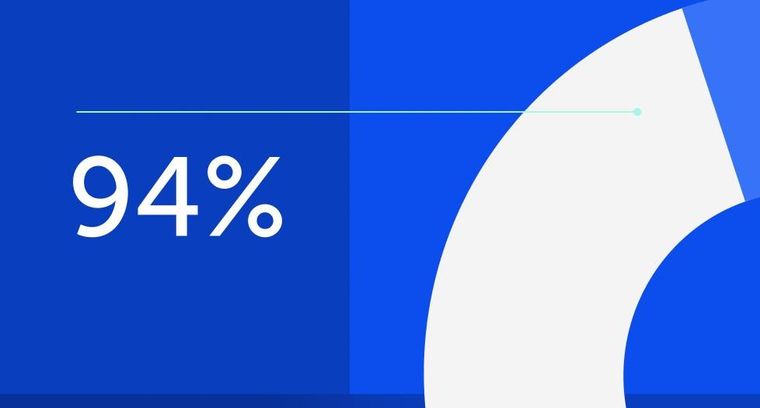
94% of researchers rate our articles as excellent or good
Learn more about the work of our research integrity team to safeguard the quality of each article we publish.
Find out more
ORIGINAL RESEARCH article
Front. Physiol., 28 October 2021
Sec. Gastrointestinal Sciences
Volume 12 - 2021 | https://doi.org/10.3389/fphys.2021.770430
Background: Motilin increases left gastric artery (LGA) blood flow in dogs via the endothelial motilin receptor (MLNR). This article investigates the signaling pathways of endothelial MLNR.
Methods: Motilin-induced relaxation of LGA rings was assessed using wire myography. Nitric oxide (NO), and cyclic guanosine monophosphate (cGMP) levels were measured using an NO assay kit and cGMP ELISA kit, respectively.
Results: Motilin concentration-dependently (EC50=9.1±1.2×10−8M) relaxed LGA rings precontracted with U46619 (thromboxane A2 receptor agonist). GM-109 (MLNR antagonist) significantly inhibited motilin-induced LGA relaxation and the production of NO and cGMP. N-ethylmaleimide (NEM; G-protein antagonist), U73122 [phospholipase C (PLC) inhibitor], and 2-aminoethyl diphenylborinate [2-APB; inositol trisphosphate (IP3) blocker] partially or completely blocked vasorelaxation. In contrast, chelerythrine [protein kinase C (PKC) inhibitor] and H89 [protein kinase A (PKA) inhibitor] had no such effect. Low-calcium or calcium-free Krebs solutions also reduced vasorelaxation. N-nitro-L-arginine methyl ester [L-NAME; nitric oxide synthase (NOS) inhibitor] and ODQ [soluble guanylyl cyclase (sGC) inhibitor] completely abolished vasodilation and synthesis of NO and cGMP. Indomethacin (cyclooxygenase inhibitor), 18α-glycyrrhetinic acid [18α-GA; myoendothelial gap junction (MEGJ) inhibitor], and K+ channel inhibition through high K+ concentrations or tetraethylammonium (TEA-Cl; KCa channel blocker) partially decreased vasorelaxation, whereas glibenclamide (KATP channel blocker) had no such effect.
Conclusion: The current study suggests that motilin-induced LGA relaxation is dependent on endothelial MLNR through the G protein-PLC-IP3 pathway and Ca2+ influx. The NOS-NO-sGC-cGMP pathway, prostacyclin, MEGJ, and K+ channels (especially KCa) are involved in endothelial-dependent relaxation of vascular smooth muscle (VSM) cells.
Motilin is a 22-amino acid intestinal peptide and an endogenous ligand of the motilin receptor (MLNR). It is cyclically released during the interdigestive period, but this pattern is halted in response to a meal. Motilin induces gastric phase III of the migrating motor complex (MMC III) in fasting humans and dogs (Lee et al., 1983; Ogawa et al., 2011; Deloose et al., 2015; Kitazawa and Kaiya, 2019), and also simultaneously induces a sustained increase in blood flow (≤ 240% resting blood flow) of the left gastric artery (LGA; Jin et al., 2002). The expression of MLNR has recently been found on the membrane of endothelial cells (ECs) in canine gastrointestinal arteries (Yang et al., 2021). However, the signal transduction pathways by which motilin induces vascular smooth muscle (VSM) relaxation is unclear.
Known functional MLNR expression sites include the myenteric plexus (Ohshiro et al., 2008; He et al., 2015) and gastrointestinal smooth muscle (Miller et al., 2000a,b). Imporantly, motilin peptide fragments have a greater affinity to neuronal tissue compared to muscle tissue (Poitras et al., 1996; Miller et al., 2000a,b), and the activation of different downstream effector molecules by the same receptors in different cell types may vary (Dass et al., 2003). MLNR mRNA or protein expression has also been found on tissues outside of the gastrointestinal tract, such as the hypothalamus and medulla oblongata (Suzuki et al., 2012), the thyroid gland and bone marrow (Feighner et al., 1999), and the lacrimal glands (Sadig et al., 2021). Yet, the physiological function of this expression is not fully understood. However, endothelial MLNR is the molecular basis to allow motilin to regulate gastric artery blood flow in dogs under physiological conditions (Jin et al., 2002; Yang et al., 2021). Thus, it is of physiological and pathophysiological significance to study its signal transduction pathway.
Human MLNR belongs to the class I G protein-coupled receptor (GPCR) family (Feighner et al., 1999). The activation of MLNR on rabbit gastrointestinal smooth muscle cells (SMCs) by its agonist causes Ca2+ release from intracellular stores via the Gq-phospholipase C-inositol trisphosphate (Gq-PLC-IP3) pathway (Depoortere and Peeters, 1995). Although the potency for agonists at the dog MLNR is lower than at humans (Leming et al., 2011), the protein sequence of MLNR in dogs is highly homologous to that in humans and rabbits (71 and 72% sequence identity, respectively; Ohshiro et al., 2008), which has only one variant. Therefore, the MLNR-coupled G protein pathway in ECs of the LGA is properly consistent with that in gastrointestinal SMCs.
Motilin-induced LGA relaxation involves cooperation between ECs and SMCs (Yang et al., 2021). Endothelium-dependent relaxation is achieved through a combination of endothelium-derived prostacyclin (PGI2), nitric oxide (NO), and endothelium-derived hyperpolarizing factor (EDHF) by different mechanisms (Kukovetz et al., 1979; Carvajal et al., 2000; Félétou, 2016). The NO-soluble guanylyl cyclase (sGC)-cyclic guanosine monophosphate (cGMP) pathway is essential for the control of vascular homeostasis, especially in elastic arteries (Hiroaki et al., 1996; Leloup et al., 2015). The contribution of PGI2 may be negligible in different sized blood vessels; however, there is a compensatory upregulation of PG synthesis when NO-mediated regulation is impaired (Sun et al., 2006). In addition, the importance of the hyperpolarizing mechanism increases as the vessel size decreases (Hiroaki et al., 1996). In the mesenteric arteries of dogs, [Leu13]motilin-induced vasorelaxation was inhibited by Nω-nitro-L-arginine [10−4M; inhibitor of nitric oxide synthase (NOS)], which confirmed the participation of endothelial NO, whereas a high dose of GM-109 (10−4M; a MLNR antagonist) only slightly decreased the relaxation, which excluded the role of MLNR (Iwai et al., 1998). However, the fact that GM-109 could inhibit motilin-induced relaxation of dog LGA both in vivo and in vitro (Jin et al., 2002; Yang et al., 2021) and that motilin induced endothelium-dependent relaxation of dog gastrointestinal arteries (Yang et al., 2021) suggests the irreplaceable roles of both endothelial MLNR and relaxation mediators.
The present work focuses on MLNR, G protein-coupled pathways, and endothelialderived relaxation mediators in the relaxation of LGA rings induced by motilin in vitro through the use of specific inhibitors or blockers. Furthermore, the NO and cGMP levels in LGA tissues were also examined to elucidate the essential role of the NO system.
This study was conducted in accordance with the Guide for the Care and Use of Laboratory Animals of the National Institutes of Health (NIH Publications No. 8023, revised in 1978). The study protocol was approved by the Animal Care and Use Committee of Jilin University (Permit No. 2016301). All efforts were made to minimize the discomfort of experimental animals. Ninety-six adult mongrel dogs of both sexes (age, 1.5–5.0years; weight, 15–30kg) were used. These purpose-bred mixed-breed animals were used by medical students from the General Theory of Surgery course to practice cutting and suturing the great saphenous vein and trachea.
The dogs were anesthetized with intravenous sodium pentobarbital (30mg/kg), and LGAs (1.8–2.2mm in diameter) were isolated and collected. The animals were euthanized, and tissues were immediately washed with ice-cold gassed (95% O2 and 5% CO2) and modified Krebs–Henseleit bicarbonate buffer (Krebs solution: 118.0mM NaCl, 4.7mM KCl, 2.5mM CaCl2, 1.2mM KH2PO4, 1.2mM MgSO4, 25mM NaHCO3, and 10mM glucose, pH 7.4; Iwai et al., 1998). Connective tissue and fat were carefully removed under a dissecting microscope (SZ61, Olympus, Japan), while avoiding over-pulling and clamping.
Porcine motilin (Peptide Institute Inc., Osaka, Japan), acetylcholine chloride (ACh; Sigma, Shanghai, China), and ethylene glycol tetra-acetic acid (EGTA; Sigma) were dissolved in distilled water. U46619 (Sigma) was dissolved in 96% ethanol to 0.4mM (stock solution) and diluted with distilled water before use. Concentrations refer to the final concentration of the drugs in the organ bath and are expressed as mol L−1 (M). Dimethyl sulfoxide (DMSO, Solarbio, Beijing, China) and ethanol concentrations in the organ bath were<0.4 and 0.1% (v/v), respectively, and caused no changes in vascular tone. Information regarding all inhibitors/blockers used are listed in Table 1.
Each LGA (approximately 18–30mm long) was cut into 6–10 rings (3mm in length). The samples were mounted between two L-shaped stainless-steel hooks (300μm in diameter) in the organ bath of a multi-wire myograph system (DMT620; Danish Myo Technology, Aarhus, Denmark). The organ bath contained 5ml of Krebs solution continuously gassed with 95% O2 and 5% CO2. The temperature and pH of the buffer were maintained at 37°C and 7.4. Vascular tension was recorded using LabChart Data Acquisition Software (LabChart 8.0; AD Instruments, New South Wales, Australia). The arterial rings were passively stretched to a tension of approximately 15–20mN [the optimal initial tension was determined in previous experiments (data not shown)], maintained under tension for approximately 60min, and washed every 15min. After tension was stabilized, tissue viability was assessed in 60mM KCl (by replacing NaCl with an equimolar amount of KCl in the Krebs solution) before each experiment.
The rings were contracted using U46619 (5×10−8M; thromboxane A2 analog). Once the U46619-induced tension remained constant, motilin was added to measure its relaxation effect. Only one concentration of motilin was added to each assay to avoid tachyphylaxis (Mitselos et al., 2007). Endothelium-intact rings were incubated with different inhibitors/blockers for 15–40min before treatment with U46619 (Table 1). In the Ca2+-free Krebs solution, Ca2+ was replaced with 10−3M EGTA. Endothelial integrity and endothelial removal were verified using acetylcholine (10−5M) at the end of each test, corresponding to a relaxation rate (RR) of >80 or<10%, respectively.
Relaxation rates were expressed as a percentage decrease in the tension induced by U46619 according to the following formula:
For the calculation of the inhibition rate (IR), tension was normalized to the corresponding values of the control group using the formula:
where T is sustained taension, L is the lowest tension, i represents the inhibitor group, and c represents the control group.
Concentration-response curves were analyzed using nonlinear regression analysis with variable slopes in GraphPad Prism version 9 (San Diego, California, United States). The x% effective concentration (ECx) and Hill slope were calculated automatically.
Left gastric artery tissues were collected and homogenized as described previously (Schachter, 2007). Tissues from three dogs were pooled, and the experiment was repeated three times. NO and cGMP levels were measured without any drugs (blank group) or following treatment with motilin (9×10−8M; motilin group). In certain experimental groups, tissues were incubated with GM-109 (10−5M) and L-NAME (10−4 M; for detecting NO and cGMP), and ODQ (10−5 M; for measuring cGMP) before treatment with motilin. The acetylcholine (10−5M) group was used to confirm the viability of ECs.
Tissues were homogenized in a solution containing 10.0mM Tris-HCl, 0.1mM EDTA-2Na, 10mM sucrose, and 136.7mM NaCl (pH 7.4; weight to volume ratio of 1:9). The homogenate was centrifuged at 2,400×g (5,000rpm) for 10min at 4°C, and the supernatant was assayed. Protein concentration was measured with a bicinchoninic acid total protein assay kit (A045-3; Jiancheng, Nanjing, China) using bovine serum albumin as the standard, and the results were expressed as μmol ml−1. NO concentrations were determined using a nitric oxide assay kit (S0023; Beyotime, Haimen, China) and expressed as μmol per g of protein. cGMP concentrations were measured using a canine cGMP ELISA kit (Cat No. ela05471Ca, SANCHEZ, Colorado, United States) and expressed as pmol per mg of protein.
Data were expressed as means±SEM. Changes in RR were analyzed using one-way ANOVA (Brown-Forsythe and Welch ANOVA tests for non-matched and paired data, and Dunnett T3 multiple comparisons test for multiple comparisons between the treatment groups), where n corresponds to the number of dogs. One LGA ring was obtained from each dog for analysis. Changes in RR induced by motilin (at EC50) with and without inhibitors were analyzed using a two-tailed Student’s paired t-test, where n represents the number of dogs. Two LGA rings from each dog were incubated with and without inhibitors, respectively. Changes in RR induced by motilin (at EC50) in Krebs solution containing different Ca2+ concentrations ([Ca2+]) were analyzed using one-way ANOVA (Geisser-Greenhouse correction for matched data, and the Holm-Sidak method for multiple comparisons between the treatment groups), where n corresponds to the number of dogs. Four LGA rings from each dog were incubated with 2.5×10−3, 1.25×10−3, 0.625×10−3, and 0M of Ca2+, respectively. Changes in the levels of NO and cGMP under different treatment conditions (i.e., motilin, acetylcholine, and motilin with inhibitors) were analyzed using one-way ANOVA (Brown-Forsythe and Welch ANOVA tests for non-matched and paired data, and Dunnett T3 multiple comparisons test for comparisons between the treatment groups and the motilin or control group), where n represents the number of repetitions. LGA tissues from three dogs were pooled in each experiment. Values of p less than 0.05 in the t-test or ANOVA were considered statistically significant.
The concentration-response curve of motilin (10−9–10−5M) showed a classic inverted “S” shape on a semi-logarithmic plot (n=7; Figure 1; Supplementary Table 1). The EC10, EC50, and EC90 were 9.4×10−9±2.9×10−9M, 9.1×10−8±1.2×10−8M, and 8.8×10−7±3.4×10−7M, respectively, and the Hill slope was 1.0±0.1. These results indicate that motilin induces relaxation of LGA rings precontracted by U46619 in a concentration-dependent manner in the range from 1×10−8 to 7×10−7M. The EC50 of motilin (9×10−8M) was used in subsequent experiments.
Figure 1. Concentration-response curve of motilin-induced relaxation of LGA and representative traces. (A) Motilin induces LGA relaxation in dogs in a concentration-dependent manner. Values are means±SEM (n=7). The dotted line shows the EC10, EC50, and EC90 on the concentration-response curve. (B) Motilin concentrations in the traces correspond to the red dots in panel (A). The arrows indicate the time of drug administration. ACh, acetylcholine; EC, effective concentration; LGA, left gastric artery; MTL, motilin; and SEM, standard error of the mean; U46619, thromboxane A2 receptor agonist.
The role of the MLNR on vasorelaxation was evaluated using the MLNR antagonist GM-109 (Tankanashi et al., 1995). The results showed that GM-109 (10−10–10−5M) inhibited LGA relaxation induced by motilin (9×10−8M) in a concentration-dependent manner, and its concentration-response curve showed an inverted “S” shape (Figure 2A). The IC50 was 7.8×10−8±0.6×10−8M, the IC90 was 1.1×10−6±0.2×10−6M, and the Hill slope was 0.8±0.1. The RRs of LGA induced by motilin (9×10−8M) before and after treatment with GM-109 were compared using a paired t-test (n=7; Supplementary Table 2). A higher concentration of GM-109 (10−6M, equivalent to IC90) significantly inhibited vasorelaxation, with IRs ranging from 77.7%±2.8 to 89.3%±2.1% (Figure 2B). The RRs before and after treatment with GM-109 (10−6M) were compared using a paired t-test (n=7; Supplementary Table 3). Representative traces are shown in Figure 2C.
Figure 2. Role of the MLNR on motilin-induced relaxation of the LGA in dogs. (A) Concentration-response curve of the inhibition of motilin-induced relaxation of LGA rings by GM-109. Data are means±SEM (n=7). The motilin concentration used in the assay was 9×10−8M. The dotted line shows IC50 and IC90 on the concentration-response curve. (B) Scatter plots of the inhibitory effect of GM-109 (10−6M) on motilin-induced vasorelaxation. The motilin concentration used in the assay was 10−8–10−6M. Data are means±SEM (n=7). (C) Representative traces of motilin (10−8, 10−7, and 10−6M) before (red) and after (blue) incubation with GM-109 (10−6M). The double slash (//) indicates that GM-109 was added to the inhibitor group before treatment with U46619; arrows indicate the time of drug administration. ACh, acetylcholine; GM-109, MLNR antagonist; IC, inhibitory concentration; LGA, left gastric artery; MLNR, motilin receptor; MTL, motilin; SEM, standard error of the mean; and U46619, thromboxane A2 receptor agonist.
The G protein antagonist N-ethylmaleimide (NEM) decreased the RR of LGA by 52.3±4.0%, reducing the RR from 49.5±2.4 to 23.4±1.7% (n=7, p<0.0001; Figure 3A). The PLC inhibitor U73122 reduced the RR by 88.5%±2.0%, reducing the RR from 44.4±5.2 to 4.9±0.8% (n=7, p=0.0002; Figure 3B). The IP3 receptor blocker 2-APB decreased vasodilation by 95.4±1.1%, reducing the RR from 38.5±5.2 to 1.6±0.2% (n=7, p=0.0004; Figure 3C).
Figure 3. Role of the G protein-PLC-IP3 pathway on motilin-induced vasorelaxation. (A–C) Effect of NEM (3×10−5M), U73122 (10−5M), and 2-APB (3×10−4M) on LGA relaxation induced by motilin (9×10−8M) and corresponding representative traces. Values are means±SEM (n=7). ***p<0.001; ****p<0.0001 by paired t-test. The double slash (//) indicates pretreatment with NEM, U73122, or 2-APB before incubation with U46619. The arrows indicate the time of drug administration. 2-APB, 2-aminoethyl diphenylborinate (IP3 blocker); ACh, acetylcholine; IP3, inositol trisphosphate; LGA, left gastric artery; MTL, motilin; NEM, N-ethylmaleimide (G-protein antagonist); PLC, phospholipase C; SEM, standard error of the mean; U73122, PLC inhibitor; and U46619, thromboxane A2 receptor agonist.
The PKC inhibitor chelerythrine increased the RR from 34.9±2.9 to 39.7±3.7% with significance (n=7, p=0.012; Figure 4), showing no inhibitory effect on motilin-induced vasorelaxation.
Figure 4. Role of the DG-PKC pathway on motilin-induced vasorelaxation. Effect of chelerythrine (2×10−6M) on LGA relaxation induced by motilin (9×10−8M) and corresponding representative traces. Values are means±SEM (n=7). *p<0.05 by paired t-test. The double slash (//) indicates pretreatment with chelerythrine before incubation with U46619. The arrows indicate the time of drug administration. ACh, acetylcholine; CHEL, chelerythrine; DG, diacylglycerol; LGA, left gastric artery; MTL, motilin; PKC, protein kinase C; SEM, standard error of the mean; and U46619, thromboxane A2 receptor agonist.
The PKA inhibitor H89 increased the RR from 34.9±2.9 to 39.7±3.7% without significance (n=7, p=0.188; Figure 5).
Figure 5. Role of the AC-PKA pathway on motilin-induced vasorelaxation. Effect of H89 (5×10−6M) on LGA relaxation induced by motilin (9×10−8M) and corresponding representative traces. Values are means±SEM (n=7). ns, non-significant at p>0.05 by paired t-test. The double slash (//) indicates pretreatment with H89 before incubation with U46619. The arrows indicate the time of drug administration. AC, adenylyl cyclase; ACh, acetylcholine; H89, PKA inhibitor; LGA, left gastric artery; MTL, motilin; PKA, protein kinase A; SEM, standard error of the mean; and U46619, thromboxane A2 receptor agonist.
Left gastric artery rings were incubated with Krebs solution containing [Ca2+] of 2.5×10−3M (control group), 1.25×10−3, 0.625×10−3, and 0M. As the [Ca2+] decreased, motilin-induced RRs also gradually reduced (n=7; Figure 6; Supplementary Table 4).
Figure 6. Role of extracellular [Ca2+] on motilin-induced vasorelaxation. The Krebs solutions containing different Ca2+ concentrations decreased motilin-induced LGA relaxation in a concentration-dependent manner. Data are means±SEM (n=7). *p<0.05; **p<0.01; and ***p<0.001 by one-way ANOVA. In representative traces, the double slash (//) indicates pretreatment with different Ca2+ concentrations before incubation with U46619. The arrows indicate the time of drug administration. ACh, acetylcholine; ANOVA, analysis of variance; LGA, left gastric artery; MTL, motilin; SEM, standard error of the mean; and U46619, thromboxane A2 receptor agonist.
The NOS inhibitor L-NAME decreased the RR of the LGA by 90.8±4.0% (from 35.5±4.1 to 3.2±0.6%; n=7, p=0.0001; Figure 7A). Similarly, the sGC inhibitor ODQ reduced RR by 90.2±1.6% (from 36.1±3.8 to 3.4±0.5%; n=7, p=0.0001; Figure 7B).
Figure 7. Role of the NOS–NO–sGC–cGMP pathway on motilin-induced vasorelaxation. (A,B) Effects of L-NAME (10−4M) and ODQ (10−5M) on LGA relaxation induced by motilin (9×10−8M) and corresponding representative traces. Values are means±SEM (n=7). ***p<0.001 by paired t-test. The double slash (//) indicates pretreatment with L-NAME and ODQ before incubation with U46619. The arrows indicate the time of drug administration. ACh, acetylcholine; cGMP, cyclic guanosine monophosphate; LGA, left gastric artery; L-NAME, N-nitro-L-arginine methyl ester (NOS inhibitor); MTL, motilin; NO, nitric oxide; NOS, nitric oxide synthase; ODQ, sGC inhibitor; SEM, standard error of the mean; sGC, soluble guanylyl cyclase; and U46619, thromboxane A2 receptor agonist.
The cyclooxygenase inhibitor indomethacin reduced vasodilation by 17.9±3.7%, reducing the RR from 39.5±3.0 to 32.7±3.4% (n=7, p=0.004; Figure 8).
Figure 8. Role of PGI2 on motilin-induced vasorelaxation. Effect of indomethacin (10−5M) on LGA relaxation induced by motilin (9×10−8M) and corresponding representative trace. Values are means±SEM (n=7). **p<0.01 by paired t-test. The double slash (//) indicates pretreatment with indomethacin before incubation with U46619. The arrows indicate the time of drug administration. INDO, indomethacin; LGA, left gastric artery; MTL, motilin; PGI2, prostacyclin; SEM, standard error of the mean; and U46619, thromboxane A2 receptor agonist.
The MEGJ inhibitor 18α-GA decreased vasorelaxation by 25.1±3.3%, reducing the RR from 35.2±3.4 to 26.4±2.9% (n=7, p=0.0006; Figure 9A).
Figure 9. Roles of MEGJs and K+ channels on motilin-induced vasorelaxation. (A–D) Effects of 18α-GA (7.5×10−5M), high K+ solution (3×10−2M), TEA-Cl (10−2M), and glibenclamide (10−6M) on LGA relaxation induced by motilin (9×10−8M) and corresponding representative traces. Values are means±SEM (n=7). **p<0.01; ***p<0.001; and ****p<0.0001 by paired t-test. The double slash (//) indicates 18α-GA, high K+ solution, TEA-Cl, or glibenclamide pretreatment before incubation with U46619. The arrows indicate the time of drug administration. 18α-GA, 18α-glycyrrhetinic acid (MEGJ inhibitor); ACh, acetylcholine; GLIB, glibenclamide (KATP channel blocker); LGA, left gastric artery; MEGJ, myoendothelial gap junction; MTL, motilin; SEM, standard error of the mean; TEA-Cl, tetraethylammonium chloride (KCa channel blocker); and U46619, thromboxane A2 receptor agonist.
A high [K+] solution containing 30mM KCl causes cell membrane depolarization through K+ channels (Nelson and Quayle, 1995). This solution decreased vasorelaxation by 87.9±2.1%, decreasing the RR from 42.4±4.4 to 5.5±1.4% (n=7, p<0.0001; Figure 9B). The non-specific KCa channel blocker TEA-Cl reduced LGA relaxation by 48.5±3.7%, reducing the RR from 36.5±2.7 to 18.8±1.9% (n=7, p<0.0001; Figure 9C). The KATP channel blocker glibenclamide increased the RR from 41.3±2.7 to 55.0±4.3% (n=7, p=0.002; Figure 9D), showing no inhibitory effect.
The baseline levels of NO and cGMP in the blank group were 2.5±0.4μmolg−1 protein and 1.9±0.02pmolmg−1 protein, respectively (Figures 10A,B). Motilin increased the levels of NO and cGMP in LGA tissues by 1.3 and 1.5 times, respectively (p=0.0106 and p=0.0001). Acetylcholine (10−5M) increased the levels of NO and cGMP by approximately 2.4 and 2.5 times compared with baseline, respectively (p=0.0049 and p=0.0058), and approximately 1.8 and 1.7 times relative to motilin treatment, respectively (p=0.0020 and p=0.0129).
Figure 10. Roles of the MLNR and its signal transduction pathway in the motilin-induced production of NO and cGMP in LGA tissues. (A,B) Effect of agonists and inhibitors on the production of NO and cGMP in the LGA. Data are means±SEM (n=3). *p< 0.05 vs. blank group; #p<0.05 vs. motilin group by one-way ANOVA. The following drug concentrations were used: motilin, 9×10−8M; ACh, 10−5M; GM-109, 10−5M; L-NAME, 10−4M; and ODQ, 10−5M. ACh, acetylcholine; ANOVA, analysis of variance; cGMP, cyclic guanosine monophosphate; GM-109, MLNR antagonist; L-NAME, N-nitro-L-arginine methyl ester (NOS inhibitor); LGA, left gastric artery; MLNR, motolin receptor; MTL, motilin; NO, nitric oxide; ODQ, sGC inhibitor; and SEM, standard error of the mean.
GM-109 (10−5M) attenuated NO production in LGA tissues (p=0.0050) and markedly decreased the synthesis of cGMP (p=0.0041 vs. blank group and p=0.0034 vs. motilin group; Figures 10A,B).
L-NAME (10−4M) significantly decreased NO and cGMP levels (p=0.0037 and p=0.0279 vs. the blank group, and p=0.0063 and p=0.0076 vs. the motilin group, respectively).
The effect of ODQ (10−5M) on cGMP levels was similar to that of L-NAME (p=0.0001 vs. blank group and p<0.0001 vs. the motilin group).
Recent studies have shown that MLNRs are present on the ECs of gastrointestinal arteries (Yang et al., 2021), and endothelial MLNR is the molecular basis for the regulation gastric blood flow by motilin in dogs (Jin et al., 2002). The present study identified endothelial MLNR signaling pathways that induce VSM relaxation in the LGA of dogs (Figure 11).
Figure 11. Roles of endothelial MLNR and its signal transduction pathway on LGA SMC relaxation. Green arrows indicate the signaling pathways in EC and SMC during motilin- or acetylcholine-induced relaxation of the dog LGA. Red arrows with flat heads indicate the blocking effect of inhibitors on the signaling pathways. The drugs in red indicate an inhibitory effect on MLNR signaling pathways, and those in blue indicate no inhibitory effect. EC, endothelial cell; LGA, left gastric artery; MLNR, motolin receptor; and SMC, smooth muscle cell.
Phase III of the migrating motor complex is marked by peristaltic waves of electrical activity that propagate from the lower esophagus along the gastrointestinal tract to clear excessive bacteria and Luminal contents (Deloose et al., 2012; Takahashi, 2013). Motilin from pigs and dogs has been shown to induce MMC III in dogs to a similar extent (Poitras et al., 1983), and injections with porcine motilin (at 12.5, 25, 50, and 100pmol/kg/h) simultaneously induces gastric MMC III and a sustained increase in LGA blood flow (Jin et al., 2002). Thus, porcine motilin was used for the assays in the current study. MLNR is differentially expressed in gastrointestinal arteries, with preferential expression and the highest motilin-induced relaxation in the LGA (Yang et al., 2021). Under physiological conditions, motilin periodically increased the blood flow of the LGA; however, the blood flow in the superior mesenteric artery (SMA) remained unchanged (Jin et al., 2002). Therefore, the LGA was used to identify the signal transduction pathway by which MLNR induces VSM relaxation.
GM-109 is a selective and competitive antagonist of MLNR in rabbit duodenum SMCs (Tankanashi et al., 1995). Data from the current study reveal that GM-109 also has an effective inhibitory effect on endothelial MLNR. The results describe that motilin-induced LGA relaxation depends on GM-109-sensitive MLNR in greater detail than a previous study (Yang et al., 2021).
Upon activation, MLNR is coupled to the Gq protein (Depoortere and Peeters, 1995; Feighner et al., 1999). The inhibitory effects of NEM, U73122, and 2-APB confirm that motilin acts via the G protein-PLC-IP3 signal transduction pathway. Motilin has been shown to increase intracellular [Ca2+] in HEK-293/aeq17 cells transfected with MLNR via this same pathway (Feighner et al., 1999). In addition, both the motilin-dependent increase of intracellular [Ca2+] and rabbit gastrointestinal SMC contraction also signal through this pathway (Depoortere and Peeters, 1995; Huang et al., 2005). Diacylglycerol (DG) is another important second messenger in GPCR signaling under the action of PLC, which further leads to the activation of PKC. However, the non-inhibitory effect of chelerythrine excluded the potential contribution of the PLC-DG-PKC pathway. Adenylate cyclase (AC)-PKA is also an essential intracellular signal transduction pathway downstream of G proteins (Ghanemi, 2015); however, the role of AC-PKA was excluded by the non-inhibitory effect of H89 in motilin-induced vasorelaxation. In line with these results, the intracellular signaling cascades involved in motilin-induced gastrointestinal smooth muscle contraction in rabbits do not depend on PKA (Depoortere and Peeters, 1995). Thus, the MLNR-G protein-PLC-IP3 signal transduction pathway is shared between ECs and gastrointestinal SMCs.
It was shown that the initial increase in cytosolic [Ca2+] in ECs of porcine aortic valves was due to Ca2+ release from intracellular stores, whereas the maintenance of a stable [Ca2+] was associated with Ca2+ influx (Higuchi et al., 1994). It was hypothesized that the motilin-activated MLNR-G protein-PLC-IP3 pathway induced Ca2+ release from the endoplasmic reticulum (ER) in ECs (Neves et al., 2002). Importantly, a reduction in extracellular [Ca2+] resulted in a synchronous decrease in vasorelaxation, indicating that extracellular Ca2+ is involved in motilin-induced LGA relaxation. Extracellular Ca2+ also participates in the motilin-induced contraction of SMCs (Kato et al., 2019). However, Ca2+ enters excitable SMCs and non-excitable ECs mainly via voltage-operated Ca2+ channels and store-operated Ca2+ channels (SOCCs), respectively (Tsoukias, 2011). SOCCs are controlled by Ca2+ stores in the ER (Groschner et al., 2017). The inhibitory effect of 2-APB further confirms the role of SOCCs in motilin-induced vasorelaxation.
The increase in cytosolic [Ca2+] induces the secretion of vasorelaxant substances from ECs (Lopez-Jaramillo et al., 1990). The inhibitory effects of L-NAME and ODQ indicate that the NOS–NO–sGC-cGMP pathway plays a crucial role. PGI2 also participated in the process; however, the low inhibitory efficacy of indomethacin suggests a negligible contribution of PGI2 (Hiroaki et al., 1996). EDHF increases cytosolic [Ca2+], which opens Ca2+-activated K+ channels and hyperpolarizes ECs. Next, direct electrical coupling through myoendothelial gap junctions (MEGJs) and K+ accumulation in the intercellular space induces endothelium-dependent hyperpolarization (EDH) of SMCs, leading to VSM relaxation (Félétou, 2016). The fact that 18α-GA and high K+ solution inhibited vasodilation, indicating the involvement of EDHF in motilin-induced vasorelaxation. Furthermore, it is likely that the KCa channel, but not the KATP channel, plays a role. This study is the first to report the effects of three endothelial-derived relaxation mediators in MLNR-dependent VSM relaxation in the LGA.
Motilin receptor agonists used in the treatment of diabetic gastroparesis improve delayed gastric emptying and mimic gastric MMC III (Barshop and Kuo, 2015; Sanger and Furness, 2016; Kumar et al., 2018; Zhong et al., 2020). Gastric blood supply is lower in patients with diabetic gastroparesis compared to healthy subjects (Shen et al., 2016). The decrease in microvascular perfusion may lead to neuropathy (Tomesˇova´ et al., 2013; Wang et al., 2015), which in turn decreases gastric motility (Kumar et al., 2018). The decrease in NO production or release is the primary manifestation of endothelial dysfunction in diabetic microangiopathy (Zhang et al., 2018). The current study verified that NO was critical for MLNR-dependent VSM relaxation in the LGA. These results suggest that the effects of motilin on gastric blood flow (Jin et al., 2002) are related to its regulation of the digestive tract-brain-pancreatic axis (Singaram et al., 2020). In this respect, the molecular mechanism by which the endothelial-derived MLNR induces VSM relaxation in the LGA may help elucidate the pathogenesis of diabetic gastroparesis and improve the prevention and treatment of this gastric complication.
In summary, motilin induces VSM relaxation in the LGA mainly via the endothelial MLNR-Gpr-PLC-IP3 and NOS-NO-sGC-cGMP signaling pathways. Extracellular Ca2+, PGI2, and EDHF are also involved in this process. These pathways constitute the molecular mechanism by which motilin regulates LGA blood flow under physiological conditions, and these data may serve as the basis for understanding and treating gastric diseases.
The original contributions presented in the study are included in the article/Supplementary Material, further inquiries can be directed to the corresponding author.
The animal study was reviewed and approved by Animal Care and Use Committee of Jilin University (Permit No. 2016301).
HL: data curation and analysis, statistical analysis, and manuscript writing. LY: data curation and analysis and manuscript revision for important intellectual content. YJ: project supervision and data analysis and validation. CJ: study conceptualization, project administration, and funding acquisition. All authors contributed to the article and approved the submitted version.
This work was supported by the National Natural Science Foundation of China (Grant No. 31271235, received by CJ).
The authors declare that the research was conducted in the absence of any commercial or financial relationships that could be construed as a potential conflict of interest.
All claims expressed in this article are solely those of the authors and do not necessarily represent those of their affiliated organizations, or those of the publisher, the editors and the reviewers. Any product that may be evaluated in this article, or claim that may be made by its manufacturer, is not guaranteed or endorsed by the publisher.
The Supplementary Material for this article can be found online at: https://www.frontiersin.org/articles/10.3389/fphys.2021.770430/full#supplementary-material
Barshop, K., and Kuo, B. (2015). The investigational drug camicinal for the treatment of gastroparesis. Expert Opin. Investig. Drugs 24, 133–140. doi: 10.1517/13543784.2015.975792
Carvajal, J. A., Germain, A. M., Huidobro-Toro, J. P., and Weiner, C. P. (2000). Molecular mechanism of cGMP-mediated smooth muscle relaxation. J. Cell. Physiol. 184, 409–420. doi: 10.1002/1097-4652(200009)184:3<409::AID-JCP16>3.0.CO;2-K
Dass, N. B., Hill, J., Muir, A., Testa, T., Wise, A., and Sanger, G. J. (2003). The rabbit motilin receptor: molecular characterisation and pharmacology. Br. J. Pharmacol. 140, 948–954. doi: 10.1038/sj.bjp.0705505
Deloose, E., Janssen, P., Depoortere, I., and Tack, J. (2012). The migrating motor complex: control mechanisms and its role in health and disease. Nat. Rev. Gastroenterol. Hepatol. 9, 271–285. doi: 10.1038/nrgastro.2012.57
Deloose, E., Vos, R., Corsetti, M., Depoortere, I., and Tack, J. (2015). Endogenous motilin, but not ghrelin plasma levels fluctuate in accordance with gastr ic phase III activity of the migr ating motor complex in man. Neurogastroenterol. Motil. 27, 63–71. doi: 10.1111/nmo.12470
Depoortere, I., and Peeters, T. L. (1995). Transduction mechanism of motilin and motilides in rabbit duodenal smooth muscle. Regul. Pept. 55, 227–235. doi: 10.1016/0167-0115(94)00111-A
Feighner, S. D., Tan, C. P., McKee, K. K., Palyha, O. C., Hreniuk, D. L., Pong, S.-S., et al. (1999). Receptor for motilin identifed in the human gastrointestinal system. Science 284, 2184–2188. doi: 10.1126/science.284.5423.2184
Félétou, M. (2016). Endothelium-dependent hyperpolarization and endothelial dysfunction. J. Cardiovasc. Pharmacol. 67, 373–387. doi: 10.1097/FJC.0000000000000346
Ghanemi, A. (2015). Targeting G protein coupled receptor-related pathways as emerging molecular therapies. Saudi Pharm. J. 23, 115–129. doi: 10.1016/j.jsps.2013.07.007
Groschner, K., Graier, W. F., and Romanin, C. (2017). Store-Operated Ca2+ Entry (SOCE) Pathways Emerging Signaling Concepts in Human (Patho)Physiology, vol. 993. 2nd Edn. Switzerland: Springer, 343–362.
He, Y., Wang, H., Yang, D., Wang, C., Yang, L., and Jin, C. (2015). Differential expression of motilin receptor in various parts of gastrointestinal tract in dogs. Gastroenterol. Res. Pract. 2015:970940. doi: 10.1155/2015/970940
Higuchi, Y., Nushimura, J., and Kanaide, H. (1994). Motilin induces the endothelium-dependent relaxation of smooth muscle and the elevation of cytosolic calcium in endothelial cells in situ. Biochem. Biophys. Res. Commun. 202, 346–353. doi: 10.1006/bbrc.1994.1934
Hiroaki, S., Hiroshi, Y., Koji, F., Koji, O. M., Ryuichi, N., Yoshihiro, F., et al. (1996). The importance of the hyperpolarizing mechanism increases as the vessel size decreases in endothelium dependent relaxations in rat mesenteric circulation. J. Cardiovasc. Pharmacol. 5:70371. doi: 10.1097/00005344-199611000-00014
Huang, J., Zhou, H., Mahavadi, S., Sriwai, W., Lyall, V., and Murthy, K. S. (2005). Signaling pathways mediating gastrointestinal smooth muscle contraction and MLC20phosphorylation by motilin receptors. Am. J. Physiol. Gastrointest. Liver Physiol. 288, G23–G31. doi: 10.1152/ajpgi.00305.2004
Iwai, T., Nakamura, H., Takanashi, H., Yogo, K., Ozaki, K.-I., Ishizuka, N., et al. (1998). Hypotensive mechanism of [Leu13]motilin in dogs in vivo and in vitro. Can. J. Physiol. Pharmacol. 76, 1103–1109. doi: 10.1139/y98-138
Jin, C., Naruse, S., Kitagawa, M., Ishiguro, H., Muxin, W., Nakajima, M., et al. (2002). Motilin regulates interdigestive gastric blood flow in dogs. Gastroenterology 123, 1578–1587. doi: 10.1053/gast.2002.36584
Kato, S., Takahashi, A., Shindo, M., Yoshida, A., Kawamura, T., Matsumoto, K., et al. (2019). Characterization of the gastric motility response to human motilin and erythromycin in human motilin receptor-expressing transgenic mice. PLoS One 14:e0205939. doi: 10.1371/journal.pone.0205939
Kitazawa, T., and Kaiya, H. (2019). Regulation of gastrointestinal motility by motilin and ghrelin in vertebrates. Front. Endocrinol. 10:278. doi: 10.3389/fendo.2019.00278
Kukovetz, W. R., Holzmann, S., Wurm, A., and Poch, G. (1979). Prostacyclin increases cAMP in coronary arteries. J. Cyclic Nucleotide Res. 5, 469–476.
Kumar, M., Chapman, A., Javed, S., Alam, U., Malik, R. A., and Azmi, S. (2018). The investigation and treatment of diabetic gastroparesis. Clin. Ther. 40, 850–861. doi: 10.1016/j.clinthera.2018.04.012
Lee, K. Y., Chang, T.-M., and Chey, W. Y. (1983). Effect of rabbit antimotilin serum on myoelectric activity and plasma motilin concentration in fasting dog. Am. J. Phys. 245, G547–G553. doi: 10.1152/ajpgi.1983.245.4.G547
Leloup, L. J. A., Hove, C. E. V., Heykers, A., Schrijvers, D. M., Meyer, G. R. Y. D., and Fransen, P. (2015). Elastic and muscular arteries differ in structure, basal NO production and voltage-gated ca(2+)-channels. Front. Physiol. 6:375. doi: 10.3389/fphys.2015.00375
Leming, S., Broad, J., Cozens, S. J., Otterson, M., Winchester, W., Lee, K., et al. (2011). GSK962040: a small molecule motilin receptor agonist which increases gastrointestinal motility in conscious dogs. Neurogastroenterol. Motil. 23, 958–e410. doi: 10.1111/j.1365-2982.2011.01770.x
Lopez-Jaramillo, P., Gonzalez, M. C., Palmer, R. M. J., and Moncada, S. (1990). The crucial role of physiological Ca2+ concentrations in the production of endothelial nitric oxide and the control of vascular tone. Br. J. Pharmacol. 101, 489–493. doi: 10.1111/j.1476-5381.1990.tb12735.x
Miller, P., Roy, A., St-Pierre, S., Dagenais, M., Lapointe, R., and Poitras, P. (2000a). Motilin receptors in the human antrum. Am. J. Physiol. Gastrointest. Liver Physiol. 278, G18–G23. doi: 10.1152/ajpgi.2000.278.1.G18
Miller, P., Trudela, L., St-Pierreb, S., Takanashic, H., and Poitrasa, P. (2000b). Neural and muscular receptors for motilin in the rabbit colon. Peptides 21, 283–287. doi: 10.1016/s0196-9781(99)00198-9
Mitselos, A., Depoortere, I., and Peeters, T. L. (2007). Delineation of the motilin domain involved in desensitization and internalization of the motilin receptor by using full and partial antagonists. Biochem. Pharmacol. 73, 115–124. doi: 10.1016/j.bcp.2006.09.011
Nelson, M. T., and Quayle, J. M. (1995). Physiological roles and properties of potassium channels in arterial smooth muscle. Am. J. Phys. 268, C799–C822. doi: 10.1152/ajpcell.1995.268.4.C799
Neves, S. R., Ram, P. T., and Iyengar, R. (2002). G protein pathways. Science 296, 1636–1639. doi: 10.1126/science.1071550
Ogawa, A., Mochiki, E., Yanai, M., Morita, H., Toyomasu, Y., Ogata, K., et al. (2011). Interdigestive migrating contractions are coregulated by ghrelin and motilin in conscious dogs. Am. J. Phys. Regul. Integr. Comp. Phys. 302, R233–R241. doi: 10.1152/ajpregu.00078.2011
Ohshiro, H., Nonaka, M., and Ichikawa, K. (2008). Molecular identification and characterization of the dog motilin receptor. Regul. Pept. 146, 80–87. doi: 10.1016/j.regpep.2007.08.012
Poitras, P., Joseph, J., Reeve, R., Hunkapiller, M. W., Hood, L. E., and Walsh, J. H. (1983). Purification and characterization of canine intestinal motilin. Regul. Pept. 5, 197–208. doi: 10.1016/0167-0115(83)90251-3
Poitras, P., Miller, P., Dickner, M., Mao, Y. K., Daniel, E. E., St-Pierre, S., et al. (1996). Heterogeneity of motilin receptors in the gastrointestinal tract of the rabbit. Peptides 17, 701–707. doi: 10.1016/0196-9781(96)00053-8
Sadig, R. R., Allende, A., Geoffrey Hall, D. T., Madigan, M. C., Watson, S. L., Ooi, K. G., et al. (2021). Motilin receptor expression found in the human main and accessory lacrimal glands. Ocul. Immunol. Inflamm. 1–6. doi: 10.1080/09273948.2021.1903937 [Epub ahead of print].
Sanger, G. J., and Furness, J. B. (2016). Ghrelin and motilin receptors as drug targets for gastrointestinal disorders. Nat. Rev. Gastroenterol. Hepatol. 13, 38–48. doi: 10.1038/nrgastro.2015.163
Schachter, D. (2007). L-glutamine in vitro regulates rat aortic glutamate content and modulates nitric oxide formation and contractility responses. Am. J. Phys. Cell Physiol. 293, C142–C151. doi: 10.1152/ajpcell.00589.2006
Shen, H.-L., Yang, S.-P., Wang, K.-J., Huang, B.-L., Huang, W.-B., Wu, J.-Z., et al. (2016). Evaluation of gastric blood supply in diabetic patients with gastroparesis by contrast-enhanced ultrasound. Br. J. Radiol. 89:20160366. doi: 10.1259/bjr.20160366
Singaram, K., Gold-Smith, F. D., and Petrov, M. S. (2020). Motilin: a panoply of communications between the gut, brain, and pancreas. Expert Rev. Gastroenterol. Hepatol. 14, 103–111. doi: 10.1080/17474124.2020.1718492
Sun, D., Liu, H., Yan, C., Jacobson, A., Ojaimi, C., Huang, A., et al. (2006). COX-2 contributes to the maintenance of flow-induced dilation in arterioles of eNOS-knockout mice. Am. J. Physiol. Heart Circ. Physiol. 291, H1429–H1435. doi: 10.1152/ajpheart.01130.2005
Suzuki, A., Ishida, Y., Aizawa, S., Sakata, I., Tsutsui, C., Mondal, A., et al. (2012). Molecular identification of GHS-R and GPR38 in suncus murinus. Peptides 36, 29–38. doi: 10.1016/j.peptides.2012.04.019
Takahashi, T. (2013). Interdigestive migrating motor complex its mechanism and clinical importance. J. Smooth Muscle Res. 49, 99–111. doi: 10.1540/jsmr.49.99
Tankanashi, H., Yogo, K., Ozaki, K., Ikuta, M., Akima, M., Koga, H., et al. (1995). GM-109 a novel, selective motilin receptor antagonist inthe smooth muscle of the rabbit small intestine. J. Pharmacol. Exp. Ther. 273, 624–628.
TomeŠová, J., Gruberova, J., Lacigova, S., Cechurova, D., Jankovec, Z., and Rusavy, Z. (2013). Differences in skin microcirculation on the upper and lower extremities in patients with diabetes mellitus: relationship of diabetic neuropathy and skin microcirculation. Diabetes Technol. Ther. 15, 968–975. doi: 10.1089/dia.2013.0083
Tsoukias, N. M. (2011). Calcium dynamics and signaling in vascular regulation. Wiley Interdiscip. Rev. Syst. Biol. Med. 3, 93–106. doi: 10.1002/wsbm.97
Wang, L., Chopp, M., Szalad, A., Jia, L., Lu, X., Lu, M., et al. (2015). Sildenafil ameliorates long term peripheral neuropathy in type II diabetic mice. PLoS One 10:e0118134. doi: 10.1371/journal.pone.0146050
Yang, L., Li, H., Jin, Y., He, Y., Mei, L., and Jin, C. (2021). Differential expression of motilin receptors on the endothelium of dog gastrointestinal arteries and motilin-induced motilin receptor dependent relaxation of corresponding arteries. Peptides 143:170574. doi: 10.1016/j.peptides.2021.170574
Zhang, H., Liu, J., Quc, D., Wangc, L., Wong, C. M., Lau, C.-W., et al. (2018). Serum exosomes mediate delivery of arginase 1 as a novel mechanism for endothelial dysfunction in diabetes. Proc. Natl. Acad. Sci. U. S. A. 115, E6927–E6936. doi: 10.1073/pnas.1721521115
Keywords: endothelial motilin receptor, signal pathway, nitric oxide, vasorelaxation, dog left gastric artery
Citation: Li H, Yang L, Jin Y and Jin C (2021) Roles of Endothelial Motilin Receptor and Its Signal Transduction Pathway in Motilin-Induced Left Gastric Artery Relaxation in Dogs. Front. Physiol. 12:770430. doi: 10.3389/fphys.2021.770430
Received: 03 September 2021; Accepted: 05 October 2021;
Published: 28 October 2021.
Edited by:
Stephen J. Pandol, Cedars Sinai Medical Center, United StatesReviewed by:
Gareth J. Sanger, Queen Mary University of London, United KingdomCopyright © 2021 Li, Yang, Jin and Jin. This is an open-access article distributed under the terms of the Creative Commons Attribution License (CC BY). The use, distribution or reproduction in other forums is permitted, provided the original author(s) and the copyright owner(s) are credited and that the original publication in this journal is cited, in accordance with accepted academic practice. No use, distribution or reproduction is permitted which does not comply with these terms.
*Correspondence: Ying Jin, amlueWluZzAyMTZAamx1LmVkdS5jbg==; ChunXiang Jin, amluY3g3MjJAamx1LmVkdS5jbg==
Disclaimer: All claims expressed in this article are solely those of the authors and do not necessarily represent those of their affiliated organizations, or those of the publisher, the editors and the reviewers. Any product that may be evaluated in this article or claim that may be made by its manufacturer is not guaranteed or endorsed by the publisher.
Research integrity at Frontiers
Learn more about the work of our research integrity team to safeguard the quality of each article we publish.