- Department of Pharmacology and Toxicology, College of Osteopathic Medicine, Michigan State University, East Lansing, MI, United States
Arterioles in the peripheral microcirculation regulate blood flow to and within tissues and organs, control capillary blood pressure and microvascular fluid exchange, govern peripheral vascular resistance, and contribute to the regulation of blood pressure. These important microvessels display pressure-dependent myogenic tone, the steady state level of contractile activity of vascular smooth muscle cells (VSMCs) that sets resting arteriolar internal diameter such that arterioles can both dilate and constrict to meet the blood flow and pressure needs of the tissues and organs that they perfuse. This perspective will focus on the Ca2+-dependent ion channels in the plasma and endoplasmic reticulum membranes of arteriolar VSMCs and endothelial cells (ECs) that regulate arteriolar tone. In VSMCs, Ca2+-dependent negative feedback regulation of myogenic tone is mediated by Ca2+-activated K+ (BKCa) channels and also Ca2+-dependent inactivation of voltage-gated Ca2+ channels (VGCC). Transient receptor potential subfamily M, member 4 channels (TRPM4); Ca2+-activated Cl− channels (CaCCs; TMEM16A/ANO1), Ca2+-dependent inhibition of voltage-gated K+ (KV) and ATP-sensitive K+ (KATP) channels; and Ca2+-induced-Ca2+ release through inositol 1,4,5-trisphosphate receptors (IP3Rs) participate in Ca2+-dependent positive-feedback regulation of myogenic tone. Calcium release from VSMC ryanodine receptors (RyRs) provide negative-feedback through Ca2+-spark-mediated control of BKCa channel activity, or positive-feedback regulation in cooperation with IP3Rs or CaCCs. In some arterioles, VSMC RyRs are silent. In ECs, transient receptor potential vanilloid subfamily, member 4 (TRPV4) channels produce Ca2+ sparklets that activate IP3Rs and intermediate and small conductance Ca2+ activated K+ (IKCa and sKCa) channels causing membrane hyperpolarization that is conducted to overlying VSMCs producing endothelium-dependent hyperpolarization and vasodilation. Endothelial IP3Rs produce Ca2+ pulsars, Ca2+ wavelets, Ca2+ waves and increased global Ca2+ levels activating EC sKCa and IKCa channels and causing Ca2+-dependent production of endothelial vasodilator autacoids such as NO, prostaglandin I2 and epoxides of arachidonic acid that mediate negative-feedback regulation of myogenic tone. Thus, Ca2+-dependent ion channels importantly contribute to many aspects of the regulation of myogenic tone in arterioles in the microcirculation.
Introduction
Arterioles are prominent resistance vessels that regulate blood flow to and within tissues and organs; determine capillary blood pressure and fluid exchange in the microcirculation; and contribute to the regulation of systemic blood pressure (Renkin, 1984). A defining characteristic of arterioles is pressure-dependent myogenic tone, the steady state vascular smooth muscle cell (VSMC) contractile activity that is induced and maintained by pressure-dependent mechanisms (Jackson, 2020, 2021). Myogenic tone sets resting arteriolar internal diameter such that these microvessels can dilate or constrict to maintain homeostasis by meeting the blood flow and pressure needs of the tissues and organs that they perfuse.
Arterioles express numerous ion channels that are essential to their function (Figure 1). Plasma membrane and endoplasmic reticulum (ER) ion channels in VSMCs are a major source of Ca2+ triggering contractile machinery activation and increased arteriolar tone (vasoconstriction). In endothelial cells (ECs), ion channels provide a key Ca2+source controlling EC autacoid production including prostacyclin (PGI2), nitric oxide (NO) and epoxides of arachidonic acid (EETs; Jackson, 2016). Intracellular Ca2+ also controls gene expression and cell proliferation in VSMCs (Cartin et al., 2000; Stevenson et al., 2001; Barlow et al., 2006) and in ECs (Quinlan et al., 1999; Nilius and Droogmans, 2001; Munaron, 2006; Minami, 2014). Ion channels play a major role in cell volume regulation in all cells (Hoffmann et al., 2009). Finally, ion channels help set and modulate VSMC and EC membrane potential (Jackson, 2016, 2020, 2021; Tykocki et al., 2017). Membrane potential, in turn, regulates the open state probability of voltage-gated Ca2+ channels (VGCCs) which provide a major source of activator Ca2+ in VSMCs (Tykocki et al., 2017), but probably not most ECs (Jackson, 2016). The electrochemical gradient for diffusion of Ca2+ and other ions depends on membrane potential in all cells (Tykocki et al., 2017). Membrane potential also has been proposed to affect Ca2+ release from ER Ca2+ stores and may influence the Ca2+ sensitivity of Ca2+-dependent processes [(see Tykocki et al., 2017) for references]. Lastly, membrane potential serves as an essential signal for cell–cell communication, because VSMCs and ECs express both homocellular and heterocellular gap junctions allowing electrical and chemical communication among cells in the arteriolar wall (de Wit and Griffith, 2010; Bagher and Segal, 2011; Dora and Garland, 2013; Garland and Dora, 2017; Schmidt and de Wit, 2020). Thus, arteriolar function critically depends on ion channels.
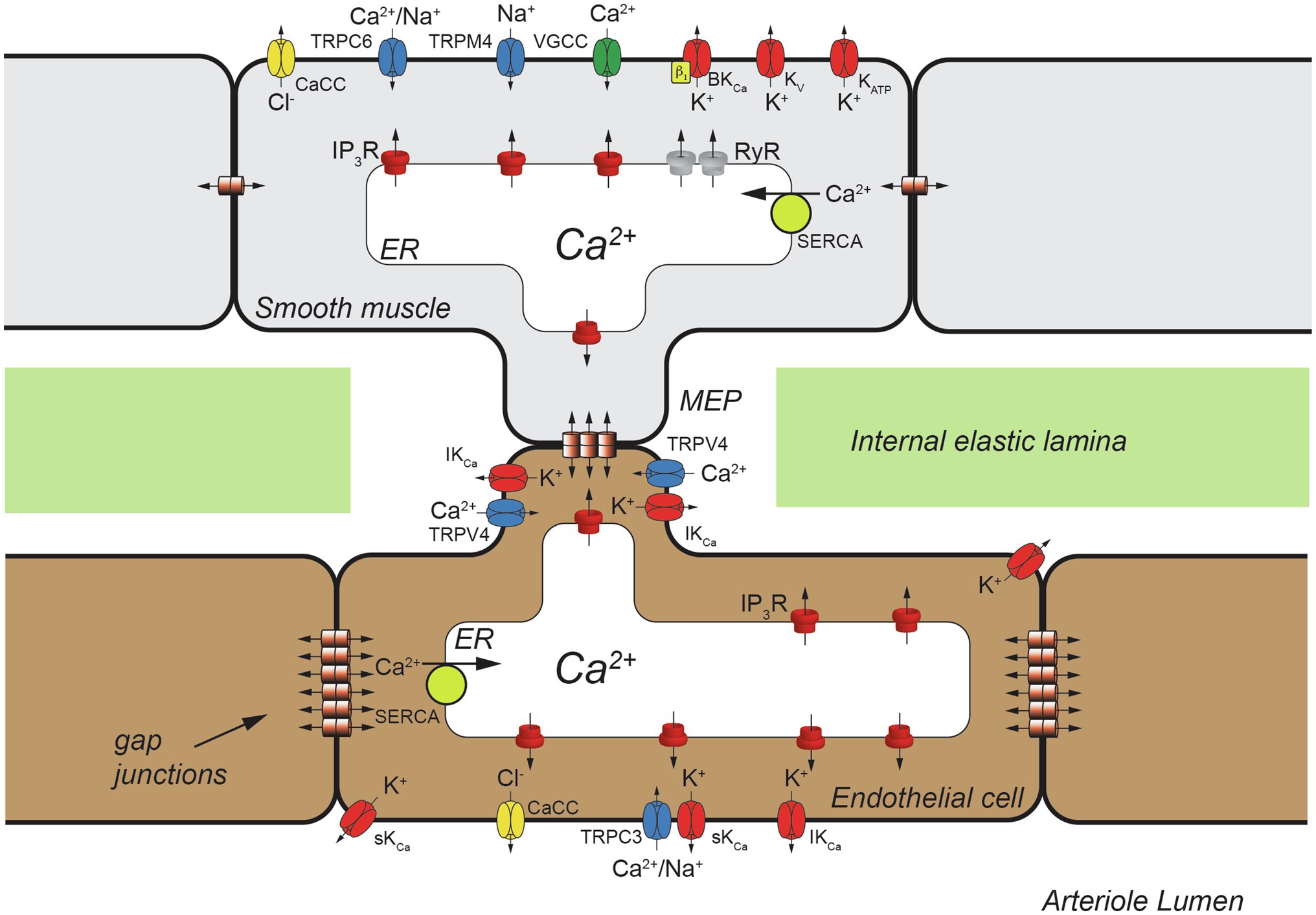
Figure 1. Schematic representation of a cross section of one wall of an arteriole showing a myoendothelial projection (MEP) passing through a hole in the internal elastic lamina (IEL). Heterocellular gap junctions are present allowing electrical and chemical (Ca2+, IP3, etc.) communication between ECs and VSMCs. Also shown are homocellular (EC-EC and VSMC-VSMC) gap junctions that also allow electrical and chemical communication as shown. Only a few classes of ion channels expressed by arteriolar VSMCs and ECs are shown for clarity. TRPC6, transient receptor potential channel C family member 6; CaCC, Ca2+-activated Cl− channels; TRPM4, transient receptor potential channel melanostatin family member 4; VGCC, voltage-gated Ca2+ channels, BKCa, large-conductance Ca2+-activated K+ channels; KV, voltage-gated K+ channels; KATP, ATP-sensitive K+ channels; IP3R, inositol 1,4,5 trisphosphate receptor; RyR, ryanodine receptor; SERCA, smooth endoplasmic reticulum Ca2+ ATPase; IKCa, intermediate-conductance Ca2+-activated K+ channel; TRPV4, Transient Receptor Potential Vanilloid-family 4 channels; TRPC3, transient receptor potential channel C family member 3; sKCa, small-conductance Ca2+-activated K+ channel.
Calcium-dependent ion channels in both VSMCs and ECs play a central role in the generation and modulation of myogenic tone and maintenance of homeostasis (Figure 1). These channels provide both positive- and negative-feedback control of intracellular Ca2+ in VSMCs that allows fine tuning of arteriolar tone as will be outlined in Section VSMC Ca2+-Dependent Ion Channels, below.
The arteriolar endothelium provides negative-feedback signals to overlying VSMCs through Ca2+-dependent autacoid production and direct electrical communication via myoendothelial gap junctions (MEGJs; Lemmey et al., 2020). Endothelial Ca2+-dependent ion channels contribute to these processes (Figure 1) as outlined in Section Endothelial Ca2+-Dependent Ion Channels and Arteriolar Tone, below.
Section Integration of Ca2+-Dependent Ion Channels Into the Mechanisms Underlying Pressure-Induced Myogenic Tone then will integrate the VSMC and EC Ca2+-dependent ion channels into the mechanisms that establish, maintain, and modulate pressure-dependent myogenic tone in resistance arteries and arterioles.
Vsmc Ca2+-Dependent Ion Channels
Arteriolar VSMCs express at least six different Ca2+-dependent ion channels (Tykocki et al., 2017) that participate in the generation, maintenance and modulation of myogenic tone. Large-conductance Ca2+-activated K+ (BKCa) channels provide negative-feedback regulation of myogenic tone. Ryanodine receptors (RyRs) can be both inhibitory (negative feedback) or excitatory (positive feedback) dependent on where in the ER they are expressed and with which ion channels they interact. Inositol 1,4,5-trisphosphate receptors (IP3Rs), transient-receptor potential melanostatin member 4 (TRPM4) channels, Ca2+-activated Cl− channels (CaCCs) and transient receptor potential polycystin-family member 1 [TRPP1 (PKD2)] channels are excitatory and contribute to the positive-feedback regulation of myogenic tone. In addition, VGCCs (Shah et al., 2006), voltage-gated K+ (KV) channels (Gelband et al., 1993; Ishikawa et al., 1993; Gelband and Hume, 1995; Post et al., 1995; Cox and Petrou, 1999) and ATP-sensitive K+ (KATP) channels (Wilson et al., 2000) are inhibited in a Ca2+-dependent fashion and will be briefly discussed.
VSMC BKCa Channels and the Regulation of Arteriolar Tone
Arteriolar VSMCs express BKCa channels that provide negative-feedback regulation of myogenic tone (Figure 1). Both membrane depolarization and increases in intracellular Ca2+ activate BKCa (Tykocki et al., 2017), and because of their large conductance (~200 pS), they powerfully dampen the excitation of VSMCs, preventing vasospasm. BKCa channels consist of a tetramer of KCa1.1 α-pore-forming subunits (gene=KCNMA1) which have seven transmembrane spanning domains (Meera et al., 1997; Figure 2A). Voltage is sensed by positively charged amino acids in membrane spanning domains S2, S3, and S4 (Ma et al., 2006; Figure 2A), while Ca2+ is sensed by two regulator of conductance of K+ (RCK) domains (RCK1 and RCK2) in the long, cytosolic C-terminus of the α-subunit (see (Hoshi et al., 2013a) for references; Figure 2A).
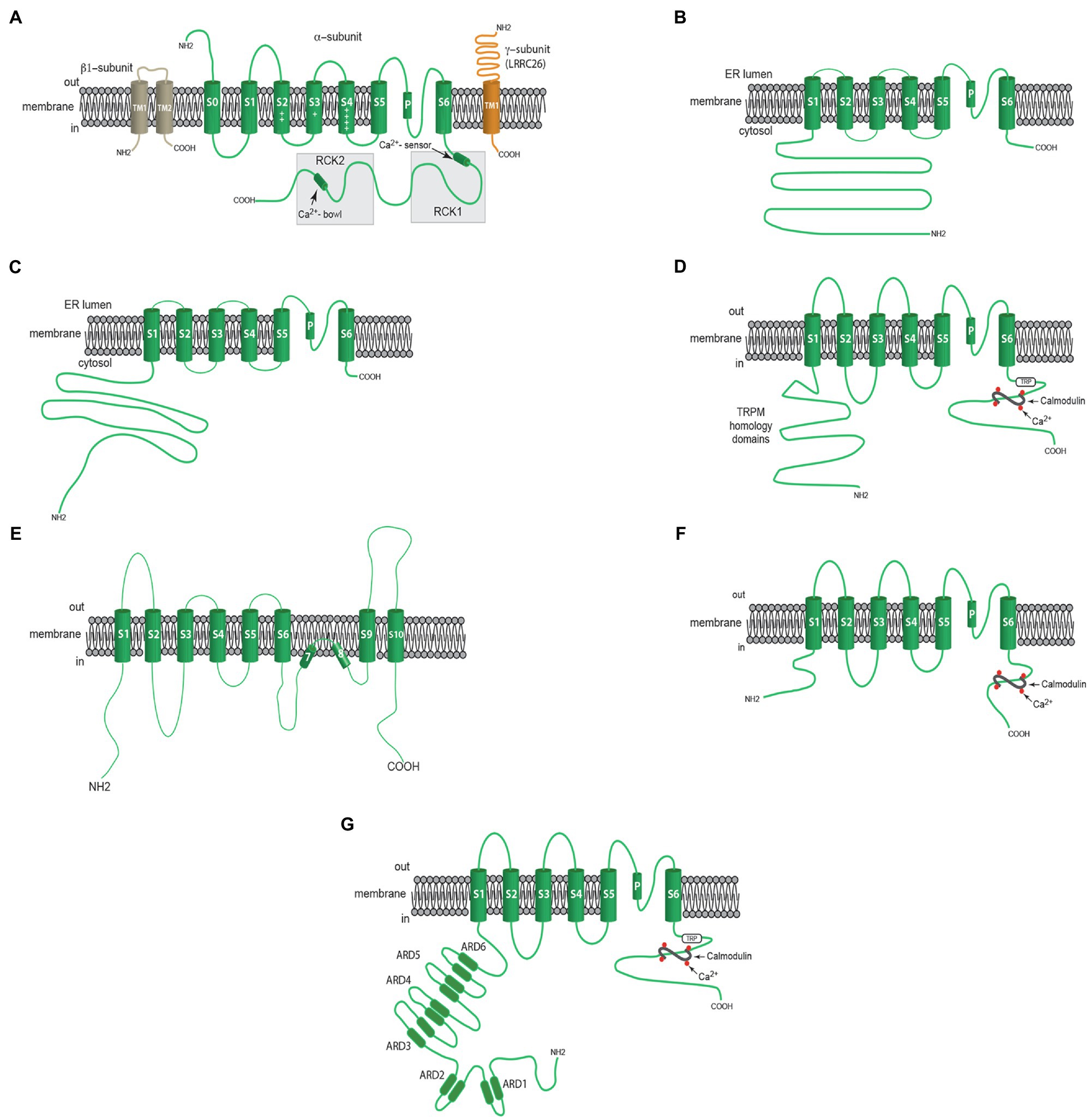
Figure 2. Membrane topology of Ca2+-dependent ion channels involved in the regulation of myogenic tone. (A) Components of VSMC BKCa channels including a β1- subunit with two membrane-spanning domains, one pore-forming α-subunit with seven membrane-spanning domains and a γ-subunit (LRRC26, for example) with one membrane-spanning domain. (B) Shows one α-subunit of an RYR with a large cytosolic N-terminal domain, 6 membrane spanning domains and a short C-terminal sequence. (C) Shows one α-subunit of an IP3R with a large cytosolic N-terminal domain, 6 membrane spanning domains and a short C-terminal sequence. (D) Shows one α-subunit of a TRPM4 channel including an N-Terminal domain with a TRPM homology sequence, 6 membrane spanning domains, and a C-terminal domain containing a TRP sequence and binding sites for calmodulin. (E) Shows one α-subunit of ANO1 (TMEM16A) CaCC with 10 membrane spanning domains. (F) Shows α-subunit of either sKCa or IKCa channels with 6 membrane spanning domains and a C-terminal domain with bindings sites for calmodulin. (G) Shows one α-subunit of a TRPV4 channel with N-terminal sequence containing ankyrin repeat domains (ARDs), 6 membrane spanning domains and a C-terminal domain with TRP sequence and calmodulin binding sites. See text for more information.
Vascular smooth muscle cells express both β and γ subunits that modulate the function of the BKCa channel α-pore-forming subunits (Figure 2A). The primary β subunits in VSMCs are β1 (KCNMB-1, KCaβ1; Tykocki et al., 2017; Figure 2A). These subunits modulate channel gating kinetics and increase the Ca2+ sensitivity of the α-subunit (McCobb et al., 1995; McManus et al., 1995; Meera et al., 1996; Tseng-Crank et al., 1996). They also are dynamically trafficked to the cell membrane from Rab11A-positive recycling endosomes, providing the ability of VSMCs to tune BKCa channel function (see (Leo et al., 2014, 2017) for details). The expression of β1-subunits may be downregulated during disease states like hypertension (Amberg et al., 2003; Tajada et al., 2013) and diabetes (McGahon et al., 2007), decreasing the ability to activate VSMC BKCa channels, increasing myogenic tone. The BKCa channel agonists dehydrosoyasaponin I (McManus et al., 1995) and 17β-estradiol require expression of β1-subunits (Valverde et al., 1999). Thus, β1-subunits control the Ca2+ sensitivity and the pharmacology of BKCa channels in VSMCs.
Arteriolar VSMC BKCa channels have a high Ca2+ setpoint requiring >3μM cytosolic Ca2+ ([Ca2+]in) to open at negative, physiological membrane potentials (−30 to −40mV; Jackson and Blair, 1998). For reference, global [Ca2+]in measured with Fura-2 in arterioles with myogenic tone is on the order of 300–400nM (Brekke et al., 2006). Patch clamp studies have shown that arteriolar BKCa channels are silent when VSMCs are dialyzed with solutions containing 300nM [Ca2+]in (Jackson, 1998), consistent with a high [Ca2+]in threshold for their activation. The high Ca2+ setpoint (threshold) in arteriolar VSMCs may be due to lower expression of the β1-subunits (Yang et al., 2009, 2013) and possible differences in expression of spliced variants of the α-pore-forming subunit (Nourian et al., 2014) compared to VSMCs in larger arteries.
There also are γ-subunits associated with BKCa channels that are leucine-rich-repeat-containing proteins (LRRCs; Yan and Aldrich, 2010; Almassy and Begenisich, 2012; Evanson et al., 2014; Gonzalez-Perez et al., 2014; Figure 2A). LRRCs allow activation of BKCa channels at negative membrane potentials, even in the absence of Ca2+, by shifting their voltage vs. activity relationships to the left (increasing their voltage-sensitivity), facilitating their negative feedback function (Yan and Aldrich, 2010; Gonzalez-Perez et al., 2014). The BKCa channel sensitivity to activation by docosahexaenoic acid (DHA) also is increased by LRRCs (Hoshi et al., 2013b). The role played by LRRCs in arteriolar VSMCs has not been studied.
BKCa channels provide strong negative feedback regulation of both pressure-induced and agonist-induced tone in resistance arteries and arterioles [(see Tykocki et al., 2017) for numerous references]. However, there is regional heterogeneity in the source of Ca2+ that activates BKCa channels in resistance arteries versus arterioles. In most resistance arteries, BKCa channels are controlled by Ca2+ sparks which represent the simultaneous release of Ca2+ from the ER through small, clustered groups of RyRs (Nelson et al., 1995). Vascular smooth muscle cells that utilize this mechanism of BKCa channel activation display the so-called spontaneous-transient-outward currents (STOCs): bursts of activity of small groups of BKCa channels coinciding with the RyR-based Ca2+ sparks [(Nelson et al., 1995), (see Tykocki et al., 2017) for additional references]. In VSMCs where this mechanism is active, pharmacological block of RyRs produces the same effect as block of BKCa channels.
In contrast to many larger resistance arteries, Ca2+ influx through VGCCs directly activates BKCa channels in skeletal muscle arteriolar VSMCs; RyRs are silent, at least under the conditions studied (Westcott and Jackson, 2011; Westcott et al., 2012). In resistance arteries immediately upstream from skeletal muscle arterioles, both RyR-dependent and VGCC-dependent control of BKCa channels is apparent (Westcott and Jackson, 2011; Westcott et al., 2012). These data suggest that there may be a spectrum of control mechanisms that are involved in Ca2+-dependent control of BKCa channels in the resistance vasculature. In cerebral penetrating arterioles, both RyRs and BKCa channels are silent at rest, but both can be activated by low pH (Dabertrand et al., 2012). The molecular mechanisms underlying pH-sensitive recruitment of RyR-control of BKCa channels has not been established. The mechanisms responsible for the differences in Ca2+ sources that control BKCa channels are not known, but likely relate to the number and location of BKCa channels expressed relative to RyRs, VGCCs and other ion channels.
VSMC Ryanodine Receptors and Arteriolar Tone
Ryanodine receptors are composed of four, >500kDa subunits that form ryanodine-sensitive Ca2+ channels in ER membranes (Figure 2B; Van Petegem, 2015; Yan et al., 2015; Zalk et al., 2015). Increases in [Ca2+]in from resting levels [~300nM in VSMCs with tone (Brekke et al., 2006).] up to ~10μM activate release of Ca2+ through RyRs, although high levels of [Ca+]in (>10μM) are inhibitory (Tykocki et al., 2017). Ryanodine receptors also serve as scaffolds for a plethora of signaling proteins [(see Tykocki et al., 2017) for numerous references]. There are three isoforms of RyRs, RyR1, RyR2 and RyR3 [genes=RYR1, RYR2 and RYR3, respectively (Lanner et al., 2010)]: RyR1 is predominantly expressed in skeletal muscle, RyR2 is expressed in cardiac muscle and RyR3 is expressed in the brain and other tissues (Ledbetter et al., 1994; Giannini et al., 1995; Reggiani and te Kronnie, 2006). Vascular smooth muscle expresses multiple isoforms of RYRs with considerable vessel-to-vessel heterogeneity (Vallot et al., 2000; Yang et al., 2005; Salomone et al., 2009; Vaithianathan et al., 2010; Westcott and Jackson, 2011; Westcott et al., 2012). In VSMCs of skeletal muscle arterioles, RyR2 is predominate, and RyR1 is absent (Westcott et al., 2012).
Ryanodine receptors are highly regulated proteins that are modulated by phosphorylation, cellular redox status and interactions with many binding partners in addition to [Ca2+]in (see Tykocki et al., 2017). The overall function of RyRs depends on exactly where they are located in cells and with which ion channels and other proteins they interact.
The elemental Ca2+ signal generated by RyRs is the Ca2+ spark which represents the simultaneous release of Ca2+ from small clusters of RyRs as noted in Section VSMC BKCa Channels and the Regulation of Arteriolar Tone. Calcium influx through VGCCs has been shown to indirectly regulate Ca2+ spark frequency and amplitude by effects on global [Ca2+]in and ER Ca2+ store loading (Essin et al., 2007). Subsequent studies have shown that the magnitude of Ca2+ influx through the persistent activity of membrane clusters of VGCCs, that can be recorded as VGCC Ca2+ sparklets (Navedo et al., 2005; Amberg et al., 2007), controls the amplitude of Ca2+ sparks (Tajada et al., 2013). These data suggest that local influx of Ca2+ is a major determinant of RyR activity in VSMCs.
In skeletal and cardiac muscle, RyRs act in a positive-feedback manner through Ca2+-induced-Ca2+-release (CICR) to cause explosive release of Ca2+ from the ER and subsequent muscle contraction. In both skeletal muscle and cardiac muscle, Ca2+ sparks form the basis of this positive feedback process. A similar positive feedback role for Ca2+ sparks has been proposed for some arteriolar VSMCs (Curtis et al., 2004, 2008; Fellner and Arendshorst, 2005, 2007; Balasubramanian et al., 2007; Tumelty et al., 2007; Kur et al., 2013). In addition to Ca2+ sparks, RyRs can cooperate with IP3Rs and contribute to Ca2+ waves and the positive regulation of myogenic tone in some resistance arteries (Jaggar, 2001; Mufti et al., 2010, 2015; Westcott and Jackson, 2011; Westcott et al., 2012). In other VSMCs, RyR-dependent Ca2+ sparks may also act in an excitatory fashion by activating plasma membrane CaCCs producing the so-called spontaneous transient inward currents (STICs) that cause membrane depolarization, VGCC activation and an increase in tone (ZhuGe et al., 1998; Cheng and Lederer, 2008).
As outlined in Section VSMC BKCa Channels and the Regulation of Arteriolar Tone, in many resistance arteries upstream from the microcirculation, RyRs function as part of a negative-feedback process limiting VSMC excitability. In these vessels, RyR-dependent Ca2+ sparks are functionally coupled to BKCa channels producing membrane hyperpolarization, VGCC deactivation and a decrease in tone (Nelson et al., 1995; Jaggar et al., 1998; Cheng and Lederer, 2008).
However, in skeletal muscle (Westcott and Jackson, 2011; Westcott et al., 2012), cerebral (Dabertrand et al., 2012), and ureteral (Borisova et al., 2009) arterioles downstream from resistance arteries, RyRs are not active and do regulate myogenic tone. Low pH has been shown to recruit RyR-dependent Ca2+ sparks in cerebral arterioles, thereby activating BKCa channels and mediating dilation (Dabertrand et al., 2012). Whether RyRs can be recruited by pH or other conditions in skeletal muscle or ureteral VSMCs has not been studied.
The mechanisms responsible for the heterogeneity in RyR function are not known but most likely result from the specific pattern and magnitude of RyR isoform expression, their cellular localization, and the expression and localization of other ion channels (for example, CaCC vs. BKCa channels) in the plasma membrane over RyRs. This area of research should be explored in more detail in the future.
VSMC IP3Rs and Arteriolar Tone
Inositol 1,4,5 trisphosphate receptors are homotetramers that, like RyRs, form large (~310kDa) Ca2+ release channels in ER membranes (Foskett et al., 2007; Figure 2C). There is one binding site for IP3 on each IP3R monomer (Foskett et al., 2007; Seo et al., 2012, 2015; Taylor et al., 2014).
Three isoforms of IP3Rs (IP3R1, IP3R2, and IP3R3) arise from three genes (ITPR1, ITPR2 and ITPR3 respectively; Foskett et al., 2007). There is regional heterogeneity in VSMC IP3R expression and multiple isoforms are usually expressed in a given VSMC (see (Narayanan et al., 2012) for review). In VSMCs from skeletal muscle resistance arteries and downstream arterioles, we have found expression of IP3R1>IP3R2 >>IP3R3 (Westcott et al., 2012).
Like RyRs, IP3Rs can be triggered to open by increases in [Ca2+]in, with IP3 affecting the sensitivity of the channels to CICR [(see Tykocki et al., 2017) for review]. In the presence of IP3, IP3Rs display a bell shaped [Ca2+]in-response relationship with high [Ca2+]in (>1μM) inhibiting Ca2+ release through these channels (Tu et al., 2005). IP3Rs serve as amplifiers of Ca2+ signals generated by other ion channels. They have a number of protein binding partners that modulate their function including FKBP12 (MacMillan et al., 2005), RACK1 (Patterson et al., 2004; Foskett et al., 2007), ankyrin (Hayashi and Su, 2001), Homer (Tu et al., 1998; Foskett et al., 2007), Bcl family members (Bcl-xL, Mcl and Bcl-2; Li et al., 2007; Eckenrode et al., 2010) and, importantly, a number of TRPC channels including TRPC1 (Boulay et al., 1999), TRPC3 (Boulay et al., 1999; Kiselyov et al., 1999), TRPC4 (Mery et al., 2001), TRPC6 (Boulay et al., 1999) and TRPC7 (Vazquez et al., 2006), either directly (Boulay et al., 1999) or as a component of larger protein complexes (Yuan et al., 2003).
Vascular smooth muscle IP3Rs are essential for the initiation and maintenance of myogenic tone in resistance arteries (Osol et al., 1993; Gonzales et al., 2010, 2014; Garcia and Earley, 2011) and some, but not all arterioles (Jackson and Boerman, 2017). Three mechanisms have been proposed to account for pressure-dependent activation of IP3Rs in resistance arteries including angiotensin receptor-mediated (Gonzales et al., 2014), or integrin-mediated (Mufti et al., 2015) activation of PLCγ1, angiotensin receptor-mediated activation of PLCβ (Mederos y Schnitzler et al., 2008; Schleifenbaum et al., 2014), or mechanisms involving membrane depolarization-induced activation of Gq-coupled receptors (Ganitkevich and Isenberg, 1993; del Valle-Rodriguez et al., 2003; Urena et al., 2007; Mahaut-Smith et al., 2008; Liu et al., 2009; Fernandez-Tenorio et al., 2010; Yamamura et al., 2012).
In contrast, myogenic tone in hamster cheek pouch arterioles (Jackson and Boerman, 2017) and in murine 4th-order mesenteric arteries (Mauban et al., 2015) does not depend on IP3 and activation of IP3Rs. Phospholipase-mediated hydrolysis of phosphatidylcholine and subsequent production of diacylglycerol was proposed to participate in the generation and maintenance of myogenic tone in murine 4th-order mesenteric arteries (Mauban et al., 2015).
Myogenic tone in rat cerebral resistance arteries is accompanied by an increase in the frequency of Ca2+ waves (Jaggar, 2001; Mufti et al., 2010, 2015) that involve both IP3Rs (Mufti et al., 2015) and RyRs (Jaggar, 2001; Mufti et al., 2010, 2015). Similarly, Ca2+ waves in skeletal muscle resistance arteries depend on both RyRs and IP3Rs (Westcott and Jackson, 2011; Westcott et al., 2012). In contrast, Ca2+ waves in downstream skeletal muscle arterioles depend only on Ca2+ release from IP3Rs (Westcott and Jackson, 2011; Westcott et al., 2012) that may amplify Ca2+ influx through VGCCs (Jackson and Boerman, 2018). However, in rat (Miriel et al., 1999) and mouse (Zacharia et al., 2007) mesenteric resistance arteries, Ca2+ waves were inhibited as myogenic tone developed. Thus, there appears to be regional heterogeneity in the role played by IP3R in the development and maintenance of myogenic tone. The mechanisms responsible for the heterogeneity in function of IP3Rs among blood vessels has not been established but likely stems from differences in the IP3R isoforms that are expressed; their localization and interactions with other proteins; and their proximity to other ion channels.
VSMC Ca2+-Activated Cl− Channels and Arteriolar Tone
VSMCs also express CaCCs that may contribute to myogenic tone. The protein anoctamin-1 (gene=ANO1), also known as transmembrane member 16A (TMEM16A), appears to be the molecular basis of CaCCs in VSMCs (Ji et al., 2019). This protein exists as a homodimer with each monomer having 10 membrane spanning domains (S1-S10), with the pore being formed by S3-S7 helices which also contains a Ca2+ binding domain (Ji et al., 2019; Figure 2E). TMEM16A demonstrates a synergistic dependence on voltage and Ca2+ to control its activity, with depolarization and increases in [Ca2+]in leading to opening of these channels (Ji et al., 2019). In vascular smooth muscle, [Cl−]in is elevated due to intracellular Cl− accumulation from the activities of the Cl−/HCO3− exchanger and the Na+/K+/Cl− co-transporter (Matchkov et al., 2013). The elevated [Cl−]in sets the equilibrium potential for Cl− [−40 to −25mV, (Matchkov et al., 2013)] to be positive to the resting membrane potential [−45 to −30mV, (Tykocki et al., 2017)] of VSMCs that develop myogenic tone. Therefore, opening of a Cl− channel results in an outward Cl− current (an inward current in electrophysiological terms), membrane depolarization, activation of VGCCs and an increase in tone (Matchkov et al., 2013).
Calcium-activated chloride channels contribute to agonist-induced tone in a variety of arteries (Bulley and Jaggar, 2014). In addition, STICs carried by Cl− and coupled to RyR-mediated Ca2+ sparks or IP3-based Ca2+ waves have been reported (Bulley and Jaggar, 2014). Cerebral resistance artery VSMCs express TMEM16A that are functionally coupled to transient receptor potential C-family member 6 (TRPC6) channels. Calcium influx through TRPC6 activates TMEM16A contributing to the membrane depolarization, VGCC activation and pressure-induced myogenic tone in these vessels (Bulley et al., 2012; Wang et al., 2016). In hamster cheek pouch arterioles, CaCCs appear to contribute to myogenic tone when VGCCs are active (Jackson, 2020), suggesting that CaCCs may be functionally coupled to VGCCs in those VSMCs. The molecular identity of CaCCs in hamster cheek pouch arteriolar VSMCs has not been established. Additional research on expression and function of CaCCs in resistance arteries and arterioles appears warranted.
VSMC TRPM4 Channels and Arteriolar Tone
VSMCs express many members of the transient receptor potential (TRP) family of ion channels that contribute to myogenic tone [(see Earley and Brayden, 2015; Tykocki et al., 2017) for more information; Figures 1, 3]. Of these, TRPM4 channels are Ca2+-activated and are essential for pressure-induced myogenic tone in cerebral resistance arteries (Gonzales et al., 2014). Like all TRP channels, the pore-forming subunit of TRPM4 channels has six transmembrane domains (S1–S6) which assemble as a tetramer to form a functional ion channel with residues in the intramembrane loop between S5 and S6 forming the channel’s pore (Earley and Brayden, 2015; Figure 2D). A conserved TRP domain located distal to S6 and a TRPM homology region in the NH2 terminus (Earley and Brayden, 2015) distinguish all members of the TRPM family (Earley and Brayden, 2015; Figure 2D). TRPM4 channels selectively conduct monovalent cations such that opening of these channels produces membrane depolarization due primarily to the influx of Na+ (Earley and Brayden, 2015). Calmodulin binding sites in the C-terminus of TRPM4 are essential for Ca2+-dependent activation and the Ca2+-sensitivity of these channels is increased by protein kinase C-dependent phosphorylation in their amino terminus (Earley, 2013). Rho kinase also has been reported to increase the Ca2+-sensitivity of TRPM4 channels in cerebral parenchymal arterioles (Li and Brayden, 2017).
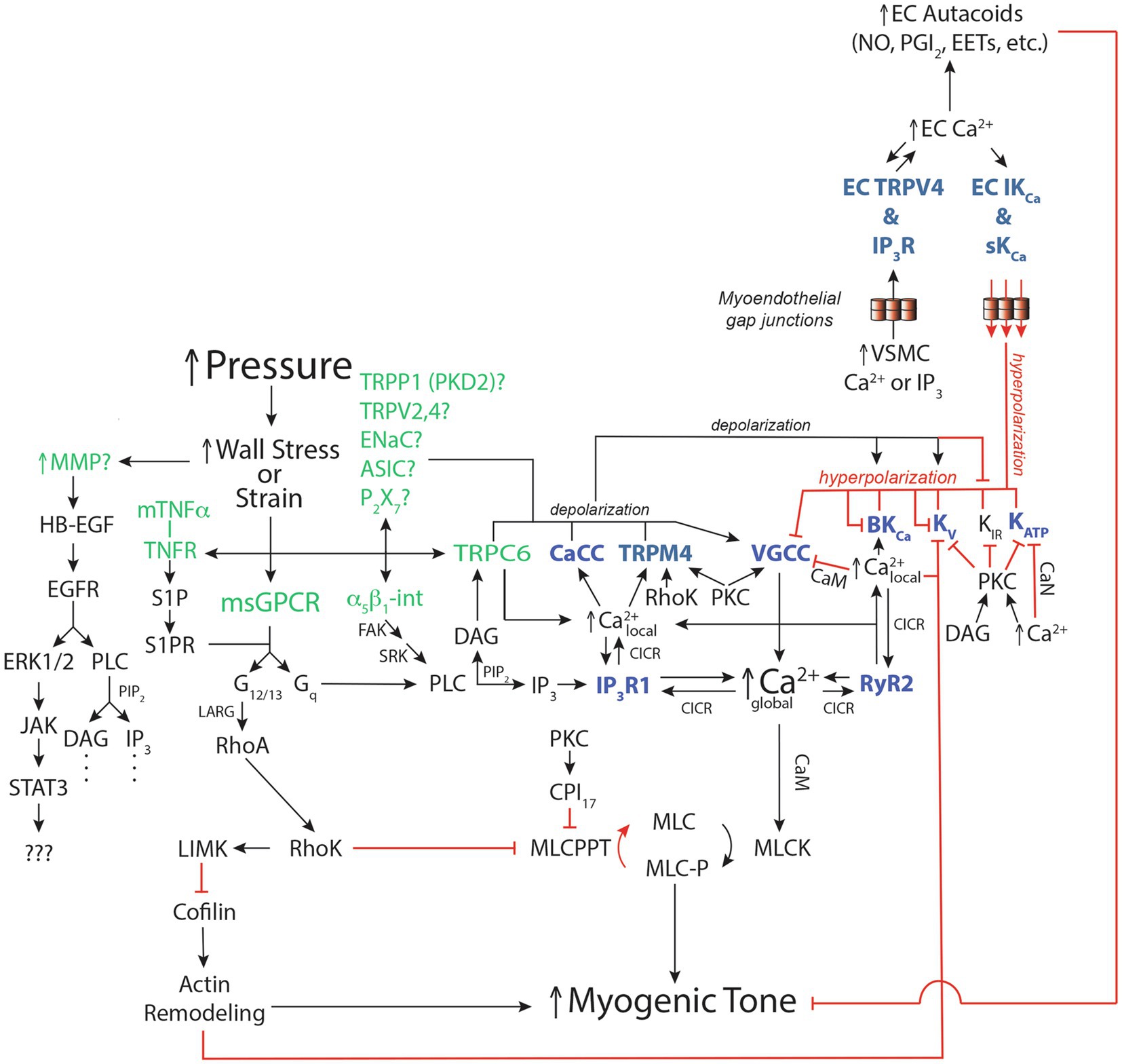
Figure 3. Ca2+-dependent ion channels and vascular smooth muscle signaling pathways for pressure-induced myogenic tone. Schematic diagram [modified from Jackson (2020), (2021)] of reported signaling pathways involved in myogenic tone in resistance arteries and arterioles highlighting the roles played by Ca2+-dependent ion channels. See Section Integration of Ca2+-Dependent Ion Channels Into the Mechanisms Underlying Pressure-Induced Myogenic Tone of text for more details and references. Green font color depicts putative mechanosensors in pressure-induced myogenic tone. Blue font color depicts Ca2+-dependent ion channels involved in regulation of myogenic tone. Black arrows show stimulation, increases or activation of signaling molecules, ion channels or enzymes that participate in myogenic tone. Red capped lines indicate inhibition, decreases or deactivation of signaling molecules, ion channels or enzymes involved in myogenic tone. EC, endothelial cell; VSMC, vascular smooth muscle cell; IKCa, intermediate conductance Ca2+-activated K+ channel; sKCa, small conductance Ca2+-activated K+ channel; MMP, matrix metalloproteinase; HB-EGF, heparin-bound epidermal growth factor; EGFR, Epidermal Growth Factor Receptor; ERK1/2, Extracellular-Signal-Related Kinases 1 or 2; JAK, Janus Kinase; STAT3, Signal Transducer and Activator of Transcription 3; mTNFα, membrane-bound Tumor Necrosis Factor α; TNFR, TNFα Receptor; S1P, Sphingosine-1-phosphate; S1PR, S1P Receptor; α5β1-int, α5β1 Integrin: FAK, Focal Adhesion Kinase; SRK, Src-related kinases; CaCC, Ca2+-activated Cl− channel; TRPP1 (PKD2), Transient Receptor Potential Polycystin family member 1; TRPV2,4, Transient Receptor Potential Vanilloid-family 2 or 4 channels; ENaC, Epithelial Na+ Channel; ASIC, Acid Sensing Ion Channel; P2X7, P2X7 Purinergic Receptor; TRPC6, transient receptor potential C family member 6; TRPM4, transient receptor potential melanostantin member 4; VGCC, voltage-gated Ca2+ channel; BKCa, large-conductance Ca2+-activated K+ channel; KV, voltage-gated K+ channel; KIR, inwardly-rectifying K+ channel; KATP, ATP-sensitive K+ channel; msGPCR, mechanosensitive G-protein-coupled receptor; DAG, diacylglycerol; PKC, protein kinase C; PLC, phospholipase C; PIP2, phosphatidylinositol bisphosphate; IP3, inositol, 1,4,5 trisphosphate; IP3R1, IP3 receptor 1; RyR, ryanodine receptor; CICR, Ca2+-induced-Ca2+ release; LARG, Guanine Nucleotide Exchange Factor LARG; RhoA, small G-protein Rho; RhoK, Rho kinase; LIMK, LIM kinase; CPI17, C-kinase potentiated Protein phosphatase-1 Inhibitor; MLCPPT, myosin light-chain phosphatase; MLC, myosin light-chain; MLCK, myosin light-chain kinase; CaN, calcineurin; CaM, calmodulin.
In cerebral resistance arteries and arterioles, TRPM4 channels are part of the signal transduction pathway for pressure-dependent myogenic tone (Gonzales et al., 2014; Li et al., 2014; Li and Brayden, 2017; see Figure 3 and Section Integration of Ca2+-Dependent Ion Channels Into the Mechanisms Underlying Pressure-Induced Myogenic Tone for more details). In this scheme, TRPM4 channels are activated by release of Ca2+ through IP3Rs into the subplasmalemmal space (Gonzales et al., 2010), with the IP3Rs being activated by IP3, formed by mechanosensitive G-protein coupled receptor-mediated stimulation of phospholipase C (PLC)γ1, and Ca2+ entry through TRPC6 channels, likely activated by both pressure and PLCγ1-production of diacylglycerol (DAG; Gonzales et al., 2014; Figure 3). As noted above, in cerebral parenchymal arterioles, rho-kinase, which also is activated and contributes to myogenic tone, appears to modulate the Ca2+ sensitivity of TRPM4 channels (Li and Brayden, 2017; Figure 3). The Na+ entry through TRPM4 channels, along with the entry of Ca2+ and Na+ through TRPC6 channels produces membrane depolarization and activation of Ca2+ entry through VGCCs, hallmark elements of pressure-dependent myogenic tone (see (Tykocki et al., 2017) for numerous references; Figure 3). The role of TRPM4 in myogenic tone of vessels in other vascular beds has been questioned because global knockout of TRPM4 has no effect on pressure-induced tone in hind limbs of mice (Mathar et al., 2010). However, the details of the mechanisms responsible for pressure-induced tone in the TRPM4 knockout animals was not determined, such that compensation for the global knockout of TRPM4 channels may have occurred. Additional research on TRPM4 and myogenic tone appears warranted.
VSMC TRPP1 (PKD2) Channels and Myogenic Tone
Another potentially Ca2+-activated ion channel that is involved in regulation of myogenic tone are TRPP1 (PKD2) channels. Similar to TRPM4 channels already described, TRPP1 channels are tetramers of 6 membrane spanning domains encoded by the PKD2 gene that have coiled-coil domains in their C-termini and a Ca2+-binding EF-hand motif that may be involved in Ca2+-dependent activation of these channels (Giamarchi and Delmas, 2007). The channels formed from TRPP1 are non-selective cation channels that conduct both Ca2+ and Na+ (Giamarchi and Delmas, 2007). The function of TRPP1 in regulation of myogenic tone is unclear. In murine mesenteric arteries, VSMC TRPP1 channels appear to inhibit myogenic tone (Sharif-Naeini et al., 2009), whereas in rat cerebral arteries VSMC TRPP1 channels significantly contribute to myogenic tone (Narayanan et al., 2013). Conditional knockout of TRPP1 from VSMCs decreases blood pressure and substantially reduces myogenic tone in murine skeletal muscle resistance arteries (Bulley et al., 2018). The plasma membrane expression of TRPP1 in VSMCs is controlled by recycling of sumoylated channels and SUMO1 modification of TRPP1 channels is required for pressure-induced myogenic tone (Hasan et al., 2019). How TRPP1 channels “fit” with other channels that have been shown to be involved in initiation and maintenance of myogenic tone (TRPC6 and TRPM4, for example) remains to be established. Nor has it been established that VSMC TRPP1 channels are activated by Ca2+ or that Ca2+-dependent activation is part of their role in pressure-dependent myogenic tone. It is known that TRPP1 channels can heterodimerize with other members of the TRP family (Giamarchi and Delmas, 2007) such that it is feasible that TRPP1 channels may be part of a multi-channel complex. Additional research will be required to determine how TRPP1 channels and all of the other VSMC ion channels implicated in the generation and maintenance of myogenic tone fit together.
Inhibition of VSMC Ion Channels by Ca2+
Voltage-gated Ca2+ channels composed of CaV1.2 α-subunits (gene=CACNA1C) play a central role myogenic tone as these channels provide the main source of intracellular Ca2+, the primary trigger of VSMC contraction (Tykocki et al., 2017). Calcium-dependent inhibition of VGCCs is mediated by calmodulin that binds to the C-terminus of CaV1.2 channels that make up VSMC VGCCs (Shah et al., 2006). Thus, VGCCs themselves may contribute to the negative-feedback regulation of myogenic tone through this process (Figure 3).
Vascular smooth muscle cells express a diverse array of KV channels that participate in the negative-feedback regulation of myogenic tone (Tykocki et al., 2017). Early studies showed Ca2+-dependent inhibition of KV channel currents in VSMCs from large arteries (Gelband et al., 1993; Ishikawa et al., 1993; Gelband and Hume, 1995; Post et al., 1995; Cox and Petrou, 1999). However, the molecular identity of the KV channel isoform that was inhibited was not identified: it was only suspected to be a channel inhibited by 4-amino pyridine (4-AP). Block of KV channels by 4-AP appears to be Ca2+-dependent, making interpretation of 4-AP sensitivity difficult (Baeyens et al., 2014). It is well established that increased [Ca2+]in inhibits KV7.2-7.5 channels via binding to calmodulin associated with these channels (Alaimo and Villarroel, 2018). KV7 channels contribute substantially to the regulation of myogenic tone in resistance arteries (Mackie et al., 2008; Greenwood and Ohya, 2009; Jepps et al., 2013; Cox and Fromme, 2016). Therefore, it is likely that at least some of the inhibitory effect of elevated [Ca2+]in on whole-cell KV currents is through inhibition of KV7 channels. Regardless, Ca2+-dependent inhibition of active KV channels will cause membrane depolarization, activation of VGCCs and a further increase in [Ca2+]in contributing to the positive-feedback regulation of myogenic tone (Figure 3). It should be noted that the density of KV channels is such that Ca2+-dependent inhibition of these channels serves only to blunt the main, negative-feedback role that KV channels play in the regulation of myogenic tone (Tykocki et al., 2017; Jackson, 2018).
Elevated [Ca2+]in also inhibits ATP-sensitive K+ (KATP) channels through Ca2+-dependent activation of the protein phosphatase, calcineurin (Wilson et al., 2000). These channels are active at rest in the microcirculation of a number of vascular beds (Tykocki et al., 2017). Closure of KATP channels by increased Ca2+ would contribute to membrane depolarization, activation of VGCCs, and a further increase in [Ca2+]in, a positive-feedback process that would increase myogenic tone (Figure 3).
Endothelial Ca2+-Dependent Ion Channels And Arteriolar Tone
Numerous ion channels also contribute to EC function and to the modulation of myogenic tone (Jackson, 2016). Calcium-dependent ion channels in ECs include IP3Rs, small conductance Ca2+-activated K+ (sKCa) channels, intermediate conductance Ca2+-activated K+ (IKCa) channels, CaCCs, transient receptor potential vanilloid-family member 4 (TRPV4) channels and TRPP1 channels.
EC IP3Rs and Arteriolar Tone
Endothelial cells express IP3Rs that contribute to the negative-feedback regulation of arteriolar myogenic tone. Early EC studies demonstrated that the initial increase in [Ca2+]in in response to agonists of EC Gαq-coupled receptors resulted from Ca2+ release from ER stores (Hallam and Pearson, 1986; Colden-Stanfield et al., 1987; Busse et al., 1988; Schilling et al., 1992; Sharma and Davis, 1994, 1995). Subsequent studies pinpointed IP3Rs as the primary Ca2+ release channel involved in this response (Sharma and Davis, 1995; Cohen and Jackson, 2005).
Endothelial cells from arteries (Mountian et al., 1999, 2001; Grayson et al., 2004; Ledoux et al., 2008) and arterioles (Jackson, 2016) appear to express all three isoforms of IP3R. However, the dominant isoform may display regional- or species-dependent heterogeneity. For example, IP3R2 is the dominant IP3R expressed in mouse mesenteric artery ECs (Ledoux et al., 2008), whereas IP3R3 is the dominant IP3R in mouse cremaster muscle arteriolar ECs (Jackson, 2016). There is little information about the specific localization of IP3R in native arteriolar ECs. In both EC-VSMC co-cultures and in intact mouse cremaster arterioles, IP3R1 localizes at sites of MEGJs (Isakson, 2008). Similarly, in mouse mesenteric resistance arteries, EC IP3Rs cluster near holes in the internal elastic lamina (Ledoux et al., 2008), that are sites of myoendothelial projections (MEPs) and MEGJs (Sandow and Hill, 2000; Figure 1). Although the IP3R isoform(s) expressed in these IP3R clusters has not been identified, they were demonstrated to be the sites of EC Ca2+ pulsars, localized IP3-dependent Ca2+ events arising from clusters of IP3Rs in the ER that extend into MEPs (Kansui et al., 2008; Ledoux et al., 2008; Figure 1).
Myoendothelial projections and MEGJs are important signaling microdomains in resistance arteries and arterioles and contain a growing list of signaling proteins including IP3Rs (Kansui et al., 2008; Ledoux et al., 2008), IKCa channels (Sandow et al., 2006), TRPA1 channels (Earley et al., 2009a), TRPV4 channels (Sonkusare et al., 2012, 2014), anchoring proteins [e.g., AKAP150 (Sonkusare et al., 2014)], protein kinases [e.g., PKC (Sonkusare et al., 2014)], NO synthase (Straub et al., 2011; Wolpe et al., 2021), Na+/K+ ATPase (Dora et al., 2008) and other proteins (Straub et al., 2014; Wolpe et al., 2021; Figure 1). Calcium influx through TRPA1 and TRPV4, which produce small, localized Ca2+ events called Ca2+ sparklets, likely serves as the source of Ca2+ that actually triggers release of Ca2+ through IP3Rs to form both localized Ca2+ pulsars (Kansui et al., 2008; Ledoux et al., 2008), Ca2+ wavelets (Tran et al., 2012) and larger Ca2+ waves (Duza and Sarelius, 2004; Kansui et al., 2008) found in ECs of resistance arteries and arterioles. These Ca2+ events are then translated into several signals that are vasodilatory and tend to reduce or temper myogenic tone. Activation of EC sKCa and IKCa channels (Section EC sKCa and IKCa Channels and Arteriolar Tone, below) leads to EC hyperpolarization, which can be conducted through MEGJs to overlying VSMCs, deactivating VGCCs, reducing VSMC Ca2+ influx and decreasing myogenic tone (Figure 3). Endothelial cell IP3R Ca2+ signals also activate EC NO synthase and production of other EC autacoids (PGI2, EETs, H2O2, etc.) that diffuse to overlying VSMCS and reduce myogenic tone (Figure 3).
Global increases in [Ca2+]in reported for ECs in intact resistance arteries or arterioles exposed to endothelium-dependent vasodilators (Dora et al., 1997; Marrelli, 2000; Cohen and Jackson, 2005; Socha et al., 2011) are a complicated blend of IP3R-mediated Ca2+ pulsars, Ca2+ wavelets and Ca2+ waves. Both the number and frequency of Ca2+ pulsars (Ledoux et al., 2008) and both synchronous (Duza and Sarelius, 2004; Socha et al., 2012) and asynchronous (Ledoux et al., 2008; Socha et al., 2012) Ca2+ waves are increased by endothelium-dependent vasodilators, such as acetylcholine (Ledoux et al., 2008; Socha et al., 2012) or adenosine (Duza and Sarelius, 2004). Additional research will be required to discover the precise IP3R isoform expression, location and function related to endothelium-dependent vasomotor activity and modulation of myogenic tone.
Arteriolar ECs Do Not Express Functional RyRs
Early studies of ECs from large arteries provided evidence for expression of functional RyRs (Lesh et al., 1993; Graier et al., 1994, 1998; Ziegelstein et al., 1994; Rusko et al., 1995; Kohler et al., 2001b). In contrast, there is a lack of evidence for expression of RyRs in resistance artery and arteriolar ECs. Mouse mesenteric resistance artery ECs do not express mRNA for the three RyR isoforms, whereas transcripts for IP3Rs are readily detected (Ledoux et al., 2008). In addition, resting Ca2+ levels or acetylcholine-evoked Ca2+ events in mouse (Ledoux et al., 2008) or rat (Kansui et al., 2008) mesenteric resistance artery ECs are unaffected by concentrations of ryanodine that block RyRs. Similarly, mouse cremaster arteriolar ECs do not express message for RyRs (Jackson, 2016), and the RyR agonist, caffeine (10mM), has no effect on [Ca2+]in in these ECs (Cohen and Jackson, 2005). These data do not support a role for RyRs in resistance artery or arteriolar EC Ca2+ signals.
EC sKCa and IKCa Channels and Arteriolar Tone
Resistance artery and arteriolar ECs express both sKCa (KCa2.3; gene=KCNN3) and IKCa (KCa3.1; gene=KCNN4) channels (Kohler et al., 2001a; Eichler et al., 2003; Taylor et al., 2003; Sandow et al., 2006; Si et al., 2006; Grgic et al., 2009). These channels are a tetramer of six transmembrane domain subunits with cytosolic N- and C-termini (Adelman et al., 2012; Figure 2F). The ion conducting pore is formed from a pore loop between membrane spanning domains 5 and 6, as in voltage-gated K+ channels (Adelman et al., 2012). Calmodulin interacts with the intracellular C-terminus to gate opening of both channels (Xia et al., 1998; Fanger et al., 1999; Adelman et al., 2012; Sforna et al., 2018). The Ca2+ sensitivity of sKCa and IKCa channels is an order of magnitude higher than for BKCa channels. The threshold for activation by Ca2+ binding to calmodulin occurs at 100nM, 50% of maximal activation at 300nM and maximal activation at 1μM for both sKCa channels (Xia et al., 1998) and IKCa channels (Ishii et al., 1997). The distinct pharmacology of sKCa and IKCa channels has helped to define their function in intact vessels (Jackson, 2016).
Endothelial cell sKCa and IKCa channels are not distributed uniformly in the plasma membrane of ECs: IKCa channels cluster at MEPs (Sandow et al., 2006; Ledoux et al., 2008; Earley et al., 2009a), the site of MEGJs (Sandow and Hill, 2000), whereas sKCa channels are more distributed around the cell periphery (Sandow et al., 2006). Both channels appear to reside in macromolecular signaling complexes. At MEPs and near MEGJ’s, IKCa channels localize with IP3Rs (Ledoux et al., 2008), TRPA1 channels (Earley et al., 2009a), TRPV4 channels (Sonkusare et al., 2012, 2014), anchoring proteins [e.g., AKAP150 (Sonkusare et al., 2014)], protein kinases [e.g., PKC (Sonkusare et al., 2014)], nitric oxide synthase (Straub et al., 2011; Wolpe et al., 2021), Na+/K+ ATPase (Dora et al., 2008), likely G-protein coupled receptors (Sonkusare et al., 2014) and other proteins (Straub et al., 2014; Wolpe et al., 2021; Figure 1). Local Ca2+ signals through TRPA1 channels (Earley et al., 2009a), TRPV4 channels (Sonkusare et al., 2012, 2014), and/or IP3Rs (Ledoux et al., 2008) activate IKCa (and sKCa) channels, leading to EC hyperpolarization and conduction of this signal to overlying VSMCs. Hyperpolarization then deactivates VSMC VGCCs reducing myogenic tone (Figure 3). EC hyperpolarization also may amplify Ca2+ influx through TRPA1 and TRPV4 channels by increasing the electrochemical gradient for Ca2+ influx (Qian et al., 2014).
Endothelial cell sKCa channels also exist in macromolecular signaling microdomains around the EC periphery. They are found in cholesterol-rich areas (caveolae or lipid rafts) and colocalize with caveolin-1 (Absi et al., 2007). Ca2+ influx through TRPC3 channels selectively activates sKCa channels in rat cerebral arteries (Kochukov et al., 2014), suggesting that TRPC3 and sKCa channels exist in the same microdomain. In mouse carotid arteries, sKCa channels are in caveolae adjacent to EC-EC gap junction plaques (Brahler et al., 2009). Conditional knockout of sKCa channels attenuates shear-stress-induced vasodilation in these arteries, suggesting that sKCa channel localization has functional consequences (Brahler et al., 2009). The respective EC localization of sKCa and IKCa channels and their signaling microdomains explain how these two channels mediate different facets of EC hyperpolarization and the regulation of myogenic tone (Crane et al., 2003; Si et al., 2006).
Because ECs are electrically coupled to VSMCs via MEGJs, resting membrane potential of ECs can impact myogenic tone. Resting EC membrane potential is determined, in part, by the activity of sKCa and IKCa channels. Overexpression of sKCa channels (which hyperpolarizes ECs) reduces myogenic tone of mesenteric resistance arteries (Taylor et al., 2003). In contrast, conditional knockout of sKCa channels has the opposite effect (EC depolarization and an increase in myogenic tone; Taylor et al., 2003). Consistent with these data, pharmacological inhibition of sKCa and IKCa channels, or both channels augment(s) myogenic tone in rat cerebral parenchymal arterioles (Cipolla et al., 2009; Hannah et al., 2011). Endothelial cell sKCa and IKCa channels seem to play a smaller role in modulating myogenic tone of larger cerebral resistance arteries, although they remain important in endothelium-dependent agonist-induced vasodilation (Cipolla et al., 2009). Nonetheless, sKCa and IKCa channels significantly contribute to EC-dependent negative-feedback regulation of myogenic tone.
Endothelium-dependent vasodilators that act through Gq-coupled receptors also activate sKCa and IKCa channels. In some vessels, such as guinea-pig carotid artery (Corriu et al., 1996), rat mesenteric arteries preconstricted with phenylephrine (Crane et al., 2003) and porcine coronary arteries (Bychkov et al., 2002) both channels appear to be involved because block of both sKCa and IKCa channels is necessary to inhibit agonist-induced EC hyperpolarization. In contrast, IKCa channels mediate endothelium-dependent hyperpolarization and vasodilation in rat cerebral arteries (Marrelli et al., 2003) and in murine arteries and arterioles (Brahler et al., 2009). The reason for this heterogeneity in the roles played by sKCa and IKCa channels between vascular beds is not apparent and will require further research.
EC BKCa Channels and Arteriolar Tone
The expression and function of BKCa channels in ECs remains debatable (Sandow and Grayson, 2009). As described for VSMCs, BKCa channels are activated by both voltage and Ca2+, have a much larger conductance (~250 pS) than sKCa and IKCa channels, do not require association with calmodulin, and display pharmacology distinct from sKCa and IKCa channels (Hoshi et al., 2013a; Tykocki et al., 2017). Cultured large artery ECs have been reported to express BKCa channels (see (Sandow and Grayson, 2009) for references). Native ECs isolated from hypoxic rats (Hughes et al., 2010; Riddle et al., 2011) or cholesterol depleted ECs (Riddle et al., 2011) express functional BKCa channels. In cultured ECs, BKCa channels are located in caveolae and caveolin inhibits their function (Wang et al., 2005). These studies open the possibility that EC BKCa channels are normally inhibited. Conversely, chronic hypoxia, and potentially other stresses or pathologies, that alter membrane lipid domains may upregulate EC BKCa channel function (Sandow and Grayson, 2009).
Electrophysiological studies of freshly isolated bovine coronary artery (Gauthier et al., 2002), mouse carotid artery (Brahler et al., 2009), and rat cerebral parenchymal arteriolar (Hannah et al., 2011) ECs found only sKCa channel and IKCa channel currents; no BKCa channel currents were detected. While it has been reported that ECs in freshly isolated rat cremaster arterioles express protein for BKCa channels (Ungvari et al., 2002), neither mRNA nor protein for this channel were detected in bovine coronary artery ECs (Gauthier et al., 2002). Murine skeletal muscle resistance artery and arteriolar ECs lack BKCa channel mRNA (Jackson, 2016). Thus, there may be regional or species heterogeneity in EC expression of BKCa channels. Additional research appears to be warranted to define if and where EC BKCa are expressed, how they are regulated and their function in the regulation of myogenic tone.
EC Ca2+-Activated Cl− Channels and Arteriolar Tone
Electrophysiological studies of bovine pulmonary artery and human umbilical vein ECs demonstrate the functional expression of CaCCs (Nilius et al., 1997; Zhong et al., 2000). Unlike VSMCs (see Section VSMC Ca2+-Activated Cl− Channels and Arteriolar Tone), initial studies did not report expression of TMEM16A in ECs in lung sections (Huang et al., 2009; Ferrera et al., 2011). However, more recent studies have identified TMEM16A expression and function in human pulmonary artery ECs and have shown that over expression of these channels leads to EC dysfunction (Skofic Maurer et al., 2020). In hypertension, EC TMEM16A also contributes to endothelial dysfunction (Ma et al., 2017). TMEM16A is expressed in murine cerebral capillary ECs where it regulates membrane potential, Ca2+ signaling, proliferation, migration, and blood brain barrier permeability (Suzuki et al., 2020). Block of TMEM16A preserves blood brain barrier function after ischemic stroke (Liu et al., 2019). Hypoxia stimulates proliferation of brain capillary ECs via increased expression of TMEM16A (Suzuki et al., 2021). Hypoxia also increases expression of TMEM16A in mouse cardiac ECs (Wu et al., 2014).
The function of TMEM16A in arteriolar ECs related to regulation of myogenic tone is not clear. In murine capillary ECs, block of TMEM16A results in membrane hyperpolarization suggesting that in ECs, like in VSMCs (see Section VSMC Ca2+-Activated Cl− Channels and Arteriolar Tone), activation of these CaCCs leads to membrane depolarization, counter to the effects of activation of EC sKCa and IKCa channels which produce robust EC hyperpolarization. Thus, it may be that CaCCs in ECs are part of a negative feedback mechanism to dampen membrane hyperpolarization induced by EC sKCa and IKCa channels when intracellular Ca2+ is elevated.
EC TRPV4 and Regulation of Arteriolar Tone
Transient receptor potential vanilloid-family member 4 channels are another prominent Ca2+-modulated ion channel expressed in ECs (Sonkusare et al., 2012, 2014; Hong et al., 2018; Chen and Sonkusare, 2020). These channels are formed from a tetramer of six membrane spanning domain subunits, with the pore of the channel formed by a pore-loop between domains 5 and 6 like many other ion channels (Figure 2G). They conduct primarily Ca2+ and are activated by a diverse array of chemicals including EETs (Nilius et al., 2004). In ECs, TRPV4 channels exist in signaling complexes near MEGJ’s along with IKCa channels, IP3Rs and other proteins (Sonkusare et al., 2012, 2014; Hong et al., 2018; Chen and Sonkusare, 2020; Figures 1, 3). Intracellular Ca2+ potentiates the activation of TRPV4 channels through calmodulin that binds to the C-terminal region of this channel (Strotmann et al., 2003).
Endothelial TRPV4 channels mediate agonist-induced, endothelium-dependent vasodilation, particularly in arterioles where activation of these receptors leads to activation of IKCa channels, EC hyperpolarization and conduction of this hyperpolarization to overlying VSMCs to induce vasodilation (Marrelli et al., 2007; Earley et al., 2009b; Sonkusare et al., 2012, 2014; Zhang et al., 2013; Zheng et al., 2013; Du et al., 2016; Diaz-Otero et al., 2018; Figure 3). In addition, TRPV4 channels play a central role in myoendothelial negative-feedback that tempers vascular tone in the absence of an endothelial agonist. Agonist-induced activation of VSMC Gq-coupled receptors leads to a global increase in EC intracellular Ca2+(Dora et al., 1997; Schuster et al., 2001; Tuttle and Falcone, 2001; Jackson et al., 2008; Kansui et al., 2008) that contributes to the negative-feedback regulation of vascular tone (Lemmey et al., 2020). Studies in murine mesenteric resistance arteries have shown that endothelial TRPV4 channels are activated during this process through a mechanism involving Ca2+ release through IP3Rs, resulting in activation of IKCa channels blunting agonist-induced vasoconstriction (Hong et al., 2018; Figure 3). Similarly, studies in rat cremaster arterioles have shown that endothelial TRPV4 channels are activated at low intravascular pressure, leading to TRPV4 Ca2+ sparklets (localized [Ca2+]in signals through small groups of TRPV4 channels), activation of IKCa channels and dampening of myogenic tone (Bagher et al., 2012). The precise signal that is communicated from VSMCs to ECs to initiate myoendothelial feedback remains in question, with data supporting Ca2+ as the signal (Garland et al., 2017) and other findings supporting IP3 as the signal (Tran et al., 2012; Hong et al., 2018). Additional research will be required to determine whether Ca2+ or IP3 mediates myoendothelial negative-feedback and whether there is heterogeneity among vessels in which signal (Ca2+ or IP3) is used.
EC TRPP1 Channels and Myogenic Tone
Endothelial cells also express TRPP1 channels where they function in shear-stress dependent vasodilation (MacKay et al., 2020). Shear-stress-induced increases in EC [Ca2+]in that activate sKCa channels, IKCa channels and EC nitric oxide synthase were shown to be substantially impaired by conditional knockout of EC TRPP1 with no change in Ca2+ signals activated by muscarinic receptor activation (MacKay et al., 2020). Calcium-dependent activation of TRPP1 channels was not established in these studies, so [Ca2+]in modulation of these channels in ECs and their role in regulating myogenic tone other than when activated by shear-stress remains to be established.
Integration Of Ca2+-Dependent Ion Channels Into The Mechanisms Underlying Pressure-Induced Myogenic Tone
As outlined in Sections above, Ca2+-dependent ion channels in VSMCs and ECs are involved in the initiation, maintenance and modulation of pressure-induced myogenic tone. Figure 3 integrates this information into a working model with the function of VSMC and EC Ca2+-dependent ion channels highlighted.
Pressure-Dependent Activation of Mechanosensors Leads to Formation of IP3 and DAG
Multiple mechano-sensors of wall stress (or strain) initiate the myogenic response culminating in steady-state myogenic tone (Figure 3). Putative sensors (in green font in Figure 3) include: several G-protein coupled receptors (Brayden et al., 2013; Narayanan et al., 2013; Schleifenbaum et al., 2014; Storch et al., 2015; Kauffenstein et al., 2016; Mederos et al., 2016; Hong et al., 2017; Pires et al., 2017; Chennupati et al., 2019), various cation channels (Welsh et al., 2002; Jernigan and Drummond, 2005; Gannon et al., 2008; VanLandingham et al., 2009; Narayanan et al., 2013; Nemeth et al., 2020), integrins (Davis et al., 2001; Martinez-Lemus et al., 2005; Colinas et al., 2015), matrix metalloproteinases and epidermal growth factor receptors (EGFR; Lucchesi et al., 2004; Amin et al., 2011); and membrane-bound tumor necrosis factor α (mTNF α), TNF α receptor (TNFR) and downstream sphingosine-1-phosphate (S1P) signaling (Kroetsch et al., 2017; Figure 3). Pressure-dependent stimulation of these putative mechano-sensors activates phospholipase C (PLC) catalyzing hydrolysis of membrane phosphatidyl inositol 4,5 bisphosphate (PIP2) to form IP3 and DAG (Figure 3).
Activation of Plasma Membrane Ion Channels Produces Membrane Depolarization
Pressure- and likely DAG-induced activation of plasma membrane TRPC6 channels results in Ca2+ influx through these channels (Slish et al., 2002; Welsh et al., 2002). The resultant local [Ca2+]in increase, along with IP3, activates IP3Rs to release Ca2+ from the ER, amplifying the local [Ca2+]in increase. This subplasmalemmal increase in [Ca2+]in then activates overlying plasma membrane TRPM4 channels. Calcium influx through TRPC6 channels also activates plasma membrane Ca2+-activated Cl− channels (CaCCs; Bulley et al., 2012; Wang et al., 2016). The cation influx through TRPC6 and TRPM4 channels, and Cl− efflux through CaCCs causes membrane depolarization (Figure 3). As noted in Section Pressure-Dependent Activation of Mechanosensors Leads to Formation of IP3 and DAG and shown in Figure 3, additional cation channels including TRPP1 channels may contribute to the pressure-induced depolarization.
Membrane Depolarization Activates VGCC, Induces Ca2+ Influx and Stimulates VSMC Contraction
Membrane depolarization induced by ionic currents through TRPC6 channels, TRPM4 channels, CaCCs and other ion channels activates plasma membrane VGCCs resulting in Ca2+ influx. VGCC-mediated Ca2+ influx across the plasma membrane, along with IP3R-mediated Ca2+ release from ER Ca2+ stores, increases cytoplasmic (global) [Ca2+]in levels, leading to calmodulin-mediated myosin light-chain kinase (MLCK) activation, phosphorylation of the myosin light-chains (MLC), actin-myosin cross-bridge formation, cross bridge cycling and an increase in myogenic tone (vasoconstriction; Cole and Welsh, 2011; Figure 3).
K+ Channels Provide Negative Feedback to Dampen Myogenic Tone
Membrane depolarization-induced activation of VGCCs is inherently a positive-feedback process because the Ca2+ influx through these channels will itself lead to depolarization and further activation of VGCCs. This process is limited in VSMCs by activation of at least three negative-feedback processes. Membrane depolarization activates KV channels, and membrane depolarization along with increased [Ca2+]in activates BKCa channels. The K+ efflux through these two K+ channels (which by themselves would cause membrane hyperpolarization) blunts and limits depolarization-induced activation of VGCC (Figure 3; Jackson, 2017, 2020). Additional negative feedback arises from Ca2+-dependent inactivation of VGCCs (Shah et al., 2006; Figure 3).
Parallel Activation of Protein Kinase C and Rho-Kinase
In addition to activating TRPC6 channels, the DAG formed from the activity of PLC along with elevated [Ca2+]in activates protein kinase C (PKC) supporting the increase in tone by increasing the activity of TRPM4 channels (supporting depolarization) and VGCCs (promoting Ca2+ influx) while blunting the activity of several K+ channels (also supporting membrane depolarization; Jackson, 2020, 2021; Figure 3). The negative feedback involving KV channels is blunted by Ca2+-dependent inhibition of these channels (Gelband et al., 1993; Ishikawa et al., 1993; Gelband and Hume, 1995; Post et al., 1995; Cox and Petrou, 1999; Figure 3). Ca2+-dependent activation of the protein phosphatase, calcineurin, inhibits ATP-sensitive K+ (KATP) channels, limiting their activity and promoting depolarization (Wilson et al., 2000; Figure 3).
Stimulation of the mechano-sensors in vascular smooth muscle also activates the small G-protein rhoA, which, in turn, activates rho-kinase (Chennupati et al., 2019; Figure 3). Rho kinase phosphorylates a number of substrates that also support myogenic tone including inhibition of myosin light chain phosphatase (MLCPPT; Cole and Welsh, 2011), stimulation of actin cytoskeleton remodeling that accompanies activation of the contractile machinery (Loirand et al., 2006; Moreno-Dominguez et al., 2013), inhibition of KV channels as a consequence of actin remodeling (Luykenaar et al., 2009) and increasing the Ca2+ sensitivity of TRPM4 channels (Li and Brayden, 2017; Figure 3). Activated PKC also may inhibit MLCPPT through phosphorylation of the inhibitory protein, CPI17 (Cole and Welsh, 2011; Figure 3).
Endothelial Cells Contribute to the Negative-Feedback Regulation of Myogenic Tone
Endothelial cells lining resistance arteries and arterioles play a negative-feedback role, dampening myogenic tone both through the Ca2+-dependent production of vasodilator autacoids (PGI2, NO, EETS, etc.) and by conduction of Ca2+-dependent membrane hyperpolarization from the endothelium to overlying VSMCs via MEGJs (Figures 1, 3). Endothelial cells chemically and electrically converse with VSMCs through MEGJs that may form at myoendothelial projections that penetrate holes in the internal elastic lamina and contact the overlying VSMCs. Heterocellular gap junctions (MEGJs) between ECs and VSMCs form and allow small molecules (like IP3) and ionic currents (including Ca2+) to move between the cells. Pressure-induced increases in VSMC [Ca2+]in or IP3 can pass to endothelial cells leading to EC IP3R-induced Ca2+ signals (Ca2+ pulsars and wavelets) that can increase the production of Ca2+-dependent EC vasodilator autacoids that feedback to the VSMCs reducing myogenic tone (Figure 3). In addition, increased EC [Ca2+]in will activate EC sKCa and IKCa channels causing EC membrane hyperpolarization. Myoendothelial gap junctions allow this hyperpolarization to be passed from ECs to VSMCs, producing VSMC hyperpolarization, deactivation of VSMC VGCCs and reduced myogenic tone (Figure 3). Thus, the production of EC autacoids and EC membrane potential are both strongly dependent on the activity of Ca2+-dependent ion channels in the endothelium including IP3Rs, TRPV4 channels, sKCa channels and IKCa channels (Lemmey et al., 2020).
Final Perspective
As outlined in this perspective, Ca2+-activated ion channels in both VSMCs and ECs contribute to the regulation of myogenic tone. However, there appears to be considerable heterogeneity in the specific details of their roles in this process among vessels in different vascular beds around the body. The mechanisms responsible for this heterogeneity remains to be established. It is also clear that there is a paucity of information about the cellular and molecular details surrounding which channels are expressed, their localization and their regulation relative to myogenic tone in arterioles around the body. Mesenteric and cerebral resistance artery ion channel expression and function has been well studied. However, we know relatively little about ion channel expression and function in the downstream arterioles in microcirculation, which is really the business end of the cardiovascular system. Future studies directed specifically at understanding control of ion channel expression and function in the microcirculation and how they vary among vascular beds in different organs is warranted.
Author Contributions
WJ conceived, wrote, and edited this manuscript.
Funding
Supported by National Heart, Lung and Blood Institute grants HL-137694 and PO1-HL-070687.
Author Disclaimer
The content is solely the responsibility of the author and does not necessarily represent the official views of the National Institutes of Health.
Conflict of Interest
The author declares that the research was conducted in the absence of any commercial or financial relationships that could be construed as a potential conflict of interest.
Publisher’s Note
All claims expressed in this article are solely those of the authors and do not necessarily represent those of their affiliated organizations, or those of the publisher, the editors and the reviewers. Any product that may be evaluated in this article, or claim that may be made by its manufacturer, is not guaranteed or endorsed by the publisher.
References
Absi, M., Burnham, M. P., Weston, A. H., Harno, E., Rogers, M., and Edwards, G. (2007). Effects of methyl beta-cyclodextrin on EDHF responses in pig and rat arteries; association between SK(Ca) channels and caveolin-rich domains. Br. J. Pharmacol. 151, 332–340. doi: 10.1038/sj.bjp.0707222
Adelman, J. P., Maylie, J., and Sah, P. (2012). Small-conductance Ca2+−activated K+ channels: form and function. Annu. Rev. Physiol. 74, 245–269. doi: 10.1146/annurev-physiol-020911-153336
Alaimo, A., and Villarroel, A. (2018). Calmodulin: a multitasking protein in Kv7.2 potassium channel functions. Biomol. Ther. 8:57. doi: 10.3390/biom8030057
Almassy, J., and Begenisich, T. (2012). The LRRC26 protein selectively alters the efficacy of BK channel activators. Mol. Pharmacol. 81, 21–30. doi: 10.1124/mol.111.075234
Amberg, G. C., Bonev, A. D., Rossow, C. F., Nelson, M. T., and Santana, L. F. (2003). Modulation of the molecular composition of large conductance, Ca(2+) activated K(+) channels in vascular smooth muscle during hypertension. J. Clin. Invest. 112, 717–724. doi: 10.1172/JCI200318684
Amberg, G. C., Navedo, M. F., Nieves-Cintron, M., Molkentin, J. D., and Santana, L. F. (2007). Calcium sparklets regulate local and global calcium in murine arterial smooth muscle. J. Physiol. 579, 187–201. doi: 10.1113/jphysiol.2006.124420
Amin, A. H., Abd Elmageed, Z. Y., Partyka, M., and Matrougui, K. (2011). Mechanisms of myogenic tone of coronary arteriole: role of down stream signaling of the EGFR tyrosine kinase. Microvasc. Res. 81, 135–142. doi: 10.1016/j.mvr.2010.11.001
Baeyens, N., Bouryi, V., and Morel, N. (2014). Extracellular calcium modulates the inhibitory effect of 4-aminopyridine on Kv current in vascular smooth muscle cells. Eur. J. Pharmacol. 723, 116–123. doi: 10.1016/j.ejphar.2013.11.042
Bagher, P., Beleznai, T., Kansui, Y., Mitchell, R., Garland, C. J., and Dora, K. A. (2012). Low intravascular pressure activates endothelial cell TRPV4 channels, local Ca2+ events, and IKCa channels, reducing arteriolar tone. Proc. Natl. Acad. Sci. U. S. A. 109, 18174–18179. doi: 10.1073/pnas.1211946109
Bagher, P., and Segal, S. S. (2011). Regulation of blood flow in the microcirculation: role of conducted vasodilation. Acta Physiol (Oxford) 202, 271–284. doi: 10.1111/j.1748-1716.2010.02244.x
Balasubramanian, L., Ahmed, A., Lo, C. M., Sham, J. S., and Yip, K. P. (2007). Integrin-mediated mechanotransduction in renal vascular smooth muscle cells: activation of calcium sparks. Am. J. Phys. Regul. Integr. Comp. Phys. 293, R1586–R1594. doi: 10.1152/ajpregu.00025.2007
Barlow, C. A., Rose, P., Pulver-Kaste, R. A., and Lounsbury, K. M. (2006). Excitation-transcription coupling in smooth muscle. J. Physiol. 570, 59–64. doi: 10.1113/jphysiol.2005.098426
Borisova, L., Wray, S., Eisner, D. A., and Burdyga, T. (2009). How structure, Ca signals, and cellular communications underlie function in precapillary arterioles. Circ. Res. 105, 803–810. doi: 10.1161/CIRCRESAHA.109.202960
Boulay, G., Brown, D. M., Qin, N., Jiang, M., Dietrich, A., Zhu, M. X., et al. (1999). Modulation of Ca(2+) entry by polypeptides of the inositol 1,4, 5-trisphosphate receptor (IP3R) that bind transient receptor potential (TRP): evidence for roles of TRP and IP3R in store depletion-activated Ca(2+) entry. Proc. Natl. Acad. Sci. U. S. A. 96, 14955–14960. doi: 10.1073/pnas.96.26.14955
Brahler, S., Kaistha, A., Schmidt, V. J., Wolfle, S. E., Busch, C., Kaistha, B. P., et al. (2009). Genetic deficit of SK3 and IK1 channels disrupts the endothelium-derived hyperpolarizing factor vasodilator pathway and causes hypertension. Circulation 119, 2323–2332. doi: 10.1161/CIRCULATIONAHA.108.846634
Brayden, J. E., Li, Y., and Tavares, M. J. (2013). Purinergic receptors regulate myogenic tone in cerebral parenchymal arterioles. J. Cereb. Blood Flow Metab. 33, 293–299. doi: 10.1038/jcbfm.2012.169
Brekke, J. F., Jackson, W. F., and Segal, S. S. (2006). Arteriolar smooth muscle Ca2+ dynamics during blood flow control in hamster cheek pouch. J. Appl. Physiol. 101, 307–315. doi: 10.1152/japplphysiol.01634.2005
Bulley, S., Fernandez-Pena, C., Hasan, R., Leo, M. D., Muralidharan, P., Mackay, C. E., et al. (2018). Arterial smooth muscle cell PKD2 (TRPP1) channels regulate systemic blood pressure. elife 7:e42628. doi: 10.7554/eLife.42628
Bulley, S., and Jaggar, J. H. (2014). Cl(−) channels in smooth muscle cells. Pflugers Arch. 466, 861–872. doi: 10.1007/s00424-013-1357-2
Bulley, S., Neeb, Z. P., Burris, S. K., Bannister, J. P., Thomas-Gatewood, C. M., Jangsangthong, W., et al. (2012). TMEM16A/ANO1 channels contribute to the myogenic response in cerebral arteries. Circ. Res. 111, 1027–1036. doi: 10.1161/CIRCRESAHA.112.277145
Busse, R., Fichtner, H., Luckhoff, A., and Kohlhardt, M. (1988). Hyperpolarization and increased free calcium in acetylcholine-stimulated endothelial cells. Am. J. Phys. 255, H965–H969. doi: 10.1152/ajpheart.1988.255.4.H965
Bychkov, R., Burnham, M. P., Richards, G. R., Edwards, G., Weston, A. H., Feletou, M., et al. (2002). Characterization of a charybdotoxin-sensitive intermediate conductance Ca2+−activated K+ channel in porcine coronary endothelium: relevance to EDHF. Br. J. Pharmacol. 137, 1346–1354. doi: 10.1038/sj.bjp.0705057
Cartin, L., Lounsbury, K. M., and Nelson, M. T. (2000). Coupling of Ca(2+) to CREB activation and gene expression in intact cerebral arteries from mouse: roles of ryanodine receptors and voltage-dependent Ca(2+) channels. Circ. Res. 86, 760–767. doi: 10.1161/01.RES.86.7.760
Chen, Y.-L., and Sonkusare, S. K. (2020). Endothelial TRPV4 channels and vasodilator reactivity. Curr. Top. Membr. 85, 89–117. doi: 10.1016/bs.ctm.2020.01.007
Cheng, H., and Lederer, W. J. (2008). Calcium sparks. Physiol. Rev. 88, 1491–1545. doi: 10.1152/physrev.00030.2007
Chennupati, R., Wirth, A., Favre, J., Li, R., Bonnavion, R., Jin, Y. J., et al. (2019). Myogenic vasoconstriction requires G12/G13 and LARG to maintain local and systemic vascular resistance. elife 8:e49374. doi: 10.7554/eLife.49374
Cipolla, M. J., Smith, J., Kohlmeyer, M. M., and Godfrey, J. A. (2009). SKCa and IKCa channels, myogenic tone, and vasodilator responses in middle cerebral arteries and parenchymal arterioles: effect of ischemia and reperfusion. Stroke 40, 1451–1457. doi: 10.1161/STROKEAHA.108.535435
Cohen, K. D., and Jackson, W. F. (2005). Membrane hyperpolarization is not required for sustained muscarinic agonist-induced increases in intracellular Ca2+ in arteriolar endothelial cells. Microcirculation 12, 169–182. doi: 10.1080/10739680590904973
Colden-Stanfield, M., Schilling, W. P., Ritchie, A. K., Eskin, S. G., Navarro, L. T., and Kunze, D. L. (1987). Bradykinin-induced increases in cytosolic calcium and ionic currents in cultured bovine aortic endothelial cells. Circ. Res. 61, 632–640. doi: 10.1161/01.RES.61.5.632
Cole, W. C., and Welsh, D. G. (2011). Role of myosin light chain kinase and myosin light chain phosphatase in the resistance arterial myogenic response to intravascular pressure. Arch. Biochem. Biophys. 510, 160–173. doi: 10.1016/j.abb.2011.02.024
Colinas, O., Moreno-Dominguez, A., Zhu, H. L., Walsh, E. J., Perez-Garcia, M. T., Walsh, M. P., et al. (2015). alpha5-integrin-mediated cellular signaling contributes to the myogenic response of cerebral resistance arteries. Biochem. Pharmacol. 97, 281–291. doi: 10.1016/j.bcp.2015.08.088
Corriu, C., Feletou, M., Canet, E., and Vanhoutte, P. M. (1996). Endothelium-derived factors and hyperpolarization of the carotid artery of the Guinea-pig. Br. J. Pharmacol. 119, 959–964. doi: 10.1111/j.1476-5381.1996.tb15765.x
Cox, R. H., and Fromme, S. (2016). Functional expression profile of voltage-gated K(+) channel subunits in rat small mesenteric arteries. Cell Biochem. Biophys. 74, 263–276. doi: 10.1007/s12013-015-0715-4
Cox, R. H., and Petrou, S. (1999). Ca(2+) influx inhibits voltage-dependent and augments Ca(2+)-dependent K(+) currents in arterial myocytes. Am. J. Phys. 277, C51–C63. doi: 10.1152/ajpcell.1999.277.1.C51
Crane, G. J., Gallagher, N., Dora, K. A., and Garland, C. J. (2003). Small- and intermediate-conductance calcium-activated K+ channels provide different facets of endothelium-dependent hyperpolarization in rat mesenteric artery. J. Physiol. 553, 183–189. doi: 10.1113/jphysiol.2003.051896
Curtis, T. M., Tumelty, J., Dawicki, J., Scholfield, C. N., and McGeown, J. G. (2004). Identification and spatiotemporal characterization of spontaneous Ca2+ sparks and global Ca2+ oscillations in retinal arteriolar smooth muscle cells. Invest. Ophthalmol. Vis. Sci. 45, 4409–4414. doi: 10.1167/iovs.04-0719
Curtis, T. M., Tumelty, J., Stewart, M. T., Arora, A. R., Lai, F. A., McGahon, M. K., et al. (2008). Modification of smooth muscle Ca2+−sparks by tetracaine: evidence for sequential RyR activation. Cell Calcium 43, 142–154. doi: 10.1016/j.ceca.2007.04.016
Dabertrand, F., Nelson, M. T., and Brayden, J. E. (2012). Acidosis dilates brain parenchymal arterioles by conversion of calcium waves to sparks to activate BK channels. Circ. Res. 110, 285–294. doi: 10.1161/CIRCRESAHA.111.258145
Davis, M. J., Wu, X., Nurkiewicz, T. R., Kawasaki, J., Davis, G. E., Hill, M. A., et al. (2001). Integrins and mechanotransduction of the vascular myogenic response. Am. J. Physiol. Heart Circ. Physiol. 280, H1427–H1433. doi: 10.1152/ajpheart.2001.280.4.H1427
de Wit, C., and Griffith, T. M. (2010). Connexins and gap junctions in the EDHF phenomenon and conducted vasomotor responses. Pflugers Arch. 459, 897–914. doi: 10.1007/s00424-010-0830-4
del Valle-Rodriguez, A., Lopez-Barneo, J., and Urena, J. (2003). Ca2+ channel-sarcoplasmic reticulum coupling: a mechanism of arterial myocyte contraction without Ca2+ influx. EMBO J. 22, 4337–4345. doi: 10.1093/emboj/cdg432
Diaz-Otero, J. M., Yen, T. C., Fisher, C., Bota, D., Jackson, W. F., and Dorrance, A. M. (2018). Mineralocorticoid receptor antagonism improves parenchymal arteriole dilation via a TRPV4-dependent mechanism and prevents cognitive dysfunction in hypertension. Am. J. Physiol. Heart Circ. Physiol. 315, H1304–H1315. doi: 10.1152/ajpheart.00207.2018
Dora, K. A., Doyle, M. P., and Duling, B. R. (1997). Elevation of intracellular calcium in smooth muscle causes endothelial cell generation of NO in arterioles. Proc. Natl. Acad. Sci. U.S.A. 94, 6529–6534.
Dora, K. A., Gallagher, N. T., McNeish, A., and Garland, C. J. (2008). Modulation of endothelial cell KCa3.1 channels during endothelium-derived hyperpolarizing factor signaling in mesenteric resistance arteries. Circ. Res. 102, 1247–1255. doi: 10.1161/CIRCRESAHA.108.172379
Dora, K. A., and Garland, C. J. (2013). Linking hyperpolarization to endothelial cell calcium events in arterioles. Microcirculation 20, 248–256. doi: 10.1111/micc.12041
Du, J., Wang, X., Li, J., Guo, J., Liu, L., Yan, D., et al. (2016). Increasing TRPV4 expression restores flow-induced dilation impaired in mesenteric arteries with aging. Sci. Rep. 6:22780. doi: 10.1038/srep22780
Duza, T., and Sarelius, I. H. (2004). Localized transient increases in endothelial cell Ca2+ in arterioles in situ: implications for coordination of vascular function. Am. J. Physiol. Heart Circ. Physiol. 286, H2322–H2331. doi: 10.1152/ajpheart.00006.2004
Earley, S. (2013). TRPM4 channels in smooth muscle function. Pflugers Arch. 465, 1223–1231. doi: 10.1007/s00424-013-1250-z
Earley, S., and Brayden, J. E. (2015). Transient receptor potential channels in the vasculature. Physiol. Rev. 95, 645–690. doi: 10.1152/physrev.00026.2014
Earley, S., Gonzales, A. L., and Crnich, R. (2009a). Endothelium-dependent cerebral artery dilation mediated by TRPA1 and Ca2+-activated K+ channels. Circ. Res. 104, 987–994. doi: 10.1161/CIRCRESAHA.108.189530
Earley, S., Pauyo, T., Drapp, R., Tavares, M. J., Liedtke, W., and Brayden, J. E. (2009b). TRPV4-dependent dilation of peripheral resistance arteries influences arterial pressure. Am. J. Physiol. Heart Circ. Physiol. 297, H1096–H1102. doi: 10.1152/ajpheart.00241.2009
Eckenrode, E. F., Yang, J., Velmurugan, G. V., Foskett, J. K., and White, C. (2010). Apoptosis protection by Mcl-1 and Bcl-2 modulation of inositol 1,4,5-trisphosphate receptor-dependent Ca2+ signaling. J. Biol. Chem. 285, 13678–13684. doi: 10.1074/jbc.M109.096040
Eichler, I., Wibawa, J., Grgic, I., Knorr, A., Brakemeier, S., Pries, A. R., et al. (2003). Selective blockade of endothelial Ca2+−activated small- and intermediate-conductance K+-channels suppresses EDHF-mediated vasodilation. Br. J. Pharmacol. 138, 594–601. doi: 10.1038/sj.bjp.0705075
Essin, K., Welling, A., Hofmann, F., Luft, F. C., Gollasch, M., and Moosmang, S. (2007). Indirect coupling between Cav1.2 channels and ryanodine receptors to generate Ca2+ sparks in murine arterial smooth muscle cells. J. Physiol. 584, 205–219. doi: 10.1113/jphysiol.2007.138982
Evanson, K. W., Bannister, J. P., Leo, M. D., and Jaggar, J. H. (2014). LRRC26 is a functional BK channel auxiliary gamma subunit in arterial smooth muscle cells. Circ. Res. 115, 423–431. doi: 10.1161/CIRCRESAHA.115.303407
Fanger, C. M., Ghanshani, S., Logsdon, N. J., Rauer, H., Kalman, K., Zhou, J., et al. (1999). Calmodulin mediates calcium-dependent activation of the intermediate conductance KCa channel, IKCa1. J. Biol. Chem. 274, 5746–5754. doi: 10.1074/jbc.274.9.5746
Fellner, S. K., and Arendshorst, W. J. (2005). Angiotensin II Ca2+ signaling in rat afferent arterioles: stimulation of cyclic ADP ribose and IP3 pathways. Am. J. Physiol. Ren. Physiol. 288, F785–F791. doi: 10.1152/ajprenal.00372.2004
Fellner, S. K., and Arendshorst, W. J. (2007). Voltage-gated Ca2+ entry and ryanodine receptor Ca2+−induced Ca2+ release in preglomerular arterioles. Am. J. Physiol. Ren. Physiol. 292, F1568–F1572. doi: 10.1152/ajprenal.00459.2006
Fernandez-Tenorio, M., Gonzalez-Rodriguez, P., Porras, C., Castellano, A., Moosmang, S., Hofmann, F., et al. (2010). Short communication: genetic ablation of L-type Ca2+ channels abolishes depolarization-induced Ca2+ release in arterial smooth muscle. Circ. Res. 106, 1285–1289. doi: 10.1161/CIRCRESAHA.109.213967
Ferrera, L., Zegarra-Moran, O., and Galietta, L. J. (2011). Ca2+−activated Cl− channels. Compr. Physiol. 1, 2155–2174. doi: 10.1002/cphy.c110017
Foskett, J. K., White, C., Cheung, K. H., and Mak, D. O. (2007). Inositol trisphosphate receptor Ca2+ release channels. Physiol. Rev. 87, 593–658. doi: 10.1152/physrev.00035.2006
Ganitkevich, V., and Isenberg, G. (1993). Membrane potential modulates inositol 1,4,5-trisphosphate-mediated Ca2+ transients in Guinea-pig coronary myocytes. J. Physiol. 470, 35–44. doi: 10.1113/jphysiol.1993.sp019845
Gannon, K. P., Vanlandingham, L. G., Jernigan, N. L., Grifoni, S. C., Hamilton, G., and Drummond, H. A. (2008). Impaired pressure-induced constriction in mouse middle cerebral arteries of ASIC2 knockout mice. Am. J. Physiol. Heart Circ. Physiol. 294, H1793–H1803. doi: 10.1152/ajpheart.01380.2007
Garcia, Z., and Earley, S. (2011). PLC gamma-1 is required for IP3-mediated activation of TRPM4 and pressure-induced depolarization and vasoconstriction in cerebral arteries. FASEB J. 25:1024.16. doi: 10.1096/fasebj.25.1_supplement.1024.16
Garland, C. J., Bagher, P., Powell, C., Ye, X., Lemmey, H. A. L., Borysova, L., et al. (2017). Voltage-dependent Ca(2+) entry into smooth muscle during contraction promotes endothelium-mediated feedback vasodilation in arterioles. Sci. Signal. 10:eaal3806. doi: 10.1126/scisignal.aal3806
Garland, C. J., and Dora, K. A. (2017). EDH: endothelium-dependent hyperpolarization and microvascular signalling. Acta Physiol (Oxford) 219, 152–161. doi: 10.1111/apha.12649
Gauthier, K. M., Liu, C., Popovic, A., Albarwani, S., and Rusch, N. J. (2002). Freshly isolated bovine coronary endothelial cells do not express the BK Ca channel gene. J. Physiol. 545, 829–836. doi: 10.1113/jphysiol.2002.029843
Gelband, C. H., and Hume, J. R. (1995). [Ca2+]i inhibition of K+ channels in canine renal artery. Novel mechanism for agonist-induced membrane depolarization. Circ. Res. 77, 121–130. doi: 10.1161/01.RES.77.1.121
Gelband, C. H., Ishikawa, T., Post, J. M., Keef, K. D., and Hume, J. R. (1993). Intracellular divalent cations block smooth muscle K+ channels. Circ. Res. 73, 24–34. doi: 10.1161/01.RES.73.1.24
Giamarchi, A., and Delmas, P. (2007). “Activation mechanisms and functional roles of TRPP2 Cation channels,” in TRP Ion Channel Function in Sensory Transduction and Cellular Signaling Cascades. eds. W. B. Liedtke and S. Heller (Boca Raton (FL): CRC Press/Taylor & Francis).
Giannini, G., Conti, A., Mammarella, S., Scrobogna, M., and Sorrentino, V. (1995). The ryanodine receptor/calcium channel genes are widely and differentially expressed in murine brain and peripheral tissues. J. Cell Biol. 128, 893–904. doi: 10.1083/jcb.128.5.893
Gonzales, A. L., Amberg, G. C., and Earley, S. (2010). Ca2+ release from the sarcoplasmic reticulum is required for sustained TRPM4 activity in cerebral artery smooth muscle cells. Am. J. Phys. Cell Physiol. 299, C279–C288. doi: 10.1152/ajpcell.00550.2009
Gonzales, A. L., Yang, Y., Sullivan, M. N., Sanders, L., Dabertrand, F., Hill-Eubanks, D. C., et al. (2014). A PLCgamma1-dependent, force-sensitive signaling network in the myogenic constriction of cerebral arteries. Sci. Signal. 7:ra49. doi: 10.1126/scisignal.2004732
Gonzalez-Perez, V., Xia, X. M., and Lingle, C. J. (2014). Functional regulation of BK potassium channels by gamma1 auxiliary subunits. Proc. Natl. Acad. Sci. U. S. A. 111, 4868–4873. doi: 10.1073/pnas.1322123111
Graier, W. F., Paltauf-Doburzynska, J., Hill, B. J., Fleischhacker, E., Hoebel, B. G., Kostner, G. M., et al. (1998). Submaximal stimulation of porcine endothelial cells causes focal Ca2+ elevation beneath the cell membrane. J. Physiol. 506, 109–125. doi: 10.1111/j.1469-7793.1998.109bx.x
Graier, W. F., Simecek, S., Bowles, D. K., and Sturek, M. (1994). Heterogeneity of caffeine- and bradykinin-sensitive Ca2+ stores in vascular endothelial cells. Biochem. J. 300, 637–641. doi: 10.1042/bj3000637
Grayson, T. H., Haddock, R. E., Murray, T. P., Wojcikiewicz, R. J. H., and Hill, C. E. (2004). Inositol 1,4,5-trisphosphate receptor subtypes are differentially distributed between smooth muscle and endothelial layers of rat arteries. Cell Calcium 36:447. doi: 10.1016/j.ceca.2004.04.005
Greenwood, I. A., and Ohya, S. (2009). New tricks for old dogs: KCNQ expression and role in smooth muscle. Br. J. Pharmacol. 156, 1196–1203. doi: 10.1111/j.1476-5381.2009.00131.x
Grgic, I., Kaistha, B. P., Hoyer, J., and Kohler, R. (2009). Endothelial Ca+−activated K+ channels in normal and impaired EDHF-dilator responses--relevance to cardiovascular pathologies and drug discovery. Br. J. Pharmacol. 157, 509–526. doi: 10.1111/j.1476-5381.2009.00132.x
Hallam, T. J., and Pearson, J. D. (1986). Exogenous ATP raises cytoplasmic free calcium in fura-2 loaded piglet aortic endothelial cells. FEBS Lett. 207, 95–99. doi: 10.1016/0014-5793(86)80019-9
Hannah, R. M., Dunn, K. M., Bonev, A. D., and Nelson, M. T. (2011). Endothelial SK(Ca) and IK(Ca) channels regulate brain parenchymal arteriolar diameter and cortical cerebral blood flow. J. Cereb. Blood Flow Metab. 31, 1175–1186. doi: 10.1038/jcbfm.2010.214
Hasan, R., Leo, M. D., Muralidharan, P., Mata-Daboin, A., Yin, W., Bulley, S., et al. (2019). SUMO1 modification of PKD2 channels regulates arterial contractility. Proc. Natl. Acad. Sci. U. S. A. 116, 27095–27104. doi: 10.1073/pnas.1917264116
Hayashi, T., and Su, T. P. (2001). Regulating ankyrin dynamics: roles of sigma-1 receptors. Proc. Natl. Acad. Sci. U. S. A. 98, 491–496. doi: 10.1073/pnas.021413698
Hoffmann, E. K., Lambert, I. H., and Pedersen, S. F. (2009). Physiology of cell volume regulation in vertebrates. Physiol. Rev. 89, 193–277. doi: 10.1152/physrev.00037.2007
Hong, K., Cope, E. L., DeLalio, L. J., Marziano, C., Isakson, B. E., and Sonkusare, S. K. (2018). TRPV4 (transient receptor potential Vanilloid 4) channel-dependent negative feedback mechanism regulates Gq protein-coupled receptor-induced vasoconstriction. Arterioscler. Thromb. Vasc. Biol. 38, 542–554. doi: 10.1161/ATVBAHA.117.310038
Hong, K., Li, M., Nourian, Z., Meininger, G. A., and Hill, M. A. (2017). Angiotensin II type 1 receptor mechanoactivation involves RGS5 (regulator of G protein signaling 5) in skeletal muscle arteries: impaired trafficking of RGS5 in hypertension. Hypertension 70, 1264–1272. doi: 10.1161/HYPERTENSIONAHA.117.09757
Hoshi, T., Pantazis, A., and Olcese, R. (2013a). Transduction of voltage and Ca2+ signals by Slo1 BK channels. Physiology (Bethesda) 28, 172–189. doi: 10.1152/physiol.00055.2012
Hoshi, T., Tian, Y., Xu, R., Heinemann, S. H., and Hou, S. (2013b). Mechanism of the modulation of BK potassium channel complexes with different auxiliary subunit compositions by the omega-3 fatty acid DHA. Proc. Natl. Acad. Sci. U. S. A. 110, 4822–4827. doi: 10.1073/pnas.1222003110
Huang, F., Rock, J. R., Harfe, B. D., Cheng, T., Huang, X., Jan, Y. N., et al. (2009). Studies on expression and function of the TMEM16A calcium-activated chloride channel. Proc. Natl. Acad. Sci. U. S. A. 106, 21413–21418. doi: 10.1073/pnas.0911935106
Hughes, J. M., Riddle, M. A., Paffett, M. L., Gonzalez Bosc, L. V., and Walker, B. R. (2010). Novel role of endothelial BKCa channels in altered vasoreactivity following hypoxia. Am. J. Physiol. Heart Circ. Physiol. 299, H1439–H1450. doi: 10.1152/ajpheart.00124.2010
Isakson, B. E. (2008). Localized expression of an Ins(1,4,5)P3 receptor at the myoendothelial junction selectively regulates heterocellular Ca2+ communication. J. Cell Sci. 121, 3664–3673. doi: 10.1242/jcs.037481
Ishii, T. M., Silvia, C., Hirschberg, B., Bond, C. T., Adelman, J. P., and Maylie, J. (1997). A human intermediate conductance calcium-activated potassium channel. Proc. Natl. Acad. Sci. U. S. A. 94, 11651–11656. doi: 10.1073/pnas.94.21.11651
Ishikawa, T., Hume, J. R., and Keef, K. D. (1993). Modulation of K+ and Ca2+ channels by histamine H1-receptor stimulation in rabbit coronary artery cells. J. Physiol. 468, 379–400. doi: 10.1113/jphysiol.1993.sp019777
Jackson, W. F. (1998). Potassium channels and regulation of the microcirculation. Microcirculation 5, 85–90. doi: 10.1111/j.1549-8719.1998.tb00057.x
Jackson, W. F. (2016). “Endothelial cell ion channel expression and function in arterioles and resistance arteries,” in Vascular Ion Channels in Physiology and Disease. eds. I. Levitan and A. M. Dopico (Switzerland: Springer International Publishing), 3–36.
Jackson, W. F. (2017). Potassium channels in regulation of vascular smooth muscle contraction and growth. Adv. Pharmacol. 78, 89–144. doi: 10.1016/bs.apha.2016.07.001
Jackson, W. F. (2018). KV channels and the regulation of vascular smooth muscle tone. Microcirculation 25:e12421. doi: 10.1111/micc.12421
Jackson, W. F. (2020). Ion channels and the regulation of myogenic tone in peripheral arterioles. Curr. Top. Membr. 85, 19–58. doi: 10.1016/bs.ctm.2020.01.002
Jackson, W. F. (2021). Myogenic tone in peripheral resistance arteries and arterioles: the pressure is On! Front. Physiol. 12:699517. doi: 10.3389/fphys.2021.699517
Jackson, W. F., and Blair, K. L. (1998). Characterization and function of Ca(2+)-activated K+ channels in arteriolar muscle cells. Am. J. Phys. 274, H27–H34. doi: 10.1152/ajpheart.1998.274.1.H27
Jackson, W. F., and Boerman, E. M. (2017). Regional heterogeneity in the mechanisms of myogenic tone in hamster arterioles. Am. J. Physiol. Heart Circ. Physiol. 313, H667–H675. doi: 10.1152/ajpheart.00183.2017
Jackson, W. F., and Boerman, E. M. (2018). Voltage-gated Ca(2+) channel activity modulates smooth muscle cell calcium waves in hamster cremaster arterioles. Am. J. Physiol. Heart Circ. Physiol. 315, H871–H878. doi: 10.1152/ajpheart.00292.2018
Jackson, W. F., Boerman, E. M., Lange, E. J., Lundback, S. S., and Cohen, K. D. (2008). Smooth muscle alpha1D-adrenoceptors mediate phenylephrine-induced vasoconstriction and increases in endothelial cell Ca2+ in hamster cremaster arterioles. Br. J. Pharmacol. 155, 514–524. doi: 10.1038/bjp.2008.276
Jaggar, J. H. (2001). Intravascular pressure regulates local and global Ca(2+) signaling in cerebral artery smooth muscle cells. Am. J. Phys. Cell Physiol. 281, C439–C448. doi: 10.1152/ajpcell.2001.281.2.C439
Jaggar, J. H., Stevenson, A. S., and Nelson, M. T. (1998). Voltage dependence of Ca2+ sparks in intact cerebral arteries. Am. J. Phys. 274, C1755–C1761. doi: 10.1152/ajpcell.1998.274.6.C1755
Jepps, T. A., Olesen, S. P., and Greenwood, I. A. (2013). One man’s side effect is another man's therapeutic opportunity: targeting Kv7 channels in smooth muscle disorders. Br. J. Pharmacol. 168, 19–27. doi: 10.1111/j.1476-5381.2012.02133.x
Jernigan, N. L., and Drummond, H. A. (2005). Vascular ENaC proteins are required for renal myogenic constriction. Am. J. Physiol. Ren. Physiol. 289, F891–F901. doi: 10.1152/ajprenal.00019.2005
Ji, Q., Guo, S., Wang, X., Pang, C., Zhan, Y., Chen, Y., et al. (2019). Recent advances in TMEM16A: structure, function, and disease. J. Cell. Physiol. 234, 7856–7873. doi: 10.1002/jcp.27865
Kansui, Y., Garland, C. J., and Dora, K. A. (2008). Enhanced spontaneous Ca2+ events in endothelial cells reflect signalling through myoendothelial gap junctions in pressurized mesenteric arteries. Cell Calcium 44, 135–146. doi: 10.1016/j.ceca.2007.11.012
Kauffenstein, G., Tamareille, S., Prunier, F., Roy, C., Ayer, A., Toutain, B., et al. (2016). Central role of P2Y6 UDP receptor in arteriolar myogenic tone. Arterioscler. Thromb. Vasc. Biol. 36, 1598–1606. doi: 10.1161/ATVBAHA.116.307739
Kiselyov, K., Mignery, G. A., Zhu, M. X., and Muallem, S. (1999). The N-terminal domain of the IP3 receptor gates store-operated hTrp3 channels. Mol. Cell 4, 423–429. doi: 10.1016/S1097-2765(00)80344-5
Kochukov, M. Y., Balasubramanian, A., Abramowitz, J., Birnbaumer, L., and Marrelli, S. P. (2014). Activation of endothelial transient receptor potential C3 channel is required for small conductance calcium-activated potassium channel activation and sustained endothelial hyperpolarization and vasodilation of cerebral artery. J. Am. Heart Assoc. 3:e000913. doi: 10.1161/JAHA.114.000913
Kohler, R., Brakemeier, S., Kuhn, M., Behrens, C., Real, R., Degenhardt, C., et al. (2001a). Impaired hyperpolarization in regenerated endothelium after balloon catheter injury. Circ. Res. 89, 174–179. doi: 10.1161/hh1401.093460
Kohler, R., Brakemeier, S., Kuhn, M., Degenhardt, C., Buhr, H., Pries, A., et al. (2001b). Expression of ryanodine receptor type 3 and TRP channels in endothelial cells: comparison of in situ and cultured human endothelial cells. Cardiovasc. Res. 51, 160–168. doi: 10.1016/s0008-6363(01)00281-4
Kroetsch, J. T., Levy, A. S., Zhang, H., Aschar-Sobbi, R., Lidington, D., Offermanns, S., et al. (2017). Constitutive smooth muscle tumour necrosis factor regulates microvascular myogenic responsiveness and systemic blood pressure. Nat. Commun. 8:14805. doi: 10.1038/ncomms14805
Kur, J., Bankhead, P., Scholfield, C. N., Curtis, T. M., and McGeown, J. G. (2013). Ca(2+) sparks promote myogenic tone in retinal arterioles. Br. J. Pharmacol. 168, 1675–1686. doi: 10.1111/bph.12044
Lanner, J. T., Georgiou, D. K., Joshi, A. D., and Hamilton, S. L. (2010). Ryanodine receptors: structure, expression, molecular details, and function in calcium release. Cold Spring Harb. Perspect. Biol. 2:a003996. doi: 10.1101/cshperspect.a003996
Ledbetter, M. W., Preiner, J. K., Louis, C. F., and Mickelson, J. R. (1994). Tissue distribution of ryanodine receptor isoforms and alleles determined by reverse transcription polymerase chain reaction. J. Biol. Chem. 269, 31544–31551. doi: 10.1016/S0021-9258(18)31728-9
Ledoux, J., Taylor, M. S., Bonev, A. D., Hannah, R. M., Solodushko, V., Shui, B., et al. (2008). Functional architecture of inositol 1,4,5-trisphosphate signaling in restricted spaces of myoendothelial projections. Proc. Natl. Acad. Sci. U. S. A. 105, 9627–9632. doi: 10.1073/pnas.0801963105
Lemmey, H. A. L., Garland, C. J., and Dora, K. A. (2020). Intrinsic regulation of microvascular tone by myoendothelial feedback circuits. Curr. Top. Membr. 85, 327–355. doi: 10.1016/bs.ctm.2020.01.004
Leo, M. D., Bannister, J. P., Narayanan, D., Nair, A., Grubbs, J. E., Gabrick, K. S., et al. (2014). Dynamic regulation of beta1 subunit trafficking controls vascular contractility. Proc. Natl. Acad. Sci. U. S. A. 111, 2361–2366. doi: 10.1073/pnas.1317527111
Leo, M. D., Zhai, X., Muralidharan, P., Kuruvilla, K. P., Bulley, S., Boop, F. A., et al. (2017). Membrane depolarization activates BK channels through ROCK-mediated beta1 subunit surface trafficking to limit vasoconstriction. Sci. Signal. 10:eaah5417. doi: 10.1126/scisignal.aah5417
Lesh, R. E., Marks, A. R., Somlyo, A. V., Fleischer, S., and Somlyo, A. P. (1993). Anti-ryanodine receptor antibody binding sites in vascular and endocardial endothelium. Circ. Res. 72, 481–488. doi: 10.1161/01.RES.72.2.481
Li, Y., Baylie, R. L., Tavares, M. J., and Brayden, J. E. (2014). TRPM4 channels couple purinergic receptor mechanoactivation and myogenic tone development in cerebral parenchymal arterioles. J. Cereb. Blood Flow Metab. 34, 1706–1714. doi: 10.1038/jcbfm.2014.139
Li, Y., and Brayden, J. E. (2017). Rho kinase activity governs arteriolar myogenic depolarization. J. Cereb. Blood Flow Metab. 37, 140–152. doi: 10.1177/0271678X15621069
Li, C., Wang, X., Vais, H., Thompson, C. B., Foskett, J. K., and White, C. (2007). Apoptosis regulation by Bcl-x(L) modulation of mammalian inositol 1,4,5-trisphosphate receptor channel isoform gating. Proc. Natl. Acad. Sci. U. S. A. 104, 12565–12570. doi: 10.1073/pnas.0702489104
Liu, P. Y., Zhang, Z., Liu, Y., Tang, X. L., Shu, S., Bao, X. Y., et al. (2019). TMEM16A inhibition preserves blood-brain barrier integrity After ischemic stroke. Front. Cell. Neurosci. 13:360. doi: 10.3389/fncel.2019.00360
Liu, Q. H., Zheng, Y. M., Korde, A. S., Yadav, V. R., Rathore, R., Wess, J., et al. (2009). Membrane depolarization causes a direct activation of G protein-coupled receptors leading to local Ca2+ release in smooth muscle. Proc. Natl. Acad. Sci. U. S. A. 106, 11418–11423. doi: 10.1073/pnas.0813307106
Loirand, G., Guerin, P., and Pacaud, P. (2006). Rho kinases in cardiovascular physiology and pathophysiology. Circ. Res. 98, 322–334. doi: 10.1161/01.RES.0000201960.04223.3c
Lucchesi, P. A., Sabri, A., Belmadani, S., and Matrougui, K. (2004). Involvement of metalloproteinases 2/9 in epidermal growth factor receptor transactivation in pressure-induced myogenic tone in mouse mesenteric resistance arteries. Circulation 110, 3587–3593. doi: 10.1161/01.CIR.0000148780.36121.47
Luykenaar, K. D., El-Rahman, R. A., Walsh, M. P., and Welsh, D. G. (2009). Rho-kinase-mediated suppression of KDR current in cerebral arteries requires an intact actin cytoskeleton. Am. J. Physiol. Heart Circ. Physiol. 296, H917–H926. doi: 10.1152/ajpheart.01206.2008
Ma, M. M., Gao, M., Guo, K. M., Wang, M., Li, X. Y., Zeng, X. L., et al. (2017). TMEM16A contributes to endothelial dysfunction by facilitating Nox2 NADPH oxidase-derived reactive oxygen species generation in hypertension. Hypertension 69, 892–901. doi: 10.1161/HYPERTENSIONAHA.116.08874
Ma, Z., Lou, X. J., and Horrigan, F. T. (2006). Role of charged residues in the S1-S4 voltage sensor of BK channels. J. Gen. Physiol. 127, 309–328. doi: 10.1085/jgp.200509421
MacKay, C. E., Leo, M. D., Fernandez-Pena, C., Hasan, R., Yin, W., Mata-Daboin, A., et al. (2020). Intravascular flow stimulates PKD2 (polycystin-2) channels in endothelial cells to reduce blood pressure. elife 9:e56655. doi: 10.7554/eLife.56655
Mackie, A. R., Brueggemann, L. I., Henderson, K. K., Shiels, A. J., Cribbs, L. L., Scrogin, K. E., et al. (2008). Vascular KCNQ potassium channels as novel targets for the control of mesenteric artery constriction by vasopressin, based on studies in single cells, pressurized arteries, and in vivo measurements of mesenteric vascular resistance. J. Pharmacol. Exp. Ther. 325, 475–483. doi: 10.1124/jpet.107.135764
MacMillan, D., Currie, S., Bradley, K. N., Muir, T. C., and McCarron, J. G. (2005). In smooth muscle, FK506-binding protein modulates IP3 receptor-evoked Ca2+ release by mTOR and calcineurin. J. Cell Sci. 118, 5443–5451. doi: 10.1242/jcs.02657
Mahaut-Smith, M. P., Martinez-Pinna, J., and Gurung, I. S. (2008). A role for membrane potential in regulating GPCRs? Trends Pharmacol. Sci. 29, 421–429. doi: 10.1016/j.tips.2008.05.007
Marrelli, S. P. (2000). Selective measurement of endothelial or smooth muscle [Ca(2+)](i) in pressurized/perfused cerebral arteries with fura-2. J. Neurosci. Methods 97, 145–155. doi: 10.1016/S0165-0270(00)00176-X
Marrelli, S. P., Eckmann, M. S., and Hunte, M. S. (2003). Role of endothelial intermediate conductance KCa channels in cerebral EDHF-mediated dilations. Am. J. Physiol. Heart Circ. Physiol. 285, H1590–H1599. doi: 10.1152/ajpheart.00376.2003
Marrelli, S. P., O’Neil, R. G., Brown, R. C., and Bryan, R. M. Jr. (2007). PLA2 and TRPV4 channels regulate endothelial calcium in cerebral arteries. Am. J. Physiol. Heart Circ. Physiol. 292, H1390–H1397. doi: 10.1152/ajpheart.01006.2006
Martinez-Lemus, L. A., Crow, T., Davis, M. J., and Meininger, G. A. (2005). alphavbeta3- and alpha5beta1-integrin blockade inhibits myogenic constriction of skeletal muscle resistance arterioles. Am. J. Physiol. Heart Circ. Physiol. 289, H322–H329. doi: 10.1152/ajpheart.00923.2003
Matchkov, V. V., Secher Dam, V., Bodtkjer, D. M., and Aalkjaer, C. (2013). Transport and function of chloride in vascular smooth muscles. J. Vasc. Res. 50, 69–87. doi: 10.1159/000345242
Mathar, I., Vennekens, R., Meissner, M., Kees, F., Van der Mieren, G., Camacho Londono, J. E., et al. (2010). Increased catecholamine secretion contributes to hypertension in TRPM4-deficient mice. J. Clin. Invest. 120, 3267–3279. doi: 10.1172/JCI41348
Mauban, J. R., Zacharia, J., Fairfax, S., and Wier, W. G. (2015). PC-PLC/sphingomyelin synthase activity plays a central role in the development of myogenic tone in murine resistance arteries. Am. J. Physiol. Heart Circ. Physiol. 308, H1517–H1524. doi: 10.1152/ajpheart.00594.2014
McCobb, D. P., Fowler, N. L., Featherstone, T., Lingle, C. J., Saito, M., Krause, J. E., et al. (1995). A human calcium-activated potassium channel gene expressed in vascular smooth muscle. Am. J. Phys. 269, H767–H777. doi: 10.1152/ajpheart.1995.269.3.H767
McGahon, M. K., Dash, D. P., Arora, A., Wall, N., Dawicki, J., Simpson, D. A., et al. (2007). Diabetes Downregulates large-conductance Ca2+-activated potassium {beta}1 channel subunit in retinal arteriolar smooth muscle. Circ. Res. 100, 703–711. doi: 10.1161/01.RES.0000260182.36481.c9
McManus, O. B., Helms, L. M., Pallanck, L., Ganetzky, B., Swanson, R., and Leonard, R. J. (1995). Functional role of the beta subunit of high conductance calcium-activated potassium channels. Neuron 14, 645–650. doi: 10.1016/0896-6273(95)90321-6
Mederos, Y. S. M., Storch, U., and Gudermann, T. (2016). Mechanosensitive Gq/11 protein-coupled receptors mediate myogenic vasoconstriction. Microcirculation 23, 621–625. doi: 10.1111/micc.12293
Mederos y Schnitzler, M., Storch, U., Meibers, S., Nurwakagari, P., Breit, A., Essin, K., et al. (2008). Gq-coupled receptors as mechanosensors mediating myogenic vasoconstriction. EMBO J. 27, 3092–3103. doi: 10.1038/emboj.2008.233
Meera, P., Wallner, M., Jiang, Z., and Toro, L. (1996). A calcium switch for the functional coupling between alpha (hslo) and beta subunits (KV,Ca beta) of maxi K channels. FEBS Lett. 382, 84–88. doi: 10.1016/0014-5793(96)00151-2
Meera, P., Wallner, M., Song, M., and Toro, L. (1997). Large conductance voltage- and calcium-dependent K+ channel, a distinct member of voltage-dependent ion channels with seven N-terminal transmembrane segments (S0-S6), an extracellular N terminus, and an intracellular (S9-S10) C terminus. Proc. Natl. Acad. Sci. U. S. A. 94, 14066–14071. doi: 10.1073/pnas.94.25.14066
Mery, L., Magnino, F., Schmidt, K., Krause, K. H., and Dufour, J. F. (2001). Alternative splice variants of hTrp4 differentially interact with the C-terminal portion of the inositol 1,4,5-trisphosphate receptors. FEBS Lett. 487, 377–383. doi: 10.1016/S0014-5793(00)02362-0
Minami, T. (2014). Calcineurin-NFAT activation and DSCR-1 auto-inhibitory loop: how is homoeostasis regulated? J. Biochem. 155, 217–226. doi: 10.1093/jb/mvu006
Miriel, V. A., Mauban, J. R., Blaustein, M. P., and Wier, W. G. (1999). Local and cellular Ca2+ transients in smooth muscle of pressurized rat resistance arteries during myogenic and agonist stimulation. J. Physiol. 518, 815–824. doi: 10.1111/j.1469-7793.1999.0815p.x
Moreno-Dominguez, A., Colinas, O., El-Yazbi, A., Walsh, E. J., Hill, M. A., Walsh, M. P., et al. (2013). Ca2+ sensitization due to myosin light chain phosphatase inhibition and cytoskeletal reorganization in the myogenic response of skeletal muscle resistance arteries. J. Physiol. 591, 1235–1250. doi: 10.1113/jphysiol.2012.243576
Mountian, I. I., Baba-Aissa, F., Jonas, J. C., Humbert De, S., Wuytack, F., and Parys, J. B. (2001). Expression of Ca(2+) transport genes in platelets and endothelial cells in hypertension. Hypertension 37, 135–141. doi: 10.1161/01.HYP.37.1.135
Mountian, I., Manolopoulos, V. G., De Smedt, H., Parys, J. B., Missiaen, L., and Wuytack, F. (1999). Expression patterns of sarco/endoplasmic reticulum Ca(2+)-ATPase and inositol 1,4,5-trisphosphate receptor isoforms in vascular endothelial cells. Cell Calcium 25, 371–380. doi: 10.1054/ceca.1999.0034
Mufti, R. E., Brett, S. E., Tran, C. H., Abd El-Rahman, R., Anfinogenova, Y., El-Yazbi, A., et al. (2010). Intravascular pressure augments cerebral arterial constriction by inducing voltage-insensitive Ca2+ waves. J. Physiol. 588, 3983–4005. doi: 10.1113/jphysiol.2010.193300
Mufti, R. E., Zechariah, A., Sancho, M., Mazumdar, N., Brett, S. E., and Welsh, D. G. (2015). Implications of alphavbeta3 integrin Signaling in the regulation of Ca2+ waves and myogenic tone in cerebral arteries. Arterioscler. Thromb. Vasc. Biol. 35, 2571–2578. doi: 10.1161/ATVBAHA.115.305619
Munaron, L. (2006). Intracellular calcium, endothelial cells and angiogenesis. Recent Pat. Anticancer Drug Discov. 1, 105–119. doi: 10.2174/157489206775246502
Narayanan, D., Adebiyi, A., and Jaggar, J. H. (2012). Inositol trisphosphate receptors in smooth muscle cells. Am. J. Physiol. Heart Circ. Physiol. 302, H2190–H2210. doi: 10.1152/ajpheart.01146.2011
Narayanan, D., Bulley, S., Leo, M. D., Burris, S. K., Gabrick, K. S., Boop, F. A., et al. (2013). Smooth muscle cell transient receptor potential polycystin-2 (TRPP2) channels contribute to the myogenic response in cerebral arteries. J. Physiol. 591, 5031–5046. doi: 10.1113/jphysiol.2013.258319
Navedo, M. F., Amberg, G. C., Votaw, V. S., and Santana, L. F. (2005). Constitutively active L-type Ca2+ channels. Proc. Natl. Acad. Sci. U. S. A. 102, 11112–11117. doi: 10.1073/pnas.0500360102
Nelson, M. T., Cheng, H., Rubart, M., Santana, L. F., Bonev, A. D., Knot, H. J., et al. (1995). Relaxation of arterial smooth muscle by calcium sparks. Science 270, 633–637. doi: 10.1126/science.270.5236.633
Nemeth, Z., Hildebrandt, E., Ryan, M. J., Granger, J. P., and Drummond, H. A. (2020). Pressure-induced constriction of the middle cerebral artery is abolished in TrpC6 knockout mice. Am. J. Physiol. Heart Circ. Physiol. 319, H42–H50. doi: 10.1152/ajpheart.00126.2020
Nilius, B., and Droogmans, G. (2001). Ion channels and their functional role in vascular endothelium. Physiol. Rev. 81, 1415–1459. doi: 10.1152/physrev.2001.81.4.1415
Nilius, B., Prenen, J., Szucs, G., Wei, L., Tanzi, F., Voets, T., et al. (1997). Calcium-activated chloride channels in bovine pulmonary artery endothelial cells. J. Physiol. 498, 381–396. doi: 10.1113/jphysiol.1997.sp021865
Nilius, B., Vriens, J., Prenen, J., Droogmans, G., and Voets, T. (2004). TRPV4 calcium entry channel: a paradigm for gating diversity. Am. J. Phys. Cell Physiol. 286, C195–C205. doi: 10.1152/ajpcell.00365.2003
Nourian, Z., Li, M., Leo, M. D., Jaggar, J. H., Braun, A. P., and Hill, M. A. (2014). Large conductance Ca2+−activated K+ channel (BKCa) alpha-subunit splice variants in resistance arteries from rat cerebral and skeletal muscle vasculature. PLoS One 9:e98863. doi: 10.1371/journal.pone.0098863
Osol, G., Laher, I., and Kelley, M. (1993). Myogenic tone is coupled to phospholipase C and G protein activation in small cerebral arteries. Am. J. Phys. 265, H415–H420. doi: 10.1152/ajpheart.1993.265.1.H415
Patterson, R. L., van Rossum, D. B., Barrow, R. K., and Snyder, S. H. (2004). RACK1 binds to inositol 1,4,5-trisphosphate receptors and mediates Ca2+ release. Proc. Natl. Acad. Sci. U. S. A. 101, 2328–2332. doi: 10.1073/pnas.0308567100
Pires, P. W., Ko, E. A., Pritchard, H. A. T., Rudokas, M., Yamasaki, E., and Earley, S. (2017). The angiotensin II receptor type 1b is the primary sensor of intraluminal pressure in cerebral artery smooth muscle cells. J. Physiol. 595, 4735–4753. doi: 10.1113/JP274310
Post, J. M., Gelband, C. H., and Hume, J. R. (1995). [Ca2+]i inhibition of K+ channels in canine pulmonary artery. Novel mechanism for hypoxia-induced membrane depolarization. Circ. Res. 77, 131–139. doi: 10.1161/01.res.77.1.131
Qian, X., Francis, M., Kohler, R., Solodushko, V., Lin, M., and Taylor, M. S. (2014). Positive feedback regulation of agonist-stimulated endothelial Ca2+ dynamics by KCa3.1 channels in mouse mesenteric arteries. Arterioscler. Thromb. Vasc. Biol. 34, 127–135. doi: 10.1161/ATVBAHA.113.302506
Quinlan, K. L., Naik, S. M., Cannon, G., Armstrong, C. A., Bunnett, N. W., Ansel, J. C., et al. (1999). Substance P activates coincident NF-AT- and NF-kappa B-dependent adhesion molecule gene expression in microvascular endothelial cells through intracellular calcium mobilization. J. Immunol. 163, 5656–5665.
Reggiani, C., and te Kronnie, T. (2006). RyR isoforms and fibre type-specific expression of proteins controlling intracellular calcium concentration in skeletal muscles. J. Muscle Res. Cell Motil. 27, 327–335. doi: 10.1007/s10974-006-9076-3
Renkin, E. M. (1984). “Control of microcirculation and blood-tissue exchange,” in Handbook of Physiology: Sec. 2, The Cardiovascular System, Vol. IV, Microcirculation, Part 2. eds. E. M. Renkin and C. C. Michel (Bethesda,MD: American Physiological Society), 627–687.
Riddle, M. A., Hughes, J. M., and Walker, B. R. (2011). Role of caveolin-1 in endothelial BKCa channel regulation of vasoreactivity. Am. J. Phys. Cell Physiol. 301, C1404–C1414. doi: 10.1152/ajpcell.00013.2011
Rusko, J., Van Slooten, G., and Adams, D. J. (1995). Caffeine-evoked, calcium-sensitive membrane currents in rabbit aortic endothelial cells. Br. J. Pharmacol. 115, 133–141. doi: 10.1111/j.1476-5381.1995.tb16330.x
Salomone, S., Soydan, G., Moskowitz, M. A., and Sims, J. R. (2009). Inhibition of cerebral vasoconstriction by dantrolene and nimodipine. Neurocrit. Care. 10, 93–102. doi: 10.1007/s12028-008-9153-0
Sandow, S. L., and Grayson, T. H. (2009). Limits of isolation and culture: intact vascular endothelium and BKCa. Am. J. Physiol. Heart Circ. Physiol. 297, H1–H7. doi: 10.1152/ajpheart.00042.2009
Sandow, S. L., and Hill, C. E. (2000). Incidence of myoendothelial gap junctions in the proximal and distal mesenteric arteries of the rat is suggestive of a role in endothelium-derived hyperpolarizing factor-mediated responses. Circ. Res. 86, 341–346. doi: 10.1161/01.RES.86.3.341
Sandow, S. L., Neylon, C. B., Chen, M. X., and Garland, C. J. (2006). Spatial separation of endothelial small- and intermediate-conductance calcium-activated potassium channels (K(Ca)) and connexins: possible relationship to vasodilator function? J. Anat. 209, 689–698. doi: 10.1111/j.1469-7580.2006.00647.x
Schilling, W. P., Cabello, O. A., and Rajan, L. (1992). Depletion of the inositol 1,4,5-Trisphosphate-sensitive intracellular Ca2+ store in vascular endothelial-cells activates the agonist-sensitive Ca2+-influx pathway. Biochem. J. 284, 521–530. doi: 10.1042/bj2840521
Schleifenbaum, J., Kassmann, M., Szijarto, I. A., Hercule, H. C., Tano, J. Y., Weinert, S., et al. (2014). Stretch-activation of angiotensin II type 1a receptors contributes to the myogenic response of mouse mesenteric and renal arteries. Circ. Res. 115, 263–272. doi: 10.1161/CIRCRESAHA.115.302882
Schmidt, K., and de Wit, C. (2020). Endothelium-derived hyperpolarizing factor and Myoendothelial coupling: The in vivo perspective. Front. Physiol. 11:602930. doi: 10.3389/fphys.2020.602930
Schuster, A., Oishi, H., Beny, J. L., Stergiopulos, N., and Meister, J. J. (2001). Simultaneous arterial calcium dynamics and diameter measurements: application to myoendothelial communication. Am. J. Physiol. Heart Circ. Physiol. 280, H1088–H1096. doi: 10.1152/ajpheart.2001.280.3.H1088
Seo, M. D., Enomoto, M., Ishiyama, N., Stathopulos, P. B., and Ikura, M. (2015). Structural insights into endoplasmic reticulum stored calcium regulation by inositol 1,4,5-trisphosphate and ryanodine receptors. Biochim. Biophys. Acta 1853, 1980–1991. doi: 10.1016/j.bbamcr.2014.11.023
Seo, M. D., Velamakanni, S., Ishiyama, N., Stathopulos, P. B., Rossi, A. M., Khan, S. A., et al. (2012). Structural and functional conservation of key domains in InsP3 and ryanodine receptors. Nature 483, 108–112. doi: 10.1038/nature10751
Sforna, L., Megaro, A., Pessia, M., Franciolini, F., and Catacuzzeno, L. (2018). Structure, gating and basic functions of the Ca2+−activated K Channel of intermediate conductance. Curr. Neuropharmacol. 16, 608–617. doi: 10.2174/1570159X15666170830122402
Shah, V. N., Chagot, B., and Chazin, W. J. (2006). Calcium-dependent regulation of ion channels. Calcium Bind. Proteins 1, 203–212.
Sharif-Naeini, R., Folgering, J. H., Bichet, D., Duprat, F., Lauritzen, I., Arhatte, M., et al. (2009). Polycystin-1 and -2 dosage regulates pressure sensing. Cell 139, 587–596. doi: 10.1016/j.cell.2009.08.045
Sharma, N. R., and Davis, M. J. (1994). Mechanism of substance P-induced hyperpolarization of porcine coronary artery endothelial cells. Am. J. Phys. 266, H156–H164. doi: 10.1152/ajpheart.1994.266.1.H156
Sharma, N. R., and Davis, M. J. (1995). Substance-P-induced calcium-entry in endothelial-cells is secondary to depletion of intracellular stores. Am. J. Phys. Heart Circ. Phys. 268, H962–H973. doi: 10.1152/ajpheart.1995.268.3.H962
Si, H., Heyken, W. T., Wolfle, S. E., Tysiac, M., Schubert, R., Grgic, I., et al. (2006). Impaired endothelium-derived hyperpolarizing factor-mediated dilations and increased blood pressure in mice deficient of the intermediate-conductance Ca2+−activated K+ channel. Circ. Res. 99, 537–544. doi: 10.1161/01.RES.0000238377.08219.0c
Skofic Maurer, D., Zabini, D., Nagaraj, C., Sharma, N., Lengyel, M., Nagy, B. M., et al. (2020). Endothelial dysfunction following enhanced TMEM16A activity in human pulmonary arteries. Cell 9:1984. doi: 10.3390/cells9091984
Slish, D. F., Welsh, D. G., and Brayden, J. E. (2002). Diacylglycerol and protein kinase C activate cation channels involved in myogenic tone. Am. J. Physiol. Heart Circ. Physiol. 283, H2196–H2201. doi: 10.1152/ajpheart.00605.2002
Socha, M. J., Domeier, T. L., Behringer, E. J., and Segal, S. S. (2012). Coordination of intercellular Ca(2+) signaling in endothelial cell tubes of mouse resistance arteries. Microcirculation 19, 757–770. doi: 10.1111/micc.12000
Socha, M. J., Hakim, C. H., Jackson, W. F., and Segal, S. S. (2011). Temperature effects on morphological integrity and Ca(2)(+) signaling in freshly isolated murine feed artery endothelial cell tubes. Am. J. Physiol. Heart Circ. Physiol. 301, H773–H783. doi: 10.1152/ajpheart.00214.2011
Sonkusare, S. K., Bonev, A. D., Ledoux, J., Liedtke, W., Kotlikoff, M. I., Heppner, T. J., et al. (2012). Elementary Ca2+ signals through endothelial TRPV4 channels regulate vascular function. Science 336, 597–601. doi: 10.1126/science.1216283
Sonkusare, S. K., Dalsgaard, T., Bonev, A. D., Hill-Eubanks, D. C., Kotlikoff, M. I., Scott, J. D., et al. (2014). AKAP150-dependent cooperative TRPV4 channel gating is central to endothelium-dependent vasodilation and is disrupted in hypertension. Sci. Signal. 7:ra66. doi: 10.1126/scisignal.2005052
Stevenson, A. S., Cartin, L., Wellman, T. L., Dick, M. H., Nelson, M. T., and Lounsbury, K. M. (2001). Membrane depolarization mediates phosphorylation and nuclear translocation of CREB in vascular smooth muscle cells. Exp. Cell Res. 263, 118–130. doi: 10.1006/excr.2000.5107
Storch, U., Blodow, S., Gudermann, T., and Mederos, Y. S. M. (2015). Cysteinyl leukotriene 1 receptors as novel mechanosensors mediating myogenic tone together with angiotensin II type 1 receptors-brief report. Arterioscler. Thromb. Vasc. Biol. 35, 121–126. doi: 10.1161/ATVBAHA.114.304844
Straub, A. C., Billaud, M., Johnstone, S. R., Best, A. K., Yemen, S., Dwyer, S. T., et al. (2011). Compartmentalized connexin 43 s-nitrosylation/denitrosylation regulates heterocellular communication in the vessel wall. Arterioscler. Thromb. Vasc. Biol. 31, 399–407. doi: 10.1161/ATVBAHA.110.215939
Straub, A. C., Zeigler, A. C., and Isakson, B. E. (2014). The myoendothelial junction: connections that deliver the message. Physiology (Bethesda) 29, 242–249. doi: 10.1152/physiol.00042.2013
Strotmann, R., Schultz, G., and Plant, T. D. (2003). Ca2+−dependent potentiation of the nonselective cation channel TRPV4 is mediated by a C-terminal calmodulin binding site. J. Biol. Chem. 278, 26541–26549. doi: 10.1074/jbc.M302590200
Suzuki, T., Suzuki, Y., Asai, K., Imaizumi, Y., and Yamamura, H. (2021). Hypoxia increases the proliferation of brain capillary endothelial cells via upregulation of TMEM16A Ca(2+)-activated Cl(−) channels. J. Pharmacol. Sci. 146, 65–69. doi: 10.1016/j.jphs.2021.03.002
Suzuki, T., Yasumoto, M., Suzuki, Y., Asai, K., Imaizumi, Y., and Yamamura, H. (2020). TMEM16A Ca(2+)-activated Cl(−) channel regulates the proliferation and migration of brain capillary endothelial cells. Mol. Pharmacol. 98, 61–71. doi: 10.1124/mol.119.118844
Tajada, S., Cidad, P., Colinas, O., Santana, L. F., Lopez-Lopez, J. R., and Perez-Garcia, M. T. (2013). Down-regulation of CaV1.2 channels during hypertension: how fewer CaV1.2 channels allow more Ca(2+) into hypertensive arterial smooth muscle. J. Physiol. 591, 6175–6191. doi: 10.1113/jphysiol.2013.265751
Taylor, M. S., Bonev, A. D., Gross, T. P., Eckman, D. M., Brayden, J. E., Bond, C. T., et al. (2003). Altered expression of small-conductance Ca2+−activated K+ (SK3) channels modulates arterial tone and blood pressure. Circ. Res. 93, 124–131. doi: 10.1161/01.RES.0000081980.63146.69
Taylor, C. W., Tovey, S. C., Rossi, A. M., Lopez Sanjurjo, C. I., Prole, D. L., and Rahman, T. (2014). Structural organization of signalling to and from IP3 receptors. Biochem. Soc. Trans. 42, 63–70. doi: 10.1042/BST20130205
Tran, C. H., Taylor, M. S., Plane, F., Nagaraja, S., Tsoukias, N. M., Solodushko, V., et al. (2012). Endothelial Ca2+ wavelets and the induction of myoendothelial feedback. Am. J. Phys. Cell Physiol. 302, C1226–C1242. doi: 10.1152/ajpcell.00418.2011
Tseng-Crank, J., Godinot, N., Johansen, T. E., Ahring, P. K., Strobaek, D., Mertz, R., et al. (1996). Cloning, expression, and distribution of a Ca(2+)-activated K+ channel beta-subunit from human brain. Proc. Natl. Acad. Sci. U. S. A. 93, 9200–9205. doi: 10.1073/pnas.93.17.9200
Tu, H., Wang, Z., and Bezprozvanny, I. (2005). Modulation of mammalian inositol 1,4,5-trisphosphate receptor isoforms by calcium: a role of calcium sensor region. Biophys. J. 88, 1056–1069. doi: 10.1529/biophysj.104.049601
Tu, J. C., Xiao, B., Yuan, J. P., Lanahan, A. A., Leoffert, K., Li, M., et al. (1998). Homer binds a novel proline-rich motif and links group 1 metabotropic glutamate receptors with IP3 receptors. Neuron 21, 717–726. doi: 10.1016/S0896-6273(00)80589-9
Tumelty, J., Scholfield, N., Stewart, M., Curtis, T., and McGeown, G. (2007). Ca2+−sparks constitute elementary building blocks for global Ca2+−signals in myocytes of retinal arterioles. Cell Calcium 41, 451–466. doi: 10.1016/j.ceca.2006.08.005
Tuttle, J. L., and Falcone, J. C. (2001). Nitric oxide release during alpha1-adrenoceptor-mediated constriction of arterioles. Am. J. Physiol. Heart Circ. Physiol. 281, H873–H881. doi: 10.1152/ajpheart.2001.281.2.H873
Tykocki, N. R., Boerman, E. M., and Jackson, W. F. (2017). Smooth muscle ion channels and regulation of vascular tone in resistance arteries and arterioles. Compr. Physiol. 7, 485–581. doi: 10.1002/cphy.c160011
Ungvari, Z., Csiszar, A., and Koller, A. (2002). Increases in endothelial Ca(2+) activate K(Ca) channels and elicit EDHF-type arteriolar dilation via gap junctions. Am. J. Physiol. Heart Circ. Physiol. 282, H1760–H1767. doi: 10.1152/ajpheart.00676.2001
Urena, J., del Valle-Rodriguez, A., and Lopez-Barneo, J. (2007). Metabotropic Ca2+ channel-induced calcium release in vascular smooth muscle. Cell Calcium 42, 513–520. doi: 10.1016/j.ceca.2007.04.010
Vaithianathan, T., Narayanan, D., Asuncion-Chin, M. T., Jeyakumar, L. H., Liu, J., Fleischer, S., et al. (2010). Subtype identification and functional characterization of ryanodine receptors in rat cerebral artery myocytes. Am. J. Phys. Cell Physiol. 299, C264–C278. doi: 10.1152/ajpcell.00318.2009
Vallot, O., Combettes, L., Jourdon, P., Inamo, J., Marty, I., Claret, M., et al. (2000). Intracellular Ca(2+) handling in vascular smooth muscle cells is affected by proliferation. Arterioscler. Thromb. Vasc. Biol. 20, 1225–1235. doi: 10.1161/01.ATV.20.5.1225
Valverde, M. A., Rojas, P., Amigo, J., Cosmelli, D., Orio, P., Bahamonde, M. I., et al. (1999). Acute activation of maxi-K channels (hSlo) by estradiol binding to the beta subunit. Science 285, 1929–1931. doi: 10.1126/science.285.5435.1929
Van Petegem, F. (2015). Ryanodine receptors: allosteric ion channel giants. J. Mol. Biol. 427, 31–53. doi: 10.1016/j.jmb.2014.08.004
VanLandingham, L. G., Gannon, K. P., and Drummond, H. A. (2009). Pressure-induced constriction is inhibited in a mouse model of reduced betaENaC. Am. J. Phys. Regul. Integr. Comp. Phys. 297, R723–R728. doi: 10.1152/ajpregu.00212.2009
Vazquez, G., Bird, G. S., Mori, Y., and Putney, J. W. Jr. (2006). Native TRPC7 channel activation by an inositol trisphosphate receptor-dependent mechanism. J. Biol. Chem. 281, 25250–25258. doi: 10.1074/jbc.M604994200
Wang, Q., Leo, M. D., Narayanan, D., Kuruvilla, K. P., and Jaggar, J. H. (2016). Local coupling of TRPC6 to ANO1/TMEM16A channels in smooth muscle cells amplifies vasoconstriction in cerebral arteries. Am. J. Phys. Cell Physiol. 310, C1001–C1009. doi: 10.1152/ajpcell.00092.2016
Wang, X. L., Ye, D., Peterson, T. E., Cao, S., Shah, V. H., Katusic, Z. S., et al. (2005). Caveolae targeting and regulation of large conductance Ca(2+)-activated K+ channels in vascular endothelial cells. J. Biol. Chem. 280, 11656–11664. doi: 10.1074/jbc.M410987200
Welsh, D. G., Morielli, A. D., Nelson, M. T., and Brayden, J. E. (2002). Transient receptor potential channels regulate myogenic tone of resistance arteries. Circ. Res. 90, 248–250. doi: 10.1161/hh0302.105662
Westcott, E. B., Goodwin, E. L., Segal, S. S., and Jackson, W. F. (2012). Function and expression of ryanodine receptors and inositol 1,4,5-trisphosphate receptors in smooth muscle cells of murine feed arteries and arterioles. J. Physiol. 590, 1849–1869. doi: 10.1113/jphysiol.2011.222083
Westcott, E. B., and Jackson, W. F. (2011). Heterogeneous function of ryanodine receptors, but not IP3 receptors, in hamster cremaster muscle feed arteries and arterioles. Am. J. Physiol. Heart Circ. Physiol. 300, H1616–H1630. doi: 10.1152/ajpheart.00728.2010
Wilson, A. J., Jabr, R. I., and Clapp, L. H. (2000). Calcium modulation of vascular smooth muscle ATP-sensitive K(+) channels: role of protein phosphatase-2B. Circ. Res. 87, 1019–1025. doi: 10.1161/01.RES.87.11.1019
Wolpe, A. G., Ruddiman, C. A., Hall, P. J., and Isakson, B. E. (2021). Polarized proteins in endothelium and their contribution to function. J. Vasc. Res. 58, 65–91. doi: 10.1159/000512618
Wu, M. M., Lou, J., Song, B. L., Gong, Y. F., Li, Y. C., Yu, C. J., et al. (2014). Hypoxia augments the calcium-activated chloride current carried by anoctamin-1 in cardiac vascular endothelial cells of neonatal mice. Br. J. Pharmacol. 171, 3680–3692. doi: 10.1111/bph.12730
Xia, X. M., Fakler, B., Rivard, A., Wayman, G., Johnson-Pais, T., Keen, J. E., et al. (1998). Mechanism of calcium gating in small-conductance calcium-activated potassium channels. Nature 395, 503–507. doi: 10.1038/26758
Yamamura, H., Ohya, S., Muraki, K., and Imaizumi, Y. (2012). Involvement of inositol 1,4,5-trisphosphate formation in the voltage-dependent regulation of the Ca(2+) concentration in porcine coronary arterial smooth muscle cells. J. Pharmacol. Exp. Ther. 342, 486–496. doi: 10.1124/jpet.112.194233
Yan, J., and Aldrich, R. W. (2010). LRRC26 auxiliary protein allows BK channel activation at resting voltage without calcium. Nature 466, 513–516. doi: 10.1038/nature09162
Yan, Z., Bai, X., Yan, C., Wu, J., Li, Z., Xie, T., et al. (2015). Structure of the rabbit ryanodine receptor RyR1 at near-atomic resolution. Nature 517, 50–55. doi: 10.1038/nature14063
Yang, X. R., Lin, M. J., Yip, K. P., Jeyakumar, L. H., Fleischer, S., Leung, G. P., et al. (2005). Multiple ryanodine receptor subtypes and heterogeneous ryanodine receptor-gated Ca2+ stores in pulmonary arterial smooth muscle cells. Am. J. Phys. Lung Cell. Mol. Phys. 289, L338–L348. doi: 10.1152/ajplung.00328.2004
Yang, Y., Murphy, T. V., Ella, S. R., Grayson, T. H., Haddock, R., Hwang, Y. T., et al. (2009). Heterogeneity in function of small artery smooth muscle BKCa: involvement of the beta1-subunit. J. Physiol. 587, 3025–3044. doi: 10.1113/jphysiol.2009.169920
Yang, Y., Sohma, Y., Nourian, Z., Ella, S. R., Li, M., Stupica, A., et al. (2013). Mechanisms underlying regional differences in the Ca2+ sensitivity of BK(Ca) current in arteriolar smooth muscle. J. Physiol. 591, 1277–1293. doi: 10.1113/jphysiol.2012.241562
Yuan, J. P., Kiselyov, K., Shin, D. M., Chen, J., Shcheynikov, N., Kang, S. H., et al. (2003). Homer binds TRPC family channels and is required for gating of TRPC1 by IP3 receptors. Cell 114, 777–789. doi: 10.1016/S0092-8674(03)00716-5
Zacharia, J., Zhang, J., and Wier, W. G. (2007). Ca2+ signaling in mouse mesenteric small arteries: myogenic tone and adrenergic vasoconstriction. Am. J. Physiol. Heart Circ. Physiol. 292, H1523–H1532. doi: 10.1152/ajpheart.00670.2006
Zalk, R., Clarke, O. B., des Georges, A., Grassucci, R. A., Reiken, S., Mancia, F., et al. (2015). Structure of a mammalian ryanodine receptor. Nature 517, 44–49. doi: 10.1038/nature13950
Zhang, L., Papadopoulos, P., and Hamel, E. (2013). Endothelial TRPV4 channels mediate dilation of cerebral arteries: impairment and recovery in cerebrovascular pathologies related to Alzheimer's disease. Br. J. Pharmacol. 170, 661–670. doi: 10.1111/bph.12315
Zheng, X., Zinkevich, N. S., Gebremedhin, D., Gauthier, K. M., Nishijima, Y., Fang, J., et al. (2013). Arachidonic acid-induced dilation in human coronary arterioles: convergence of signaling mechanisms on endothelial TRPV4-mediated Ca2+ entry. J. Am. Heart Assoc. 2:e000080. doi: 10.1161/JAHA.113.000080
Zhong, N., Fang, Q. Z., Zhang, Y., and Zhou, Z. N. (2000). Volume- and calcium-activated chloride channels in human umbilical vein endothelial cells. Acta Pharmacol. Sin. 21, 215–220.
ZhuGe, R., Sims, S. M., Tuft, R. A., Fogarty, K. E., and Walsh, J. V. Jr. (1998). Ca2+ sparks activate K+ and Cl- channels, resulting in spontaneous transient currents in Guinea-pig tracheal myocytes. J. Physiol. 513, 711–718. doi: 10.1111/j.1469-7793.1998.711ba.x
Keywords: ion channels, calcium ions, arterioles, microcirculation, vascular smooth muscle, endothelial cells
Citation: Jackson WF (2021) Calcium-Dependent Ion Channels and the Regulation of Arteriolar Myogenic Tone. Front. Physiol. 12:770450. doi: 10.3389/fphys.2021.770450
Edited by:
Yoshiaki Suzuki, Nagoya City University, JapanReviewed by:
Steven S. Segal, University of Missouri, United StatesM. Teresa Perez-Garcia, University of Valladolid, Spain
Copyright © 2021 Jackson. This is an open-access article distributed under the terms of the Creative Commons Attribution License (CC BY). The use, distribution or reproduction in other forums is permitted, provided the original author(s) and the copyright owner(s) are credited and that the original publication in this journal is cited, in accordance with accepted academic practice. No use, distribution or reproduction is permitted which does not comply with these terms.
*Correspondence: William F. Jackson, amFja3M3ODNAbXN1LmVkdQ==