- Department of International Tourism, Nagasaki International University, Nagasaki, Japan
Obesity and diabetes have been shown to interfere with energy metabolism and cause peripheral insulin resistance in skeletal muscle. However, recent studies have focused on the effect metabolic insult has on the loss of muscle size, strength, and physical function. Contractile dysfunction has been linked to impaired intracellular Ca2+ concentration ([Ca2+]i) regulation. In skeletal muscle, [Ca2+]i homeostasis is highly regulated by Ca2+ transport across the sarcolemma/plasma membrane, the golgi apparatus, sarcoplasmic reticulum (SR), and mitochondria. Particularly, the SR and or mitochondria play an important role in the fine-tuning of this metabolic process. Recent studies showed that obesity and insulin resistance are associated with interactions between the SR and mitochondrial networks (the dynamic tubular reticulum formed by mitochondria), suggesting that metabolic disorders alter Ca2+ handling by these organelles. These interactions are facilitated by specific membrane proteins, including ion channels. This review considers the impact of metabolic disorders, such as obesity and type 2 diabetes, on the regulation of [Ca2+]i in skeletal muscle. It also discusses the mechanisms by which this occurs, focusing chiefly on the SR and mitochondria networks. A deeper understanding of the effect of metabolic disorders on calcium handling might be useful for therapeutic strategies.
Introduction
Obesity due to overeating and lack of exercise has become one of the major burdens of modern societies and is associated with many comorbidities, including type 2 diabetes mellitus (T2DM). Skeletal muscle is important for maintaining healthy body composition, physical function, and locomotion. Obesity is likely to cause a decrease in muscle mass and to lower muscle strength, which is associated with decreased mobility (Freedman et al., 2002). Previous studies have shown decreased muscle strength is observed in obese and T2DM patients (Park et al., 2006; Maffiuletti et al., 2007). Consistent with these findings, recent studies using animal models confirmed that obesity and diabetes lead to decreased muscle contractile force normalized to muscle mass and decrease in muscle performance (Eshima et al., 2017b; Hurst et al., 2019). However, an understanding of the mechanism for dysfunction of muscle contraction in obesity and T2DM has not been fully elucidated.
Transient elevation of intracellular Ca2+ concentration ([Ca2+]i) is necessary for the initiation of tension development in skeletal muscle tissue (Allen et al., 2008). Dysfunction of muscle contraction in metabolic disease may depend on the impaired capacity for Ca2+ release and reuptake by the sarcoplasmic reticulum (SR; Westerblad et al., 2010). These features include decreased SR calcium release and or decreased SR calcium reuptake (Bruton et al., 2002; Bayley et al., 2016). We recently demonstrated Ca2+ regulatory impairments during muscle contraction in metabolic disease using the db/db mice, a common model of obesity associated with T2DM (Eshima et al., 2019). Similar findings were seen in diet-induced obese mice (Eshima et al., 2020). On the other hand, type 1 diabetes mellitus (T1DM) affects [Ca2+]i independent of SR (Eshima et al., 2013, 2015). In this regard, recent studies suggest that mitochondria play a major role in the [Ca2+]i buffering with evidence for increased mitochondrial Ca2+ concentration ([Ca2+]mito) during contractions in skeletal muscle (Shkryl and Shirokova, 2006; Ainbinder et al., 2015; Eshima et al., 2017a). Previous studies have shown the [Ca2+]mito increases during electrical stimulation-induced contractions in skeletal muscle in vitro, suggesting that mitochondria are involved in the regulation of [Ca2+]i (Aydin et al., 2009; Rossi et al., 2011; Yamada et al., 2012). Consistent with this, mitochondrial Ca2+ uptake is a key supporter of excitation-contraction coupling in skeletal myotubes (Eisner et al., 2014). Indeed, altered [Ca2+]mito is a common characteristic of some skeletal muscle myopathies (Debattisti et al., 2019; Favaro et al., 2019), suggesting that diabetic myopathy may also display elevated [Ca2+]mito. This review considers the impact of obesity and diabetes on calcium handling by skeletal muscle, focusing on the SR and mitochondria. We propose that interactions between these organelles in skeletal muscles of obese and T2DM animals and patients alter calcium handling by skeletal muscles (see Figure 1).
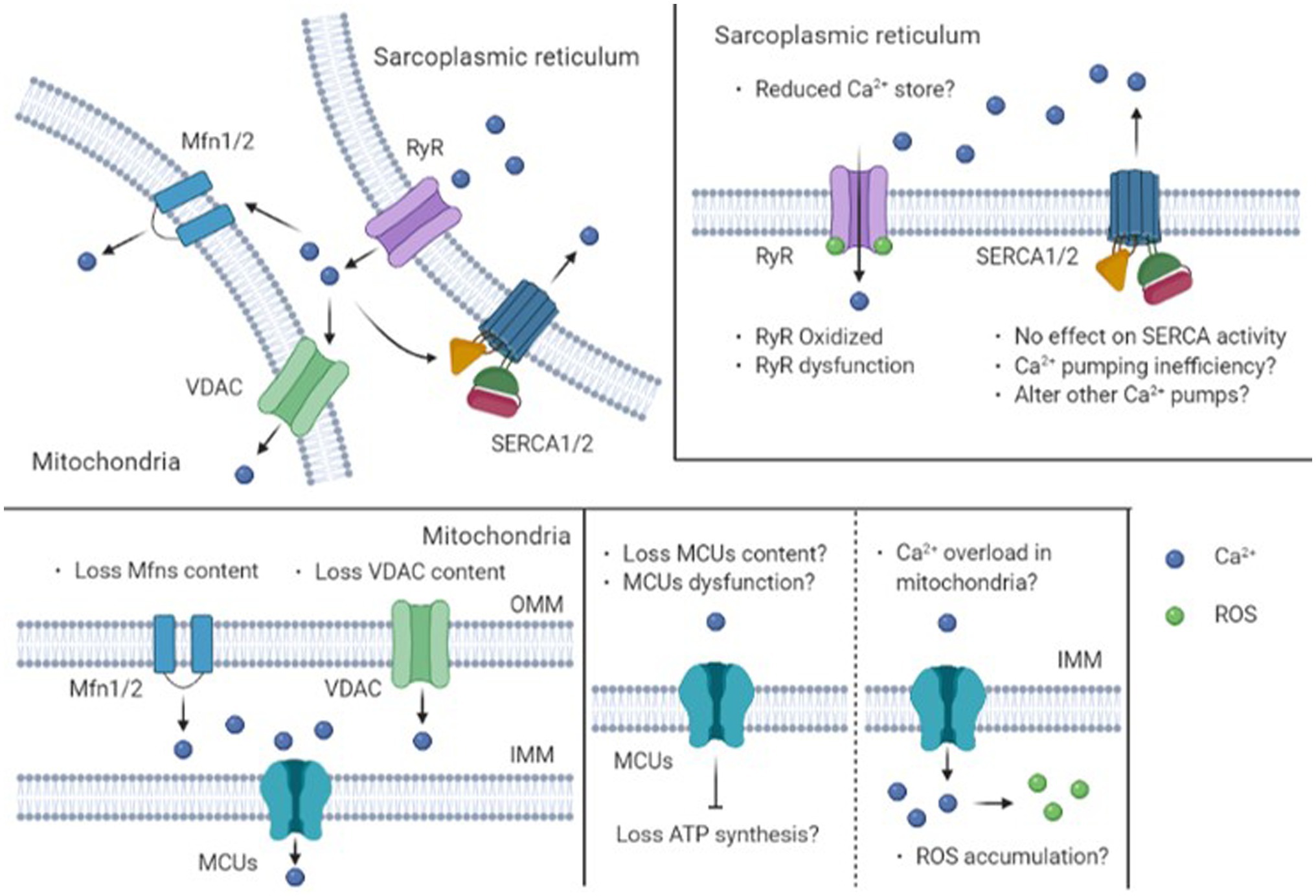
Figure 1. Schematic diagram of intracellular mechanisms for Ca2+ regulation by sarcoplasmic reticulum and mitochondria in obese and type 2 diabetic skeletal muscle. Obesity and type 2 diabetes mellitus may cause impairments in Ca2+ release capacity via a decrease in content or oxidation of RyR. On the other hand, there is no effect on SERCA activity. Note that the mitochondria also have an important sequestration role during the recovery process through a uniporter in OMM or IMM. IMM, The Inner Mitochondrial Membrane; MCUs, Mitochondrial Calcium Uniporter(s); Mfns, Mito Fusion 1 and/or 2; OMM, The Outer Mitochondrial Membrane; ROS, Reactive Oxygen Species; RyR, Ryanodine Receptor; SERCA, Sarcoplasmic reticulum Ca2+-ATPase; VDAC, Voltage Gated-Dependent Anion Channel.
Obesity And Type 2 Diabetes Alter Ca2+ Handling In Skeletal Muscle
Ca2+ is a ubiquitous intracellular messenger that can regulate different cellular processes in living tissue (Berridge et al., 2003). In particular, it is well known that elevated [Ca2+]i and subsequent Ca2+ signaling directly regulate cellular metabolism in various tissues. By contrast, obesity has been shown to impair Ca2+ homeostasis in adipose, cardiac, B-cell, and liver (Dong et al., 2006; Park et al., 2010; Tong et al., 2016; Wright et al., 2017). Therefore, certain aspects of Ca2+ homeostasis in skeletal muscle may also be compromised in obesity and T2DM. Previous findings related to alteration in SR or mitochondrial Ca2+ handling by obesity and type 2 diabetes are summarized in Table 1. Bruton et al. demonstrated that ob/ob mice (genetic-induced obesity model) impaired Ca2+ handling in skeletal muscle fibers (Bruton et al., 2002). Recently, we demonstrated electrically stimulated Ca2+ peak levels were reduced by HFD feeding. Indeed, Ca2+ peak levels during the ryanodine receptor (RyR) agonist stimulation decrease in this model (Eshima et al., 2020). Consistent with this, db/db mice (an obese type 2 diabetes model) displayed impaired contractile force and reduced SR Ca2+-ATPase (SERCA) pump expression (Bayley et al., 2016). Similarly, our study demonstrated a substantial degree of impairment in [Ca2+]i homeostasis in skeletal muscle of db/db mice (Eshima et al., 2019). Muscle contractile force and [Ca2+]i levels were both lower during electrical stimulation in this model, suggesting that decreased Ca2+ release may contribute to skeletal muscle contractile dysfunction in obese type 2 diabetic rodent models. In addition, Ca2+ release induced by the caffeine was decreased in db/db mice. Interestingly, dysfunction of Ca2+ release and contractile force was improved by endurance of exercise training in db/db mice. This study also found a consistent reduction in sarcalumenin, which is a Ca2+-binding protein localized in the SR of the intracellular Ca2+ store in the skeletal muscles of these mice. This protein decreases in aging animals (O'connell et al., 2008; Andersson et al., 2011). Collectively, it is expected that exercise intervention may increase the Ca2+ store content and improve calcium handling and contractile dysfunction associated with diabetic myopathy (Ferreira et al., 2010a).
Impact of Obesity and Type 2 Diabetes on Sarcoplasmic Reticulum and Mitochondrial Reticulum
Sarcoplasmic Reticulum
Ryanodine Receptor
In skeletal muscle, the increases in [Ca2+]i are initiated by sarcolemmal and transverse tubule depolarization, triggering Ca2+ release from the SR via the RyR. Previous studies demonstrated that T1DM impaired Ca2+ release from the SR and decreased RyR protein contents (Eshima et al., 2013, 2015). Similar to T1DM, RyR protein content was decreased in db/db mice (Eshima et al., 2019). By contrast, no differences in the RyR protein content between HFD-induced obese mice and mice fed a normal diet (Eshima et al., 2017b; Gamu et al., 2019). On the other hand, oxidation of RyR has been implicated in Ca2+ leakage from the SR that causes muscle weakness in aged mice (Andersson et al., 2011). Consistent with this finding, Holloway and colleagues demonstrated that the nitrosylated tyrosine residues on RyRs was increased in HFD-induced obese rats, suggesting that Ca2+ leakage from RyR is regulated by reactive oxygen species (ROS; Jain et al., 2014). Ceramides, a family of lipid molecules composed of sphingosine and fatty acid, have been implicated in the induction of oxidative stress in skeletal muscle (Nikolova-Karakashian and Reid, 2011). In murine C2C12 myotubes, direct exposure to ceramide increased ROS and exogenous ceramide depressed diaphragm force production in mice. This weakness was prevented by antioxidant N-acetylcysteine treatment (Ferreira et al., 2010b). Interestingly, sphingosine blocks Ca2+ release from RyR and reduces the activity of channels reconstituted into planar lipid bilayers (Sabbadini et al., 1999; Sharma et al., 2000). This suggests that lipid mediators may play important roles in calcium kinetics. Indeed, exogenous sphingolipids and accumulation of ceramide in muscle depresses Ca2+ sensitivity of the contractile apparatus (Ferreira et al., 2012). HFD feeding increased muscle ceramides and induced glucose intolerance in mice (Frangioudakis et al., 2010). This provides evidence to support the hypothesis that obesity alters lipid species composition, particularly sphingolipids, and causes impairments in Ca2+ release capacity via RyR dysfunction.
SR Ca2+-ATPase
Following muscle contraction, elevated [Ca2+]i rapidly decreases due to Ca2+ taken up by the SR and other intracellular organelles and returns almost immediately to basal resting levels via the activity of SR Ca2+-ATPase (SERCA). Most of the studies addressing calcium signaling have been focused on this protein which has a significant influence on skeletal muscle metabolism. A previous study demonstrates reduced SERCA content in db/db mouse (Bayley et al., 2016). In contrast, previous studies have shown genetic obese rodent models displayed no changes in SERCA protein expression levels independent of diabetes (Jain et al., 2014; Eshima et al., 2019). Indeed, many studies have shown that HFD-induced obese rodents displayed no changes in SERCA activity and protein content (Bal et al., 2012; Fajardo et al., 2017; Eshima et al., 2017b; Gamu et al., 2019). Phospholipid composition plays a major role in determining membrane fluidity, and HFD feeding is known to alter phospholipid species abundance in mice (Montgomery et al., 2017). A reduction in phosphatidylethanolamine (PE) has been associated with decreased SERCA activity (Funai et al., 2016). A recent study demonstrates the absence of phosphatidylethanolamine N-methyltransferase (PEMT) promotes an increase in metabolic rate and protects from diet-induced obesity, potentially due to decreasing SERCA efficiency in skeletal muscle. However, this lack of PE methyltransferase also causes muscle weakness (Verkerke et al., 2019). In humans, recent studies utilizing muscle biopsies obtained from obese and T2DM patients demonstrate SERCA expression levels were increased compared to muscle from healthy participants (Chae et al., 2018; Gancheva et al., 2019). These data suggest certain aspects of the Ca2+ uptake into the SR are upregulated in skeletal muscle of patients with obesity and T2DM patients. Therefore, fundamental questions regarding muscle Ca2+ buffering associated with obesity remain to be more fully addressed. It may also be insightful to consider relevant organelles other than the SR in order to resolve the mechanistic basis for Ca2+ buffering alterations in obese skeletal muscle.
Mitochondrial Networks
Mitochondria are the organelles responsible for ATP production by oxidative phosphorylation. Mitochondria are in a dynamic state of fusion and division with respect to other mitochondria forming dynamic tubular structures called mitochondrial networks. Mitochondrial fragmentation may be associated with metabolic disorders (Fealy et al., 2021). A previous study showed that mitochondria-associated endoplasmic reticulum membrane (MAM) integrity, VDAC1-inositol 1,4,5-trisphosphate receptor type1 (IP3R1) interactions are decreased in obese and T2DM patients, suggesting that metabolic disorders alter Ca2+ handling by these organelles (Tubbs et al., 2018). Indeed, recent evidence suggests that MAMs contribute to obesity and insulin resistance (Townsend et al., 2020). The mitochondrial networks are regulated by molecular structures in the mitochondrial membranes as indicated below.
Voltage-Dependent Anion Channel
Voltage-dependent anion channel (VDAC) is expressed on the outer mitochondrial membrane (OMM) and regulates mitochondrial oxidative phosphorylation. A previous study has shown decreased VDAC proteins and other mitochondrial related proteins in T2DM patients (Moller et al., 2017). VDAC overexpression affects the interaction between SR and mitochondria and enhances [Ca2+]mito in vitro (Rapizzi et al., 2002). These data suggest mitochondrial dysfunction might contribute to the impaired [Ca2+]i regulation in obesity and T2DM.
Mitofusin
Mitofusin (Mfn) participates in the fusion of the mitochondrial outer membranes of two adjacent mitochondria and may contribute to Ca2+ uptake into the mitochondria (Santel and Fuller, 2001). A previous study demonstrated Mfn2 knockdown decreased in [Ca2+]mito after muscle contraction in mouse skeletal muscle (Ainbinder et al., 2015). Many studies have shown that Mfn is implicated in obesity and T2DM [see reviews (Zorzano et al., 2009; Fealy et al., 2018)]. Multiple studies have shown that obese rodent models display decreased Mfn2 in skeletal muscle, but there is no direct evidence of Mfn2 involvement in [Ca2+]mito regulation (Bach et al., 2003; Kong et al., 2013; Liu et al., 2014; Li et al., 2018). In addition, SR-mitochondria interactions are reduced in obesity and T2DM in vivo and in vitro. This suggests the metabolic disease may influence the interactions between these organelles (Tubbs et al., 2018). SR-mitochondria interaction is also required for insulin action as seen by decreases in insulin signaling with dysfunction in organelle interaction. Indeed, silencing Mfn2 attenuates increased mitochondrial Ca2+ uptake induced by insulin action in skeletal muscle cells (Del Campo et al., 2014). While direct evidence to support such claims is still lacking, these observations support the hypothesis that obesity and T2DM may contribute to dysfunction of [Ca2+]mito regulation.
Mitochondrial Ca2+ Uniporters
The inner mitochondrial membranes of the mitochondria (IMM) contain mitochondrial Ca2+ uptake-related proteins, called the mitochondrial Ca2+ uniporters (MCU; Baughman et al., 2011). The MCUs are regulated by mitochondrial Ca2+ uptake 1 (MICU1), MICU2, and mitochondrial calcium uniporter regulator 1 (MCUR1), which binds Ca2+ with high affinity and promotes uptake by mitochondria (Mammucari et al., 2016). Overexpressing MCU increases the mitochondrial size and causes muscle hypertrophy (Barclay et al., 2007; Mammucari et al., 2015). In contrast, other studies have shown the opposite alteration patterns in mitochondrial calcium uniporter related proteins in T2DM and upregulation of other proteins related to Ca2+ transporter/homeostasis (Hwang et al., 2010; Chae et al., 2018). In heart muscle from individuals with T1DM, impaired mitochondrial Ca2+ uptake is significantly improved by MCU restoration (Suarez et al., 2018). A previous study demonstrated that the mitochondrial calcium retention capacity is reduced in diet-induced obese mice, suggesting that dysregulation of MCU components is associated with insulin resistance (Taddeo et al., 2014). Collectively, this evidence suggests the possibility of a heretofore unappreciated role for [Ca2+]mito regulation in the function of obese and diabetic muscle.
Conclusion
The present review addressed how obesity and T2DM influence Ca2+ handling in skeletal muscle. Recent findings from studies in rodents demonstrated that genetic- and diet-induced obesity has detrimental effects on Ca2+ handling. As shown in Figure 1, this observation may be associated with impaired SR Ca2+ release and mitochondrial Ca2+ uptake. These dysfunctions may be explained at least in part by a decrease in RyR content (or oxidation), a decrease in VDAC, mitofusin, and MCU in diabetic and obese skeletal muscle. These mechanisms are likely to be responsible for the muscle weakness that occurs as a result of obesity and T2DM and may prove useful for defining the optimal therapeutic strategies. Previous studies showed that patients with malignant hyperthermia have altered SR Ca2+ release and impaired glucose tolerance (Altamirano et al., 2019; Bojko et al., 2021), suggesting that calcium handling is associated with glucose metabolism. Although mitochondrial calcium uniporter influences on systemic metabolism (Gherardi et al., 2019), the effect of mitochondrial Ca2+ uptake on metabolic disorders has not been fully clarified. Future studies will allow us to determine potential physiological mechanisms involving the SR and mitochondrial networks that are responsible for the impairment of Ca2+ homeostasis in skeletal muscle under conditions of metabolic disease.
Author Contributions
HE drafted the manuscript, prepared a figure, edited, and revised the manuscript and approved the final version of manuscript.
Funding
This article was supported by the KAKENHI from the Ministry of Education, Culture, Sports, Science, and Technology of Japan.
Conflict of Interest
The author declares that the research was conducted in the absence of any commercial or financial relationships that could be construed as a potential conflict of interest.
Publisher’s Note
All claims expressed in this article are solely those of the authors and do not necessarily represent those of their affiliated organizations, or those of the publisher, the editors and the reviewers. Any product that may be evaluated in this article, or claim that may be made by its manufacturer, is not guaranteed or endorsed by the publisher.
Acknowledgments
I gratefully acknowledge Justin Shahtout at University of Utah for helpful comments on the manuscript. A figure was created with Biorender.com.
Abbreviations
[Ca2+]i, Intracellular Ca2+ concentration; [Ca2+]mito, Mitochondrial Ca2+ concentration; CSQ, Calsequestrin; DHPR, Dihydropyridine receptor; HFD, High-fat diet; IMM, Inner mitochondrial membranes of the mitochondria; IP3R1, inositol 1,4,5-trisphosphate receptor type 1; MAM, Mitochondria-associated endoplasmic reticulum membrane; MCU, Mitochondrial Ca2+ uniporter; MCUR1, Mitochondrial calcium uniporter regulator 1; Mfn, Mitofusin; MICU1, Mitochondrial Ca2+ uptake 1; MICU2, Mitochondrial Ca2+ uptake 2; OMM, Outer mitochondrial membrane; PE, Phosphatidylethanolamine; PEMT, phosphatidylethanolamine N-methyltransferase; ROS, Reactive oxygen species; RyR, Ryanodine receptor; SERCA, SR Ca2+-ATPase; T1DM, Type 1 diabetes mellitus; T2DM, Type 2 diabetes mellitus; VDAC, Voltage-dependent anion channel; SLN, Sarcolipin; SR, Sarcoplasmic reticulum.
References
Agil, A., Elmahallawy, E. K., Rodriguez-Ferrer, J. M., Adem, A., Bastaki, S. M., Al-Abbadi, I., et al. (2015). Melatonin increases intracellular calcium in the liver, muscle, white adipose tissues and pancreas of diabetic obese rats. Food Funct. 6, 2671–2678. doi: 10.1039/C5FO00590F
Ainbinder, A., Boncompagni, S., Protasi, F., and Dirksen, R. T. (2015). Role of Mitofusin-2 in mitochondrial localization and calcium uptake in skeletal muscle. Cell Calcium 57, 14–24. doi: 10.1016/j.ceca.2014.11.002
Allen, D. G., Lamb, G. D., and Westerblad, H. (2008). Skeletal muscle fatigue: cellular mechanisms. Physiol. Rev. 88, 287–332. doi: 10.1152/physrev.00015.2007
Altamirano, F., Riazi, S., Ibarra Moreno, C. A., Kraeva, N., Uryash, A., Allen, P. D., et al. (2019). Is malignant hyperthermia associated with hyperglycaemia? Br. J. Anaesth. 122, e3–e5. doi: 10.1016/j.bja.2018.09.014
Andersson, D. C., Betzenhauser, M. J., Reiken, S., Meli, A. C., Umanskaya, A., Xie, W., et al. (2011). Ryanodine receptor oxidation causes intracellular calcium leak and muscle weakness in aging. Cell Metab. 14, 196–207. doi: 10.1016/j.cmet.2011.05.014
Aydin, J., Andersson, D. C., Hanninen, S. L., Wredenberg, A., Tavi, P., Park, C. B., et al. (2009). Increased mitochondrial Ca2+ and decreased sarcoplasmic reticulum Ca2+ in mitochondrial myopathy. Hum. Mol. Genet. 18, 278–288. doi: 10.1093/hmg/ddn355
Bach, D., Naon, D., Pich, S., Soriano, F. X., Vega, N., Rieusset, J., et al. (2005). Expression of Mfn2, the Charcot-Marie-tooth neuropathy type 2A gene, in human skeletal muscle: effects of type 2 diabetes, obesity, weight loss, and the regulatory role of tumor necrosis factor alpha and interleukin-6. Diabetes 54, 2685–2693. doi: 10.2337/diabetes.54.9.2685
Bach, D., Pich, S., Soriano, F. X., Vega, N., Baumgartner, B., Oriola, J., et al. (2003). Mitofusin-2 determines mitochondrial network architecture and mitochondrial metabolism. A novel regulatory mechanism altered in obesity. J. Biol. Chem. 278, 17190–17197. doi: 10.1074/jbc.M212754200
Bal, N. C., Maurya, S. K., Sopariwala, D. H., Sahoo, S. K., Gupta, S. C., Shaikh, S. A., et al. (2012). Sarcolipin is a newly identified regulator of muscle-based thermogenesis in mammals. Nat. Med. 18, 1575–1579. doi: 10.1038/nm.2897
Barclay, C. J., Woledge, R. C., and Curtin, N. A. (2007). Energy turnover for Ca2+ cycling in skeletal muscle. J. Muscle Res. Cell Motil. 28, 259–274. doi: 10.1007/s10974-007-9116-7
Baughman, J. M., Perocchi, F., Girgis, H. S., Plovanich, M., Belcher-Timme, C. A., Sancak, Y., et al. (2011). Integrative genomics identifies MCU as an essential component of the mitochondrial calcium uniporter. Nature 476, 341–345. doi: 10.1038/nature10234
Bayley, J. S., Pedersen, T. H., and Nielsen, O. B. (2016). Skeletal muscle dysfunction in the db/db mouse model of type 2 diabetes. Muscle Nerve 54, 460–468. doi: 10.1002/mus.25064
Berridge, M. J., Bootman, M. D., and Roderick, H. L. (2003). Calcium signalling: dynamics, homeostasis and remodelling. Nat. Rev. Mol. Cell Biol. 4, 517–529. doi: 10.1038/nrm1155
Bojko, B., Vasiljevic, T., Boyaci, E., Roszkowska, A., Kraeva, N., Ibarra Moreno, C. A., et al. (2021). Untargeted metabolomics profiling of skeletal muscle samples from malignant hyperthermia susceptible patients. Can. J. Anaesth. 68, 761–772. doi: 10.1007/s12630-020-01895-y
Bruton, J. D., Katz, A., Lannergren, J., Abbate, F., and Westerblad, H. (2002). Regulation of myoplasmic ca(2+) in genetically obese (Ob/Ob) mouse single skeletal muscle fibres. Pflugers Arch. 444, 692–699. doi: 10.1007/s00424-002-0882-1
Chae, S., Kim, S. J., Do Koo, Y., Lee, J. H., Kim, H., Ahn, B. Y., et al. (2018). A mitochondrial proteome profile indicative of type 2 diabetes mellitus in skeletal muscles. Exp. Mol. Med. 50, 1–14. doi: 10.1038/s12276-018-0154-6
Ciapaite, J., Van Den Berg, S. A., Houten, S. M., Nicolay, K., Van Dijk, K. W., and Jeneson, J. A. (2015). Fiber-type-specific sensitivities and phenotypic adaptations to dietary fat overload differentially impact fast- versus slow-twitch muscle contractile function in C57BL/6J mice. J. Nutr. Biochem. 26, 155–164. doi: 10.1016/j.jnutbio.2014.09.014
Debattisti, V., Horn, A., Singh, R., Seifert, E. L., Hogarth, M. W., Mazala, D. A., et al. (2019). Dysregulation of mitochondrial ca(2+) uptake and sarcolemma repair underlie muscle weakness and wasting in patients and mice lacking MICU1. Cell Rep. 29:e1276. doi: 10.1016/j.celrep.2019.09.063
Del Campo, A., Parra, V., Vasquez-Trincado, C., Gutierrez, T., Morales, P. E., Lopez-Crisosto, C., et al. (2014). Mitochondrial fragmentation impairs insulin-dependent glucose uptake by modulating Akt activity through mitochondrial Ca2+ uptake. Am. J. Physiol. Endocrinol. Metab. 306, E1–E13. doi: 10.1152/ajpendo.00146.2013
Dong, F., Zhang, X., Yang, X., Esberg, L. B., Yang, H., Zhang, Z., et al. (2006). Impaired cardiac contractile function in ventricular myocytes from leptin-deficient Ob/Ob obese mice. J. Endocrinol. 188, 25–36. doi: 10.1677/joe.1.06241
Eisner, V., Lenaers, G., and Hajnoczky, G. (2014). Mitochondrial fusion is frequent in skeletal muscle and supports excitation-contraction coupling. J. Cell Biol. 205, 179–195. doi: 10.1083/jcb.201312066
Eshima, H., Miura, S., Senoo, N., Hatakeyama, K., Poole, D. C., and Kano, Y. (2017a). Improved skeletal muscle ca(2+) regulation in vivo following contractions in mice overexpressing PGC-1alpha. Am. J. Physiol. Regul. Integr. Comp. Physiol. 312, R1017–R1028. doi: 10.1152/ajpregu.00032.2017
Eshima, H., Poole, D. C., and Kano, Y. (2015). In vivo Ca2+ buffering capacity and microvascular oxygen pressures following muscle contractions in diabetic rat skeletal muscles: fiber-type specific effects. Am. J. Physiol. Regul. Integr. Comp. Physiol. 309, R128–R137. doi: 10.1152/ajpregu.00044.2015
Eshima, H., Tamura, Y., Kakehi, S., Kakigi, R., Hashimoto, R., Funai, K., et al. (2020). A chronic high-fat diet exacerbates contractile dysfunction with impaired intracellular ca(2+) release capacity in the skeletal muscle of aged mice. J. Appl. Physiol. 128, 1153–1162. doi: 10.1152/japplphysiol.00530.2019
Eshima, H., Tamura, Y., Kakehi, S., Kurebayashi, N., Murayama, T., Nakamura, K., et al. (2017b). Long-term, but not short-term high-fat diet induces fiber composition changes and impaired contractile force in mouse fast-twitch skeletal muscle. Physiol. Rep. 5:e13250. doi: 10.14814/phy2.13250
Eshima, H., Tamura, Y., Kakehi, S., Nakamura, K., Kurebayashi, N., Murayama, T., et al. (2019). Dysfunction of muscle contraction with impaired intracellular ca(2+) handling in skeletal muscle and the effect of exercise training in male db/db mice. J. Appl. Physiol. 126, 170–182. doi: 10.1152/japplphysiol.00048.2018
Eshima, H., Tanaka, Y., Sonobe, T., Inagaki, T., Nakajima, T., Poole, D. C., et al. (2013). In vivo imaging of intracellular Ca2+ after muscle contractions and direct Ca2+ injection in rat skeletal muscle in diabetes. Am. J. Physiol. Regul. Integr. Comp. Physiol. 305, R610–R618. doi: 10.1152/ajpregu.00023.2013
Fajardo, V. A., Trojanowski, N., Castelli, L. M., Miotto, P. M., Amoye, F., Ward, W. E., et al. (2017). Saturation of SERCA's lipid annulus may protect against its thermal inactivation. Biochem. Biophys. Res. Commun. 484, 456–460. doi: 10.1016/j.bbrc.2017.01.154
Favaro, G., Romanello, V., Varanita, T., Andrea Desbats, M., Morbidoni, V., Tezze, C., et al. (2019). DRP1-mediated mitochondrial shape controls calcium homeostasis and muscle mass. Nat. Commun. 10:2576. doi: 10.1038/s41467-019-10226-9
Fealy, C. E., Grevendonk, L., Hoeks, J., and Hesselink, M. K. C. (2021). Skeletal muscle mitochondrial network dynamics in metabolic disorders and aging. Trends Mol. Med. doi: 10.1016/j.molmed.2021.07.013 (in press).
Fealy, C. E., Mulya, A., Axelrod, C. L., and Kirwan, J. P. (2018). Mitochondrial dynamics in skeletal muscle insulin resistance and type 2 diabetes. Transl. Res. 202, 69–82. doi: 10.1016/j.trsl.2018.07.011
Ferreira, J. C., Bacurau, A. V., Bueno, C. R. Jr., Cunha, T. C., Tanaka, L. Y., Jardim, M. A., et al. (2010a). Aerobic exercise training improves Ca2+ handling and redox status of skeletal muscle in mice. Exp. Biol. Med. 235, 497–505. doi: 10.1258/ebm.2009.009165
Ferreira, L. F., Moylan, J. S., Gilliam, L. A., Smith, J. D., Nikolova-Karakashian, M., and Reid, M. B. (2010b). Sphingomyelinase stimulates oxidant signaling to weaken skeletal muscle and promote fatigue. Am. J. Physiol. Cell Physiol. 299, C552–C560. doi: 10.1152/ajpcell.00065.2010
Ferreira, L. F., Moylan, J. S., Stasko, S., Smith, J. D., Campbell, K. S., and Reid, M. B. (2012). Sphingomyelinase depresses force and calcium sensitivity of the contractile apparatus in mouse diaphragm muscle fibers. J. Appl. Physiol. 112, 1538–1545. doi: 10.1152/japplphysiol.01269.2011
Frangioudakis, G., Garrard, J., Raddatz, K., Nadler, J. L., Mitchell, T. W., and Schmitz-Peiffer, C. (2010). Saturated- and n-6 polyunsaturated-fat diets each induce ceramide accumulation in mouse skeletal muscle: reversal and improvement of glucose tolerance by lipid metabolism inhibitors. Endocrinology 151, 4187–4196. doi: 10.1210/en.2010-0250
Fraser, D. R., and Trayhurn, P. (1983). Mitochondrial Ca2+ transport in lean and genetically obese (Ob/Ob) mice. Biochem. J. 214, 163–170. doi: 10.1042/bj2140163
Freedman, D. S., Khan, L. K., Serdula, M. K., Galuska, D. A., and Dietz, W. H. (2002). Trends and correlates of class 3 obesity in the United States from 1990 through 2000. JAMA 288, 1758–1761. doi: 10.1001/jama.288.14.1758
Funai, K., Lodhi, I. J., Spears, L. D., Yin, L., Song, H., Klein, S., et al. (2016). Skeletal muscle phospholipid metabolism regulates insulin sensitivity and contractile function. Diabetes 65, 358–370. doi: 10.2337/db15-0659
Gamu, D., Juracic, E. S., Fajardo, V. A., Rietze, B. A., Tran, K., Bombardier, E., et al. (2019). Phospholamban deficiency does not alter skeletal muscle SERCA pumping efficiency or predispose mice to diet-induced obesity. Am. J. Physiol. Endocrinol. Metab. 316, E432–E442. doi: 10.1152/ajpendo.00288.2018
Gancheva, S., Ouni, M., Jelenik, T., Koliaki, C., Szendroedi, J., Toledo, F. G. S., et al. (2019). Dynamic changes of muscle insulin sensitivity after metabolic surgery. Nat. Commun. 10:4179. doi: 10.1038/s41467-019-12081-0
Gherardi, G., Nogara, L., Ciciliot, S., Fadini, G. P., Blaauw, B., Braghetta, P., et al. (2019). Loss of mitochondrial calcium uniporter rewires skeletal muscle metabolism and substrate preference. Cell Death Differ. 26, 362–381. doi: 10.1038/s41418-018-0191-7
Hurst, J., James, R. S., Cox, V. M., Hill, C., and Tallis, J. (2019). Investigating a dose-response relationship between high-fat diet consumption and the contractile performance of isolated mouse soleus, EDL and diaphragm muscles. Eur. J. Appl. Physiol. 119, 213–226. doi: 10.1007/s00421-018-4017-6
Hwang, H., Bowen, B. P., Lefort, N., Flynn, C. R., De Filippis, E. A., Roberts, C., et al. (2010). Proteomics analysis of human skeletal muscle reveals novel abnormalities in obesity and type 2 diabetes. Diabetes 59, 33–42. doi: 10.2337/db09-0214
Jain, S. S., Paglialunga, S., Vigna, C., Ludzki, A., Herbst, E. A., Lally, J. S., et al. (2014). High-fat diet-induced mitochondrial biogenesis is regulated by mitochondrial-derived reactive oxygen species activation of CaMKII. Diabetes 63, 1907–1913. doi: 10.2337/db13-0816
Jaque-Fernandez, F., Beaulant, A., Berthier, C., Monteiro, L., Allard, B., Casas, M., et al. (2020). Preserved ca(2+) handling and excitation-contraction coupling in muscle fibres from diet-induced obese mice. Diabetologia 63, 2471–2481. doi: 10.1007/s00125-020-05256-8
Jheng, H. F., Tsai, P. J., Guo, S. M., Kuo, L. H., Chang, C. S., Su, I. J., et al. (2012). Mitochondrial fission contributes to mitochondrial dysfunction and insulin resistance in skeletal muscle. Mol. Cell. Biol. 32, 309–319. doi: 10.1128/MCB.05603-11
Kong, D., Song, G., Wang, C., Ma, H., Ren, L., Nie, Q., et al. (2013). Overexpression of mitofusin 2 improves translocation of glucose transporter 4 in skeletal muscle of highfat dietfed rats through AMPactivated protein kinase signaling. Mol. Med. Rep. 8, 205–210. doi: 10.3892/mmr.2013.1457
Kugler, B. A., Gundersen, A. E., Li, J., Deng, W., Eugene, N., Gona, P. N., et al. (2020). Roux-en-Y gastric bypass surgery restores insulin-mediated glucose partitioning and mitochondrial dynamics in primary myotubes from severely obese humans. Int. J. Obes. 44, 684–696. doi: 10.1038/s41366-019-0469-y
Leduc-Gaudet, J. P., Reynaud, O., Chabot, F., Mercier, J., Andrich, D. E., St-Pierre, D. H., et al. (2018). The impact of a short-term high-fat diet on mitochondrial respiration, reactive oxygen species production, and dynamics in oxidative and glycolytic skeletal muscles of young rats. Physiol. Rep. 6:e13548. doi: 10.14814/phy2.13548
Li, J., Kanasaki, M., Xu, L., Kitada, M., Nagao, K., Adachi, Y., et al. (2018). A ketogenic amino acid rich diet benefits mitochondrial homeostasis by altering the AKT/4EBP1 and autophagy signaling pathways in the gastrocnemius and soleus. Biochim. Biophys. Acta Gen. Subj. 1862, 1547–1555. doi: 10.1016/j.bbagen.2018.03.013
Liu, R., Jin, P., Yu, L., Wang, Y., Han, L., Shi, T., et al. (2014). Impaired mitochondrial dynamics and bioenergetics in diabetic skeletal muscle. PLoS One 9:e92810. doi: 10.1371/journal.pone.0116352
Maffiuletti, N. A., Jubeau, M., Munzinger, U., Bizzini, M., Agosti, F., De Col, A., et al. (2007). Differences in quadriceps muscle strength and fatigue between lean and obese subjects. Eur. J. Appl. Physiol. 101, 51–59. doi: 10.1007/s00421-007-0471-2
Mammucari, C., Gherardi, G., Zamparo, I., Raffaello, A., Boncompagni, S., Chemello, F., et al. (2015). The mitochondrial calcium uniporter controls skeletal muscle trophism in vivo. Cell Rep. 10, 1269–1279. doi: 10.1016/j.celrep.2015.01.056
Mammucari, C., Raffaello, A., Vecellio Reane, D., and Rizzuto, R. (2016). Molecular structure and pathophysiological roles of the mitochondrial calcium uniporter. Biochim. Biophys. Acta 1863, 2457–2464. doi: 10.1016/j.bbamcr.2016.03.006
Moller, A. B., Kampmann, U., Hedegaard, J., Thorsen, K., Nordentoft, I., Vendelbo, M. H., et al. (2017). Altered gene expression and repressed markers of autophagy in skeletal muscle of insulin resistant patients with type 2 diabetes. Sci. Rep. 7:43775. doi: 10.1038/srep43775
Montgomery, M. K., Brown, S. H. J., Mitchell, T. W., Coster, A. C. F., Cooney, G. J., and Turner, N. (2017). Association of muscle lipidomic profile with high-fat diet-induced insulin resistance across five mouse strains. Sci. Rep. 7:13914. doi: 10.1038/s41598-017-14214-1
Nikolova-Karakashian, M. N., and Reid, M. B. (2011). Sphingolipid metabolism, oxidant signaling, and contractile function of skeletal muscle. Antioxid. Redox Signal. 15, 2501–2517. doi: 10.1089/ars.2011.3940
O’connell, K., Gannon, J., Doran, P., and Ohlendieck, K. (2008). Reduced expression of sarcalumenin and related Ca2+ −regulatory proteins in aged rat skeletal muscle. Exp. Gerontol. 43, 958–961. doi: 10.1016/j.exger.2008.07.006
Paran, C. W., Verkerke, A. R., Heden, T. D., Park, S., Zou, K., Lawson, H. A., et al. (2015). Reduced efficiency of sarcolipin-dependent respiration in myocytes from humans with severe obesity. Obesity 23, 1440–1449. doi: 10.1002/oby.21123
Park, S. W., Goodpaster, B. H., Strotmeyer, E. S., De Rekeneire, N., Harris, T. B., Schwartz, A. V., et al. (2006). Decreased muscle strength and quality in older adults with type 2 diabetes: the health, aging, and body composition study. Diabetes 55, 1813–1818. doi: 10.2337/db05-1183
Park, S. W., Zhou, Y., Lee, J., Lee, J., and Ozcan, U. (2010). Sarco(endo)plasmic reticulum Ca2+-ATPase 2b is a major regulator of endoplasmic reticulum stress and glucose homeostasis in obesity. Proc. Natl. Acad. Sci. U. S. A. 107, 19320–19325. doi: 10.1073/pnas.1012044107
Rapizzi, E., Pinton, P., Szabadkai, G., Wieckowski, M. R., Vandecasteele, G., Baird, G., et al. (2002). Recombinant expression of the voltage-dependent anion channel enhances the transfer of Ca2+ microdomains to mitochondria. J. Cell Biol. 159, 613–624. doi: 10.1083/jcb.200205091
Rossi, A. E., Boncompagni, S., Wei, L., Protasi, F., and Dirksen, R. T. (2011). Differential impact of mitochondrial positioning on mitochondrial ca(2+) uptake and ca(2+) spark suppression in skeletal muscle. Am. J. Physiol. Cell Physiol. 301, C1128–C1139. doi: 10.1152/ajpcell.00194.2011
Sabbadini, R. A., Danieli-Betto, D., and Betto, R. (1999). The role of sphingolipids in the control of skeletal muscle function: a review. Ital. J. Neurol. Sci. 20, 423–430. doi: 10.1007/s100720050062
Santel, A., and Fuller, M. T. (2001). Control of mitochondrial morphology by a human mitofusin. J. Cell Sci. 114, 867–874. doi: 10.1242/jcs.114.5.867
Sharma, C., Smith, T., Li, S., Schroepfer, G. J. Jr., and Needleman, D. H. (2000). Inhibition of Ca2+ release channel (ryanodine receptor) activity by sphingolipid bases: mechanism of action. Chem. Phys. Lipids 104, 1–11. doi: 10.1016/S0009-3084(99)00106-1
Shkryl, V. M., and Shirokova, N. (2006). Transfer and tunneling of Ca2+ from sarcoplasmic reticulum to mitochondria in skeletal muscle. J. Biol. Chem. 281, 1547–1554. doi: 10.1074/jbc.M505024200
Suarez, J., Cividini, F., Scott, B. T., Lehmann, K., Diaz-Juarez, J., Diemer, T., et al. (2018). Restoring mitochondrial calcium uniporter expression in diabetic mouse heart improves mitochondrial calcium handling and cardiac function. J. Biol. Chem. 293, 8182–8195. doi: 10.1074/jbc.RA118.002066
Taddeo, E. P., Laker, R. C., Breen, D. S., Akhtar, Y. N., Kenwood, B. M., Liao, J. A., et al. (2014). Opening of the mitochondrial permeability transition pore links mitochondrial dysfunction to insulin resistance in skeletal muscle. Mol Metab 3, 124–134. doi: 10.1016/j.molmet.2013.11.003
Tong, X., Kono, T., Anderson-Baucum, E. K., Yamamoto, W., Gilon, P., Lebeche, D., et al. (2016). SERCA2 deficiency impairs pancreatic beta-cell function in response to diet-induced obesity. Diabetes 65, 3039–3052. doi: 10.2337/db16-0084
Townsend, L. K., Brunetta, H. S., and Mori, M. A. S. (2020). Mitochondria-associated ER membranes in glucose homeostasis and insulin resistance. Am. J. Physiol. Endocrinol. Metab. 319, E1053–E1060. doi: 10.1152/ajpendo.00271.2020
Tubbs, E., Chanon, S., Robert, M., Bendridi, N., Bidaux, G., Chauvin, M. A., et al. (2018). Disruption of mitochondria-associated endoplasmic reticulum membrane (MAM) integrity contributes to muscle insulin resistance in mice and humans. Diabetes 67, 636–650. doi: 10.2337/db17-0316
Verkerke, A. R. P., Ferrara, P. J., Lin, C. T., Johnson, J. M., Ryan, T. E., Maschek, J. A., et al. (2019). Phospholipid methylation regulates muscle metabolic rate through ca(2+) transport efficiency. Nat. Metab. 1, 876–885. doi: 10.1038/s42255-019-0111-2
Westerblad, H., Place, N., and Yamada, T. (2010). Mechanisms of skeletal muscle weakness. Adv. Exp. Med. Biol. 682, 279–296. doi: 10.1007/978-1-4419-6366-6_16
Wright, L. E., Vecellio Reane, D., Milan, G., Terrin, A., Di Bello, G., Belligoli, A., et al. (2017). Increased mitochondrial calcium uniporter in adipocytes underlies mitochondrial alterations associated with insulin resistance. Am. J. Physiol. Endocrinol. Metab. 313, E641–E650. doi: 10.1152/ajpendo.00143.2016
Yamada, T., Ivarsson, N., Hernandez, A., Fahlstrom, A., Cheng, A. J., Zhang, S. J., et al. (2012). Impaired mitochondrial respiration and decreased fatigue resistance followed by severe muscle weakness in skeletal muscle of mitochondrial DNA mutator mice. J. Physiol. 590, 6187–6197. doi: 10.1113/jphysiol.2012.240077
Zemel, M. B., Sowers, J. R., Shehin, S., Walsh, M. F., and Levy, J. (1990). Impaired calcium metabolism associated with hypertension in Zucker obese rats. Metabolism 39, 704–708. doi: 10.1016/0026-0495(90)90104-K
Keywords: sarcoplasmic reticulum, mitochondria, calcium, obesity, diabetes, skeletal muscle
Citation: Eshima H (2021) Influence of Obesity and Type 2 Diabetes on Calcium Handling by Skeletal Muscle: Spotlight on the Sarcoplasmic Reticulum and Mitochondria. Front. Physiol. 12:758316. doi: 10.3389/fphys.2021.758316
Edited by:
Bret H. Goodpaster, AdventHealth, United StatesReviewed by:
Vincenzo Sorrentino, University of Siena, ItalyD. George Stephenson, La Trobe University, Australia
Copyright © 2021 Eshima. This is an open-access article distributed under the terms of the Creative Commons Attribution License (CC BY). The use, distribution or reproduction in other forums is permitted, provided the original author(s) and the copyright owner(s) are credited and that the original publication in this journal is cited, in accordance with accepted academic practice. No use, distribution or reproduction is permitted which does not comply with these terms.
*Correspondence: Hiroaki Eshima, aGVzaGltYUBuaXUuYWMuanA=