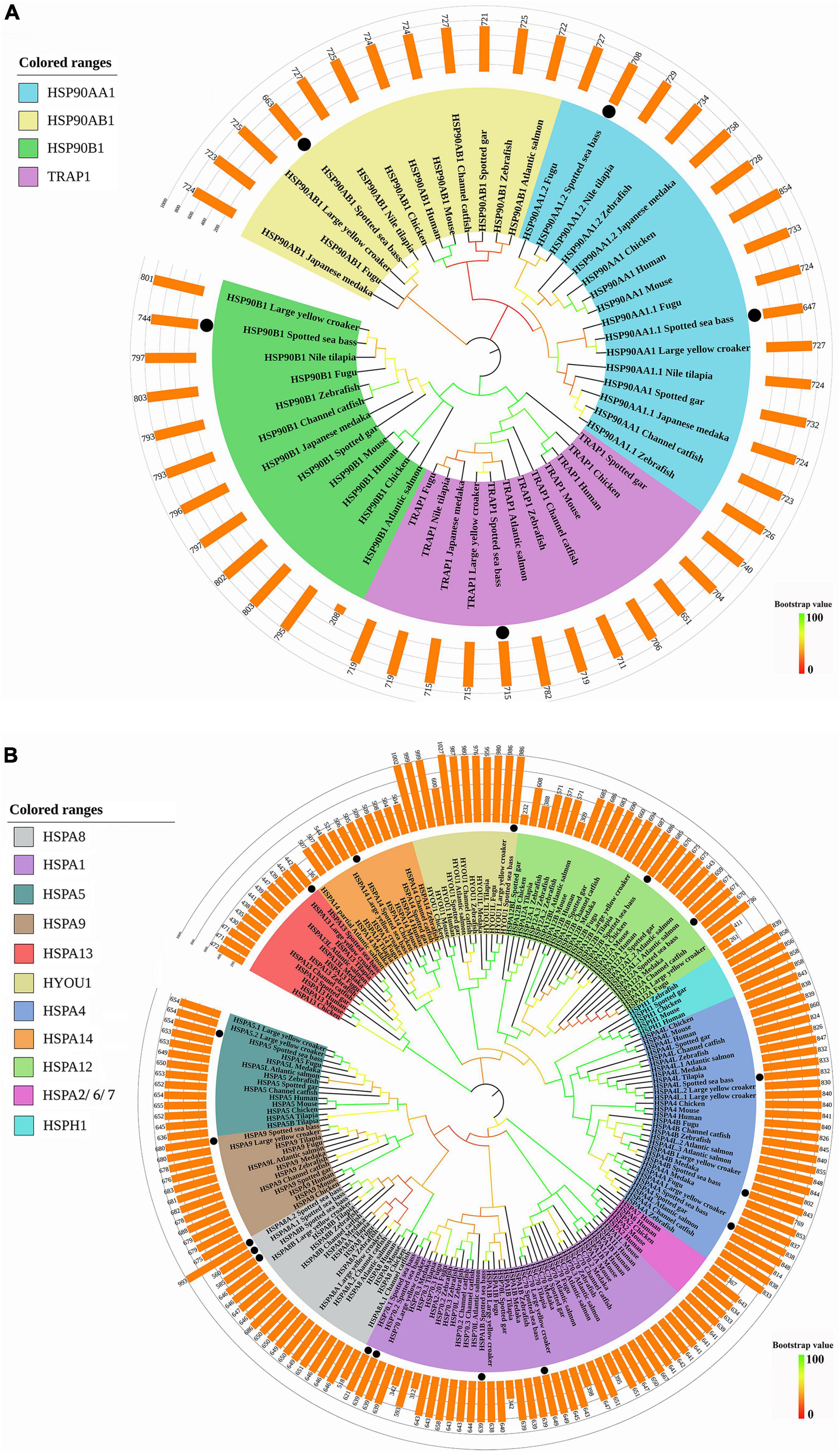
Figure 1. Phylogenetic analyses of (A) HSP90 and (B) HSP70 genes. The phylogenetic tree was constructed by the deduced amino acid sequences with 1,000 bootstrap replications in MEGA 7. Different sizes of predicted amino acids of these genes were represented by the bars upon the phylogenetic tree. The black dots represented the genes of spotted seabass.
All the HSP70 genes of spotted seabass were well distributed into distinct clades and grouped with homologous genes of selected species, supported by strong bootstrap values (Figure 1B). Eleven clades were generated, such as HSPA8, HSPA1, HSPA5, HSPA9, HSPA13, HYOU1, HSPA4, HSPA14, HSPA12, HSPA2/6/7, and HSPH1, and HSP70 genes of spotted seabass were distributed in nine of them (HSPA8, HSPA1, HSPA5, HSPA9, HSPA13, HYOU1, HSPA4, HSPA14, and HSPA12). HSPH1 genes were only discovered for selected tetrapods and a few teleosts, meanwhile hspa2/6/7 was specific to tetrapods. The annotation of five genes (hspa5, hspa9, hspa13, hyou1, and hspa14) with a single copy and duplicated genes, such as hspa12 (hspa12a and hspa12b) and hspa4 (hspa4a, hspa4b, and hspa4l), could be well supported by the phylogenetic relationships, but it is hard to distinguish and name the remaining HSP70 genes with duplicated copies solely by phylogeny.
Thus, syntenic analysis was performed to provide additional evidence for the annotation of three duplicated HSP genes in spotted seabass (Figure 2). For hsp90aa1, the hsp90aa1.1 and hsp90aa1.2 of zebrafish and spotted seabass were tandem located, with highly similar upstream neighbor genes like rps29 and mgat2 and downstream genes as ppp2r5cb, dio3b, slc25a47a and slc25a29. A unique gene copy of HSP90AA1 was found for human, and its neighbor genes, such as WDR20, PPP2R5C, and DIO3, were conserved with tested teleosts (Figure 2A). For all tested three species, hspa8/hspa8a and hyou1 were located at the same chromosome. Duplicated gene copies of hspa8a (hspa8a.1 and hspa8a.2) were identified for spotted seabass, alternatively, human and zebrafish harbored single hspa8 and hspa8a genes, respectively. Neighboring regions of hspa8a were more conserved between zebrafish and spotted seabass in comparison with human (Figure 2B). The synteny analyses of hsp70.1 and hsp70.2 were only conducted in zebrafish and spotted seabass due to the absence of hsp70.1 and hsp70.2 genes in human. As shown in Figure 2C, hsp70.1 and hsp70.2 were tandem duplicated in the zebrafish genome, while hsp70.1 and hsp70.2 in spotted seabass were separated by nlk2, cdipt, and TAO2. However, they shared a relatively conserved genomic neighborhood.
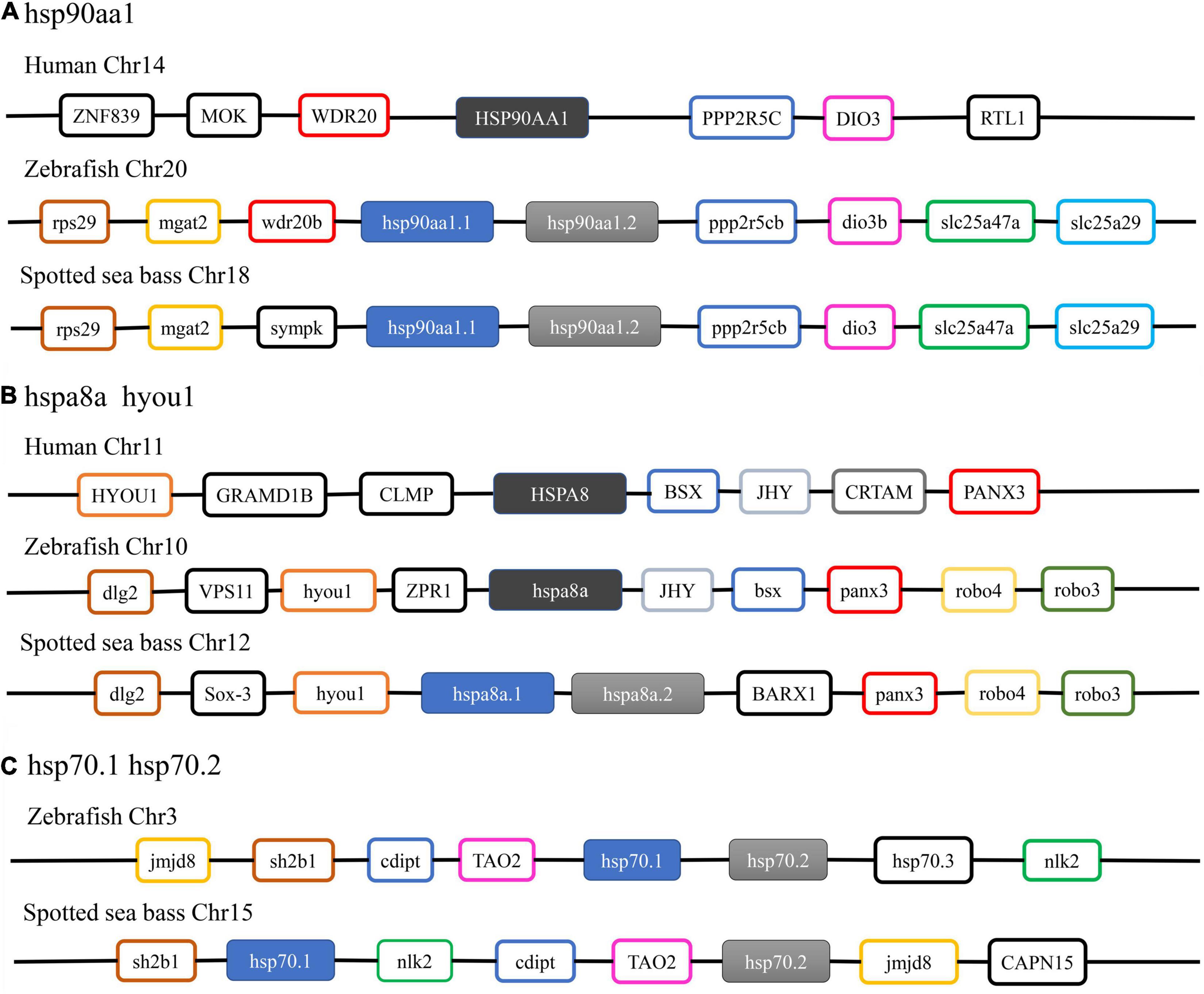
Figure 2. Syntenic analysis of (A) hsp90aa1; (B) hspa8a; (C) hsp70.1 and hsp70.2 among human, zebrafish, and spotted seabass.
Taken together, the phylogenetic and syntenic analysis results not only well support the annotation and nomenclature of the HSP90 and HSP70 genes in spotted seabass but also indicate that these HSP gene families were highly conserved in the evolution.
Chromosome Distribution
For the chromosomal location of target HSP genes, 5 HSP90 genes were distributed on three chromosomes, and 17 HSP70 genes were located on 10 chromosomes and one un-anchored scaffold, respectively (Figure 3). In detail, for the HSP90 family, hsp90ab1 and tandem duplicated hsp90aa1.1 and hsp90aa1.2 were located on chr18, meanwhile, hsp90b1 and trap1 were located on chr6 and chr15, respectively. For the HSP70 family, chr12 possessed the largest gene number of HSP70s, that four genes, such as the tandem arranged hspa8a.2 and hspa8a.1, and hyou1 and hspa4a, were located on it. The tandem duplicated hsp70.1 and hsp70.2, and hsc70 were positioned on chr15. Additionally, two HSP70 genes, hspa13 and hspa8b, were located on chr5. For the rest nine HSP70 genes (hspa1b, hspa12a, hspa4b, hspa5, hspa4l, hspa14, hspa12b, and hspa9), they were distributed on the different chromosomes and scaffold fragment (Chr9, Chr13, Chr14, Chr17, Chr19, Chr22, Chr23, and scaffold_52), respectively.
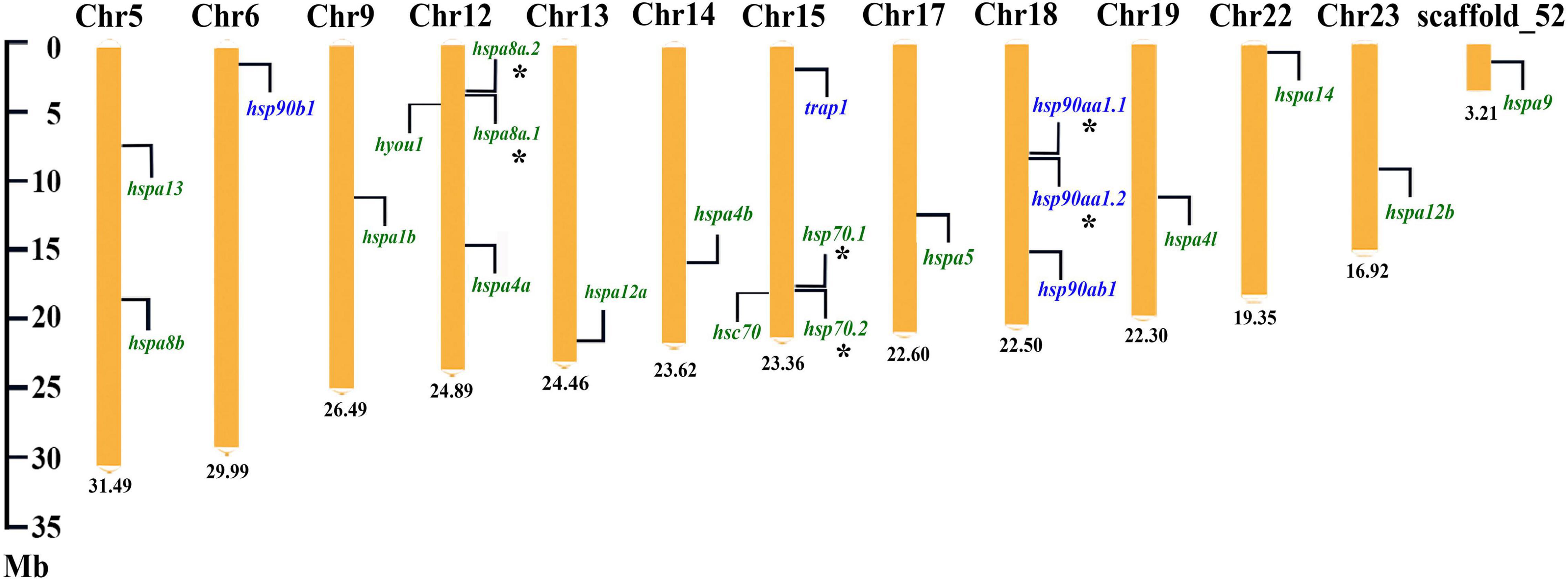
Figure 3. Chromosome location of the HSP90 and HSP70 genes in spotted seabass. The HSP90 and HSP70 genes were indicated by the purple and green fonts, respectively. Asterisks (*) indicated tandem duplicated genes. The number at the bottom represented the length of the chromosomes or scaffold.
Gene Copy Numbers of HSP90 and HSP70 Genes
Copy numbers of the HSP90 and HSP70 genes in representative vertebrates are summarized in Table 3. In general, the number of HSP90 genes was relatively conserved across a broad spectrum of vertebrate species from mammals to fishes, that the total gene numbers of HSP90 were slightly varied from 4 to 5 among different species. Only one copy of each gene was present except for that hsp90aa1. hsp90aa1 was duplicated in several detected teleosts, such as zebrafish, Japanese medaka, fugu, Nile tilapia, and spotted seabass, but a single copy was detected in tetrapods.
Gene copy number of the HSP70 family ranged from 13 to 19 among these detected species. In accordance with the phylogenetic analysis results, hspa6 and hspa7 genes were only discovered in human, and hspa2 was only present in higher vertebrates, such as human, mouse, and chicken. Four HSP70 genes were single-copy in all selected species, such as hspa9, hspa13, hspa14, and hyou1. Multiple gene copies were found in five HSP70 genes of teleosts, such as hspa1, hspa4, hspa5, hspa8, and hspa12. Among them, hspa1 harbored the highest copy numbers in most detected species, and hspa5 and hspa8 were only duplicated in teleost species (Table 3).
Exon-Intron Structure and Motif Analysis
Exon-intron structures and motif analysis were employed to investigate the conservatism and diversity of gene structures in HSP90 and HSP70 genes (Figure 4). The intron numbers of the HSP90 genes varied from 8 to 18. Among them, hsp90aa1.1 and hsp90aa1.2 harbored highly similar exon-intron structures (Figure 4A). Contrastingly, the intron numbers of the HSP70 genes were remarkably varied from 0 to 22 (Figure 4B). According to the number of introns, HSP70 genes could be divided into two modes: mode 1 contained no intron, such as hspa1b, hsp70.1, and hsp70.2, and the rest genes belonged to mode 2, which possessed multiple introns, with intron numbers varied from 4 to 22. In general, the paralogous gene pairs shared similar exon-intron structures (Figure 4B).
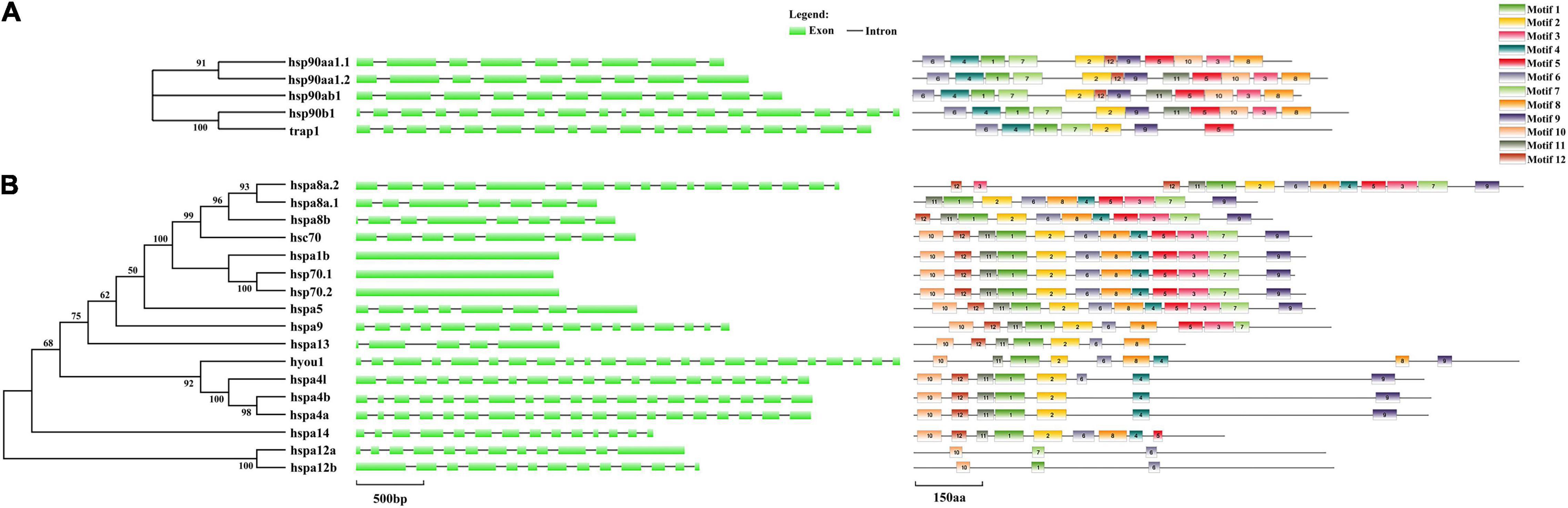
Figure 4. Exon-intron structure and motif analyses of (A) HSP90 and (B) HSP70 in spotted seabass. Exons were shown by green rectangles and introns were indicated by black lines. Lengths of exons and introns are displayed proportionally according to the scale.
For the motif analysis results, the motif numbers among HSP90 genes varied from 7 to 12, whereas all HSP90 members harbored seven conservative motifs, such as motif 1, motif 2, motif 4, motif 5, motif 6, motif 7, and motif 9 (Figure 4A). For the HSP70 family, most genes shared five conservative motifs, namely, motif 1, motif 2, motif 6, motif 10, and motif 11. Among them, hsc70, hspa1b, hsp70.1, hsp70.2, and hspa5 shared highly similar motif pattern, although belonging to two distinct exon-intron structure modes (with or without intron). Moreover, the paralogous gene groups, such as hspa4 genes (hspa4a, hspa4b, and hspa4l) and hspa12 genes (hspa12a and hspa12b), shared conserved motif pattern (Figure 4B). The length of each HSP90 and HSP70 motifs was varied from 21 to 50 and 29 to 50 amino acids, respectively.
Putative Heat Shock Elements and Hypoxia Response Elements in the Promoter Regions of the Heat Shock Protein Genes
As cis-regulatory elements, HSEs and HREs are the master regulators which bind to HSFs and hypoxia-inducible factors (HIFs) to mediate the transcriptional response of their target genes, respectively. Therefore, the distributions of these elements in the promoter regions of HSP90 and HSP70 genes were predicted for spotted seabass. As shown in Figure 5, 28 HSEs and 43 HREs were predicted in the selected promoter regions of HSPs genes. The HSE numbers were various among different HSP genes ranging from 0 to 3. In the 2 kb upstream region from the TSS, three HSEs were detected for hsp90ab1, hspa8a.1, hsp70.1, and hspa4a, two HSEs were identified for hsp90aa1.2, hsp90b1, hspa8b, hspa1b, and hspa12a genes, meanwhile only one HSE was existed in hsp90aa1.1, hsp70.2, hspa5, hspa13, hspa4l, and hspa12b (Figure 5).
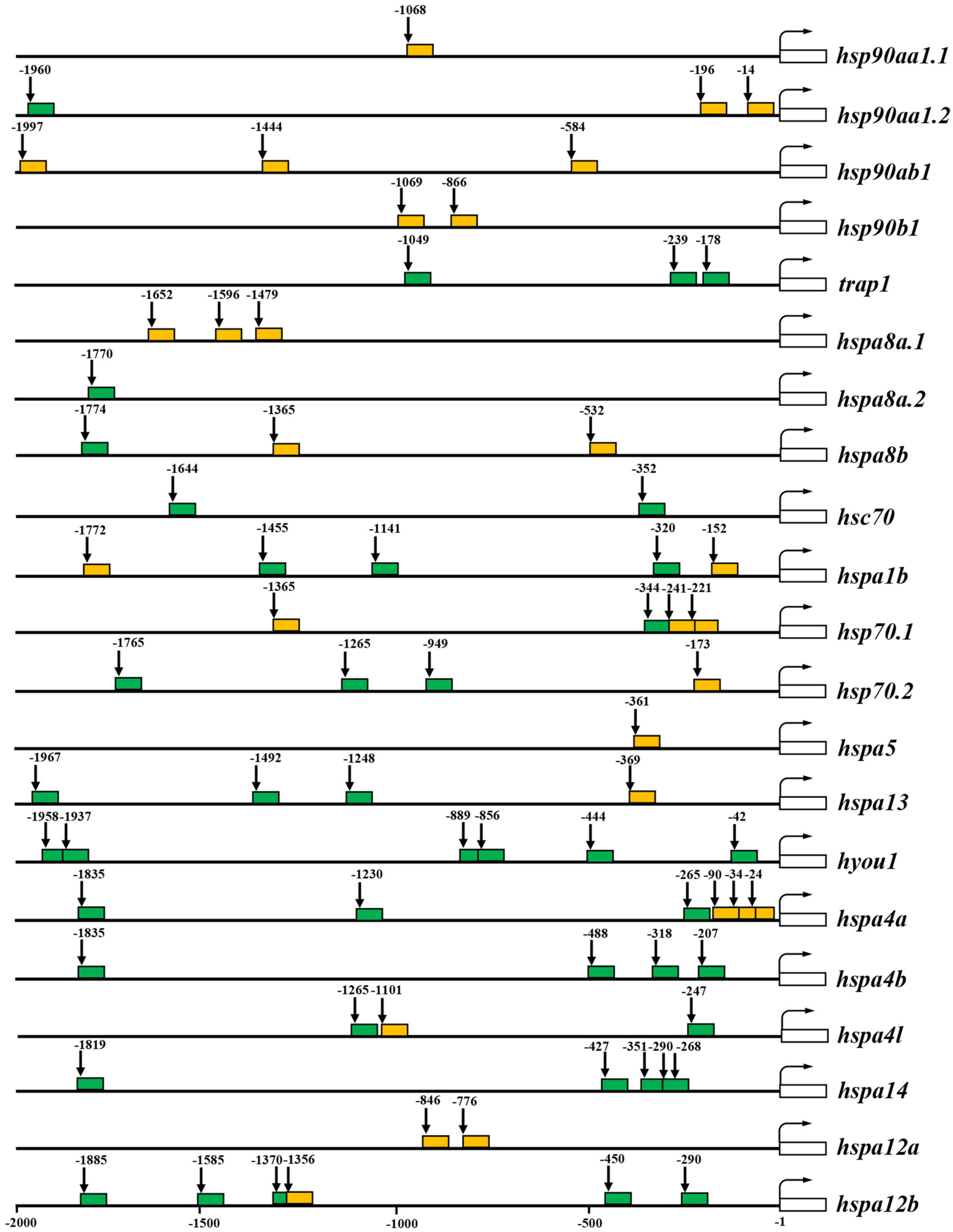
Figure 5. Putative heat shock elements (HSEs) and hypoxia response elements (HREs) in 2 kb upstream regions from the transcription start site (TSS) of HSP90 and HSP70 genes. The predicted HSEs and HREs were shown with orange and green filled boxes, respectively. The numbers indicated spacing (in base pairs) between elements and TSS. The white open box indicates the transcribed sequence. The bent arrow indicates the TSS.
For the prediction results of HREs, 0–6 HRE numbers were discovered in the 2 kb upstream of TSS of target HSP genes. Hyou1 harbored six HREs, meanwhile, hspa14 and hspa12b harbored five HREs. Four HREs were identified in hspa4b, three HREs were detected for trap1, hspa1b, hsp70.2, hspa13, and hspa4a. Hsc70 and hspa4l contained two HREs. Unique HRE position has existed in 2 kb upstream from the TSS of hsp90aa1.2, hspa8a.2, hspa8b, and hsp70.1 (Figure 5).
It can be deduced from the above result that six HSP genes (hsp90aa1.1, hspab1, hsp90b1, hspa5, hspa8a.1, and hspa12a) contained only HSE in their promoter regions, meanwhile six genes (trap1, hspa4b, hspa8a.2, hspa14, hsc70, and hyou1) only possessed HREs. Alternatively, nine genes, namely, hsp90aa1.2, hspa8b, hspa1b, hsp70.1, hsp70.2, hspa13, hspa4a, hspa4l, and hspa12b, harbored both HSE and HRE in their promoter regions (Figure 5).
Protein-Protein Interaction Network Analysis
As the transcription of HSPs is mainly regulated by heat shock factor 1 [HSF1 (Buckley and Hofmann, 2001], the PPI networks of HSPs and HSF1 in spotted seabass were constructed using STRING database based on the orthologs in zebrafish. The results indicated that 22 HSPs of spotted seabass were closely associated with 21 known zebrafish HSPs participated in the interaction network (Supplementary Figure 2 and Supplementary Table 2). As expected, extensive interactions were observed between the HSF1 and HSPs, which was revealed by a direct link representing “transcriptional regulation” in Supplementary Figure 2.
Additionally, HSP70s interacted with HSP70s, and HSP90s also be related to HSP90s, respectively. For example, HSPA4A, HSPA5, and HSPA9 showed a wide range of interactions (reaction, binding, and catalysis) with other HSP70 family proteins. Meanwhile, HSP90AA1.1, HSP90AA1.2, and HSP90AB1 proteins were connected by blue lines, suggesting their “binding” relationship (Supplementary Figure 2). Furthermore, strong interaction relationships were commonly found between HSP70 and HSP90 proteins, for instance, HSP90AA1.1, HSP90AA1.2, and HSP90AB1, were closely connected to HSPA4A, implying that the former may play a negative role in transcriptional regulation of the latter (Supplementary Figure 2).
Expression Patterns of HSP90 and HSP70 Genes in Response to Heat Stress by Quantitative PCR Analysis
The expression patterns of HSP90 and HSP70 genes in gill, liver, and muscle tissues of spotted seabass were systematically examined at 0, 3, 12, and 24 h after heat stress. Results showed that except for trap1 in the muscle, all HSP90 genes were significantly induced after heat stress in tissue- and time-dependent manner (Figure 6A). Among them, hsp90aa1.1 and hsp90aa1.2 exhibited the biggest expression changes in all three detected tissues, with their expression levels remarkably increased to at least 14.8-fold. The highest expression level of hsp90aa1.1 and hsp90aa1.2 appeared at 24 h in the muscle, with a 2,330-fold and 285-fold increase compared with 0 h, respectively. In gill, three genes (hsp90aa1.1, hsp90aa1.2, and hsp90b1) exhibited similar expression patterns after heat stress, which were significantly upregulated at 3 and 12 h and then reduced their expression after 24 h. The results were paralleled with the observation of their expression patterns in the liver (Figure 6A). In contrast, in muscle, the induced expressions of hsp90aa1.1, hsp90aa1.2, and hsp90ab1 genes were appeared for 3 h, maintaining the high expression levels until the end of the challenge experiment. A significant heat stress-responsive expressions were also detected for hsp90ab1 and trap1 in the gill and liver and hsp90b1 in the muscle, with their upregulated expression levels appeared since 12 h after heat stress treatment (Figure 6A).
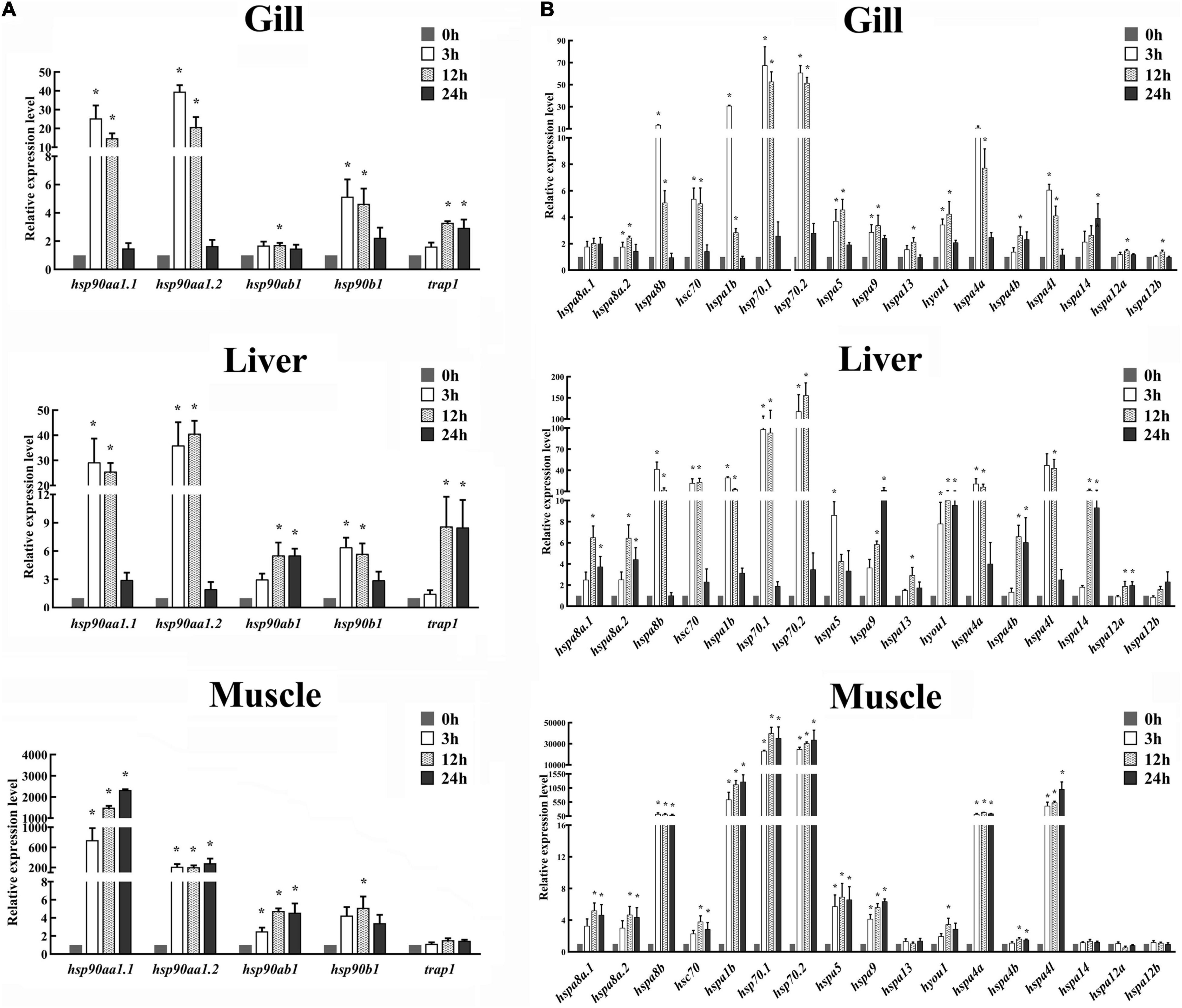
Figure 6. Expression patterns of (A) HSP90 and (B) HSP70 genes in the gill, liver, and muscle of spotted seabass at 0, 3, 12, and 24 h after heat stress. The mRNA expression levels were determined by qPCR analysis. 18S rRNA was used as an internal control. Gene expressions were presented as mean ± SE. Asterisk (*) represented the significant differences (P < 0.05). HSP, heat shock proteins.
Similarity, significant expression variations were commonly identified for several HSP70 genes in three target tissues after heat stress (Figure 6B). In gill tissue, excerpt for hspa8a.1, all HSP70 genes displayed significant differential expression patterns after heat treatment. Among them, highly heat stress-induced expressions in gill were discovered for hspa8b, hspa1b, hsp70.1, hsp70.2, and hspa4a genes, with the maximum expression values exceeded 10-fold compared with those under normal conditions (0 h). Their expression was dramatically induced at the initial tested phase of post-heat stress (3 h) and gradually scaled down during subsequent time points (Figure 6B). In the liver, remarkable upregulations were detected for all HSP70 genes with one exception that the expression change of hspa12b was not statistically significant (Figure 6B). The highest expression variations were discovered for hspa8b, hsc70, hspa1b, hsp70.1, hsp70.2, hspa4l, and hspa4a, which showed similar expression profile after heat stress treatment, that their expressions were dramatically induction at 3 h, remaining highly until 12 h, and then decreased to their normal expression level at 24 h (Figure 6B). The other heat stress-responsive HSP70 genes, namely, hspa8a.1, hspa8a.2, hspa9, hspa4b, and hspa14, exhibited dramatically induced expressions at 12 and 24 h. The expression of hyou1 was highly upregulated at all three tested time points after heat treatment (Figure 6B). For muscle tissues, the expressions of 13 HSP70 genes, such as hspa8a.1, hspa8a.2, hspa8b, hsc70, hspa1b, hsp70.1, hsp70.2, hspa5, hspa9, hyou1, hspa4a, hspa4b, and hspa4l, were significantly increased after heat stress. Among them, the upregulated expression levels of hspa1b, hsp70.1, hsp70.2, and hspa4l were extremely significant, exceeding hundreds of times than their normal expression values. Unlike the expression pattern in gill and liver, most differential expressed HSP70s, such as hspa8b, hspa1b, hsp70.1, hsp70.2, hspa5, hspa9, hspa4a, and hspa4l, displayed upregulation at all time points after heat treatment (Figure 6B). It was worth noting that hsp70.1 and hsp70.2 were the most intense responsive genes in all three tissues after heat stress treatment, suggesting the two genes may play significant roles in response to heat stress in spotted seabass.
Expression Patterns of HSP90 and HSP70 Genes in Response to Alkalinity Challenge and Hypoxia Stress by Examining RNA-Seq Data Sets
As revealed by RNA-Seq analysis, after alkalinity stress, the HSP genes in gills of spotted seabass exhibited dynamic time-dependent expression pattern, and the number of differential expressed genes increased as the alkalinity treating time was prolonged (Figure 7A and Supplementary Table 3). A total of 11 genes, namely, hsp90aa1.2, hsp90b1, trap1, hsp70.2, hspa8a.1, hsc70, hspa13, hspa4a, hspa14, hspa5, and hyou1, showed downregulated expression profile (fold change: − 1.23 to − 3.06, P < 0.05). In detail, downregulated expressions of hspa8a.1 and hspa13 were found since 12 h after alkalinity treatment, a trend that continues until the final experimental time point (72 h). The other genes showed suppressed expression levels starting from the later time points (24 or 72 h). The highest expression changes of HSP genes during the alkalinity challenge experimental period were detected for hspa13, with fold change values nearly double in comparison with 0 h. Conversely, only two HSP genes (hspa12a and hspa12b) showed upregulated expressions after alkalinity stress (P < 0.05).
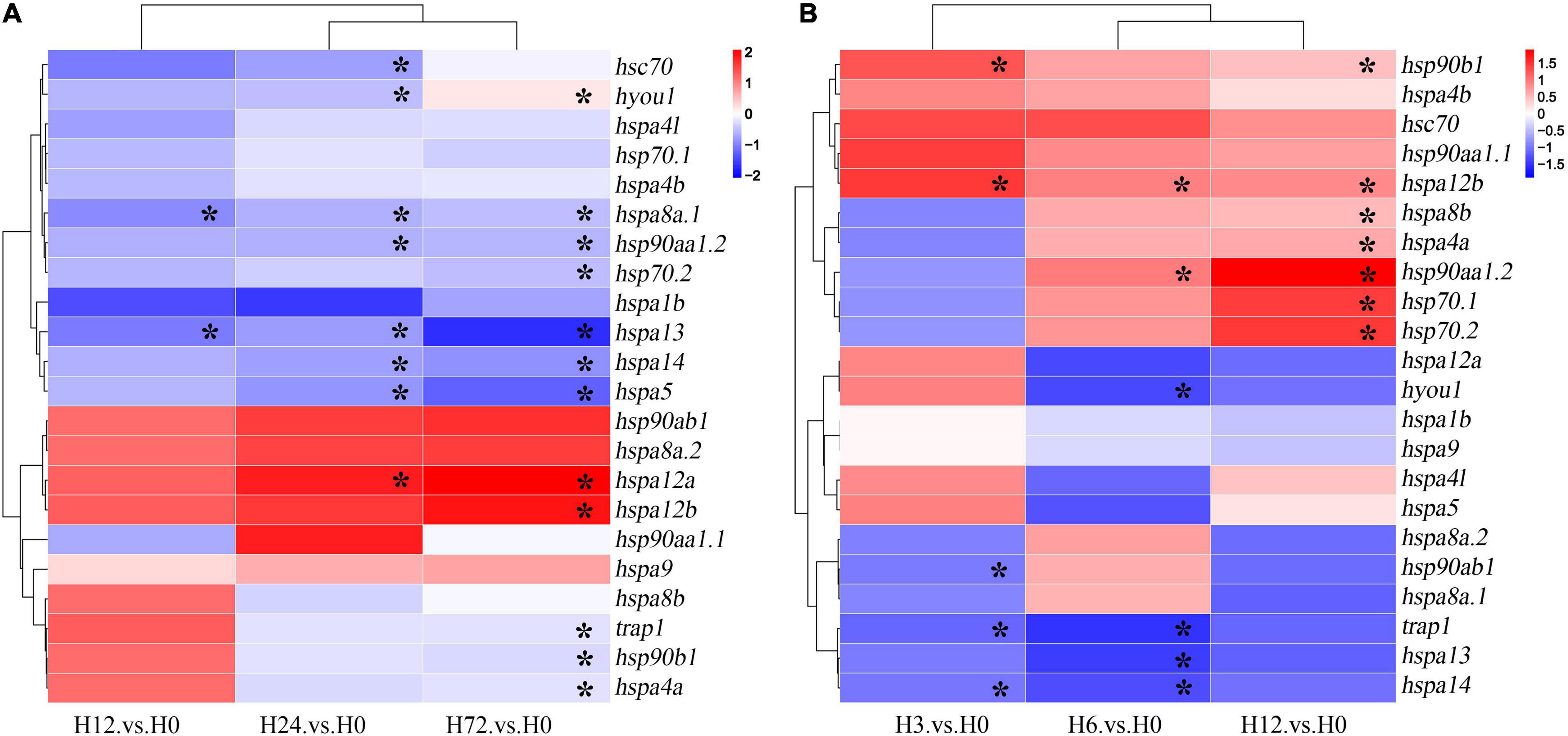
Figure 7. Expression profiles of HSP90 and HSP70 genes in gills of spotted seabass in response to (A) alkalinity and (B) hypoxia stresses. The abundance of mRNA was determined by analyzing the RNA-Seq data. A color scale in the heatmaps represented the fold change values. Asterisk (*) indicated the significant differences (P < 0.05). HSP, heat shock proteins.
After the hypoxia stress, a time-dependent expression profile was also detected for HSP genes in gill, however, the expression trend was distinct from those under alkalinity stress. As shown in Figure 7B and Supplementary Table 4, a total of 12 genes (hsp90aa1.2, hspab1, hsp90b1, trap1, hsp70.1, hsp70.2, hspa8b, hspa13, hspa4a, hspa14, hspa12b, and hyou1) in the gill of spotted seabass were changed significantly after the hypoxia challenge (P < 0.05). In general, five genes, namely, hsp90ab1, trap1, hyou1, hspa13, and hspa14, exhibited downregulated trends (fold change: − 1.23 to − 1.47, P < 0.05), conversely, more genes, such as hsp90aa1.2, hsp90b1, hsp70.1, hsp70.2, hspa8b, hspa4a, and hspa12b, showed remarkably induced expression at 12 h during low-oxygen treatment experiment (fold change: 1.50–3.97, P < 0.05). Among the hypoxia-responsive expressed genes, hsp90aa1.2, hsp70.1, and hsp70.2 had more significant upregulated changes than other HSPs, with expression changes at 12 h exceeding threefold (Figure 7B and Supplementary Table 4).
Discussion
In this study, we performed a systemic analysis of the HSP90 and HSP70 gene families of spotted seabass, revealing the gene features by phylogenic, syntenic, and gene structure analysis and investigated their potential involvement in response to environmental stresses by examining the gene expression patterns. Our results may lay an important basis for further evolutionary and functional studies of HSPs in fish species.
A total of 5 HSP90 (hsp90aa1.1, hsp90aa1.2, hsp90ab1, hsp90b1, and trap1) and 17 HSP70 genes (hspa8a.1, hspa8a.2, hspa8b, hsc70, hspa1b, hsp70.1, hsp70.2, hspa5, hspa9, hspa13, hyou1, hspa4a, hspa4b, hspa4l, hspa14, hspa12a, and hspa12b) were identified in the spotted seabass by searching genome and several transcriptomic databases. The annotation of identified HSP genes was validated by phylogenetic and syntenic analysis, and the evolution and expansion/contraction of HSP90 and HSP70 genes were revealed through the analysis of the phylogenetic relationship and gene copy numbers. These were evidences for the evolutionary conservativeness of HSP90 and HSP70 genes. The HATPase_c and HSPA/HYOU1_(like_) NBD domains were detected in all HSP90 or HSP70 genes of spotted seabass, respectively (Table 2), which was considered to be a classification standard for unknown HSP targets (Mao et al., 2006; Wu et al., 2016). For the HSP90 family, in comparison with tetrapods that each gene harbored the unique copy, duplicated genes of hsp90aa1 existed in several teleosts genomes (Table 3). Based on the conserved linear arrangement of genes between zebrafish and spotted seabass (Figures 2A, 3), we named the two hsp90aa1 copies as hsp90aa1.1 and hsp90aa1.2 accordingly. Their tandem arrangement in genome indicated that the additional gene copy may have arisen through local gene tandem duplication or called small-scale duplication (SSD) events instead of whole-genome duplication (WGD). As in the HSP70 family, hspa8a.1 and hspa8a.2 were tandem located at the same chromosome (Chr12), which may also derive from SSD event (Figures 2B, 3). Alternatively, as shown in the phylogenetic tree of the HSP70 family (Figure 1B), after the divergence between teleosts and tetrapods, hspa4 in teleosts replicates into two independent genes (hspa4a and hspa4b), which was a newer duplication than hspa4l. These three gene copies were situated at different chromosomes, which indicated the paralogs may arise from a teleost-specific WGD event (Figure 3). In contrast, hspa2 did not exist in all selected fishes, while hspa6 and hspa7 were only discovered in human, suggesting that these genes were gained in higher vertebrate during evolution (Xie et al., 2015).
To obtain insight into the structural diversity of the spotted seabass HSP genes, the intron–exon organization was analyzed (Figure 4). The introns numbers of the HSP90 genes varied from 8 to 18, while HSP70 genes harbored introns ranging from 4 to 22 or had no intron (Figure 4B). Previous studies have demonstrated that different exon-intron structures of HSP genes were closely associated with their unique biological functions (Huang et al., 2018; Xu et al., 2018). For example, depending on the intron-exon structure, members of the HSP70 genes have two regulatory patterns at the transcriptional level. Firstly, the constitutively expressed HSPs, usually contain multiple introns, presenting in the cell under all conditions to help folding the newly translated proteins. Secondly, the inducible members of HSP70 genes, typically lacking introns, are significantly expressed under stress conditions (Xu et al., 2018). In spotted seabass, hspa1b, hsp70.1, and hsp70.2 were identified as the most responsive genes in all three tested tissues after heat stress, which was probably due to their intron-less structure (Figures 4, 6B). It has been proved that the intron-less genes might be produced by the loss of multiple introns from the intron-rich gene by retrotransposition during gene family diversification (Wang et al., 2019).
The expression of HSP is mainly regulated by the binding of HSF1 to HSEs in the promoter region of the HSP genes. Under non-stressful conditions, HSF1 exists as an inactive monomer. However, when exposed to heat or other types of cellular stress, HSF1 is converted into trimeric form, binding to promoters of downstream target genes, whereby initiating the transcription (Kmiecik et al., 2020). Previous studies have shown that distance from the TSS to the HSEs may alter the strength of the HSP70 promoter, and closer spacing facilitates more rapid transcription (Tian et al., 2010). In spotted seabass, the HSEs in the promoter regions of hsp90aa1.2, hspa1b, hsp70.1, hsp70.2, and hspa4a were relatively closer to the TSS (Figure 5), which may lead to the strong responsive expression levels after heat stress (Figure 6). On the contrary, no HSE was detected in several genes (Figure 5), indicating that alternative regulatory mechanisms might underlie these genes mediated by HSR (Huang et al., 2018). Besides, the HIF-1 complex has been reported to activate the transcription of some HSP genes containing the HREs when adapting to cellular stress imposed by hypoxia (Huang et al., 2009; Gogate et al., 2012). In spotted seabass, the predicted HRE numbers and distance from the TSS to the HREs were also varied among different HSP genes (Figure 5), which were speculated to be related to their different degree of response to hypoxic stress, and the detailed mechanism need to be investigated further.
To explore the interaction of gene-encoded proteins of the HSP genes, we predicted the PPI network using the deduced protein sequence of HSP90 and HSP70. The results showed that extensive connections existed among the predicted HSP90 and HSP70 family proteins (Supplementary Figure 2), which was in line with the expectation as the two chaperone machines participate together in countless cellular processes (Luengo et al., 2019).
Although the previous study has reported the expressions of one HSP90 and one HSP70 gene in spotted seabass during thermal stress experiment (Shin et al., 2018), the distinguish and participation of the full gene sets of the two gene families in response to heat stress in this species has not been systematically analyzed. In our study, the heat stress-responsive expression changes of HSP90 and HSP70 gene family in gill, liver, and muscle, which was reported as primary tissues responsible for heat stress in teleost (Giri et al., 2014; Purohit et al., 2014; Shi et al., 2015; Peng et al., 2016; Shin et al., 2018; Xu et al., 2018), have been investigated thoroughly. As the results showed, hsp90aa1.1 and hsp90aa1.2 in the HSP90 family and hspa8b, hsc70, hspa1b, hsp70.1, hsp70.2, hspa4a, and hsp4l in the HSP70 family displayed remarkable heat-inducible expression patterns in two or three tested tissues of spotted seabass (Figure 6B). Although only HSP70 family were examined, in line with our findings, five HSP70 genes (hspa4a, hsc70, hspa5.1, hspa8b, and hsp70) were significantly upregulated in liver of large yellow croaker under heat treatment, and hsp70 and hspa8b were the most inducible genes which were considered as the strictly heat-inducible HSP70 genes (Xu et al., 2018). As we stated above, the strong induction of these genes during heat shock may be generated due to the lack of introns allows efficient transcription (e.g., hspa1b, hsp70.1, and hsp70.2), or the close distance from the HSEs to the TSS facilitates rapid transcription (e.g., hsp90aa1.2, hspa1b, hsp70.1, hsp70.2, and hspa4a).
In addition, we examined the potential involvement of HSP90 and HSP70 genes of spotted seabass in response to other two important abiotic stressors, alkalinity and hypoxia, by analyzing the transcriptomic datasets. In contrast to the overall highly inducible expression of HSP genes after heat stress, a significant portion of genes exhibited significantly suppressed expression levels under alkalinity and/or hypoxia challenge (Figure 7). For example, compared with the dramatic upregulated expressions of hsp70.1, hsp70.2, and hspa1b under heat stress, mild downregulated expression of hsp70.2 was found at 72 h in gills after alkalinity treatment (Figure 7A, P < 0.05). Besides, hsp70.1 and hspa1b exhibited a downregulated expression trend under alkalinity treatment, in spite of being not statistically significant (Figure 7A). The distinct expression patterns indicated that different responding mechanisms might underlie these HSP genes mediated by specific environmental stressors. Similar results have been also reported for Litopenaeus vannamei, that by testing the response sensitivity and intensity of HSPs to different environmental stresses, hsp70 gene was considered as the biomarker indicating thermal stress, but not suitable for acting as an indicator of pH stress (Qian et al., 2012). The downregulated expression of HSP70 genes has also been reported in the brain of the large yellow croaker under hypoxia stress (Ao et al., 2015), and in the gills of Boleophthalmus pectinirostris under the oxidative stress caused by high environmental ammonia (Deng et al., 2019). Moreover, the significant upregulated expressions of hsp90aa1.2, hsp70.1 and hsp70.2 after hypoxic challenge were identified in spotted seabass (Figure 7B), and similar expression profiles have also been reported for Indian Catfish (Clarias batrachus) and Amur sturgeon (Acipenser schrenckii) (Ni et al., 2014; Mohindra et al., 2015). Although the mechanism underlying the stress-regulated expression levels of HSP genes has not yet been fully elucidated, the HSPs with differential expression patterns may play their respective roles in protection and survival under stress conditions.
Conclusion
In this study, the entire HSP90 and HSP70 gene families were systematically characterized in spotted seabass. Phylogenetic, syntenic, and gene copy numbers analyses provided sufficient evidences for the annotation and evolutionary footprint of these genes. Gene structures and cis-regulatory elements including HSEs and HREs were further characterized, which not only showed that genes with closer homology relationships had similar structures and conserved motifs, but also give a hint to explain the significant stress-regulated expression patterns of several HSP genes. Moreover, the potential involvement of HSPs in response to heat stress was determined by the qPCR experiment and dynamic time-dependent expression pattern after alkalinity and hypoxic challenge were revealed through the analysis of RNA-Seq data. Our findings provided a comprehensive overview of HSP90 and HSP70 families in spotted seabass and laid the basis for a better understanding of the physiological function of HSP90 and HSP70 genes in response to abiotic stress.
Data Availability Statement
The original contributions presented in the study are included in the article/Supplementary Material, further inquiries can be directed to the corresponding author/s.
Ethics Statement
The animal study was reviewed and approved by Animal Research and Ethics Committee of Ocean University of China (Permit Number: 20141201).
Author Contributions
YS, YT, JuL, XM, and YH performed bioinformatics analysis. YuL and JiL provided funding support. YuL and YS conceived the study. YS, YaL, XL, and XM performed stress experiments. HW and YuL administrated the project. All authors read and approved the submitted version.
Funding
This study was supported by the National Key R&D Program of China (2020YFD0900204), the National Natural Science Foundation of China (NSFC, 32072947), and the China Agriculture Research System of MOF and MARA (CARS-47).
Conflict of Interest
The authors declare that the research was conducted in the absence of any commercial or financial relationships that could be construed as a potential conflict of interest.
Publisher’s Note
All claims expressed in this article are solely those of the authors and do not necessarily represent those of their affiliated organizations, or those of the publisher, the editors and the reviewers. Any product that may be evaluated in this article, or claim that may be made by its manufacturer, is not guaranteed or endorsed by the publisher.
Supplementary Material
The Supplementary Material for this article can be found online at: https://www.frontiersin.org/articles/10.3389/fphys.2021.784803/full#supplementary-material
Supplementary Figure 1 | Schematic diagram of heat stress experiment. Spotted seabass individuals were acclimated for 48 h at ambient temperature (25oC) before heat stress treatment. Water temperature was elevated at a constant rate of 1oC/h until 32°C. Fish individuals were sampled at four time points including 0 h (H0), 10 h (3 h after heat stress, H3), 19 h (12 h after heat stress, H12) and 31 h (24 h after heat stress, H24), respectively.
Supplementary Figure 2 | Predicted protein-protein interaction network of HSF1 and HSPs in spotted seabass. This network was predicted by the online STRING database. The balls represented the gene nodes, and the connecting lines and arrowheads indicated the interactions. HSP, heat shock proteins; HSF, heat shock factor.
Footnotes
- ^ http://www.ncbi.nlm.nih.gov/
- ^ https://www.ncbi.nlm.nih.gov/gorf/gorf.html
- ^ http://www.ensembl.org
- ^ http://itol.embl.de/
- ^ https://www.genomicus.bio.ens.psl.eu/genomicus-100.01/cgi-bin/search.pl
- ^ http://www.expasy.ch/tools/protparam.html
- ^ http://smart.embl.de/
- ^ https://www.ncbi.nlm.nih.gov/Structure/cdd/wrpsb.cgi
- ^ http://gsds.cbi.pku.edu.cn
- ^ http://meme.nbcr.net/meme/
- ^ http://jaspar.genereg.net/
- ^ https://string-db.org/
References
Ao, J., Mu, Y., Xiang, L.-X., Fan, D., Feng, M., Zhang, S., et al. (2015). Genome sequencing of the perciform fish Larimichthys crocea provides insights into molecular and genetic mechanisms of stress adaptation. PLoS Genet. 11:e1005118. doi: 10.1371/journal.pgen.1005118
Brocchieri, L., Conway de Macario, E., and Macario, A. J. (2008). hsp70 genes in the human genome: conservation and differentiation patterns predict a wide array of overlapping and specialized functions. BMC Evol. Biol. 8:19. doi: 10.1186/1471-2148-8-19
Buckley, B. A., and Hofmann, G. E. (2001). Thermal acclimation changes DNA-binding activity of heat shock factor 1(HSF1) in the goby Gillichthys mirabilis: implications for plasticity in the heat-shock response in natural populations. J. Exp. Biol. 205, 3231–3240. doi: 10.1242/jeb.205.20.3231
Bukau, B., Weissman, J., and Horwich, A. (2006). Molecular chaperones and protein quality control. Cell 125, 443–451. doi: 10.1016/j.cell.2006.04.014
Chen, B., Piel, W. H., Gui, L., Bruford, E., and Monteiro, A. (2005). The HSP90 family of genes in the human genome: insights into their divergence and evolution. Genomics 86, 627–637. doi: 10.1016/j.ygeno.2005.08.012
Deng, Z., Sun, S., Gao, T., and Han, Z. (2019). The Hsp70 gene family in Boleophthalmus pectinirostris: genome-wide identification and expression analysis under high ammonia stress. Animals 9:36. doi: 10.3390/ani9020036
Doyle, S. M., Hoskins, J. R., Kravats, A. N., Heffner, A. L., Garikapati, S., and Wickner, S. (2019). Intermolecular Interactions between Hsp90 and Hsp70. J. Mol. Cell Biol. 431, 2729–2746. doi: 10.1016/j.jmb.2019.05.026
Feder, M. E., and Hofmann, G. E. (1999). Heat-shock proteins, molecular chaperones, and the stress response: evolutionary and ecological physiology. Annu. Rev. Physiol. 61, 243–282. doi: 10.1146/annurev.physiol.61.1.243
Giri, S. S., Sen, S. S., and Sukumaran, V. (2014). Role of HSP70 in cytoplasm protection against thermal stress in rohu, Labeo rohita. Fish Shellfish Immunol. 41, 294–299. doi: 10.1016/j.fsi.2014.09.013
Gogate, S. S., Fujita, N., Skubutyte, R., Shapiro, I. M., and Risbud, M. V. (2012). TonEBP and HIF coordinate Hsp70 expression in hypoxic nucleus pulposus cells: role of Hsp70 in HIF-1α degradation. J. Bone Miner. Res. 27, 1106–1117. doi: 10.1002/jbmr.1571
Gupta, A., Bansal, A., and Hashimoto-Torii, K. (2020). HSP70 and HSP90 in neurodegenerative diseases. Neurosci. Lett. 716:134678. doi: 10.1016/j.neulet.2019.134678
Hangzo, H., Banerjee, B., Saha, S., and Saha, N. (2016). Ammonia stress under high environmental ammonia induces Hsp70 and Hsp90 in the mud eel, Monopterus cuchia. Fish Physiol. Biochem. 43, 77–88. doi: 10.1007/s10695-016-0269-4
Huang, W. J., Xia, L. M., Zhu, F., Huang, B., Zhou, C., Zhu, H. F., et al. (2009). Transcriptional upregulation of HSP70-2 by HIF-1 in cancer cells in response to hypoxia. Int. J. Oncol. 124, 298–305. doi: 10.1002/ijc.23906
Huang, X., Li, S., Gao, Y., and Zhan, A. (2018). Genome-Wide identification, characterization and expression analyses of heat shock protein-related genes in a highly invasive Ascidian Ciona savignyi. Front. Physiol. 9:1043. doi: 10.3389/fphys.2018.01043
Kmiecik, S. W., Breton, L. L., and Mayer, M. P. (2020). Feedback regulation of heat shock factor 1 (Hsf1) activity by Hsp70-mediated trimer unzipping and dissociation from DNA. EMBO J. 39:e104096. doi: 10.15252/embj.2019104096
Li, B., Tian, Y., Wen, H., Qi, X., Wang, L., Zhang, J., et al. (2021). Systematic identification and expression analysis of the Sox gene family in spotted sea bass (Lateolabrax maculatus). Comp. Biochem. Physiol. Part D Genomics Proteomics 38:100817. doi: 10.1016/j.cbd.2021.100817
Liu, Y., Wang, H., Wen, H., Shi, Y., Zhang, M., Qi, X., et al. (2020). First high-density linkage map and QTL fine mapping for growth-related traits of spotted sea bass (Lateolabrax maculatus). Mar. Biotechnol. 22, 526–538. doi: 10.1007/s10126-020-09973-4
Luengo, T. M., Mayer, M. P., and Rüdiger, S. G. (2019). The Hsp70–Hsp90 chaperone cascade in protein folding. Trends Cell Biol. 29, 164–177. doi: 10.1016/j.tcb.2018.10.004
Ma, F., and Luo, L. (2020). Genome-wide identification of Hsp70/110 genes in rainbow trout and their regulated expression in response to heat stress. PeerJ 8:e10022. doi: 10.7717/peerj.10022
Ma, F., Liu, Z., Kang, Y., and Quan, J. (2020). Genome-Wide identification of hsp90 gene in rainbow trout (Oncorhynchus mykiss) and their regulated expression in response to heat stress. DNA Cell Biol. 39, 428–440. doi: 10.1089/dna.2019.4936
Mao, Y., Deng, A., Qu, N., and Wu, X. (2006). ATPase domain of Hsp70 exhibits intrinsic ATP-ADP exchange activity. Biochemistry (moscow) 71, 1222–1229. doi: 10.1134/s0006297906110071
Mohindra, V., Tripathi, R. K., Yadav, P., Singh, R. K., and Lal, K. K. (2015). Hypoxia induced altered expression of heat shock protein genes (Hsc71, Hsp90α and Hsp10) in Indian Catfish, Clarias batrachus (Linnaeus, 1758) under oxidative stress. Mol. Biol. Rep. 42, 1197–1209. doi: 10.1007/s11033-015-3855-0
Ni, M., Wen, H., Li, J., Chi, M., Ren, Y., Song, Z., et al. (2014). Two HSPs gene from juvenile Amur sturgeon (Acipenser schrenckii): cloning, characterization and expression pattern to crowding and hypoxia stress. Fish Physiol. Biochem. 40, 1801–1816. doi: 10.1007/s10695-014-9969-9
Peng, G., Zhao, W., Shi, Z., Chen, H., Liu, Y., Wei, J., et al. (2016). Cloning HSP70 and HSP90 genes of kaluga (Huso dauricus) and the effects of temperature and salinity stress on their gene expression. Cell Stress Chaperones 21, 349–359. doi: 10.1007/s12192-015-0665-1
Prodromou, C., Roe, S. M., O’Brien, R., Ladbury, J. E., Piper, P. W., and Pearl, L. H. (1997). Identification and structural characterization of the ATP/ADP-binding site in the Hsp90 molecular chaperone. Cell 90, 65–75. doi: 10.1016/s0092-8674(00)80314-1
Purohit, G. K., Mahanty, A., Suar, M., Sharma, A. P., Mohanty, B. P., and Mohanty, S. (2014). Investigating hsp gene expression in liver of Channa striatus under heat stress for understanding the upper thermal acclimation. BioMed Res. Int. 2014:381719. doi: 10.1155/2014/381719
Qian, Z., Liu, X., Wang, L., Wang, X., Li, Y., Xiang, J., et al. (2012). Gene expression profiles of four heat shock proteins in response to different acute stresses in shrimp, Litopenaeus vannamei. Comp. Biochem. Physiol. C Toxicol. Pharmacol. 156, 211–220. doi: 10.1016/j.cbpc.2012.06.001
Ritossa, F. (1962). A new pattern induced by a temperature shock and DNP in Drosophila. Experientia 18, 571–573. doi: 10.1007/bf02172188
Schlecht, R., Erbse, A. H., Bukau, B., and Mayer, M. P. (2011). Mechanics of Hsp70 chaperones enables differential interaction with client proteins. Nat. Struct. Mol. Biol. Evol 18, 345–351. doi: 10.1038/nsmb.2006
Shi, H. N., Liu, Z., Zhang, J. P., Kang, Y. J., Wang, J. F., Huang, J. Q., et al. (2015). Short communication: effect of heat stress on heat-shock protein (Hsp60) mRNA expression in rainbow trout Oncorhynchus mykiss. Genet. Mol. Res. 14, 5280–5286. doi: 10.4238/2015.May.18.20
Shi, Q., Yu, C., Zhu, D., Li, S., and Wen, X. (2020). Effects of dietary Sargassum horneri on resisting hypoxia stress, which changes blood biochemistry, antioxidant status, and hepatic HSP mRNA expressions of juvenile black sea bream Acanthopagrus schlegelii. J. Appl. Phycol. 32, 3457–3466. doi: 10.1007/s10811-020-02132-1
Shin, M.-K., Park, H.-R., Yeo, W.-J., and Han, K.-N. (2018). Effects of thermal stress on the mRNA expression of SOD, HSP90, and HSP70 in the spotted sea bass (Lateolabrax maculatus). Ocean Sci. J. 53, 43–52. doi: 10.1007/s12601-018-0001-7
Sib Sankar, G., Shib Sankar, S., and Sukumaran, V. (2014). Role of HSP70 in cytoplasm protection against thermal stress in rohu, Labeo rohita. Fish Shellfish Immunol. 41, 294–299.
Song, L., Li, C., Xie, Y., Liu, S., Zhang, J., Yao, J., et al. (2014). Genome-wide identification of Hsp70 genes in channel catfish and their regulated expression after bacterial infection. Fish Shellfish Immunol. 9, 154–162. doi: 10.1016/j.fsi.2015.12.009
Street, T., Lavery, L., and Agard, D. (2011). Substrate binding drives large-scale conformational changes in the Hsp90 molecular chaperone. Mol. Cell. 42, 96–105. doi: 10.1016/j.molcel.2011.01.029
Tian, S., Haney, R. A., and Feder, M. E. (2010). Phylogeny disambiguates the evolution of heat-shock cis-regulatory elements in Drosophila. PLoS One 5:e10669. doi: 10.1371/journal.pone.0010669
Tissiéres, A., Mitchell, H. K., and Tracy, U. M. (1974). Protein synthesis in salivary glands of Drosophila melanogaster: relation to chromosome puffs. J. Mol. Cell Biol. 84, 389–398. doi: 10.1016/0022-2836(74)90447-1
Umam, K., Tang, C.-H., and Lee, T.-H. (2016). The roles of heat shock protein 70 (HSP70) and heat shock protein 90 (HSP90) in osmoregulatory and metabolic tissues of milkfish (Chanos chanos) under osmotic stress. J. Aqua. Asia 2, 30–37.
Wang, H., Wen, H., Li, Y., Zhang, K., and Liu, Y. (2018). Evaluation of potential reference genes for quantitative RT-PCR analysis in spotted sea bass (Lateolabrax maculatus) under normal and salinity stress conditions. PeerJ 6:e5631. doi: 10.7717/peerj.5631
Wang, Y., Zhang, J., Hu, Z., Guo, X., Tian, S., and Chen, G. (2019). Genome-wide analysis of the MADS-box transcription factor family in Solanum lycopersicum. Int. J. Mol. Sci. 20:2961. doi: 10.3390/ijms20122961
Wegele, H., Wandinger, S. K., Schmid, A. B., Reinstein, J., and Buchner, J. (2006). Substrate transfer from the chaperone Hsp70 to Hsp90. J. Mol. Cell Biol. 356, 802–811. doi: 10.1016/j.jmb.2005.12.008
Wu, M., Li, Y., Chen, D., Liu, H., Zhu, D., and Xiang, Y. (2016). Genome-wide identification and expression analysis of the IQD gene family in moso bamboo (Phyllostachys edulis). Sci. Rep. 6:24520. doi: 10.1038/srep24520
Xie, Y., Song, L., Weng, Z., Liu, S., and Liu, Z. (2015). Hsp90, Hsp60 and sHsp families of heat shock protein genes in channel catfish and their expression after bacterial infections. Fish Shellfish Immunol. 44, 642–651. doi: 10.1016/j.fsi.2015.03.027
Xu, K., Xu, H., and Han, Z. (2018). Genome-Wide identification of Hsp70 genes in the large yellow croaker (Larimichthys crocea) and their regulated expression under cold and heat stress. Genes 9:590. doi: 10.3390/genes9120590
Yu, P., Zhou, Y., Qi, X., Fan, H., Zhang, K., Zhang, X., et al. (2020). Identification, expression analysis, and functional characterization of ghrelin and its receptors in spotted sea bass (Lateolabrax maculatus). J. Mar. Sci. Technol. 2, 349–359. doi: 10.1007/s42995-020-00055-x
Keywords: HSP90, HSP70, spotted seabass, environmental stresses, gene expression, transcriptome
Citation: Sun Y, Wen H, Tian Y, Mao X, Li X, Li J, Hu Y, Liu Y, Li J and Li Y (2021) HSP90 and HSP70 Families in Lateolabrax maculatus: Genome-Wide Identification, Molecular Characterization, and Expression Profiles in Response to Various Environmental Stressors. Front. Physiol. 12:784803. doi: 10.3389/fphys.2021.784803
Received: 28 September 2021; Accepted: 25 October 2021;
Published: 22 November 2021.
Edited by:
Lingling Wang, Dalian Ocean University, ChinaReviewed by:
Xiaodan Wang, East China Normal University, ChinaTing Chen, South China Sea Institute of Oceanology, Chinese Academy of Sciences (CAS), China
Copyright © 2021 Sun, Wen, Tian, Mao, Li, Li, Hu, Liu, Li and Li. This is an open-access article distributed under the terms of the Creative Commons Attribution License (CC BY). The use, distribution or reproduction in other forums is permitted, provided the original author(s) and the copyright owner(s) are credited and that the original publication in this journal is cited, in accordance with accepted academic practice. No use, distribution or reproduction is permitted which does not comply with these terms.
*Correspondence: Yun Li, eXVubGkwMTE2QG91Yy5lZHUuY24=