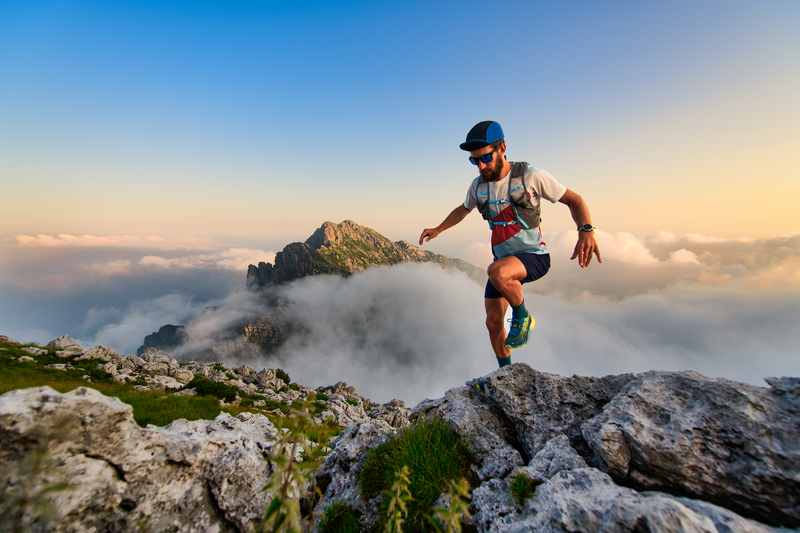
94% of researchers rate our articles as excellent or good
Learn more about the work of our research integrity team to safeguard the quality of each article we publish.
Find out more
REVIEW article
Front. Physiol. , 16 December 2021
Sec. Vascular Physiology
Volume 12 - 2021 | https://doi.org/10.3389/fphys.2021.768383
This article is part of the Research Topic Nutritional Intervention in Cardiovascular Diseases: from Basic Science to Applied Investigations View all 6 articles
Caloric restriction (CR) and intermittent fasting (IF) are strategies aimed to promote health beneficial effects by interfering with several mechanisms responsible for cardiovascular diseases. Both dietary approaches decrease body weight, insulin resistance, blood pressure, lipids, and inflammatory status. All these favorable effects are the result of several metabolic adjustments, which have been addressed in this review, i.e., the improvement of mitochondrial biogenesis, the reduction of reactive oxygen species (ROS) production, and the improvement of cardiac and vascular function. CR and IF are able to modulate mitochondrial function via interference with dynamics (i.e., fusion and fission), respiration, and related oxidative stress. In the cardiovascular system, both dietary interventions are able to improve endothelium-dependent relaxation, reduce cardiac hypertrophy, and activate antiapoptotic signaling cascades. Further clinical studies are required to assess the long-term safety in the clinical setting.
Increased energy intake with a continuous positive, energetic balance is obviously associated with obesity and results in high cardiometabolic morbidity and mortality (Pierce et al., 2008). An increasing number of experimental and clinical studies have lately addressed the beneficial effects of reduced caloric intake. Accordingly, caloric restriction (CR) is able to prevent the development of cardiovascular disease (CVD), type 2 diabetes mellitus, cancer, and neurodegenerative disorders (Speakman and Mitchell, 2011; Di Daniele et al., 2021; Senesi et al., 2021), as well as pathologies with frequent association with each other (Ristow, 2004; Albai et al., 2020). While early research in this field was focused on the effects of conventional hypocaloric diets with continuous energy restriction, in the past decade, a new model of dietary intervention, i.e., intermittent fasting (IF), has gained scientific interest. IF is a dietary model that includes alternation of periods with reduced caloric intake with periods of unrestricted feeding. Even though both dietary models can result in a decreased energy intake, the long-term adherence to CR is more difficult as compared with IF (Wegman et al., 2015; Lee et al., 2020). The most studied models of IF used CR on 2 days/week (5:2), alternate day energy restriction (energy restriction of 60–70% below estimated requirements) and time-restricted feeding (TRF) (timely restriction of calories intake during a specific time window during the day) (Nowosad and Sujka, 2021). Regardless of the model, IR and CR have been systematically associated with several beneficial effects in animal models and humans (Di Daniele et al., 2021). The cardiometabolic benefits can be summarized as follows: decrease in body weight and fat mass, improvement of insulin sensitivity, decrease in blood pressure, lipid-lowering effect, and decrease in the level of serum inflammatory markers (Johnson et al., 2016; Brandhorst and Longo, 2019). Mechanistically, all these beneficial effects are the results of several metabolic adaptations, which will be discussed in this review: the improvement of mitochondrial biogenesis, the decreased reactive oxygen species (ROS) production, and the improvement of cardiac and vascular function.
Mitochondrial dysfunction has emerged in the past decade as a central pathomechanism of cardiometabolic disorders. One important trigger in the normalization of mitochondrial quality and quantity in disease is represented by calorie restriction diets. There are several studies demonstrating that CR and IF may develop the protective effects of mitochondria, but the exact mechanisms underlying the improvement in mitochondrial function and dynamics are not fully understood. We further summarized the main studies aimed at investigating the effects of both CR and IF in animal experiments and humans. The main effects of CR and IF dietary interventions on mitochondrial function, endothelial dysfunction, and cardiovascular parameters are presented in Tables 1, 2.
Table 1. The main effects of caloric restriction (CR) dietary intervention on mitochondrial function, endothelial dysfunction, and cardiovascular parameters.
Table 2. The main effects of intermittent fasting (IF) dietary intervention on mitochondrial function, endothelial dysfunction, and cardiovascular parameters.
Mitochondria are dynamic intracellular organelles that fuse and divide, being actively recruited to different cellular locations. Three large GTPases are required in the process of mitochondrial fusion: protein OPA1 (Mitochondrial Dynamin-Like GTPase) from the inner mitochondrial membrane, and protein mitofusins 1 and 2 (MFN1 and MFN2) at the outer membrane. Importantly, the expression of MFN1 and MFN2 was observed to be increased (in the white and brown adipose tissue, heart, brain, and liver) in animals subjected to CR (3 or 12 months) (Nisoli et al., 2005). This effect was mediated by endothelial nitric oxide synthase (eNOS) since it was absent in eNOS KO animals (Nisoli et al., 2005).
Mitochondrial fission requires GTPase dynamin-related protein 1 (DRP1) and mitochondrial fission protein 1 (FIS1) from the outer membrane. Khraiwesh et al. analyzed the ultrastructural changes and markers of fission/fusion in hepatocyte mitochondria from mice submitted to 40% CR for a period of 6 months. The results showed that proteins related to mitochondrial fission (FIS1 and DRP1) increased with CR, but the three fusion proteins examined (MFN1, MFN2, and OPA1) did not exhibit changes (Khraiwesh et al., 2013).
Mitochondrial fusion and fission are crucial for mitochondrial function. In an experimental model aimed to characterize the impact of CR on mitochondrial morphology and dynamics in muscular tissue, it was reported that CR (starting with a 20% restriction for 2 weeks, followed by a 40% CR for 13 months) attenuated the age-related mitochondrial fragmentation and also increased the muscle level of fusion protein MFN2 (Faitg et al., 2019).
At present, the effects of IF on mitochondrial ultrastructure and dynamics have been less explored.
Mitochondria are the major sources of intracellular ROS being also vulnerable to oxidative stress. There are numerous studies in animals and humans showing the beneficial effects of diet intervention on mitochondria-related ROS production. Importantly, a decrease in ROS generation has been associated with caloric intake and age, as reported by a pioneering study where the level of mitochondrial superoxide and hydrogen peroxide was significantly higher in mice fed ad libitum vs. mice subjected to 40% CR for a period of 23 months (Sohal et al., 1994). The long-term exposure to CR is able to reduce not only the oxidative stress but also the oxidative damage of the mitochondrial DNA (by 30%) (López-Torres et al., 2002). Mechanistically, it seems that CR can influence both ROS generation and antioxidant capacity. Specifically, CR reduced the activity of NADPH oxidase and increased the activity of the antioxidant enzymes such as glutathione peroxidase (GPX) and superoxide dismutase (SOD), in the setting of cardiac hypertrophy associated with chronic pressure overload (Kobara et al., 2015). Even short periods of CR (3 weeks) were able to prevent the development of isoproterenol-induced cardiac hypertrophy via the reduction of ROS generation (decreased H2O2 and lower protein sulfhydryl oxidation) and the preservation of the antioxidant enzymes (i.e., catalase, SOD, and GPX) activity, respectively (David et al., 2018). Interestingly, severe CR in rats (complete restriction for 72 h) decreased the expression of monoamine oxidase (MAO), which is a mitochondrial enzyme at the outer mitochondrial membrane in the white adipose tissue (Iffiú-Soltész et al., 2009). MAO expression was increased in obese patients with the inflammatory status being responsible for high ROS production (Sturza et al., 2019). Apparently, CR is able to interfere with the monoaminergic system by increasing the level of noradrenaline in the brain and improving the peripheral levels of insulin, leptin, calcium, and HDLc in old animals (Portero-Tresserra et al., 2020).
Also, in an experimental model of ischemia-reperfusion aimed to investigate the effect of CR on mitochondrial function and subsequent molecular mechanisms of CR-induced cardioprotection, a significant reduction of mitochondrial oxidative stress (H2O2) in cardiac samples isolated from the CR group (6 months) (Shinmura et al., 2011). Also, in the same study, a reduction in the acetylated forms of NADH-ubiquinone oxidoreductase, i.e., the largest subunit of complex I (NDUFS1) and cytochrome bc1 has been reported in the CR group (Shinmura et al., 2011). In almost all animal studies aimed to investigate the effect of CR on oxidative stress in the heart, H2O2 was found to be reduced together with an increase in the antioxidant capacity in response to CR and with an increase in mitochondrial differentiation (mitochondrial protein/gram of tissue and mitochondrial protein/mitochondrial DNA) (Colom et al., 2007). The effect is mainly mediated by the regulation of mitochondrial complexes I and III (Colom et al., 2007), and in some experiments, the effect was present even after 1 month of CR (Chen et al., 2012). In leptin-resistant-obese mice, CR (1 month) reduced the levels of malondialdehyde (MDA) in the serum and increased the level of peroxisome proliferator-activated receptor-gamma coactivator 1 alpha (PGC-1α protein), which is a member of a family of transcription coactivators with a central role in the regulation of energy metabolism) (Waldman et al., 2018). Of note, the maximal H2O2 production from rat heart mitochondrial complexes I and III was lower in females compared to males and was decreased by a 3-month period of CR (Colom et al., 2007).
An interesting observation is the effect of CR on the antioxidant mitochondrial enzymes vs. the cytosolic ones. It was noticed that a short period of CR (2 months) in rats significantly increased the activity of mitochondrial (but not of cytosolic) SOD and GPX activity (Judge et al., 2004). Also, long periods of CR (6 months) in senescent rats alleviated mitochondrial dysfunction resulting in less oxidative damage and reduced mortality (Niemann et al., 2010).
The protective effect induced by 6 months of CR on mitochondria also involved the regulation of SIRT3 (i.e., NAD-dependent deacetylase sirtuin-3), a mitochondrial deacetylase (Qiu et al., 2010; Shinmura et al., 2011). SIRT3 deacetylase increased mitochondrial respiration and reduced ROS production via the activation of SOD2. In mice lacking SIRT3, the protective effects of CR were significantly reduced (Qiu et al., 2010).
The amount of studies investigating the effect of IF on mitochondrial ROS production is rather limited. A protocol of alternate-day fasting (ADF, 24 months) in rats was able to reduce oxidative stress (assessed by the increased level of glutathione) and decrease inflammatory status in the heart during aging similar to young animals (Castello et al., 2010). Also, every-other-day IF in combination with high-intensity intermittent exercise protocol optimized the metabolic pathways, with a strong reduction in MDA levels and a decrease in plasma protein oxidation (Real-Hohn et al., 2018).
Mitochondrial respiration is the most important mechanism of cellular energy generation, and its impairment had been linked to a plethora of pathological conditions. Several studies have investigated whether oxidative damage and impairment of mitochondrial respiration can be modulated by the reduction in caloric intake. In an experimental study in rats using a protocol of CR (40%, 23 months), it was noticed that mitochondrial state 4 increased with age (with subsequent high ROS production) in the ad libitum fed, but not in the CR group (Sohal et al., 1994). Importantly, CR can generate rapid effects (1 month) on the electron transport system (Chen et al., 2012). Another study was aimed to assess the impact of CR on mitochondrial respiration in the aging brain (25-month-old animals) and showed that CR was able to reduce the age-related membrane rigidization and limit the oxy-radical production, following the metabolic stimulation of mitochondria with succinate (Gabbita et al., 1997). At variance, in a study focused on the antiaging effect of CR on mitochondria isolated from different rat tissues (brown adipose tissue, liver, heart, kidney, and brain), no significant effects of CR on state 4 mitochondrial respiration rate were found in all tissues, except for the brown adipose tissue, where state 4 was ~3-fold higher as compared to mitochondria from fully fed controls (Lambert et al., 2004).
In other studies, CR (short period: 6 weeks and long period: 1 year) decreased the amount of ROS generated at the level of the respiratory chain (Gredilla et al., 2001). In mice subjected to CR diets (3 and 12 months), oxygen consumption was higher, especially in the white adipose tissue; this process was correlated with a higher ATP level (Nisoli et al., 2005). In rat hearts subjected to an ischemia-reperfusion injury episode, prior 6 months of CR preserved state 3 respiratory rate and increased the respiratory control index when pyruvate and malate were used as respiratory substrates, indicating that mitochondria isolated at the post-ischemic reperfusion were well-coupled (Shinmura et al., 2011). In a recent elegant study, the Kowalkowski group highlighted the tissue-specific changes in bioenergetics, mitochondrial calcium handling, and redox balance in cardiac and skeletal muscle mitochondria in response to CR (6 months). Accordingly, CR decreased respiration in the presence of ATP synthesis in the heart and soleus muscle. In the heart (but not in skeletal muscle), lower maximal respiratory rates and a reduced rate of hydrogen peroxide release were reported. In this model, no changes in the respiratory control ratios were found (Serna et al., 2020).
The evaluation of mitochondrial respiration in rats subjected to IF for 8 weeks showed that an every-other-day protocol lead to an improved O2 flux and ATP production coupling demonstrated by high respiratory control ratios. These beneficial effects were synergic when combined with a high-intensity intermittent exercise protocol (Real-Hohn et al., 2018).
Despite the fact that CVD remains the leading cause of morbidity and mortality all over the world, many of the CVD risk factors can be partially controlled (and reversed) by dietary intervention and physical exercise. Currently, several studies demonstrated that CR and IF represent powerful protective mechanisms in both heart and vasculature.
In the heart, CR can attenuate age-related changes that occurred in the myocardium, i.e., hypertrophy, fibrosis, and apoptosis (Weiss and Fontana, 2011), and can preserve or improve diastolic function. Similarly, short periods of CR (2–3 weeks) prevented the development of cardiac hypertrophy (Kobara et al., 2015; David et al., 2018); long periods (3 months) were able to improve cardiac insulin sensitivity and to prevent the reduction in myocardial contractility associated with aging (Granado et al., 2019).
Most authors aimed to investigate whether CR can reduce risk factors for CVD and insulin resistance in non-obese humans; these authors showed that CR decreased visceral and subcutaneous abdominal adipose tissue, blood pressure, and homeostatic model assessment (HOMA) index and also improved lipid profile, and the 10-year risk of CVD was reduced by 30% (Most et al., 2018).
Importantly, the high generation of angiotensin 2 (Gavras and Gavras, 2002) and the high level of plasma lipoproteins associated with elevated triglyceride levels (Balint et al., 2011) are able to increase the cardiovascular risk and complications. CR was able to attenuate the angiotensin 2-induced hypertension in diabetic mice (Waldman et al., 2018), ameliorate angiotensin 2-induced cardiomyocyte hypertrophy, fibrosis, and apoptosis (Finckenberg et al., 2012) and to improve the lipid profile (Waldman et al., 2018). CR leads to the downregulation of the genes associated with cardiac overload, i.e., brain natriuretic peptide (Niemann et al., 2010; Waldman et al., 2018) and atrial natriuretic peptide (Niemann et al., 2010; Finckenberg et al., 2012), of genes related to remodeling (transforming growth factor-beta (TGF-β), tumor necrosis factor-alpha (TNF-α), and matrix metallopeptidase 2 (MMP2) (Waldman et al., 2018); furthermore, CR also reduced the expression of proapoptotic genes Bcl-xS/Bcl-xL, mitochondrial translocation of BAX, release of cytochrome c into cytosol, and caspase-9 activation (Niemann et al., 2010).
The CR for 3 or 12 months increased the expression of eNOS and cGMP formation in various murine tissues. This influenced mitochondrial biogenesis with increased oxygen consumption and ATP production (Nisoli et al., 2005).
Fontana et al. followed 18 individuals who had been on the CR diet for an average of 6 years and 18 age-matched healthy individuals on typical American diets. They found significantly better lipid profiles and blood pressure values in the CR group. Importantly, carotid artery IMT was 40% less in the CR group (Fontana et al., 2004). However, not only the long periods but also the short periods of CR can be beneficial. In obese patients, a short period of CR and a high-intensity exercise program (3 weeks) reduced body mass index (BMI), heart rate, and blood pressure and also improved maximal oxygen consumption during exercise (Facchini et al., 2003). In other studies in humans, a hypocaloric diet was able to reduce arterial stiffness in obese adults (Dengo et al., 2010). In the animal model of obese pre-diabetic rats, 13 weeks of CR significantly decreased body weight, visceral fat deposition, blood pressure (systolic and diastolic), and improved insulin sensitivity (HOMA index) and left ventricular function (Palee et al., 2020). Also, calcium homeostasis and cardiac mitochondrial function were normalized (Palee et al., 2020). Even short periods of CR have visible effects; thus, only 4 days with CR (reduced dietary intake by 50%) significantly reduced the blood pressure in spontaneously hypertensive rats (Young et al., 1978).
However, it has to be mentioned that severe CR impaired the SERCA2a activity and lowered the expression of L-type Ca2+ channels with a negative impact on the cardiac contractile function (Deus et al., 2019).
Regarding the effect of CR on endothelial dysfunction, several studies showed an improvement of the endothelium-dependent vasorelaxation in different experimental settings and by several mechanisms: increase in eNOS level and decrease of iNOS (Zanetti et al., 2010), reverse of high-fat diet-induced endothelial dysfunction and vascular superoxide production in C57Bl/6 mice (Ketonen et al., 2010), improvement of endothelium-dependent vasodilation (evaluated by brachial artery flow-mediated dilation) in obese patients with essential hypertension (Sasaki et al., 2002), reversal of vascular endothelial dysfunction in old mice by increasing nitric oxide and decreasing NADPH oxidase-related ROS production (Rippe et al., 2010), improvement of pulse wave velocity, carotid artery wall thickness, and endothelium-dependent relaxation also in aged mice (Donato et al., 2013).
However, a short period (2 weeks) of severe food restriction in rats was responsible for endothelial dysfunction in mesenteric arteries and for ischemia-reperfusion-induced arrhythmias and cardiac pathology (Almeida et al., 2020).
The impact of IF diet protocols on heart metabolism was also studied. It was revealed that alternate-day-fasting can reduce left ventricular fibrosis in the aged rat hearts (Castello et al., 2010), infarct size (Ahmet et al., 2005; Okoshi et al., 2019), cardiomyocyte apoptosis, and infiltration with neutrophils and macrophages in rats subjected to myocardial infarction (Ahmet et al., 2005). Importantly, the protective effects of IF were persistent since echocardiography revealed that after 10–12 weeks after myocardial infarction, the left ventricular remodeling and diastolic posterior wall thickness was decreased in the IF group (IF regimen continued) (Ahmet et al., 2005; Okoshi et al., 2019). Of note, in these experiments, the mortality rate in the IF group was significantly reduced than in controls (Ahmet et al., 2005; Okoshi et al., 2019). Mechanistically, it was demonstrated that the activation of PI3kinase/Akt and VEGF pathway is responsible for the IF-induced protection in chronic myocardial ischemia (Katare et al., 2009). Molecular studies showed the increase of several angiogenic factors (sHIF-1-α, BDNF, and VEGF) in the fasted hearts (Katare et al., 2009). Also, it was showed an increased capillary density in the ischemic myocardium and the synthesis of VEGF by cardiomyocytes and the upregulation of antiapoptotic factors expression (Akt and Bcl-2) (Katare et al., 2009). IF activated cell survival cascade and also improved the survival rate after large myocardial infarction (Katare et al., 2009). The cardioprotective effects induced by IF are mediated by interaction with pro-inflammatory cytokines; alternate-day-fasting reduced the amount of TNFα, IL-6, and IL-1β in aged myocardium animals together with the reduction of TGF-β1, collagen contents, and NF-κB DNA-binding activity (Castello et al., 2010). Other studies aimed to investigate the influence of IF (associated with exercise training) on cardiac remodeling, which reported a reduction in cardiac interstitial collagen fraction (Basilio et al., 2020; Carvalho et al., 2021), a better glycemic control, a low expression of proapoptotic gene BAX and of cytochrome c, and increased expression of the antiapoptotic protein Bcl-2 (Carvalho et al., 2021).
Despite the positive findings in animal research, currently, there are no long-term safety data with IF diets, especially in the normal-weight population. Also, it has to be emphasized that severe/drastic IF diets (reduction with 70% of caloric intake) have been associated with adverse effects such as fatigue, irritability, mood disorders, concentration difficulties, and uncontrolled hyperphagia on the days of unrestricted feeding. Nevertheless, this is not the case for overweight and obese individuals, which followed intermittent CR diets (Katsarou et al., 2021).
Regarding the endothelial function, most of the studies using IF regimens showed beneficial effects: improved brachial artery flow-mediated dilation (Bhutani et al., 2013), improved endothelial- and non-endothelial-dependent vasodilation response and decreased blood pressure in human subjects (Ezad and Borne, 2016), and improved endothelial function in adults with metabolic syndrome after 8 weeks of IF (Guo et al., 2021). Also, there are studies with no significant effect of IF on endothelial function; two consecutive days of energy restriction (IF = 5:2 diet) had no effect on endothelial function (evaluated by brachial artery flow-mediated dilation) (Headland et al., 2018). Also, no effect of TRF on vascular endothelial function in middle-aged and older adults (Martens et al., 2020), despite other benefits (e.g., reduction of insulin resistance) (Hoddy et al., 2016) were reported. Still, TRF is an IF dietary approach used for weight loss and overall health, being able to improve metabolic syndrome parameters and cardiovascular risk in obese women (Schroder et al., 2021).
Main beneficial effects of CR and IF dietary interventions on mitochondria, ROS production, and cardiovascular parameters are depicted in Figure 1.
Figure 1. Main beneficial effects of caloric restriction (CR) and intermittent fasting (IF) dietary interventions on mitochondria, reactive oxygen species (ROS) production, and cardiovascular parameters.
In the last years, the interest in dietary strategies aimed at decreasing weight and also counteracting CV disease is increasing. The use of CR diets, more recent of IF regimens, represents the nutritional approaches that may normalize mitochondrial, endothelial/vascular function, and cardiomyocyte/heart metabolism mechanisms that have been unequivocally proven in various experimental settings. However, large clinical trials are required to allow definitive conclusions regarding the long-term safety of these simple approaches in both prevention and therapy of cardiometabolic diseases.
DCM, DMM, and AS proposed the conception. CES and AL contributed equally to the original draft preparation. AMB, GF, and OMC were involved in all aspects of writing, editing, and decision to publish. All authors contributed to the article and approved the submitted version.
The authors declare that the research was conducted in the absence of any commercial or financial relationships that could be construed as a potential conflict of interest.
All claims expressed in this article are solely those of the authors and do not necessarily represent those of their affiliated organizations, or those of the publisher, the editors and the reviewers. Any product that may be evaluated in this article, or claim that may be made by its manufacturer, is not guaranteed or endorsed by the publisher.
Ahmet, I., Wan, R., Mattson, M. P., Lakatta, E. G., and Talan, M. (2005). Cardioprotection by intermittent fasting in rats. Circulation 112, 3115–3121. doi: 10.1161/CIRCULATIONAHA.105.563817
Albai, O., Frandes, M., Timar, B., Paun, D. L., Roman, D., and Timar, R. (2020). Long-term risk of malignant neoplastic disorders in type 2 diabetes mellitus patients with metabolic syndrome. Diabetes Metab. Syndr. Obes. 13, 1317–1326. doi: 10.2147/DMSO.S243263
Almeida, J. F. Q., Shults, N., de Souza, A. M. A., Ji, H., Wu, X., Woods, J., et al. (2020). Short-term very low caloric intake causes endothelial dysfunction and increased susceptibility to cardiac arrhythmias and pathology in male rats. Exp. Physiol. 105, 1172–1184. doi: 10.1113/EP088434
Balint, G. S., Borza, C., Cristescu, C., Andoni, M., Simu, G. M., Malita, D., et al. (2011). Endogenous and exogenous antioxidant protection for endothelial dysfunction. Rev. Chim. 62, 680–683. doi: 10.37358/Rev.Chim.1949
Barros, M. H., Bandy, B., Tahara, E. B., and Kowaltowski, A. J. (2004). Higher respiratory activity decreases mitochondrial reactive oxygen release and increases life span in Saccharomyces cerevisiae. J. Biol. Chem. 279, 49883–49888. doi: 10.1074/jbc.M408918200
Basilio, P. G., Oliveira, A. P. C., Castro, A. C. F., Carvalho, M. R., Zagatto, A. M., Martinez, P. F., et al. (2020). Intermittent fasting attenuates exercise training-induced cardiac remodeling. Arq. Bras. Cardiol. 115, 184–193. doi: 10.36660/abc.20190349
Bhutani, S., Klempel, M. C., Kroeger, C. M., Trepanowski, J. F., Phillips, S. A., Norkeviciute, E., et al. (2013). Alternate day fasting with or without exercise: effects on endothelial function and adipokines in obese humans. eSPEN J. 8, e205–e209. doi: 10.1016/j.clnme.2013.07.005
Brandhorst, S., and Longo, V. D. (2019). Dietary restrictions and nutrition in the prevention and treatment of cardiovascular disease. Circ. Res. 124, 952–965. doi: 10.1161/CIRCRESAHA.118.313352
Carvalho, M. R., Mendonça, M. L. M., Oliveira, J. M. L., Romanenghi, R. B., Morais, C. S., Ota, G. E., et al. (2021). Influence of high-intensity interval training and intermittent fasting on myocardium apoptosis pathway and cardiac morphology of healthy rats. Life Sci. 264:118697. doi: 10.1016/j.lfs.2020.118697
Castello, L., Froio, T., Maina, M., Cavallini, G., Biasi, F., Leonarduzzi, G., et al. (2010). Alternate-day fasting protects the rat heart against age-induced inflammation and fibrosis by inhibiting oxidative damage and NF-kB activation. Free Radic. Biol. Med. 48, 47–54. doi: 10.1016/j.freeradbiomed.2009.10.003
Chen, Y., Hagopian, K., McDonald, R. B., Bibus, D., López-Lluch, G., Villalba, J. M., et al. (2012). The influence of dietary lipid composition on skeletal muscle mitochondria from mice following 1 month of calorie restriction. J. Gerontol. A Biol. Sci. Med. Sci. 67, 1121–1131. doi: 10.1093/gerona/gls113
Colom, B., Oliver, J., Roca, P., and Garcia-Palmer, F. J. (2007). Caloric restriction and gender modulate cardiac muscle mitochondrial H2O2 production and oxidative damage. Cardiovasc. Res. 74, 456–465. doi: 10.1016/j.cardiores.2007.02.001
David, C. E. B., Lucas, A. M. B., Araújo, M. T. S., Coelho, B. N., Neto, J. B. S., Portela, B. R. C., et al. (2018). Calorie restriction attenuates hypertrophy-induced redox imbalance and mitochondrial ATP-sensitive K(+) channel repression. J. Nutr. Biochem. 62, 87–94. doi: 10.1016/j.jnutbio.2018.08.008
Dengo, A. L., Dennis, E. A., Orr, J. S., Marinik, E. L., Ehrlich, E., Davy, B. M., et al. (2010). Arterial destiffening with weight loss in overweight and obese middle-aged and older adults. Hypertension 55, 855–861. doi: 10.1161/HYPERTENSIONAHA.109.147850
Deus, A. F., Silva, V. L. D., de Souza, S. L. B., Mota, G. A. F., Sant'Ana, P. G., Vileigas, D. F.„, et al. (2019). Myocardial dysfunction after severe food restriction is linked to changes in the calcium-handling properties in rats. Nutrients 11:1985. doi: 10.3390/nu11091985
Di Daniele, N., Marrone, G., Di Lauro, M., Di Daniele, F., Palazzetti, D., Guerriero, C., et al. (2021). Effects of caloric restriction diet on arterial hypertension and endothelial dysfunction. Nutrients 13, 274. doi: 10.3390/nu13010274
Donato, A. J., Walker, A. E., Magerko, K. A., Bramwell, R. C., Black, A. D., Henson, G. D., et al. (2013). Life-long caloric restriction reduces oxidative stress and preserves nitric oxide bioavailability and function in arteries of old mice. Aging Cell 12, 772–783. doi: 10.1111/acel.12103
Ezad, N., and Borne, P. (2016). Does intermittent fasting improve microvascular endothelial function in healthy middle-aged subjects? Biol. Med. 8, 1–7. doi: 10.4172/0974-8369.1000337
Facchini, M., Malfatto, G., Sala, L., Silvestri, G., Fontana, P., Lafortuna, C., et al. (2003). Changes of autonomic cardiac profile after a 3-week integrated body weight reduction program in severely obese patients. J. Endocrinol. Invest. 26, 138–142. doi: 10.1007/BF03345142
Faitg, J., Leduc-Gaudet, J. P., Reynaud, O., Ferland, G., Gaudreau, P., and Gouspillou, G. (2019). Effects of aging and caloric restriction on fiber type composition, mitochondrial morphology and dynamics in rat oxidative and glycolytic muscles. Front. Physiol. 10:420. doi: 10.3389/fphys.2019.00420
Finckenberg, P., Eriksson, O., Baumann, M., Merasto, S., Lalowski, M. M., Levijoki, J., et al. (2012). Caloric restriction ameliorates angiotensin II-induced mitochondrial remodeling and cardiac hypertrophy. Hypertension 59, 76–84. doi: 10.1161/HYPERTENSIONAHA.111.179457
Fontana, L., Meyer, T. E., Klein, S., and Holloszy, J. O. (2004). Long-term calorie restriction is highly effective in reducing the risk for atherosclerosis in humans. Proc. Natl. Acad. Sci. U. S. A. 101, 6659–6663. doi: 10.1073/pnas.0308291101
Gabbita, S. P., Butterfield, D. A., Hensley, K., Shaw, W., and Carney, J. M. (1997). Aging and caloric restriction affect mitochondrial respiration and lipid membrane status: an electron paramagnetic resonance investigation. Free Radic. Biol. Med. 23, 191–201. doi: 10.1016/S0891-5849(97)00043-9
Gavras, I., and Gavras, H. (2002). Angiotensin II as a cardiovascular risk factor. J. Hum. Hypertens. 16(Suppl. 2), S2–6. doi: 10.1038/sj.jhh.1001392
Granado, M., Amor, S., Martín-Carro, B., Guerra-Menéndez, L., Tejera-Muñoz, A., González-Hedström, D. Á, et al. (2019). Caloric restriction attenuates aging-induced cardiac insulin resistance in male Wistar rats through activation of PI3K/Akt pathway. Nutr. Metab. Cardiovasc. Dis. 29, 97–105. doi: 10.1016/j.numecd.2018.09.005
Gredilla, R., Sanz, A., Lopez-Torres, M., and Barja, G. (2001). Caloric restriction decreases mitochondrial free radical generation at complex I and lowers oxidative damage to mitochondrial DNA in the rat heart. FASEB J. 15, 1589–1591. doi: 10.1096/fj.00-0764fje
Guo, Y., Luo, S., Ye, Y., Yin, S., Fan, J., and Xia, M. (2021). Intermittent fasting improves cardiometabolic risk factors and alters gut microbiota in metabolic syndrome Patients. J. Clin. Endocrinol. Metab. 106, 64–79. doi: 10.1210/clinem/dgaa644
Headland, M. L., Clifton, P. M., and Keogh, J. B. (2018). Effect of intermittent energy restriction on flow mediated dilatation, a measure of endothelial function: a short report. Int. J. Environ. Res. Public Health 15:1166. doi: 10.3390/ijerph15061166
Hoddy, K. K., Bhutani, S., Phillips, S. A., and Varady, K. A. (2016). Effects of different degrees of insulin resistance on endothelial function in obese adults undergoing alternate day fasting. Nutr. Healthy Aging 4, 63–71. doi: 10.3233/NHA-1611
Iffiú-Soltész, Z., Prévot, D., and Carpéné, C. (2009). Influence of prolonged fasting on monoamine oxidase and semicarbazide-sensitive amine oxidase activities in rat white adipose tissue. J. Physiol. Biochem. 65, 11–23. doi: 10.1007/BF03165965
Johnson, M. L., Distelmaier, K., Lanza, I. R., Irving, B. A., Robinson, M. M., Konopka, A. R., et al. (2016). Mechanism by which caloric restriction improves insulin sensitivity in sedentary obese adults. Diabetes 65, 74–84. doi: 10.2337/db15-0675
Judge, S., Judge, A., Grune, T., and Leeuwenburgh, C. (2004). Short-term CR decreases cardiac mitochondrial oxidant production but increases carbonyl content. Am. J. Physiol. Regul. Integr. Comp. Physiol. 286, R254–R259. doi: 10.1152/ajpregu.00502.2003
Katare, R. G., Kakinuma, Y., Arikawa, M., Yamasaki, F., and Sato, T. (2009). Chronic intermittent fasting improves the survival following large myocardial ischemia by activation of BDNF/VEGF/PI3K signaling pathway. J. Mol. Cell Cardiol. 46, 405–412. doi: 10.1016/j.yjmcc.2008.10.027
Katsarou, A. L., Katsilambros, N. L., and Koliaki, C. C. (2021). intermittent energy restriction, weight loss and cardiometabolic risk: a critical appraisal of evidence in humans. Healthcarel 9:495. doi: 10.3390/healthcare9050495
Ketonen, J., Pilvi, T., and Mervaala, E. (2010). Caloric restriction reverses high-fat diet-induced endothelial dysfunction and vascular superoxide production in C57Bl/6 mice. Heart Vessels 25, 254–262. doi: 10.1007/s00380-009-1182-x
Khraiwesh, H., López-Domínguez, J. A., López-Lluch, G., Navas, P., de Cabo, R., Ramsey, J. J., et al. (2013). Alterations of ultrastructural and fission/fusion markers in hepatocyte mitochondria from mice following calorie restriction with different dietary fats. J. Gerontol. A Biol. Sci. Med. Sci. 68, 1023–1034. doi: 10.1093/gerona/glt006
Kobara, M., Furumori-Yukiya, A., Kitamura, M., Matsumura, M., Ohigashi, M., Toba, H., et al. (2015). Short-term caloric restriction suppresses cardiac oxidative stress and hypertrophy caused by chronic pressure overload. J. Card. Fail. 21, 656–666. doi: 10.1016/j.cardfail.2015.04.016
Lambert, A. J., Wang, B., Yardley, J., Edwards, J., and Merry, B. J. (2004). The effect of aging and caloric restriction on mitochondrial protein density and oxygen consumption. Exp. Gerontol. 39, 289–295. doi: 10.1016/j.exger.2003.12.009
Lee, S. A., Sypniewski, C., Bensadon, B. A., McLaren, C., Donahoo, W. T., Sibille, K. T., et al. (2020). Determinants of adherence in time-restricted feeding in older adults: lessons from a pilot study. Nutrients 12:874. doi: 10.3390/nu12030874
López-Torres, M., Gredilla, R., Sanz, A., and Barja, G. (2002). Influence of aging and long-term caloric restriction on oxygen radical generation and oxidative DNA damage in rat liver mitochondria. Free Radic. Biol. Med. 32, 882–889. doi: 10.1016/S0891-5849(02)00773-6
Martens, C. R., Rossman, M. J., Mazzo, M. R., Jankowski, L. R., Nagy, E. E., Denman, B. A., et al. (2020). Short-term time-restricted feeding is safe and feasible in non-obese healthy midlife and older adults. Geroscience 42, 667–686. doi: 10.1007/s11357-020-00156-6
Most, J., Gilmore, L. A., Smith, S. R., Han, H., Ravussin, E., and Redman, L. M. (2018). Significant improvement in cardiometabolic health in healthy nonobese individuals during caloric restriction-induced weight loss and weight loss maintenance. Am. J. Physiol. Endocrinol. Metab. 314, E396–E405. doi: 10.1152/ajpendo.00261.2017
Niemann, B., Chen, Y., Issa, H., Silber, R. E., and Rohrbach, S. (2010). Caloric restriction delays cardiac ageing in rats: role of mitochondria. Cardiovasc. Res. 88, 267–276. doi: 10.1093/cvr/cvq273
Nisoli, E., Tonello, C., Cardile, A., Cozzi, V., Bracale, R., Tedesco, L., et al. (2005). Calorie restriction promotes mitochondrial biogenesis by inducing the expression of eNOS. Science 310, 314–317. doi: 10.1126/science.1117728
Nowosad, K., and Sujka, M. (2021). Effect of various types of intermittent fasting (IF) on weight loss and improvement of diabetic parameters in human. Curr. Nutr. Rep. 10, 146–154. doi: 10.1007/s13668-021-00353-5
Okoshi, K., Cezar, M. D. M., Polin, M. A. M., Paladino, J. R. Jr., Martinez, P. F., Oliveira, S. A., et al. (2019). Influence of intermittent fasting on myocardial infarction-induced cardiac remodeling. BMC Cardiovasc. Disord. 19:126. doi: 10.1186/s12872-019-1113-4
Palee, S., Jaiwongkam, T., Kerdphoo, S., Pratchayasakul, W., Chattipakorn, S. C., and Chattipakorn, N. (2020). Exercise with calorie restriction improves cardiac function via attenuating mitochondrial dysfunction in ovariectomized prediabetic rats. Exp. Gerontol. 135:110940. doi: 10.1016/j.exger.2020.110940
Pierce, G. L., Beske, S. D., Lawson, B. R., Southall, K. L., Benay, F. J., Donato, A. J., et al. (2008). Weight loss alone improves conduit and resistance artery endothelial function in young and older overweight/obese adults. Hypertension 52, 72–79. doi: 10.1161/HYPERTENSIONAHA.108.111427
Portero-Tresserra, M., Rojic-Becker, D., Vega-Carbajal, C., Guillazo-Blanch, G., Vale-Martínez, A., and Martí-Nicolovius, M. (2020). Caloric restriction modulates the monoaminergic system and metabolic hormones in aged rats. Sci. Rep. 10:19299. doi: 10.1038/s41598-020-76219-7
Qiu, X., Brown, K., Hirschey, M. D., Verdin, E., and Chen, D. (2010). Calorie restriction reduces oxidative stress by SIRT3-mediated SOD2 activation. Cell Metab. 12, 662–667. doi: 10.1016/j.cmet.2010.11.015
Razzak, R. L., Abu-Hozaifa, B. M., Bamosa, A. O., and Ali, N. M. (2011). Assessment of enhanced endothelium-dependent vasodilation by intermittent fasting in Wistar albino rats. Indian J. Physiol. Pharmacol. 55, 336–342.
Real-Hohn, A., Navegantes, C., Ramos, K., Ramos-Filho, D., Cahuê, F., Galina, A., et al. (2018). The synergism of high-intensity intermittent exercise and every-other-day intermittent fasting regimen on energy metabolism adaptations includes hexokinase activity and mitochondrial efficiency. PLoS ONE 13:e0202784. doi: 10.1371/journal.pone.0202784
Rippe, C., Lesniewski, L., Connell, M., LaRocca, T., Donato, A., and Seals, D. (2010). Short-term calorie restriction reverses vascular endothelial dysfunction in old mice by increasing nitric oxide and reducing oxidative stress. Aging Cell 9, 304–312. doi: 10.1111/j.1474-9726.2010.00557.x
Ristow, M. (2004). Neurodegenerative disorders associated with diabetes mellitus. J. Mol. Med. 82, 510–529. doi: 10.1007/s00109-004-0552-1
Sasaki, S., Higashi, Y., Nakagawa, K., Kimura, M., Noma, K., Sasaki, S., et al. (2002). A low-calorie diet improves endothelium-dependent vasodilation in obese patients with essential hypertension*. Am. J. Hypertens. 15, 302–309. doi: 10.1016/S0895-7061(01)02322-6
Schroder, J. D., Falqueto, H., Mânica, A., Zanini, D., de Oliveira, T., de Sá, C. A., et al. (2021). Effects of time-restricted feeding in weight loss, metabolic syndrome and cardiovascular risk in obese women. J. Transl. Med. 19:3. doi: 10.1186/s12967-020-02687-0
Senesi, P., Ferrulli, A., Luzi, L., and Terruzzi, I. (2021). Diabetes mellitus and cardiovascular diseases: nutraceutical interventions related to caloric restriction. Int. J. Mol. Sci. 22:7772. doi: 10.3390/ijms22157772
Serna, J. D. C., Caldeira da Silva, C. C., and Kowaltowski, A. J. (2020). Functional changes induced by caloric restriction in cardiac and skeletal muscle mitochondria. J. Bioenerg. Biomembr. 52, 269–277. doi: 10.1007/s10863-020-09838-4
Shinmura, K., Tamaki, K., Sano, M., Nakashima-Kamimura, N., Wolf, A. M., Amo, T., et al. (2011). Caloric restriction primes mitochondria for ischemic stress by deacetylating specific mitochondrial proteins of the electron transport chain. Circ. Res. 109, 396–406. doi: 10.1161/CIRCRESAHA.111.243097
Sohal, R. S., Ku, H. H., Agarwal, S., Forster, M. J., and Lal, H. (1994). Oxidative damage, mitochondrial oxidant generation and antioxidant defenses during aging and in response to food restriction in the mouse. Mech. Ageing Dev. 74, 121–133. doi: 10.1016/0047-6374(94)90104-X
Speakman, J. R., and Mitchell, S. E. (2011). Caloric restriction. Mol. Aspects Med. 32, 159–221. doi: 10.1016/j.mam.2011.07.001
Sturza, A., Olariu, S., Ionica, M., Duicu, O. M., Vaduva, A. O., Boia, E., et al. (2019). Monoamine oxidase is a source of oxidative stress in obese patients with chronic inflammation (1). Can. J. Physiol. Pharmacol. 97, 844–849. doi: 10.1139/cjpp-2019-0028
Varady, K. A., Bhutani, S., Church, E. C., and Klempel, M. C. (2009). Short-term modified alternate-day fasting: a novel dietary strategy for weight loss and cardioprotection in obese adults. Am. J. Clin. Nutr. 90, 1138–1143. doi: 10.3945/ajcn.2009.28380
Waldman, M., Cohen, K., Yadin, D., Nudelman, V., Gorfil, D., Laniado-Schwartzman, M., et al. (2018). Regulation of diabetic cardiomyopathy by caloric restriction is mediated by intracellular signaling pathways involving 'SIRT1 and PGC-1α'. Cardiovasc. Diabetol. 17:111. doi: 10.1186/s12933-018-0754-4
Wegman, M. P., Guo, M. H., Bennion, D. M., Shankar, M. N., Chrzanowski, S. M., Goldberg, L. A., et al. (2015). Practicality of intermittent fasting in humans and its effect on oxidative stress and genes related to aging and metabolism. Rejuvenation Res. 18, 162–172. doi: 10.1089/rej.2014.1624
Weiss, E. P., and Fontana, L. (2011). Caloric restriction: powerful protection for the aging heart and vasculature. Am. J. Physiol. Heart Circ. Physiol. 301, H1205–H1219. doi: 10.1152/ajpheart.00685.2011
Young, J. B., Mullen, D., and Landsberg, L. (1978). Caloric restriction lowers blood pressure in the spontaneously hypertensive rat. Metabolism 27, 1711–1714. doi: 10.1016/0026-0495(78)90256-1
Zanetti, M., Gortan Cappellari, G., Burekovic, I., Barazzoni, R., Stebel, M., and Guarnieri, G. (2010). Caloric restriction improves endothelial dysfunction during vascular aging: effects on nitric oxide synthase isoforms and oxidative stress in rat aorta. Exp. Gerontol. 45, 848–855. doi: 10.1016/j.exger.2010.07.002
Keywords: intermittent fasting, caloric restriction, cardiovascular disease, mitochondrial function, endothelial dysfunction
Citation: Savencu CE, Linţa A, Farcaş G, Bînă AM, Creţu OM, Maliţa DC, Muntean DM and Sturza A (2021) Impact of Dietary Restriction Regimens on Mitochondria, Heart, and Endothelial Function: A Brief Overview. Front. Physiol. 12:768383. doi: 10.3389/fphys.2021.768383
Received: 31 August 2021; Accepted: 17 November 2021;
Published: 16 December 2021.
Edited by:
Vladimir Lj Jakovljevic, University of Kragujevac, SerbiaReviewed by:
Christopher R. Martens, University of Delaware, United StatesCopyright © 2021 Savencu, Linţa, Farcaş, Bînă, Creţu, Maliţa, Muntean and Sturza. This is an open-access article distributed under the terms of the Creative Commons Attribution License (CC BY). The use, distribution or reproduction in other forums is permitted, provided the original author(s) and the copyright owner(s) are credited and that the original publication in this journal is cited, in accordance with accepted academic practice. No use, distribution or reproduction is permitted which does not comply with these terms.
*Correspondence: Daniel Claudiu Maliţa, ZG1hbGl0YUBnbWFpbC5jb20=; Danina Mirela Muntean, ZGFuaW5hbXVudGVhbkB1bWZ0LnJv
†These authors have contributed equally to this work
Disclaimer: All claims expressed in this article are solely those of the authors and do not necessarily represent those of their affiliated organizations, or those of the publisher, the editors and the reviewers. Any product that may be evaluated in this article or claim that may be made by its manufacturer is not guaranteed or endorsed by the publisher.
Research integrity at Frontiers
Learn more about the work of our research integrity team to safeguard the quality of each article we publish.