- State Key Laboratory of Metal Matrix Composites, School of Materials Science and Engineering, Shanghai Jiao Tong University, Shanghai, China
Due to the high morbidity and mortality of cardiovascular diseases, there is an urgent need for research on antithrombotic strategies. In view of the short half-life, insufficient drug penetration, poor targeting capabilities, and hemorrhagic side-effects of traditional thrombus treatment methods, the combination of thrombolytic therapy and nanocarriers brought by the development of nanotechnology in recent years may provide effective solutions for these undesirable side-effects caused by insufficient targeting. Polymeric nanocarriers, based on macromolecules and various functional groups, can connect specific targeting molecules together through chemical modification to achieve the protection and targeted delivery of thrombolytic drugs. However, simple chemical molecular modifications may be easily affected by the physiological environment encountered in the circulatory system. Therefore, the modification of nanocarriers with cell membranes can provide camouflage to these platforms and help to extend their circulation time while also imparting them with the biological functions of cell membranes, thus providing them with precise targeting capabilities, among which the most important is the biological modification of platelet membranes. In addition, some nanoparticles with their own therapeutic functions have also been developed, such as polypyrrole, which can exhibit a photothermal effect to induce thrombolysis. Herein, combined with the mechanism of thrombosis and thrombolysis, we outline the recent advances achieved with thrombus-targeting nanocarriers with regard to thrombosis treatment. On this basis, the design considerations, advantages, and challenges of these thrombolytic therapies in clinical transformation are discussed.
Introduction
At present, cardiovascular disease is still one of the most threatening diseases to human health and life in the world. Cardiovascular diseases have a high morbidity and mortality rate. According to statistics, approximately 179 million people die from cardiovascular diseases each year, accounting for about 31% of the world’s death toll, and this number is anticipated to reach over 236 million by 2030 (Benjamin et al., 2020; Zenych et al., 2020). There are three main types of cardiovascular diseases, including myocardial infarction, cerebral stroke, and deep vein thrombosis. These three cardiovascular diseases are primarily caused by thrombosis, which is a blood clot blockage of blood vessels (Fuster et al., 1992; Gao et al., 2021; Rodriguez and Harrington, 2021; Xue et al., 2021). Therefore, the prevention and treatment of thrombosis are an urgent issue, and it is also of great research significance.
Currently, the most important remedy for thrombosis and related cardiovascular diseases is prevention, but in cases involving long-term thrombosis, the main treatment options include balloon catheterization, surgical embolectomy, thrombolytic therapy, and other relevant surgeries (Powers et al., 2018). Taking into account the cost of surgical treatment and the damage it causes to the body, the application of thrombolytic drugs has increasingly become an important strategy. Thrombolytic agents include tissue plasminogen activators (tPA), recombinant tissue plasminogen activators (rtPA), urokinase (UK), streptokinase (SK), and other plasminogen activators (PAs; Marder, 2013; Cheng et al., 2018; Zamanlu et al., 2018; Ma et al., 2019; Hassanpour et al., 2020). The diverse antigenicity, half-life, lytic potential, fibrin specificity, and hemorrhagic risks associated with these agents lead to their differing effectiveness (Marshall, 2015). In addition, anticoagulants and antiplatelet drugs are often used to prevent clotting (Bala et al., 2018; Al Rawahi et al., 2019). These drugs often have only limited effectiveness when they are used alone, for example, their penetration into clots tends to be trivial and requires larger doses. Making matters worse, they may also cause hemorrhagic transformation leading to fatal intracerebral hemorrhage, as well as some other undesirable side-effects (Pfefferkorn and Rosenberg, 2003; Mao et al., 2017; Powers et al., 2018; Xu et al., 2021).
With the rapid development of nanotechnology and biotechnology (Jiang et al., 2020; Cornel et al., 2021), the combination of thrombolytic therapy and nanocarriers may provide a novel solution to key issues, such as the short half-life, low targeting ability, and unexpected bleeding complications of the existing therapeutic drugs (Zamanlu et al., 2018; Ma et al., 2019; Hassanpour et al., 2020). Polymeric nanocarriers based on macromolecules can provide the protection and targeted delivery of thrombolytic drugs through the unique physicochemical property brought by the nanoscale and the facile design of polymer chains. In addition, biologically inspired cell membrane modification strategies can transfer the specific functions and biological characteristics of cell membranes to the nanocarriers, thus improving the biocompatibility and precise targeting ability, and greatly enhancing the effect of thrombolytic therapy (Figure 1).
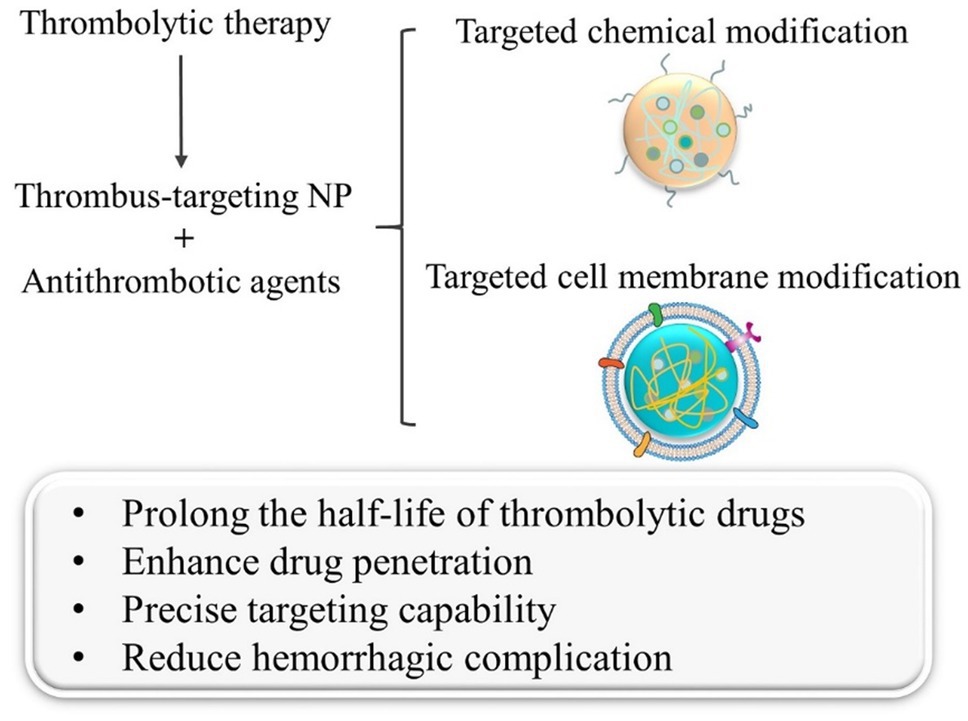
Figure 1. Schematic diagram of the two main modification methods in thrombus-targeting nanoparticles and their effects in thrombolytic therapy.
Mechanism of Thrombosis and Thrombolysis
Thrombosis is primarily triggered by collagen, tissue factor, thrombin, and other factors, which induce local platelet activation and fibronectin complex formation (Zenych et al., 2020). These key components jointly participate in the construction of the intravascular microenvironment that can lead to thrombosis and promote cancer. Fibrinogen is an important reaction substrate for thrombosis and is the key step involved in thrombosis (Undas and Ariens, 2011). When the endogenous coagulation system is abnormally activated, fibrinogen will form fibrin monomers under the action of thrombin, activated factor XIII, Ca2+, and other coagulation factors, subsequently covalently binding with each other to form a fibrin polymer. This stable fibrin network finally captures red blood cells, platelets, α2-antiplasmin, and other components to form a stable thrombus structure (Chernysh et al., 2011; Alkarithi et al., 2021).
A thrombus has a complex structure and composition, and notably, it bears numerous targetable receptors for nanocarriers that can themselves carry various ligands. By modifying the surfaces of nanocarriers with targeting moieties (such as antibodies, aptamers, peptides, and cell membrane proteins; Li et al., 2020), they can selectively target various biomarkers (P-selectin, Integrin GPIIb/IIIa, Factor XIII, and fibrin) at the thrombus site, thus achieving specific thrombus-targeting capabilities and enhancing the therapeutic effect due to the accumulation of thrombolytic drug at the surface of a clot (Karagkiozaki et al., 2015; Zenych et al., 2020). As far as the modification of the platelet membrane is concerned, the targeting mechanism may proceed via the upregulation of GP-Ib-V-IX glycoprotein complex and integrin GPIIb/IIIa residing on the surface of platelet membrane which in turn promotes the adhesion of fibrin and collagen, thus enabling the nanocarrier to target thrombi (Doshi et al., 2012; Chang et al., 2015).
In addition, the proportion of platelets is higher in the case of arterial thrombosis, while venous thrombosis contains more red blood cells and a denser fibrin network (Mackman, 2008; Martinelli et al., 2010). Consequently, existing strategies for thrombus treatment are primarily aimed at platelets and fibrin networks. For example, when the nanocarriers are targeted to reach the thrombus site, the plasminogen activator that they release can activate the plasminogen near the thrombus to become plasmin (Altaf et al., 2021), and then dissolve the cross-linked bonds between fibrin, destroy its network structure, and thus dissolve the blood clot (Figure 2; Collen and Lijnen, 2005; Hassanpour et al., 2020).
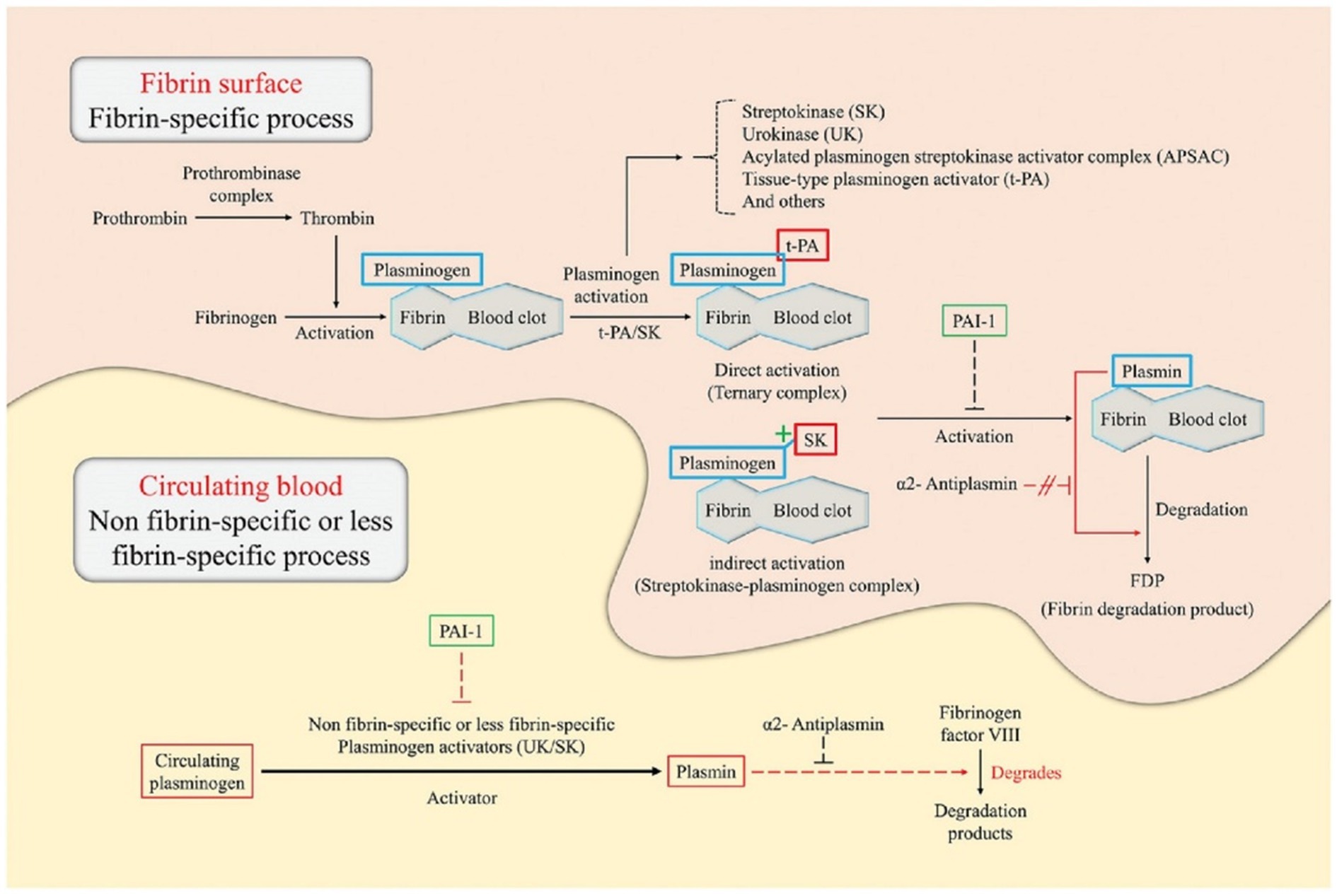
Figure 2. Illustration of the principles of thrombolysis in a fibrin surface and circulating blood environment (Hassanpour et al., 2020). This figure describes the catalytic principle of the conversion of plasminogen to plasmin according to the binding method of the plasminogen activator [e.g., tissue-type plasminogen activator (tPA), urokinase (UK), and streptokinase (SK)]) Plasminogen specifically binds to the surface of the fibrin blood clot. In direct activation, tPA preferentially attaches to plasminogen, resulting in the formation of a ternary complex. On the other hand, in indirect activation, SK cannot directly bind to the plasminogen but induce conformational changes of the plasminogen to form a streptokinase-plasminogen complex. Subsequently, these complexes form plasmin through cleavage of the fibrin-associated plasminogen. Plasmin formed by direct/indirect activation breaks down fibrin into fibrin degradation products, which eventually dissolves blood clots. The thrombolytic process in circulating blood is triggered by non-fibrin-specific or less fibrin-specific plasminogen activators. Plasminogen activators, such as the UK and SK, induce plasmin production by cleavage of circulating plasminogen. Subsequently, plasmin degrades fibrinogen factor VIII instead of fibrinogen. Plasmin activator inhibitor-1 acts on plasminogen, blocking cleavage into plasmin, and causing blood clot formation. α2-antiplasmin acts only on circulating blood and can inhibit thrombolysis by interfering with plasmin binding sites with fibrinogen factor VIII.
Targeted Chemical Modification of Nanocarriers and Applications
The development of antithrombotic therapy has been a high priority for many years (Zhao et al., 2020a). Owing to the rapid progression of nanotechnology, new antithrombotic nanotherapeutics have sprung up in recent years. The nanoscale characteristics of nanocarriers can introduce unique physical and chemical properties, and extend the circulation time of drugs in the bloodstream (Wang et al., 2018a; Zhang et al., 2018). Moreover, the long-chain structure of polymer macromolecules also facilitates the modification of these platforms for targeting capability and enables researchers to design nanocarriers and impart them with specific functions in order to meet a given requirement (Guo et al., 2018; Wang et al., 2018b; Shakiba et al., 2021). Therefore, the design, modification, and application of polymeric nanocarriers for thrombolytic therapy have become a significant research focus in recent years (He et al., 2021).
The upregulation of integrin GPIIb/IIIa on the surfaces of activated platelets is considered to be an important sign of thrombosis (Bai et al., 2020). The ligand corresponding to this integrin is known to be cyclic arginine-glycine-aspartic acid (cRGD; Barre, 2007; Ye et al., 2020). Therefore, Huang et al. reported an activated-platelet-sensitive nanocarrier capable of inducing selective thrombolysis through targeted delivery and controlled release of tPA to blood clot (Huang et al., 2019). The nanothrombolytic system loaded with tPA was based on PEGylated liposome, which can provide better in vivo stability. The incorporation of cRGD on the surface of liposome is the source of activated platelet sensitivity, it has been reported that cRGD peptide has high specificity and affinity for integrin GPIIb/IIIa on activated platelets (Ye et al., 2020).
Several studies have demonstrated that cRGD-modified nanosystems can effectively induce the selective lysis of fibrin and blood clots, thus suggesting that they are promising candidates for targeted thrombolytic therapy (Li et al., 2019; Zhang et al., 2019; Zhong et al., 2020). However, it is worth noting that studies have shown that platelet activation primarily occurs during the early stage of thrombosis, and the influence of integrin GPIIb/IIIa on platelet aggregation and thrombosis also decreases with the progression from the early to the late stage of the thrombosis process (Yan et al., 2000; Zhou et al., 2011; Gambino et al., 2021). In other words, the treatment brought by cRGD-modified GPIIb/IIIa-targeting nanocarriers primarily targets the early stages of thrombosis. So in practical applications, attention should thus be paid to the specific development stage of thrombosis and suit the right medicine to the case. In addition to the above systems, an organic semiconducting nanoparticle has also been chemically modified with cRGD peptides for use as a photoacoustic (PA) contrast agent for selectively reducing of early thrombosis (Cui et al., 2017), and the addition of the PA signal enhancing molecules can achieve specific imaging and lysis of blood clots (Wang et al., 2021).
Injection of rtPA is the standard drug treatment for thrombolysis (Hassanpour et al., 2020). However, due to the short half-life of rtPA, it only exhibits limited therapeutic efficiency even at high doses, and it brings a higher risk of bleeding, resulting in a 6% intracranial hemorrhage rate and a 50% chance of subsequent mortality (Yaghi et al., 2014). Therefore, polysaccharide-poly(isobutylcyanoacrylate) nanoparticles functionalized with fucoidan and loaded with rtPA were designed to accumulate on the surface of a thrombus (Juenet et al., 2018). Low-molecular weight fucoidan has a nanomolar affinity for P-selectin, which is expressed by activated platelets in thrombus (Bachelet et al., 2009; Zenych et al., 2021). Positively charged aminated dextran was incorporated into the polysaccharide shell to promote electrostatic interactions (Mukwaya et al., 2019), thereby mimicking the natural fibrin binding sites. The experimental results emphasized the relevance of targeting P-selectin for the treatment of thrombosis with rtPA for the first time, which is of great significance for the subsequent development of nanoparticles with P-selectin as the target of thrombosis treatment. But in the actual treatment process, it is best to use it in combination with other targets, because P-selectin can also be used as a target in tumor treatment (Farokhzad, 2015).
By taking into account of the biological characteristics of thrombosis, including the upregulation of H2O2 and the abundance of fibrin (Dayal et al., 2013; Andreadou et al., 2021), Zhao et al. (2020b) have developed a H2O2-responsive nanocarrier for the thrombus-targeting delivery of the antithrombotic agent tirofiban (Zhao et al., 2020b). This nanocarrier was composed of a dextran nanocore and a red blood cell (RBC) membrane shell, and tirofiban was conjugated to dextran through a H2O2-cleavable phenylboronic ester linkage. In contrast with the previous study on the nanosystem modified by fusion protein CREKA and H2O2-scavenging boronate group (Kang et al., 2017), the coating of the RBC membrane can enhance the circulation ability in vivo, and the functionalized peptide CREKA on RBC membrane can provide the desired thrombus-specific targeting capability. The ingenuity of the structural design in this study is that it can not only use the H2O2 to cleave the phenylboronic ester linkage to release thrombolytic drugs, but also effectively scavenges H2O2 and protects cells against H2O2-induced cytotoxicity. It is noteworthy that the platelet membrane may be more suitable for thrombus-targeting than the RBC membrane due to its unique thrombus-homing property, but tirofiban is likely to compromise the receptor on platelet membrane and thus may weaken the targeting capability (Chang et al., 2015). This work can also consider strengthening the research on the use of erythrocyte membrane to overcome barriers in biological microenvironments (Castro et al., 2021).
In addition, some nanoparticles with their own therapeutic functions have also been developed to utilize the photothermal effect as a means to achieve thrombolysis (Burnouf et al., 2019; Huang et al., 2020; Yuan et al., 2021). In order to avoid side-effects, such as hemorrhagic risk, that are generally associated with thrombolytic drugs, near-infrared light-mediated photothermal thrombolysis has been developed as a new treatment for thrombus (Ghosh and Pal, 2007; Singh et al., 2016). Following the initial work by Singh et al. (2016) and Chuang et al. (Mi et al., 2017; Satapathy et al., 2018), a new thrombolytic therapy utilizing photothermal decomposition of fibrin clots was explored by using dual-targeting glycol chitosan/heparin-modified polypyrrole nanoparticles to enhance targeted delivery and thrombolytic effect (Lu et al., 2021). Among them, glycol chitosan showed specific self-adaptive targeting capabilities in the acidic microenvironment of pathologically inflamed tissues at the thrombus (Lee et al., 2014), and heparin had potential biological affinity toward P-selectin that is highly expressed at the thrombus (Ludwig et al., 2004; Schwarz et al., 2020) and finally achieved high-efficiency thrombolysis by leveraging the photothermal effect exhibited by polypyrrole. In contrast with traditional thrombolytic therapy, this novel approach used external near-infrared light to cooperate with the nanoparticles delivered into the body to achieve thrombotic therapy and has promising prospects in the field of thrombolytic therapy.
Targeted Natural Cell Membrane Modification of Nanocarriers and Applications
Although great progress has been made with chemically modified polymeric nanocarriers with regard to targeted thrombolytic therapy, simple chemical molecular modification may be easily affected by the physiological environment that is encountered during the blood circulation process, thus resulting in inactivation of targeting molecules, agglomeration and adhesion of nanocarriers, and other issues. To address these issues, biologically inspired polymeric nanocarriers which were modified by cell membrane have been developed. This modification strategy serves to camouflage the nanocarriers and provides an extended circulation time, while also imparting the nanocarriers with the biological functions of natural cell membranes, so as to achieve more precise targeting capabilities (Xuan et al., 2019; Guan et al., 2021).
Inspired by the innate roles of platelets in hemostasis and pathological thrombus (Falati et al., 2002; Lippi et al., 2011), platelet membrane-camouflaged polymeric nanoparticles (nanoplatelets) have been developed to enable the targeted delivery of thrombolytic drug to local thrombus sites (Hu et al., 2015). In a recent study, Xu et al. (2020) bound a platelet membrane to the surface of poly(lactic-co-glycolic acid; PLGA) polymeric inner cores, and rtPA was then chemically conjugated to the activated sulfhydryl groups residing on the external surface of the platelet membrane to form PNP-PA (Xu et al., 2020). It was found that PNP-PA possesses the major membrane adhesion-associated proteins, which can be used to achieve targeted thrombolysis. In addition, researchers also determined the two most effective receptors on activated platelets for PNP-PA recruitment, namely, GPIIb/IIIa and P-selectin mentioned above. Furthermore, the analysis of in vivo coagulation indicators in different thrombosis models suggested that the nanoplatelets exhibit a low risk of bleeding complications. Therefore, this nanoplatelet strategy offers an integrated solution to address the drawbacks of clinically used thrombolytic drugs and has great potential to refine the current state of thrombosis treatment.
Using a conceptually similar strategy, Wang et al. (2020) developed platelet membrane-coated PLGA cores loading lumbrokinase as nanoplatelets (PNPs/LBK) to achieve effective thrombolysis with reduced hemorrhagic risk (Wang et al., 2020). Through platelet membrane coating, the circulation time of PNPs is as long as that of RBC membrane-coated nanoparticles. After the PNPs were anchored into the thrombus site through heteromultivalent ligand-mediated binding to active platelet integrin GPIIb/IIIa and P-selectin, the thrombolytic payload was released due to vesicle destabilization triggered by clot-relevant enzyme phospholipase-A2 (Pawlowski et al., 2017). Importantly, hemorrhagic tests reveal that the administration of free LBK leads to a significant prolongation of tail bleeding time, while administration of PNPs/LBK has little effect on the bleeding time. In addition, it is also a good choice to increase the function of responsive drug release on nanoplatelets, such as the use of hydrogen peroxide-responsive platelet membrane-coated nanoparticles for thrombus therapy (Zhao et al., 2021). These studies indicated that the nanoplatelets provide a promising thrombotherapeutic agent, which can effectively target the thrombus site, prolong the internal circulation time, and greatly reduce the hemorrhagic side-effects.
For traditional plasminogen activators, in addition to the hemorrhagic side-effects, there is also the risk of damaging the blood–brain barrier (BBB) and causing neurotoxic effects during ischemic stroke treatment (Su et al., 2008; Niego et al., 2012). In order to obtain the synergistic therapeutic effects provided by thrombolytics and neuroprotectants, a more complex dual-modified nanoplatelet (tP-NP-rtPA/ZL006e) has been developed, which was composed of a neuroprotectant-loaded dextran derivative core and a platelet membrane shell that was conjugated with thrombin-cleavable Tat-peptide-coupled rtPA (Xu et al., 2019). This dual-modified nanoplatelet can be used to sequentially deliver rtPA and the neuroprotectant (ZL006e) in a site-specific manner. After reaching the thrombus site through platelet membrane targeting, the release of rtPA was triggered by the upregulated thrombin, and the Tat peptide exposed in situ enhanced the penetration of nanoplatelet across the BBB into the ischemic brain for the site-specific delivery of ZL006e. In vitro and in vivo evaluation showed that tP-NP-rtPA/ZL006e could significantly improve the anti-ischemic stroke efficacy in rat models, enhance the neuroprotective effect. It would be better if this study could be combined with reducing hemorrhagic function.
In the future, the above-mentioned modification method of nanoplatelets can be used for reference, by pre-processing platelet cells to over-express certain proteins on the platelet membrane, and then transfer the platelet membrane to the surface of polymeric nanoparticle to obtain more functionalized nanosystems.
Conclusion
With the further study of thrombosis mechanisms and the continuous development of bio-nanotechnology, strategies for thrombus treatment have been constantly improved. Polymeric nanocarrier-based delivery systems have been developed to address a series of challenges that are still encountered in thrombolytic drug therapy, and the application of novel biomimetic cell membrane-modified nanocarriers in thrombolytic therapy has also become the focus of current research.
Many factors should be considered with regard to the design of nanocarrier-based drug delivery systems, such as the collocation and encapsulation between thrombolytic drugs and polymeric nanocarriers, the specific targeting function and responsive release function of the nanosystem, as well as the biocompatibility and biosafety. Utilizing the modifiability of polymer nanoparticles, a variety of targeting molecules (cRGD and heparin, etc.) can be modified simultaneously on polymer nanoparticles, while targeting integrin, P-selectin, and fibrin to improve the targeting accuracy of thrombolytic drugs. Moreover, the drug utilization and therapeutic effect can be improved by various responsive drug release modifications, such as shear-stress response modification on the surface of polymer nanoparticles. In addition, some corresponding imaging diagnosis and other functions can also be considered.
Unlike most tumors, thrombus sites are located in the bloodstream, so the thrombolytic agent can more easily reach the pathological target via the circulatory system without crossing layers of barriers. Consequently, it is more necessary for the antithrombotic nanosystems to have stronger targeting and adhesion characteristics, be able to target and bind into the thrombus site in the bloodstream, and achieve deep penetration.
In addition, there are still many areas that can be improved in order to advance nanocarrier-based thrombus treatment technology from the laboratory to clinical practice. Since thrombosis occurs at different locations and at varying development levels among different patients, it is possible to explore the utilization of fluorescent imaging, photoacoustic imaging and other diagnostic methods to determine the thrombosis development stage, and carry out corresponding personalized treatment, realize the integration of diagnosis, and treatment in the same nanosystem. If a platelet membrane-modified nanocarrier system is used, the patient’s own platelets can be used as the membrane source to achieve individualized treatment. Moreover, leveraging the facile design of biomimetic polymer nanosystems to strengthen the synergetic treatment of multiple therapies will help to combine the advantages of various thrombolytic strategies, enhance their active targeting capabilities, and reduce the undesirable side-effects, such as hemorrhagic risk and neurotoxicity of thrombolytic therapy.
In conclusion, although extensive research has been conducted in recent decades, the translation of thrombolytic therapy from the experimental research stage to clinical applications still faces many challenges. With the continuous optimization of nanomaterials and the rapid advancement of nanotechnology, more innovative and efficient biomimetic polymeric nanocarrier-based systems can be anticipated, which will provide versatile platforms and opportunities for significant advances in antithrombotic therapy.
Author Contributions
QG reviewed the literatures and wrote the manuscript. HD revised and finalized the manuscript. All authors contributed to the article and approved the submitted version.
Funding
This work was supported by the National Natural Science Foundation of China (No. 21871180), the Tracking Program for Professor of Special Appointment (Eastern Scholar) at Shanghai Institutions of Higher Learning (No. SHDP201802), the Science and Technology Commission of Shanghai Municipality (Nos. 18520710300 and 18JC1413500), and the Open Project of Translational Medicine of SJTU (No. TMSK-2021-108).
Conflict of Interest
The authors declare that the research was conducted in the absence of any commercial or financial relationships that could be construed as a potential conflict of interest.
Publisher’s Note
All claims expressed in this article are solely those of the authors and do not necessarily represent those of their affiliated organizations, or those of the publisher, the editors and the reviewers. Any product that may be evaluated in this article, or claim that may be made by its manufacturer, is not guaranteed or endorsed by the publisher.
Acknowledgments
The authors would like to thank the members of the research group for their helpful discussions.
References
Al Rawahi, B., Almegren, M., and Carrier, M. (2019). The efficacy and safety of anticoagulation in cerebral vein thrombosis: A systematic review and meta-analysis. Thromb. Res. 180:28. doi: 10.1016/j.thromres.2019.05.020
Alkarithi, G., Duval, C., Shi, Y., Macrae, F. L., and Ariens, R. A. S. (2021). Thrombus structural composition in cardiovascular disease. Arterioscler. Thromb. Vasc. Biol. 41, 2370–2383. doi: 10.1161/atvbaha.120.315754
Altaf, F., Wu, S., and Kasim, V. (2021). Role of Fibrinolytic enzymes in anti-thrombosis therapy. Front. Mol. Biosci. 8:476. doi: 10.3389/fmolb.2021.680397
Andreadou, I., Daiber, A., Baxter, G. F., Brizzi, M. F., Lisa, F. D., Kaludercic, N., et al. (2021). Influence of cardiometabolic comorbidities on myocardial function, infarction, and cardioprotection: role of cardiac redox signaling. Free Radic. Biol. Med. 166, 33–52. doi: 10.1016/j.freeradbiomed.2021.02.012
Bachelet, L., Bertholon, I., Lavigne, D., Vassy, R., Jandrot-Perrus, M., Chaubet, F., et al. (2009). Affinity of low molecular weight fucoidan for P-selectin triggers its binding to activated human platelets. Biochim. Biophys. Acta Gen. Subj. 1790, 141–146. doi: 10.1016/j.bbagen.2008.10.008
Bai, S., Liao, J., Zhang, B., Zhao, M., You, B., Li, P., et al. (2020). Multimodal and multifunctional nanoparticles with platelet targeting ability and phase transition efficiency for the molecular imaging and thrombolysis of coronary microthrombi. Biomater. Sci. 8, 5047–5060. doi: 10.1039/d0bm00818d
Bala, M. M., Paszek, E. B., Lesniak, W., Wloch-Kopec, D., Jasinska, K., and Undas, A. (2018). Antiplatelet and anticoagulant agents for primary prevention of thrombosis in individuals with antiphospholipid antibodies. Cochrane Database Syst. Rev. 7:CD012534. doi: 10.1002/14651858.CD012534.pub2
Barre, D. E. (2007). Arginyl-glycyl-aspartyl (RGD) epitope of human apolipoprotein (a) inhibits platelet aggregation by antagonizing the IIb subunit of the fibrinogen (GPIIb/IIIa) receptor. Thromb. Res. 119, 601–607. doi: 10.1016/j.thromres.2006.04.013
Benjamin, E. J., Muntner, P., Alonso, A., Bittencourt, M. S., Callaway, C. W., and Virani, S. S. (2020). Heart disease and stroke Statistics-2019 update: A report From the American Heart Association. Circulation 141:E33. doi: 10.1161/cir.0000000000000746
Burnouf, T., Chen, C.-H., Tan, S.-J., Tseng, C.-L., Lu, K.-Y., Chang, L.-H., et al. (2019). A bioinspired hyperthermic macrophage-based polypyrrole-polyethylenimine (Ppy-PEI) nanocomplex carrier to prevent and disrupt thrombotic fibrin clots. Acta Biomater. 96, 468–479. doi: 10.1016/j.actbio.2019.06.053
Castro, F., Martins, C., Silveira, M. J., Moura, R. P., Pereira, C. L., and Sarmento, B. (2021). Advances on erythrocyte-mimicking nanovehicles to overcome barriers in biological microenvironments. Adv. Drug Deliv. Rev. 170, 312–339. doi: 10.1016/j.addr.2020.09.001
Chang, S.-T., Chung, C.-M., Chu, C.-M., Yang, T.-Y., Pan, K.-L., Hsu, J.-T., et al. (2015). Platelet glycoprotein IIb/IIIa inhibitor Tirofiban ameliorates cardiac reperfusion injury. Int. Heart J. 56, 335–340. doi: 10.1536/ihj.14-322
Cheng, J.-W., Zhang, X.-J., Cheng, L.-S., Li, G.-Y., Zhang, L.-J., Ji, K.-X., et al. (2018). Low-dose tissue plasminogen activator in acute ischemic stroke: A systematic review and meta-analysis. J. Stroke Cerebrovasc. Dis. 27, 381–390. doi: 10.1016/j.jstrokecerebrovasdis.2017.09.014
Chernysh, I. N., Nagaswami, C., and Weisel, J. W. (2011). Visualization and identification of the structures formed during early stages of fibrin polymerization. Blood 117, 4609–4614. doi: 10.1182/blood-2010-07-297671
Collen, D., and Lijnen, H. R. (2005). Thrombolytic agents. Thromb. Haemost. 93, 627–630. doi: 10.1160/th04-11-0724
Cornel, E. J., Jiang, J., Chen, S., and Du, J. (2021). Principles and characteristics of polymerization-induced self-assembly with various polymerization techniques. CCS Chem. 3, 2104–2125. doi: 10.31635/ccschem.020.202000470
Cui, C., Yang, Z., Hu, X., Wu, J., Shou, K., Ma, H., et al. (2017). Organic semiconducting nanoparticles as efficient Photoacoustic agents for lightening early thrombus and monitoring thrombolysis in living mice. ACS Nano 11, 3298–3310. doi: 10.1021/acsnano.7b00594
Dayal, S., Wilson, K. M., Motto, D. G., Miller, F. J. Jr., Chauhan, A. K., and Lentz, S. R. (2013). Hydrogen peroxide promotes aging-related platelet Hyperactivation and thrombosis. Circulation 127, 1308–1316. doi: 10.1161/circulationaha.112.000966
Doshi, N., Orje, J. N., Molins, B., Smith, J. W., Mitragotri, S., and Ruggeri, Z. M. (2012). Platelet mimetic particles for targeting thrombi in flowing blood. Adv. Mater. 24, 3864–3869. doi: 10.1002/adma.201200607
Falati, S., Gross, P., Merrill-Skoloff, G., Furie, B. C., and Furie, B. (2002). Real-time in vivo imaging of platelets, tissue factor and fibrin during arterial thrombus formation in the mouse. Nat. Med. 8, 1175–1180. doi: 10.1038/nm782
Farokhzad, O. C. (2015). Nanotechnology Platelet mimicry. Nature 526, 47–48. doi: 10.1038/nature15218
Fuster, V., Badimon, L., Badimon, J. J., and Chesebro, J. H. (1992). The pathogenesis of coronary artery disease and the acute coronary syndromes. New Eng. J. Med. 326, 242–250. doi: 10.1056/nejm199201233260406
Gambino, G., Gambino, T., Connah, L., La Cava, F., Evrard, H., and Angelovski, G. (2021). RGD-peptide functionalization affects the In vivo diffusion of a responsive Trimeric MRI contrast agent through interactions with Integrins. J. Med. Chem. 64, 7565–7574. doi: 10.1021/acs.jmedchem.1c00264
Gao, Y., Liu, Y., Yang, X., Zhang, T., Hou, Y., Wang, P., et al. (2021). Pseudoginsenoside-F11 ameliorates thromboembolic stroke injury in rats by reducing thromboinflammation. Neurochem. Int. 149:105108. doi: 10.1016/j.neuint.2021.105108
Ghosh, S. K., and Pal, T. (2007). Interparticle coupling effect on the surface plasmon resonance of gold nanoparticles: From theory to applications. Chem. Rev. 107, 4797–4862. doi: 10.1021/cr0680282
Guan, Q., Guo, H., and Dou, H. (2021). Nanocarriers modified by cell membrane and their applications in tumor immunotherapy. Prog. Chem. 33, 150–167. doi: 10.7536/pc201019
Guo, H.-Z., Song, S., Dai, T.-T., Li, S.-L., and Dou, H.-J. (2018). Trypsin-responsive near-infrared fluorescent/magnetic resonance dual-imaging composite Nanospheres based on self-assembly. Acta Polym. Sin. 8, 1127–1140. doi: 10.11777/j.issn1000-3304.2018.18079
Hassanpour, S., Kim, H.-J., Saadati, A., Tebon, P., Xue, C., van den Dolder, F. W., et al. (2020). Thrombolytic agents: Nanocarriers in controlled release. Small 16:2001647. doi: 10.1002/smll.202001647
He, W., Zhang, Z., and Sha, X. (2021). Nanoparticles-mediated emerging approaches for effective treatment of ischemic stroke. Biomaterials 277:121111. doi: 10.1016/j.biomaterials.2021.121111
Hu, C.-M. J., Ronnie, H. F., Wang, K.-C., Luk, B. T., Thamphiwatana, S., Dehaini, D., et al. (2015). Nanoparticle biointerfacing by platelet membrane cloaking. Nature 526, 118–121. doi: 10.1038/nature15373
Huang, W., Gao, M., and Dou, H. (2020). Polypyrrole and its Nanocomposites applied in Photothermal therapy. Prog. Chem. 32, 371–380. doi: 10.7536/pc190906
Huang, Y., Yu, L., Ren, J., Gu, B., Longstaff, C., Hughes, A. D., et al. (2019). An activated-platelet-sensitive nanocarrier enables targeted delivery of tissue plasminogen activator for effective thrombolytic therapy. J. Control. Release 300, 1–12. doi: 10.1016/j.jconrel.2019.02.033
Jiang, J., Zhu, Y., and Du, J. (2020). Challenges and perspective on ring-opening polymerization-induced self-assembly. Acta Chim. Sin. 78, 719–724. doi: 10.6023/a20050162
Juenet, M., Aid-Launais, R., Li, B., Berger, A., Aerts, J., Ollivier, V., et al. (2018). Thrombolytic therapy based on fucoidan-functionalized polymer nanoparticles targeting P-selectin. Biomaterials 156, 204–216. doi: 10.1016/j.biomaterials.2017.11.047
Kang, C., Gwon, S., Song, C., Kang, P. M., Park, S.-C., Jeon, J., et al. (2017). Fibrin-targeted and H2O2-responsive nanoparticles as a Theranostics for Thrombosed vessels. ACS Nano 11, 6194–6203. doi: 10.1021/acsnano.7b02308
Karagkiozaki, V., Logothetidis, S., and Pappa, A.-M. (2015). Nanomedicine for atherosclerosis: molecular imaging and treatment. J. Biomed. Nanotechnol. 11, 191–210. doi: 10.1166/jbn.2015.1943
Lee, S. J., Min, H. S., Ku, S. H., Son, S., Kwon, I. C., Kim, S. H., et al. (2014). Tumor-targeting glycol chitosan nanoparticles as a platform delivery carrier in cancer diagnosis and therapy. Nanomedicine 9, 1697–1713. doi: 10.2217/nnm.14.99
Li, B., Chen, R., Zhang, Y., Zhao, L., Liang, H., Yan, Y., et al. (2019). RGD modified protein-polymer conjugates for pH-triggered targeted thrombolysis. ACS Appl. Bio Matter. 2, 437–446. doi: 10.1021/acsabm.8b00644
Li, M., Li, J., Chen, J., Liu, Y., Cheng, X., Yang, F., et al. (2020). Platelet membrane biomimetic magnetic Nanocarriers for targeted delivery and in situ generation of nitric oxide in early ischemic stroke. ACS Nano 14, 2024–2035. doi: 10.1021/acsnano.9b08587
Lippi, G., Franchini, M., and Targher, G. (2011). Arterial thrombus formation in cardiovascular disease. Nat. Rev. Cardiol. 8, 502–512. doi: 10.1038/nrcardio.2011.91
Lu, T.-Y., Chiang, C.-Y., Fan, Y.-J., Jheng, P.-R., Quinones, E. D., Liu, K.-T., et al. (2021). Dual-targeting glycol chitosan/heparin-decorated Polypyrrole nanoparticle for augmented Photothermal thrombolytic therapy. ACS Appl. Mater. Interfaces 13, 10287–10300. doi: 10.1021/acsami.0c20940
Ludwig, R. J., Boehme, B., Podda, M., Henschler, R., Jager, E., Tandi, C., et al. (2004). Endothelial P-selectin as a target of heparin action in experimental melanoma lung metastasis. Cancer Res. 64, 2743–2750. doi: 10.1158/0008-5472.can-03-1054
Ma, Y.-H., Liu, C.-H., Liang, Y., Chen, J.-P., and Wu, T. (2019). Targeted delivery of plasminogen activators for thrombolytic therapy: An integrative evaluation. Molecules 24, 1–15. doi: 10.3390/molecules24183407
Mackman, N. (2008). Triggers, targets and treatments for thrombosis. Nature 451, 914–918. doi: 10.1038/nature06797
Mao, L., Li, P., Zhu, W., Cai, W., Liu, Z., Wang, Y., et al. (2017). Regulatory T cells ameliorate tissue plasminogen activator-induced brain haemorrhage after stroke. Brain 140, 1914–1931. doi: 10.1093/brain/awx111
Marder, V. J. (2013). Thrombolytic Therapy. Cons. Hemo. Thrombosis. 28, 526–537. doi: 10.1016/b978-1-4557-2296-9.00028-2
Marshall, R. S. (2015). Progress in intravenous thrombolytic therapy for acute stroke. JAMA Neurol. 72, 928–934. doi: 10.1001/jamaneurol.2015.0835
Martinelli, I., Bucciarelli, P., and Mannucci, P. M. (2010). Thrombotic risk factors: basic pathophysiology. Crit. Care Med. 38, S3–S9. doi: 10.1097/CCM.0b013e3181c9cbd9
Mi, F.-L., Burnouf, T., Lu, S.-Y., Lu, Y.-J., Lu, K.-Y., Ho, Y.-C., et al. (2017). Self-targeting, immune transparent plasma protein coated Nanocomplex for noninvasive Photothermal anticancer therapy. Adv. Healthc. Mater. 6, 1–10. doi: 10.1002/adhm.201700181
Mukwaya, V., Wang, C., and Dou, H. (2019). Saccharide-based nanocarriers for targeted therapeutic and diagnostic applications. Polym. Int. 68, 306–319. doi: 10.1002/pi.5702
Niego, B. E., Freeman, R., Puschmann, T. B., Turnley, A. M., and Medcalf, R. L. (2012). T-PA-specific modulation of a human blood-brain barrier model involves plasmin-mediated activation of the rho kinase pathway in astrocytes. Blood 119, 4752–4761. doi: 10.1182/blood-2011-07-369512
Pawlowski, C. L., Li, W., Sun, M., Ravichandran, K., Hickman, D., Kos, C., et al. (2017). Platelet microparticle-inspired clot-responsive nanomedicine for targeted fibrinolysis. Biomaterials 128, 94–108. doi: 10.1016/j.biomaterials.2017.03.012
Pfefferkorn, T., and Rosenberg, G. A. (2003). Closure of the blood-brain barrier by matrix metalloproteinase inhibition reduces rtPA-mediated mortality in cerebral ischemia with delayed reperfusion. Stroke 34, 2025–2030. doi: 10.1161/01.str.0000083051.93319.28
Powers, W. J., Rabinstein, A. A., Ackerson, T., Adeoye, O. M., Bambakidis, N. C., and Tirschwell, D. L. (2018). 2018 guidelines for the early Management of Patients With Acute Ischemic Stroke: A guideline for healthcare professionals From the American Heart Association/American Stroke Association. Stroke 49, E233–E234. doi: 10.1161/str.0000000000000172
Rodriguez, F., and Harrington, R. A. (2021). Management of Antithrombotic Therapy after acute coronary syndromes. New Engl. J. Med. 384, 452–460. doi: 10.1056/NEJMra1607714
Satapathy, M. K., Nyambat, B., Chiang, C.-W., Chen, C.-H., Wong, P.-C., Ho, P.-H., et al. (2018). A gelatin hydrogel-containing Nano-organic PEI-Ppy with a Photothermal responsive effect for tissue engineering applications. Molecules 23, 1–17. doi: 10.3390/molecules23061256
Schwarz, S., Gockel, L. M., Naggi, A., Barash, U., Gobec, M., Bendas, G., et al. (2020). Glycosaminoglycans as tools to decipher the platelet tumor cell interaction: A focus on P-Selectin. Molecules 25, 1–16. doi: 10.3390/molecules25051039
Shakiba, S., Astete, C. E., Cueto, R., Rodrigues, D. F., Sabliov, C. M., and Louie, S. M. (2021). Asymmetric flow field-flow fractionation (AF4) with fluorescence and multi-detector analysis for direct, real-time, size-resolved measurements of drug release from polymeric nanoparticles. J. Control. Release 338, 410–421. doi: 10.1016/j.jconrel.2021.08.041
Singh, N., Varma, A., Verma, A., Maurya, B. N., and Dash, D. (2016). Relief from vascular occlusion using photothermal ablation of thrombus with a multimodal perspective. Nano Res. 9, 2327–2337. doi: 10.1007/s12274-016-1119-5
Su, E. J., Fredriksson, L., Geyer, M., Folestad, E., Cale, J., Andrae, J., et al. (2008). Activation of PDGF-CC by tissue plasminogen activator impairs blood-brain barrier integrity during ischemic stroke. Nat. Med. 14, 731–737. doi: 10.1038/nm1787
Undas, A., and Ariens, R. A. S. (2011). Fibrin clot structure and function A role in the pathophysiology of arterial and venous thromboembolic diseases. Arterioscler. Thromb. Vasc. Biol. 31, E88–E99. doi: 10.1161/atvbaha.111.230631
Wang, H., Dai, T.-T., Lu, B.-L., Li, S.-L., Lu, Q., Mukwaya, V., et al. (2018a). Hybrid dextran-gadolinium Nano-suitcases as high-relaxivity MRI contrast agents. Chin. J. Polym. Sci. 36, 391–398. doi: 10.1007/s10118-018-2083-1
Wang, J., Hu, X., and Xiang, D. (2018b). Nanoparticle drug delivery systems: an excellent carrier for tumor peptide vaccines. Drug Deliv. 25, 1319–1327. doi: 10.1080/10717544.2018.1477857
Wang, S., Wang, R., Meng, N., Guo, H., Wu, S., Wang, X., et al. (2020). Platelet membrane-functionalized nanoparticles with improved targeting ability and lower hemorrhagic risk for thrombolysis therapy. J. Control. Release 328, 78–86. doi: 10.1016/j.jconrel.2020.08.030
Wang, Y., Xu, X., Zhao, X., and Yin, Z. (2021). Functionalized polymeric hybrid micelles as an efficient nanotheranostic agent for thrombus imaging and thrombolysis. Acta Biomater. 122, 278–290. doi: 10.1016/j.actbio.2020.10.015
Xu, J., Wang, X., Yin, H., Cao, X., Hu, Q., Lv, W., et al. (2019). Sequentially site-specific delivery of Thrombolytics and Neuroprotectant for enhanced treatment of ischemic stroke. ACS Nano 13, 8577–8588. doi: 10.1021/acsnano.9b01798
Xu, J., Zhang, Y., and Nie, G. (2021). Intelligent antithrombotic nanomedicines: Progress, opportunities, and challenges. Viewpoints 2:20200145. doi: 10.1002/viw.20200145
Xu, J., Zhang, Y., Xu, J., Liu, G., Di, C., Zhao, X., et al. (2020). Engineered Nanoplatelets for targeted delivery of plasminogen activators to reverse thrombus in multiple mouse thrombosis models. Adv. Mater. 32:1905145. doi: 10.1002/adma.201905145
Xuan, M., Shao, J., and Li, J. (2019). Cell membrane-covered nanoparticles as biomaterials. Natl. Sci. Rev. 6, 551–561. doi: 10.1093/nsr/nwz037
Xue, J., Ma, D., Jiang, J., and Liu, Y. (2021). Diagnostic and prognostic value of immune/inflammation biomarkers for venous thromboembolism: is it reliable for clinical practice. J. Inflamm. Res. 14, 5059–5077. doi: 10.2147/jir.s327014
Yaghi, S., Eisenberger, A., and Willey, J. Z. (2014). Symptomatic Intracerebral hemorrhage in acute ischemic stroke After thrombolysis With intravenous recombinant tissue plasminogen activator A review of natural history and treatment. JAMA Neurol. 71, 1181–1185. doi: 10.1001/jamaneurol.2014.1210
Yan, B., Hu, D. D., Knowles, S. K., and Smith, J. W. (2000). Probing chemical and conformational differences in the resting and active conformers of platelet integrin alpha(IIb)beta(3). J. Biol. Chem. 275, 7249–7260. doi: 10.1074/jbc.275.10.7249
Ye, S., Liu, Y., Lu, Y., Ji, Y., Mei, L., Yang, M., et al. (2020). Cyclic RGD functionalized liposomes targeted to activated platelets for thrombosis dual-mode magnetic resonance imaging. J. Mat. Chem. 8, 447–453. doi: 10.1039/c9tb01834d
Yuan, J., Li, L., Yang, Q., Ran, H., Wang, J., Hu, K., et al. (2021). Targeted treatment of ischemic stroke by bioactive nanoparticle-derived reactive oxygen species responsive and inflammation-resolving Nanotherapies. ACS Nano 15, 16076–16094. doi: 10.1021/acsnano.1c04753
Zamanlu, M., Farhoudi, M., Eskandani, M., Mahmoudi, J., Barar, J., Rafi, M., et al. (2018). Recent advances in targeted delivery of tissue plasminogen activator for enhanced thrombolysis in ischaemic stroke. J. Drug Target. 26, 95–109. doi: 10.1080/1061186x.2017.1365874
Zenych, A., Fournier, L., and Chauvierre, C. (2020). Nanomedicine progress in thrombolytic therapy. Biomaterials 258, 120297–120225. doi: 10.1016/j.biomaterials.2020.120297
Zenych, A., Jacqmarcq, C., Aid, R., Fournier, L., Ramirez, L. M. F., Chaubet, F., et al. (2021). Fucoidan-functionalized polysaccharide submicroparticles loaded with alteplase for efficient targeted thrombolytic therapy. Biomaterials 277:121102. doi: 10.1016/j.biomaterials.2021.121102
Zhang, Y., Li, N., Suh, H., and Irvine, D. J. (2018). Nanoparticle anchoring targets immune agonists to tumors enabling anti-cancer immunity without systemic toxicity. Nat. Commun. 9, 6–15. doi: 10.1038/s41467-017-02251-3
Zhang, F., Liu, Y., Lei, J., Wang, S., Ji, X., Liu, H., et al. (2019). Metal-organic-framework-derived carbon nanostructures for site-specific dual-modality Photothermal/photodynamic thrombus therapy. Adv. Sci. 6, 1–8. doi: 10.1002/advs.201901378
Zhao, Y., Xie, R., Yodsanit, N., Ye, M., Wang, Y., and Gong, S. (2020a). Biomimetic fibrin-targeted and H2O2-responsive nanocarriers for thrombus therapy. Nano Today 35, 1–12. doi: 10.1016/j.nantod.2020.100986
Zhao, Y., Xie, R., Yodsanit, N., Ye, M., Wang, Y., Wang, B., et al. (2021). Hydrogen peroxide-responsive platelet membrane-coated nanoparticles for thrombus therapy. Biomater. Sci. 9, 2696–2708. doi: 10.1039/d0bm02125c
Zhao, Z., Yang, F., Zhang, X., Sun, J., He, Z., and Luo, C. (2020b). Emerging nanotherapeutics for antithrombotic treatment. Biomaterials 255:120200. doi: 10.1016/j.biomaterials.2020.120200
Zhong, Y., Gong, W. J., Gao, X. H., Li, Y. N., Liu, K., Hu, Y. G., et al. (2020). Synthesis and evaluation of a novel nanoparticle carrying urokinase used in targeted thrombolysis. J. Biomed. Mater. Res. Part A 108, 193–200. doi: 10.1002/jbm.a.36803
Keywords: thrombosis, polymeric nanocarriers, targeted delivery, biomimetic technology, antithrombotic strategies
Citation: Guan Q and Dou H (2021) Thrombus-Targeting Polymeric Nanocarriers and Their Biomedical Applications in Thrombolytic Therapy. Front. Physiol. 12:763085. doi: 10.3389/fphys.2021.763085
Edited by:
Kangkang Zhi, Shanghai Changzheng Hospital, ChinaReviewed by:
Huocong Huang, University of Texas Southwestern Medical Center, United StatesBing Xia, Nanjing Forestry University, China
Thi Tuong Vy Phan, Duy Tan University, Vietnam
Copyright © 2021 Guan and Dou. This is an open-access article distributed under the terms of the Creative Commons Attribution License (CC BY). The use, distribution or reproduction in other forums is permitted, provided the original author(s) and the copyright owner(s) are credited and that the original publication in this journal is cited, in accordance with accepted academic practice. No use, distribution or reproduction is permitted which does not comply with these terms.
*Correspondence: Hongjing Dou, hjdou@sjtu.edu.cn