- INSERM, U1059 Sainbiose, Université Jean Monnet, Mines Saint-Étienne, Université de Lyon, Saint-Étienne, France
Bone adaptation to spaceflight results in bone loss at weight bearing sites following the absence of the stimulus represented by ground force. The rodent hindlimb unloading model was designed to mimic the loss of mechanical loading experienced by astronauts in spaceflight to better understand the mechanisms causing this disuse-induced bone loss. The model has also been largely adopted to study disuse osteopenia and therefore to test drugs for its treatment. Loss of trabecular and cortical bone is observed in long bones of hindlimbs in tail-suspended rodents. Over the years, osteocytes have been shown to play a key role in sensing mechanical stress/stimulus via the ECM-integrin-cytoskeletal axis and to respond to it by regulating different cytokines such as SOST and RANKL. Colder experimental environments (~20–22°C) below thermoneutral temperatures (~28–32°C) exacerbate bone loss. Hence, it is important to consider the role of environmental temperatures on the experimental outcomes. We provide insights into the cellular and molecular pathways that have been shown to play a role in the hindlimb unloading and recommendations to minimize the effects of conditions that we refer to as confounding factors.
Introduction
The organism continuously renews its skeleton, by constantly resorbing and building bone tissue throughout its life. If bone resorption outpaces its formation, bone mass and strength are diminished. Bed rest, immobilization, paralysis, and spaceflight all share the common feature of bone loss, detected as reduced bone density at specific bone sites, potentially leading to osteopenia and osteoporosis (Takata and Yasui, 2001). The bone loss seen in such conditions is thought to be primarily caused by loss of mechanical loading (Lau and Guo, 2011). Microgravity causes bone loss especially, though not exclusively, at the weight-bearing sites (Oganov et al., 1992; Vico et al., 2000; Linossier et al., 2017). Similarly, in long term bed rest the loss of ground force reaction is accompanied by reduced muscle contractions and subsequent decrease in bone mineral density at distal femur, distal tibia and patella (Rittweger et al., 2009).
If bed rest volunteers have been used to understand disuse osteopenia in clinical studies, the need to better understand the organismal response to microgravity resulted in the development of the preclinical rodent hindlimb unloading (HLU) model in the 1970s (Morey, 1979) (Figure 1). The HLU model as such has been extensively detailed by Morey-Holton and Globus (Morey-Holton and Globus, 1998, 2002; Globus and Morey-Holton, 2016). Briefly, the model encompasses the unloading of the hindquarters of the rodent via tail suspension while the animal is left free to walk on its forelimbs. This position mimics the cephalic fluid shift and the decrease in mechanical loading on lower limbs experienced by astronauts during spaceflight (Morey-Holton and Globus, 1998), with the human equivalent being head-down tilt bed rest.
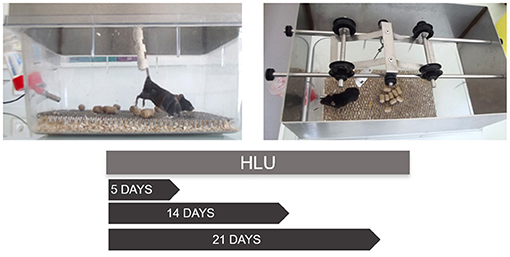
Figure 1. The suspension of the hindlimbs of a C57BL/6J mouse via tail suspension and the usual protocol durations. The suspension system consists of the padded rod to which the tail is attached using three tonoplast bandages wrapped at a distance from each other to not cover the tail completely. The rod is then hooked to a freely rotating swivel fixed to a pulley sliding on a roller axis to allow the mouse to freely rotate in 360° axis. The mouse is singly caged and allowed to move on its forelimbs with the support of the grid below. The angle of unloading is maintained at 30° from the ground. The periods of suspension may vary based on the experimental requirements. The different periods of suspension allow one to follow the kinetics of bone adaptation to unloading. At all-time points adaptation can be identified at different levels: molecular (gene expression profiling), cellular (histomorphometry) and tissular (X-ray tomography). Although tail traction with orthopedic tape is the preferred method of suspension, other methods including use of a body harness to stimulate partial weight bearing have been illustrated (Wagner et al., 2010).
The animal analog has now been adopted as a model to study muscle atrophy and disuse osteoporosis on ground conditions. Several alternatives to it exist such as tenotomy, neurectomy, botulin-induced paralysis, and unilateral limb casting (Komori, 2015). HLU has several benefits such as requiring minimal specialized equipment and not requiring a surgical intervention, unlike tenotomy or neurectomy. When compared to unilateral limb casting, HLU also additionally diminishes the mechanical load (Speacht et al., 2018). Unlike neurectomy, limb unloading itself is partially reversible with the recovery of bone mass being achieved to a certain extent when rodents are let free to use the four limbs again. HLU can be applied to rodents of the age, sex or genetic make-up of interest, allowing the modeling of the various complex clinical scenarios of bone loss observed in humans and the testing of anti-osteopenic drug therapies.
We want to bring to attention of the reader that although the HLU was developed in rats (reviewed in Morey-Holton et al., 2005) it has been well-adapted to mice. We have chosen primarily to focus on mice-based experiments rather than rats as mice offer certain advantages. Beyond ease of handling, their smaller weight allowing for smaller cages and for longer suspension periods. Also, increased availability of transgenic and mutant strains of mice makes it possible the study of specific molecular mechanisms (Ishijima et al., 2001; Iwaniec et al., 2005; Maurel et al., 2016; Yang et al., 2020). Moreover, rats achieve skeletal maturity toward the end of their life (Roach et al., 2003). Therefore, adult mice are preferred to adult but continuously growing rats for suspension models to study skeletal systems, in growing rats the loss of bone is attributed to a failure in increased bone formation and growth, where as in adult mice there is increased bone resorption and net bone loss, which is similar and more relevant to the case of humans in spaceflight (Globus and Morey-Holton, 2016).
This review sets to explore the cellular and molecular underpinnings of the response of bone resident cells to mechanical unloading in HLU. We examine the involved pathways and the role played by the different bone cells (with a particular focus on osteocytes). In addition, we highlight the effects of several key parameters of the experimental setup (e.g., environmental conditions) on the observed outcomes.
Bone Tissue Changes in HLU
HLU is reported to lead to a number of changes in bone structure both at the cortical and the trabecular levels. The majority of the studies adopting HLU have highlighted a more prominent loss in bone mass and architecture in the trabecular compartment secondary to increased osteoclastic resorption and decreased bone formation. Bone loss is reflected by a decrease in the relative trabecular bone volume (Bone Volume/Total volume) and it is connected to a disequilibrium in bone remodeling. This unbalance is apparent when measuring key static and dynamic bone parameters by histomorphometry, such as bone surface covered by osteoclasts (osteoclast surface over Bone surface, Oc.S/BS), osteoclast number per Bone surface (Oc.N/BS), mineralized trabecular surface per bone surface (MS/BS), mineral apposition rate (MAR), and bone formation rate (BFR) (Komori, 2015). HLU results in increased Oc.S/BS and Oc.N/BS and reduced MS/BS, MAR and BFR. Skeletal unloading also induces cortical thinning (Iwaniec et al., 2005; Morey-Holton et al., 2005; Speacht et al., 2018). Altered bone deposition and resorption patterns contribute to these effects with changes in both periosteum and endosteum formation rates that are not uniform along the bone length (Yang et al., 2020).
These results are consistent with those from ex vivo cultures of bone cells derived from tail suspended rats showing reduced osteoblasts' osteogenic potential with a decrease in the number of alkaline phosphatase (ALP)-positive colonies. Notably, an increase in tartrate resistant acid phosphatase (TRAP) positive cells was reported as well, therefore hinting at an increased osteoclastic activity (Maurel et al., 2016). Table 1 summarizes the bone trabecular and cortical changes seen in the hindlimbs of tail suspended mice.
Osteocyte Lacuno-Canalicular Space and Mechanosensors
Frost proposed that bone has an intrinsic regulatory mechanism, that he called the mechanostat. This regulatory “machinery” would respond to mechanical stimulation by adapting bone morphological, biochemical, and physical properties to serve its mechanical function in the most economical way. This model would anticipate an increased net bone resorption below a threshold of mechanical stimulation/use and net bone formation above a specific threshold (Frost, 1987). Frost's mechanostat theory provided a conceptual framework to rationalize bone adaptation to mechanical stimuli but it did not clarify which cells would sense the loading or how this information would be transmitted to bone forming and bone resorbing cells.
Eventually, osteoblasts, osteoclasts, and osteocytes, the three key cell types in bone, and their precursors have all been demonstrated to contribute, directly or indirectly, to bone homeostasis. In particular, osteocytes stand out as the key orchestrators of bone remodeling via regulation of osteoblastic bone mineralized matrix deposition and osteoclastic bone resorption (Bonewald, 2006, 2011).
Osteocytes reside in cavities called lacunae within the mineralized matrix, and send extensions called dendrites or osteocytic processes through the canaliculi (tunnels) crossing the bone matrix. The lacunae together with the canaliculi form the lacuno-canalicular space (LCS), that is filled with a fluid responsible for transporting solutes to and from the osteocyte (Figure 2). Dye injection into the tail vein of a mouse shows passage of the dye from circulation into the LCS within minutes (Knothe Tate et al., 2000; Price et al., 2011). Extravascular pressure drives the baseline flow of the canalicular fluid, but rapid alterations can occur in the flow due to changes in the mechanical loading [theoretically modeled by (Weinbaum et al., 1994)]. Later revisions of such model are detailed in a recent review by Hadida and Marchat (2020).
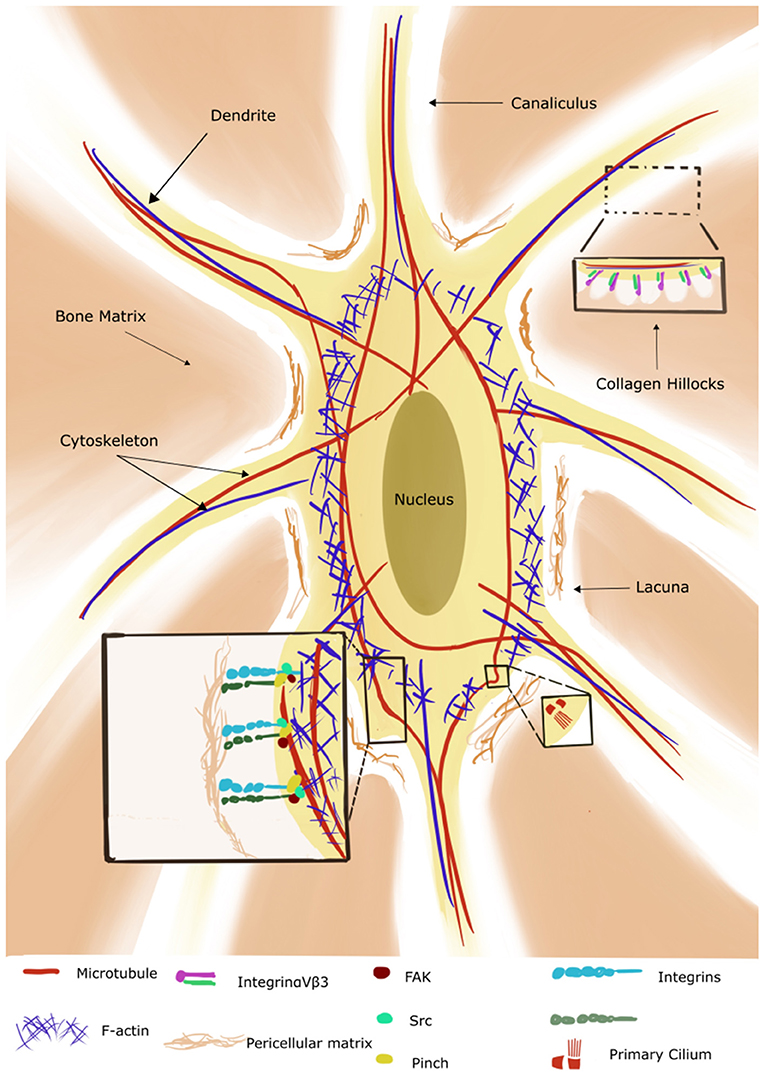
Figure 2. Illustration of an osteocyte with its lacuno-canalicular system (adapted from Qin et al., 2020). The cytoskeleton consists of microtubules (red) which extend to the primary cilium, actin (blue) and intermediate filaments (not shown in the figure). The bone osteoid forms collagen hillocks at the canaliculi. Integrins are present on the cell body and dendrites/osteocyte processes and interact with the pericellular matrix and cytoskeleton via the focal adhesion components (only three shown for simplicity). (Reproduced with permission: http://creativecommons.org/licenses/by/4.0/).
In this section we focus on the mechanosensors that have been explored in conjunction with HLU. The complex architecture of this space allows the transmission of the mechanical information from the scale of the fluid movement to the 3D matrix of the osteocyte cytoskeleton via transmembrane molecules like the protein Piezo1 (as reviewed in Qin et al., 2020). Piezo is a family of mechanosensitive cell membrane ion channels expressed in osteoblasts and osteocytes. It has been demonstrated that Piezo1 opens in response to mechanical stimuli and allows the entry of calcium ions into the cytoplasm (Coste et al., 2010). Mice with osteoblasts and osteocytes deficient in the Piezo channel show decreased trabecular bone volume, cortical thickness, and increased osteoclasts. However, these mice do not undergo bone loss when used in HLU experiments, highlighting the role of Piezo-1 as a key regulator in the response to mechanical loading (Sun et al., 2019; Wang et al., 2020). The ECM-integrin-cytoskeleton axis is crucial to mechanotransduction (reviewed in Yavropoulou and Yovos, 2016). The pericellular matrix of osteocytes forms hillock structures called “collagen hillocks” connecting the dendrites to the matrix (Figure 2). The matrix is in turn connected with the integrin-focal adhesion complexes in the cell body. Mechanical strain induces assembly of the focal adhesion molecules in association with integrins, and activation of the focal adhesion kinase (FAK) and the Src pathways ultimately resulting in the activation of the phosphoinositide 3-kinase (PI3K) and the mitogen activated protein kinase (MAPK) pathways (Marie et al., 2014). The MAPK pathways then specifically upregulate the expression levels of runt-related transcription factor 2 (RUNX2), Osterix, and activating transcription factor 4 (ATF4) promoting osteoblastogenesis (Franceschi and Ge, 2017). The three major cytoskeletal components in the osteocyte include actin filaments, which extend into the dendrites and are essential for osteocyte integrity, intermediate filaments like vimentin, whose levels are sensitive to mechanical stimulation, and microtubules, with the latter extending into the primary cilium (Figure 2) (Tanaka-Kamioka et al., 1998; Klein-Nulend et al., 2012). Primary cilia are “solitary” organelles projecting from the cell surface and mainly functioning as chemo- and mechanosensors. Kwon et al. explored their way of functioning in osteocytes and demonstrated that primary cilia bend under physiological levels of flow with a consequent decrease in cAMP levels mediated by adenylyl cyclase 6. They speculated that this decrease is transient and followed by the accumulation of cAMP which subsequently causes transcriptional changes in cyclooxygenase 2 (COX-2) and thus prostaglandin 2 (PGE2) expression (Kwon et al., 2010). PGE2 is a rapidly induced signaling molecule which is released in response to fluid flow shear stress and further acts via PKA, β-catenin pathways (Kamel et al., 2010; Kitase et al., 2010). However, it should be mentioned here that only a small percentage of bone cells (4%) have been found to carry primary cilia in vivo, indicating that the primary cilia probably function with other mechanosensory systems/organelles (Coughlin et al., 2015). The osteocytes communicate with each other and with the surrounding cells via gap junctions containing the protein connexin 43 (Cx43). Gap junctions enable quicker propagation of secondary messengers to adjacent cells, creating a functional syncytium throughout the bone (Buo and Stains, 2014). Gap junctions open in response to mechanical stress with resultant release of PGE2, and activation of the PI3K/AKT and cyclic adenosine monophosphate/protein kinase A pathways (cAMP/PKA) (Cherian et al., 2003; Xia et al., 2010). Cx43 knockout mice show an osteopenic phenotype with decrease in cortical BMD, thickness and increased porosity (Lloyd et al., 2012). Interestingly, the selective deletion of Cx43 in osteocytes also desensitized bone to 3 weeks of hindlimb unloading. In these animals, no increase in osteoclastic resorption was seen resulting in no decline in trabecular bone volume, thickness and density, indicating the inability of Cx43−/− osteocytes to efficiently detect, “communicate” or respond to unloading. Thus, deletion of Cx43 affects not only the physiological bone phenotype but also its response to loading (Lloyd et al., 2013).
Therefore, it can be derived that mechanical stimuli exert their stimulatory role by primarily modifying fluid flow which can induce matrix deformation, trigger membrane mechanoreceptors and induce cytoskeletal responses (Kitase et al., 2010).
Although the fluid flow theory provides a mechanical framework and allows a mechanistic analysis in terms of cell responses, it is not the only validated/critical mechanism for bone adaptation to mechanical loading and is not limited to the mechanosensors mentioned above. Also, comprehensive explanation of the biomolecular responses to perturbations in the fluid microenvironment in the HLU model remain elusive. To better understand the role played by mechanotransduction in bone health, it is important to further review the molecular pathways it relies on.
YAP/TAZ Pathway
YAP (Yes associated protein) and TAZ (Transcriptional factor with PDZ binding motif) are transcriptional factors that are increasingly being investigated for their role in cellular processes and have been found implicated in a number of physio-pathological conditions (e.g., arthritis, arthrosis, tumor metastases). Their activity can also be regulated by the rigidity and deformation of the ECM as it has been demonstrated in various cell types including the osteoblastic lineage (reviewed in Panciera et al., 2017). Their function is dependent on the integrity of actin cytoskeleton and requires Ras homologous protein (Rho) activity (Dupont et al., 2011). In vitro studies show ECM rigidity causes activation of the integrin-Rho pathways in cells which undergo cytoskeletal reorganization (e.g., increased polymerization, contractility, and stress fiber pooling) and consequent nuclear translocation of the YAP-TAZ complex, promoting differentiation of human mesenchymal stem cells (Kegelman et al., 2020).
Regarding their role in the regulation of osteoblastogenesis, data on the effects of YAP/TAZ signaling is contradictory and it appears that they have opposing effects at different stages of osteoblast differentiation. The concomitant deletion of YAP and TAZ in osteoprogenitor cells (Prx-1 Cre targeted mice lacking two copies of TAZ and one copy of YAP) resulted in increased osteoblast differentiation, whereas their deletion in mature osteoblasts/osteocytes in Dmp1-Cre mice reduced bone formation and also increased osteoclast number (Xiong et al., 2018). In vivo studies show that Piezo1 regulates YAP pathways in bone cells: mechanical stimulation transduced by Piezo1 leads to YAP nuclear accumulation and downstream of Ca2+ influx and Calcineurin activation, concomitant to Wnt/beta-Catenin pathway (Zhou et al., 2020). In Piezo1 conditional knockout mice, reduced nuclear localization of YAP with resultant osteoporosis. The same knockout mice, when suspended, resist bone loss (Wang et al., 2020). Atrophied muscle fibers from suspension do show a decrease in YAP protein which increases on reloading (Brooks et al., 2018). As such it would be interesting to look at the changes in YAP/TAZ pathways in bone during mechanical unloading. It has been shown that the pathway also interacts with Wnt canonical pathways (see below) (Azzolin et al., 2014).
In vitro studies show that YAP can promote or inhibit osteogenic differentiation in bone marrow mesenchymal stem cells (BMSCs) (Sen et al., 2015; Liu et al., 2019), whereas TAZ was shown to promote osteogenic differentiation in BMSC and MC3T3 cultures (Kim et al., 2014; Feng et al., 2015).
Overall, the YAP/TAZ pathway seems to be an important molecular pathway for the translation of mechanical stimuli into biochemical signals. However, further research in both in vitro and in vivo models is required to understand its dynamic role in response to unloading.
Wnt Pathway
Wingless-related integration site (Wnt) pathways are evolutionary conserved pathways comprising of a family of 19 glycoproteins which regulate several crucial aspects of cell fate and migration as well as organogenesis (reviewed in Komiya and Habas, 2008).
Wnt pathways have been known to positively contribute to bone mass via a number of mechanisms which include stem cell renewal, induction of osteoblastogenesis and prevention of both osteoblast and osteocyte death (Krishnan et al., 2006; Moorer and Riddle, 2018).
Briefly, in Wnt pathway OFF state, a destruction complex consisting of APC (Adenomatosis Polyposis Coli), axin, GSK3 (glycogen synthase kinase 3), and casein kinase 1 (CK1) is formed and it phosphorylates β-catenin therefore leading to its degradation in the proteasome. In its ON state, Wnt ligand binds to the frizzled receptors, LRP5/6 (low-density-lipoprotein-related protein 5/6), causing the inactivation of GSK3 via Disheveled (Dsh), a key component of Wnt-signaling pathways (Lerner and Ohlsson, 2015). This results in the accumulation and nuclear translocation of β-catenin. Here, β-catenin displaces the transcriptional co-repressors bound to TCF/LEF (t-cell factor/lymphoid enhanced factor) and recruits co-activators, regulating the expression of target genes such as cyclinD, c-Myc, peroxisome proliferator activated receptor (PPAR) and axin2, which participates in a negative feedback loop and limits the duration of Wnt signaling pathway (Jho et al., 2002; Krishnan et al., 2006).
Increased expression of Wnt target genes (i.e., Wnt10B, SFRP1, cyclin D1) was reported in response to mechanical loading both in vivo and in vitro (Robinson et al., 2006). On the contrary, decreased mRNA expression of LRP6 and β-catenin was seen in rats after 4 weeks of tail-suspension (Jia et al., 2019). Mice with an activating point mutation in the Wnt coreceptor LRP5 have high bone mass and they were found to be resistant to bone loss induced by hindlimb unloading (Niziolek et al., 2015). Also, artificial stabilization of β-catenin in osteocytes prevented the disuse-induced bone loss in unloaded mice (Bullock et al., 2019). These results imply an important role for Wnt/β-catenin in bone response to mechanical stimulation.
Secretory Signaling Proteins
Sclerostin is a protein encoded by the SOST gene and secreted mainly by mature osteocytes. SOST knockout mice exhibit a high bone mass phenotype with increased bone mineral density and bone strength due to increased bone formation. In humans, mutations in this gene are associated with rare genetic disorders associated with high bone mass, as sclerosteosis and van Buchem disease (Balemans et al., 2001; Li et al., 2008). Sclerostin is an antagonist of the Wnt downstream signaling pathway and it acts by binding to the LRP5/6 receptors (Li et al., 2005). Thus, it is a negative regulator of bone formation and it was shown to respond to mechanical loading. In vivo mRNA levels of sclerostin are reduced in the ulnar cortex of mice exposed to loading, whereas they are increased in the tibia of tail suspended mice at day 3 of suspension but subside to non-significant levels at day 7 (Robling et al., 2008). However, some experiments show a more complex pattern of SOST expression, with its levels varying with anatomical site. Unloaded hindlimbs (tibiae) of 3 month old rats showed a decrease in SOST expression in metaphyseal cortical bone, and upregulated SOST levels in diaphyseal bone (Macias et al., 2013).
In vitro experiments demonstrated that osteocyte cell lines, Ocy454 cells, when subjected to simulated microgravity, as achieved in the NASA rotating wall bioreactors, showed a significant increase in SOST expression when compared to the static controls (Spatz et al., 2015). Inversely, Ocy454 responded to fluid shear stress (FSS) (Lyons et al., 2017) by reducing SOST levels, de-repressing the Wnt signaling pathways. This occurs by a rapid lysosomal degradation of sclerostin within 5 min of exposure to an anabolic stimulus like FSS (Gould et al., 2021).
DKK1 is another antagonist of the Wnt pathway, acting by binding directly to LRP5/6. Its expression levels in bone were found to be reduced upon ulnar loading (Bafico et al., 2001), but no significant changes were seen on hindlimb unloading (Robling et al., 2008).
Both SOST and DKK1 antagonism using monoclonal antibodies has emerged as a therapeutic approach to treat osteoporosis (Ke et al., 2012). Romosozumab, a humanized monoclonal antibody against sclerostin, has been shown to reduce the risk of vertebral fractures in postmenopausal women and is already in use in United States and European Union (Paik and Scott, 2020).
RANK-RANKL signaling is necessary for the differentiation and activation of osteoclasts and subsequent bone resorption (reviewed in Ono et al., 2020). RANKL was believed to be secreted primarily by osteoblasts but the deletion of RANKL in late osteoblasts/osteocytes (Tnfsf11-floxed mice crossed with Dmp 1-Cre) showed that RANKL was mostly produced at this stage in adult mice (Xiong et al., 2015). In the cancellous bones of distal femurs of hindlimb unloaded rats elevated levels of pro-inflammatory cytokines (e.g., IL-1, TNF-α) were reported to lead to an increase in RANKL (Metzger et al., 2017). Several authors have described an increase in RANKL production by osteocytes neighboring osteocytes that were believed to undergo apoptosis as a response to loss of mechanical load or conversely excessive loading with resultant microfractures (reviewed in Xiong and O'Brien, 2012). Increase in bone resorption following osteocyte apoptosis has also been reported in cortical bone of OVX mice (Emerton et al., 2010).
Femurs of suspended mice showed an increase in osteocyte apoptosis with increase in RANKL and bone resorption (Cabahug-Zuckerman et al., 2016). However, Plotkin et al. (2015) demonstrated that bone resorption and bone loss occur even after blocking of osteocyte apoptosis using the inhibitor of apoptosis IG9402 (bisphosphate analog that maintains osteoblast and osteocyte viability). Other studies have also demonstrated that spaceflight and HLU induced bone loss occurs even in the absence of osteocyte apoptosis (Blaber et al., 2013; Farley et al., 2020). Moreover, the results from papers reporting apoptotic osteocytes should be treated with caution because they derive from immunohistochemical analyses run on thin (5 μm) sections, potentially leading to false positives when counting empty osteocyte lacunae (Jilka et al., 2013). False positives have also been reported for activated caspase 3 immunostaining and TUNEL staining with certain decalcification and pre-labeling techniques (Emans et al., 2005).
Also, counting empty lacunae does not give any information on the process causing cell death. It would therefore not be possible to distinguish between apoptosis or senescence. Further research is therefore warranted.
Bone Mediators of Energy Metabolism
Altered glucose metabolism, including glucose intolerance and insulin resistance, has been documented in astronauts in spaceflight (Stein et al., 1994; Hughson et al., 2016). Similarly, altered glucose metabolism was also seen in ground-based analogs such as head-down bed rest and dry immersion (Heer et al., 2014; De Abreu et al., 2017; Linossier et al., 2017). In hindlimb unloaded mice, fasting glucose levels are higher compared to control mice and insulin resistance is seen after 3 weeks from the beginning of the unloading (Wang et al., 2019). The cause for this metabolic dysfunction is not well-understood but maybe linked to altered levels/functions of osteokines and myokines in HLU.
The osteokine osteocalcin (Ocn) is expressed by mature osteoblasts and considered a marker of bone formation. Its expression is decreased during tail suspension and upregulated during mechanical loading (Han et al., 2018). Osteocalcin has been shown to promote the uptake of glucose in muscles at the onset of exercise and enhance the oxidation of glucose and fatty acids to be used by the muscle fibers (Mera et al., 2016). An Ocn−/− mouse line generated by the Karsenty's group shows impaired glucose metabolism, hinting at a role for osteocalcin in this metabolism (Lee et al., 2007). However, these results were questioned by two recent, independent studies using different Ocn−/− mouse lines (Diegel et al., 2020; Komori, 2020). The studies cast doubt on the metabolic roles of osteocalcin as the OCN−/− mice generated demonstrate that OCN is involved in bone quality and collagen maturity but has no effect on glucose metabolism or body weight (Diegel et al., 2020).
Lipocalin 2 (Lcn2) is expressed in adipose tissue, earning it the title of an adipokine, but it is also expressed by osteoblasts and plays a role in energy metabolism. The serum levels of Lcn2 in healthy volunteers in a prolonged bed rest study (15 days) were found to be elevated (Rucci et al., 2015). In mice, inactivation of lipocalin 2 in osteoblasts results in glucose intolerance and insulin resistance following an increase in food intake (Mosialou et al., 2017). Rucci et al. (2015) demonstrated an increase in lipocalin mRNA expression in bones (distal femur) of mice that had been suspended by their tail for 3 weeks, hypothesizing its role as a novel mechanoresponsive/mechanosensor gene. Transgenic mice overexpressing LCN2 in bone show a decrease in bone mass due to a negative effect on growth plate, decreased osteoblast differentiation and increased osteoclastic resorption (Costa et al., 2013). However, global deletion of lipocalin 2 demonstrated an osteopenic phenotype in mice, with lower trabecular bone volume (Capulli et al., 2018).
The myokine irisin is the cleavage product of the fibronectin type III domain-containing protein 5 (FNDC5) being secreted by muscles post exercise and it was identified for its role in the browning of white adipose tissues (Boström et al., 2012). Irisin was found to be significantly decreased in the soleus muscle in HLU mice and this decrease had a positive correlation to trabecular bone mineral density (BMD) (Kawao et al., 2018). Treatment of tail-suspended mice with r-irisin ameliorates disuse-induced osteoporosis, shown to be due to decrease in osteocyte apoptosis (Colaianni et al., 2017; Colucci et al., 2020). Irisin upregulated Opg (osteoprotegerin) in an in vitro 3D co-culture system of osteoblasts, osteoclasts and endothelial cells and prevented the downregulation of osteoblastic key transcription factors induced by microgravity (Colucci et al., 2020). However, Estell et al. (2020) showed that irisin can also directly act on osteoclasts to increase bone resorption. These results were obtained with lower doses and continuous administration of irisin when compared to experiments from Grano's lab (Colaianni et al., 2015), and they further support its role in bone remodeling probably as a counter regulatory hormone like PTH (parathyroid hormone). Thus, dosing and timing of irisin can be important determinants of its physiological impact on skeletal tissues.
It should be remembered that the HLU model is inherently stressful for mice and altered corticosteroids can mediate the metabolic changes (Pasieka and Rafacho, 2016). There have been contradictory findings on measures of stress in the HLU model in different laboratories (Morey-Holton and Globus, 2002). A few studies show an initial peak in cortisol levels which later reached basal levels (Steffen and Musacchia, 1987; Sugiyama et al., 2006). However, there are also studies reporting no changes in cortisol levels after unloading (Gaignier et al., 2014). The circadian rhythm of the glucocorticoid peak levels in blood may be a cause for this variability (Yang et al., 2017).
Confounding Factors And Recommendations
Although no model is universal, it is important to consider the effects of so-called confounding factors while setting the experimental design to guarantee reproducibility and repeatability.
Housing Temperature
Ambient temperature can affect net energy balance and lead to unpredictable outcomes in metabolic data, in isolated animals especially. An inverse relationship between food intake and housing temperatures has been reported (DeRuisseau et al., 2004). In mice, widely used standard vivarium temperature (20–22°C) conditions cause an increase in energy expenditure with a shift toward increased glucose utilization by Brown adipose Tissue (BAT) for non-shivering thermogenesis (David et al., 2013). Ideally, for mammals, the housing/environmental temperatures should be such that the energy expenditure is 1.6–1.7-fold the basal metabolic rate (Speakman and Keijer, 2012). Fischer et al. (2017) believe that the energy expenditure in mice at 21°C is almost three times higher than the basal metabolic rate and that, therefore, at this temperature they are under considerable metabolic stress (Fischer et al., 2017). C57BL/6J mice show a preference for higher temperatures especially during maintenance and inactive behaviors (Gaskill et al., 2009). Social housing at room temperature results in huddling of mice to conserve heat, or nesting in cases of social isolation, but these behaviors do not completely alleviate cold stress (Maher et al., 2015). Thus, housing temperatures are a critical parameter to consider while conducting these experiments. Cold stress is a major confounding factor and needs to be addressed and taken into consideration by the scientific community when drawing conclusive remarks from experiments carried out in these conditions. As a consequence, an ideal temperature to allow mice to alleviate cold-induced thermogenesis would be 28–29°C (Škop et al., 2020).
Studies show that housing mice individually at 22°C results in premature cancellous bone loss which is not seen in thermoneutral conditions (28–32°C) (Patel et al., 2012; Iwaniec et al., 2016; Martin et al., 2019). HLU experiments that were conducted at 28–32°C showed a decrease in cancellous bone volume in distal femur in suspended mice (Keune et al., 2017, 2019; Farley et al., 2020). Though they did not conduct the same experiments at standard temperature in parallel, their results show trabecular bone loss which is lesser than in comparable experiments at 22°C (Amblard et al., 2003; Lin et al., 2009).
Environmental temperature can affect bone remodeling, and exposure of growing mice to higher temperatures has shown to lengthen long bones (Racine et al., 2018). In a recent paper by Chevalier et al., warmth exposure (34°C) was reported to protect against ovariectomy-induced bone loss in mice. However, the same authors reported that the protective effect of warmth exposure was abolished when microbiota was depleted. Similarly, transplantation of warm-adapted microbiota (i.e., from male mice exposed to warm temperatures for 4 weeks into young male mice kept at room temperature) led to a higher cortical bone volume in the experimental mice (Chevalier et al., 2020). The increasing volume of research on the microbial-skeletal axis opens up new perspectives and likely possibility of new treatments (Behera et al., 2020).
Recommendations
Following are recommendations to consider when setting up an HLU experiment using mice. Experimental details are summarized in Figure 3.
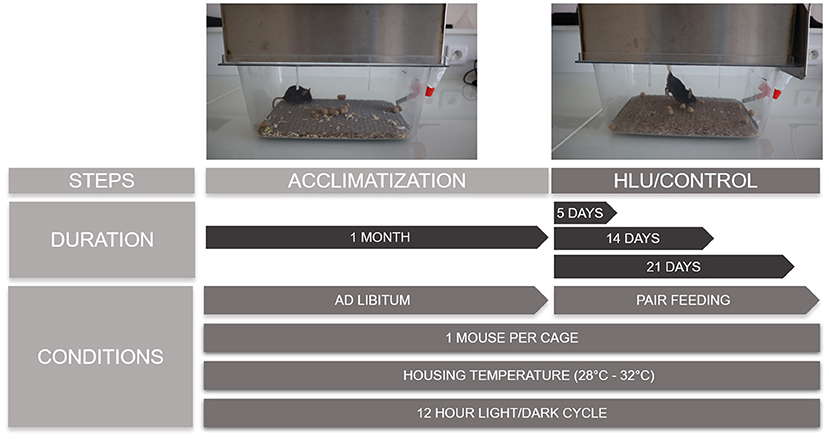
Figure 3. The scheme is reporting pictures of a C57BL/6J mouse during the acclimatization and suspension periods and the conditions and duration of each phase. Sufficiently long periods of acclimatization with ad libitum food and water have to be guaranteed, followed by the required periods of suspension where the control mice are pair fed with the suspended ones. Mice are singly housed without straw at thermoneutral temperatures of 28–32°C with 12-h light and dark cycles.
Mice Genotype
C57BL/6 (B6) is the most commonly used strain as it demonstrates a rapid bone loss to tail suspension (Amblard et al., 2003). It exhibits greater bone mehanosensitiviy and is widely used in bone loss studies (Kodama et al., 2000). B6 mice have lower BMD, lower osteoblastic acitivity but higher osteoclastic resorption when compared to C3H/HeJ mice (Linkhart et al., 1999).
Age
Mice reach skeletal maturity before 6 months of age and show a pattern of bone loss with aging similar to that in humans (Somerville et al., 2004). Mice total bone mass peaks when they are 4 months old, when it is considered to be representative of the skeletal maturity in human young adults (Beamer et al., 1996). Therefore, depending on the research question an appropriate age of the mice needs to be used. Since the growing skeleton in younger mice can have confounding effects on the results from tail suspension (Simske et al., 1990), a basal control group should be needed and sacrificed at the same time points of the experimental groups.
Feeding Conditions
During the acclimitization period, the mice are fed ad libitum. The control mice tend to eat more than the suspended mice when provided with food ad libitum, at least at the beginning of the suspension period. Since dietary restrictions can influence BMD and cause an expansion of the BMAT (Devlin, 2011; Cawthorn et al., 2016), it is necessary to eliminate a possible difference in food intake between the suspended and control groups. This is achieved by pair feeding the control mice to weight matched suspension group.
Control Conditions
Since we want identical conditions to the experimental group, the control mice are also housed singly without any straw or wood. This is not without consequences and can lead to stress in mice as they cannot huddle to warm up (Tahimic et al., 2019). A grid is placed over the litter to allow easy mobility for the suspended mice and the same should be done for controls as well. Without the grid, the control mice tend to nest in the litter which could create a potential difference of environment between the control and suspended mice. Control mice when attached to the suspension system without unloading show a tendency to chew off the tonoplast bandage/tail. It is therefore suggested to avoid the suspension system in the control groups. Each control mouse are fed the same amount of food as consumed by the weight paired suspended mouse on the previous day.
Acclimatization and Experimental Duration
Periods of suspension may vary based on whether early or later events are being looked at, but usually they vary between 5, 14, and 21 days and in some studies 28 days. It is important to standardize the experiment wherever possible with proper acclimatization of the mice, preferably singly housed at the recommended room temperature of 28°C for 4 weeks in their respective cages for 12 h light/dark cycle with food and water ad libitum to compensate for the comfounding effects of social isolation. Obernier and Baldwin showed that a sufficient duration of acclimitization is required to recouperate from the physiological disturbances caused by transport (Obernier and Baldwin, 2006). In addition a duration of 4 weeks will allow the mice to adapt to the social isolation, the HLU cages, housing temperature.
It is important to monitor cortisol levels after suspension to ensure that the influence of stress is taken into account.
Conclusion
The hindlimb unloading model developed in the 70s' to gain better understanding of the organismal response to microgravity, has then been adopted as a model to study other diseases such as disuse osteopenia.
The hindlimb unloading model has also contributed to identify potential drug candidates (e.g., Sclerostin antagonism using monoclonal antibodies) for the treatment of conditions such as osteopenia and osteoporosis. However, gaps still persist in our knowledge and understanding about the cellular and molecular pathways underpinning body response to microgravity. We caution on the importance of the experimental parameters that are adopted when running experiments based on murine hindlimb unloading. Overall, the murine hindlimb unloading still represents a key experimental model that willcertainly benefit the scientific community in the future to deepen our knowledge on pathophysiological processes that are of relevance for humans.
Author Contributions
PG, MS, LP, LV, and DI wrote the first draft and carried out subsequent revisions. All authors contributed to the article and approved the submitted version.
Funding
The authors acknowledge the financial contribution by the European Space Agency contract no. 4000128599/19/NL/PG, covering the MAP project BONUS - In vitro and in vivo pre-screening models and services for space and terrestrial interventions against bone and muscle fragility and CNES (Centre National d'Etudes Spatiales) contracts: no. 4800001065 OSTEOFLOW, no. 4800000966 ADIPOSTEO, and contract no. 4800000676 BION M1.
Conflict of Interest
The authors declare that the research was conducted in the absence of any commercial or financial relationships that could be construed as a potential conflict of interest.
Publisher's Note
All claims expressed in this article are solely those of the authors and do not necessarily represent those of their affiliated organizations, or those of the publisher, the editors and the reviewers. Any product that may be evaluated in this article, or claim that may be made by its manufacturer, is not guaranteed or endorsed by the publisher.
References
Amblard, D., Lafage-Proust, M.-H., Laib, A., Thomas, T., Rüegsegger, P., Alexandre, C., et al. (2003). Tail suspension induces bone loss in skeletally mature mice in the C57BL/6J strain but not in the C3H/HeJ strain. J. Bone Miner. Res. 18, 561–569. doi: 10.1359/jbmr.2003.18.3.561
Azzolin, L., Panciera, T., Soligo, S., Enzo, E., Bicciato, S., Dupont, S., et al. (2014). YAP/TAZ incorporation in the β-Catenin destruction complex orchestrates the Wnt response. Cell 158, 157–170. doi: 10.1016/j.cell.2014.06.013
Bafico, A., Liu, G., Yaniv, A., Gazit, A., and Aaronson, S. A. (2001). Novel mechanism of Wnt signalling inhibition mediated by Dickkopf-1 interaction with LRP6/Arrow. Nat. Cell Biol. 3, 683–686. doi: 10.1038/35083081
Balemans, W., Ebeling, M., Patel, N., Van Hul, E., Olson, P., Dioszegi, M., et al. (2001). Increased bone density in sclerosteosis is due to the deficiency of a novel secreted protein (SOST). Hum. Mol. Genet. 10, 537–543. doi: 10.1093/hmg/10.5.537
Beamer, W. G., Donahue, L. R., Rosen, C. J., and Baylink, D. J. (1996). Genetic variability in adult bone density among inbred strains of mice. Bone 18, 397–403. doi: 10.1016/8756-3282(96)00047-6
Behera, J., Ison, J., Tyagi, S. C., and Tyagi, N. (2020). The role of gut microbiota in bone homeostasis. Bone 135:115317. doi: 10.1016/j.bone.2020.115317
Blaber, E. A., Dvorochkin, N., Lee, C., Alwood, J. S., Yousuf, R., Pianetta, P., et al. (2013). Microgravity induces pelvic bone loss through osteoclastic activity, osteocytic osteolysis, and osteoblastic cell cycle inhibition by CDKN1a/p21. PLoS ONE 8:e61372. doi: 10.1371/journal.pone.0061372
Bonewald, L. F. (2006). Mechanosensation and transduction in osteocytes. BoneKEy Osteovision 3, 7–15. doi: 10.1138/20060233
Bonewald, L. F. (2011). The amazing osteocyte. J. Bone Miner. Res. 26, 229–238. doi: 10.1002/jbmr.320
Boström, P., Wu, J., Jedrychowski, M. P., Korde, A., Ye, L., Lo, J. C., et al. (2012). A PGC1-α-dependent myokine that drives brown-fat-like development of white fat and thermogenesis. Nature 481, 463–468. doi: 10.1038/nature10777
Brooks, M. J., Hajira, A., Mohamed, J. S., and Alway, S. E. (2018). Voluntary wheel running increases satellite cell abundance and improves recovery from disuse in gastrocnemius muscles from mice. J. Appl. Physiol. 124, 1616–1628. doi: 10.1152/japplphysiol.00451.2017
Bullock, W. A., Hoggatt, A. M., Horan, D. J., Lewis, K. J., Yokota, H., Hann, S., et al. (2019). Expression of a degradation-resistant β-catenin mutant in osteocytes protects the skeleton from mechanodeprivation-induced bone wasting. J. Bone Miner. Res. 34, 1964–1975. doi: 10.1002/jbmr.3812
Buo, A. M., and Stains, J. P. (2014). Gap junctional regulation of signal transduction in bone cells. FEBS Lett. 588, 1315–1321. doi: 10.1016/j.febslet.2014.01.025
Cabahug-Zuckerman, P., Frikha, D., Majeska, R., Tuthill, A., Yakar, S., Judex, S., et al. (2016). Osteocyte apoptosis caused by hindlimb unloading is required to trigger osteocyte RANKL production and subsequent resorption of cortical and trabecular bone in mice femurs. J. Bone Miner. Res. 31:2807. doi: 10.1002/jbmr.2807
Capulli, M., Ponzetti, M., Maurizi, A., Gemini-Piperni, S., Berger, T., Mak, T. W., et al. (2018). A complex role for lipocalin 2 in bone metabolism: global ablation in mice induces osteopenia caused by an altered energy metabolism. J. Bone Miner. Res. 33, 1141–1153. doi: 10.1002/jbmr.3406
Cawthorn, W. P., Scheller, E. L., Parlee, S. D., Pham, H. A., Learman, B. S., Redshaw, C. M. H., et al. (2016). Expansion of bone marrow adipose tissue during caloric restriction is associated with increased circulating glucocorticoids and not with hypoleptinemia. Endocrinology 157, 508–521. doi: 10.1210/en.2015-1477
Cherian, P. P., Cheng, B., Gu, S., Sprague, E., Bonewald, L. F., and Jiang, J. X. (2003). Effects of mechanical strain on the function of gap junctions in osteocytes are mediated through the prostaglandin EP2 receptor. J. Biol. Chem. 278, 43146–43156. doi: 10.1074/jbc.M302993200
Chevalier, C., Kieser, S., Çolakoglu, M., Hadadi N, Brun, J., Rigo, D., et al. (2020). Warmth prevents bone loss through the gut microbiota. Cell. Metab. 32, 575–590.e7. doi: 10.1016/j.cmet.2020.08.012
Colaianni, G., Cuscito, C., Mongelli, T., Pignataro, P., Buccoliero, C., Liu, P., et al. (2015). The myokine irisin increases cortical bone mass. Proc. Natl. Acad. Sci. U.S.A. 112, 12157–12162. doi: 10.1073/pnas.1516622112
Colaianni, G., Mongelli, T., Cuscito, C., Pignataro, P., Lippo, L., Spiro, G., et al. (2017). Irisin prevents and restores bone loss and muscle atrophy in hind-limb suspended mice. Sci. Rep. 7:2811. doi: 10.1038/s41598-017-02557-8
Colucci, S., Colaianni, G., Brunetti, G., Ferranti, F., Mascetti, G., Mori, G., et al. (2020). Irisin prevents microgravity-induced impairment of osteoblast differentiation in vitro during the space flight CRS-14 mission. FASEB J. 34, 10096–10106. doi: 10.1096/fj.202000216R
Costa, D., Lazzarini, E., Canciani, B., Giuliani, A., Span, Ò R, Marozzi, K., et al. (2013). Altered bone development and turnover in transgenic mice over-expressing Lipocalin-2 in bone. J. Cell Physiol. 228, 2210–2221. doi: 10.1002/jcp.24391
Coste, B., Mathur, J., Schmidt, M., Earley, T. J., Ranade, S., Petrus, M. J., et al. (2010). Piezo1 and Piezo2 are essential components of distinct mechanically activated cation channels. Science 330, 55–60. doi: 10.1126/science.1193270
Coughlin, T. R., Voisin, M., Schaffler, M. B., Niebur, G. L., and McNamara, L. M. (2015). Primary cilia exist in a small fraction of cells in trabecular bone and marrow. Calcif. Tissue Int. 96, 65–72. doi: 10.1007/s00223-014-9928-6
David, J. M., Chatziioannou, A. F., Taschereau, R., Wang, H., and Stout, D. B. (2013). The hidden cost of housing practices: using noninvasive imaging to quantify the metabolic demands of chronic cold stress of laboratory mice. Comp. Med. 63, 386–391.
De Abreu, S., Amirova, L., Murphy, R., Wallace, R., Twomey, L., Gauquelin-Koch, G., et al. (2017). Multi-system deconditioning in 3-day dry immersion without daily raise. Front. Physiol. 8:799. doi: 10.3389/fphys.2017.00799
DeRuisseau, L. R., Parsons, A. D., and Overton, J. M. (2004). Adaptive thermogenesis is intact in b6 and a/j mice studied at thermoneutrality. Metabolism 53, 1417–1423. doi: 10.1016/j.metabol.2004.06.007
Devlin, M. J. (2011). Why does starvation make bones fat? Am. J. Hum. Biol. 23, 577–585. doi: 10.1002/ajhb.21202
Diegel, C. R., Hann, S., Ayturk, U. M., Hu, J. C. W., Lim, K.-E., Droscha, C. J., et al. (2020). An osteocalcin-deficient mouse strain without endocrine abnormalities. PLoS Genet. 16:e1008361. doi: 10.1371/journal.pgen.1008361
Dupont, S., Morsut, L., Aragona, M., Enzo, E., Giulitti, S., Cordenonsi, M., et al. (2011). Role of YAP/TAZ in mechanotransduction. Nature 474, 179–183. doi: 10.1038/nature10137
Ellman, R., Grasso, D. J., van Vliet, M., Brooks, D. J., Spatz, J. M., Conlon, C., et al. (2014). Combined effects of botulinum toxin injection and hind limb unloading on bone and muscle. Calcif. Tissue Int. 94, 327–337. doi: 10.1007/s00223-013-9814-7
Emans, P. J., Bulstra, S. K., and Kuijer, R. (2005). The effects of different decalcification protocols on TUNEL and general cartilage staining. Biotech. Histochem. 80, 111–115. doi: 10.1080/10520290500159253
Emerton, K. B., Hu, B., Woo, A. A., Sinofsky, A., Hernandez, C., Majeska, R. J., et al. (2010). Osteocyte apoptosis and control of bone resorption following ovariectomy in mice. Bone 46, 577–583. doi: 10.1016/j.bone.2009.11.006
Estell, E. G., Le, P. T., Vegting, Y., Kim, H., Wrann, C., Bouxsein, M. L., et al. (2020). Irisin directly stimulates osteoclastogenesis and bone resorption in vitro and in vivo. eLife 9:e58172. doi: 10.7554/eLife.58172.sa2
Farley, A., Gnyubkin, V., Vanden-Bossche, A., Laroche, N., Neefs, M., Baatout, S., et al. (2020). Unloading-induced cortical bone loss is exacerbated by low-dose irradiation during a simulated deep space exploration mission. Calcif. Tissue Int. 107, 170–179. doi: 10.1007/s00223-020-00708-0
Feng, J., Sun, Q., Liu, L., and Xing, D. (2015). Photoactivation of TAZ via Akt/GSK3β signaling pathway promotes osteogenic differentiation. Int. J. Biochem. Cell Biol. 66, 59–68. doi: 10.1016/j.biocel.2015.07.002
Fischer, A. W., Cannon, B., and Nedergaard, J. (2017). Optimal housing temperatures for mice to mimic the thermal environment of humans: an experimental study. Mol. Metab. 7, 161–170. doi: 10.1016/j.molmet.2017.10.009
Franceschi, R. T., and Ge, C. (2017). Control of the osteoblast lineage by mitogen-activated protein kinase signaling. Curr. Mol. Biol. Rep. 3, 122–132. doi: 10.1007/s40610-017-0059-5
Frost, H. M. (1987). Bone “mass” and the “mechanostat”: a proposal. Anat. Rec. 219, 1–9. doi: 10.1002/ar.1092190104
Gaignier, F., Schenten, V., Bittencourt, M. D. C., Gauquelin-Koch, G., Frippiat, J.-P., and Legrand-Frossi, C. (2014). Three weeks of murine hindlimb unloading induces shifts from B to T and from Th to Tc splenic lymphocytes in absence of stress and differentially reduces cell-specific mitogenic responses. PLoS ONE 9:e92664. doi: 10.1371/journal.pone.0092664
Gaskill, B. N., Rohr, S. A., Pajor, E. A., Lucas, J. R., and Garner, J. P. (2009). Some like it hot: Mouse temperature preferences in laboratory housing. Appl. Anim. Behav. Sci. 116, 279–285. doi: 10.1016/j.applanim.2008.10.002
Globus, R. K., and Morey-Holton, E. (2016). Hindlimb unloading: rodent analog for microgravity. J. Appl. Physiol. 120, 1196–1206. doi: 10.1152/japplphysiol.00997.2015
Gould, N. R., Williams, K. M., Joca, H. C., Torre, O. M., Lyons, J. S., Leser, J. M., et al. (2021). Disparate bone anabolic cues activate bone formation by regulating the rapid lysosomal degradation of sclerostin protein. eLife 10:e64393. doi: 10.7554/eLife.64393
Hadida, M., and Marchat, D. (2020). Strategy for achieving standardized bone models. Biotechnol. Bioeng. 117, 251–271. doi: 10.1002/bit.27171
Han, B., Wei, S.-P., Zhang, X.-C., Li, H., Li, Y., Li, R.-X., et al. (2018). Effects of constrained dynamic loading, CKIP-1 gene knockout and combination stimulations on bone loss caused by mechanical unloading. Mol. Med. Rep. 18, 2506–2514. doi: 10.3892/mmr.2018.9222
Heer, M., Baecker, N., Wnendt, S., Fischer, A., Biolo, G., and Frings-Meuthen, P. (2014). How fast is recovery of impaired glucose tolerance after 21-day bed rest (NUC Study) in healthy adults? ScientificWorldJournal. 2014:803083. doi: 10.1155/2014/803083
Hughson, R. L., Robertson, A. D., Arbeille, P., Shoemaker, J. K., Rush, J. W. E., Fraser, K. S., et al. (2016). Increased postflight carotid artery stiffness and inflight insulin resistance resulting from 6-mo spaceflight in male and female astronauts. Am. J. Physiol. Heart Circ. Physiol. 310, H628–H638. doi: 10.1152/ajpheart.00802.2015
Ishijima, M., Rittling, S. R., Yamashita, T., Tsuji, K., Kurosawa, H., Nifuji, A., et al. (2001). Enhancement of osteoclastic bone resorption and suppression of osteoblastic bone formation in response to reduced mechanical stress do not occur in the absence of osteopontin. J. Exp. Med. 193, 399–404. doi: 10.1084/jem.193.3.399
Iwaniec, U. T., Philbrick, K. A., Wong, C. P., Gordon, J. L., Kahler-Quesada, A. M., Olson, D. A., et al. (2016). Room temperature housing results in premature cancellous bone loss in growing female mice: implications for the mouse as a preclinical model for age-related bone loss. Osteoporos Int. 27, 3091–3101. doi: 10.1007/s00198-016-3634-3
Iwaniec, U. T., Wronski, T. J., Amblard, D., Nishimura, Y., van der Meulen, M. C. H., Wade, C. E., et al. (2005). Effects of disrupted β1-integrin function on the skeletal response to short-term hindlimb unloading in mice. J. Appl. Physiol. 98, 690–696. doi: 10.1152/japplphysiol.00689.2004
Jho, E., Zhang, T., Domon, C., Joo, C.-K., Freund, J.-N., and Costantini, F. (2002). Wnt/beta-catenin/Tcf signaling induces the transcription of Axin2, a negative regulator of the signaling pathway. Mol. Cell. Biol. 22, 1172–1183. doi: 10.1128/MCB.22.4.1172-1183.2002
Jia, H., Tian, A., Zhang, X., Ma, X., Ma, J., Wang, J., et al. (2019). The effect of tail suspension and treadmill exercise on LRP6 expression, bone mass and biomechanical properties of hindlimb bones in SD rats. Am. J. Transl. Res. 11, 5847–5857.
Jilka, R. L., Noble, B., and Weinstein, R. S. (2013). Osteocyte apoptosis. Bone 54, 264–271. doi: 10.1016/j.bone.2012.11.038
Kamel, M. A., Picconi, J. L., Lara-Castillo, N., and Johnson, M. L. (2010). Activation of β-catenin signaling in MLO-Y4 osteocytic cells versus 2T3 osteoblastic cells by fluid flow shear stress and PGE2: implications for the study of mechanosensation in bone. Bone 47, 872–881. doi: 10.1016/j.bone.2010.08.007
Kawao, N., Moritake, A., Tatsumi, K., and Kaji, H. (2018). Roles of Irisin in the linkage from muscle to bone during mechanical unloading in mice. Calcif. Tissue Int. 103, 24–34. doi: 10.1007/s00223-018-0387-3
Ke, H. Z., Richards, W. G., Li, X., and Ominsky, M. S. (2012). Sclerostin and dickkopf-1 as therapeutic targets in bone diseases. Endocr. Rev. 33, 747–783. doi: 10.1210/er.2011-1060
Kegelman, C. D., Collins, J. M., Nijsure, M. P., Eastburn, E. A., and Boerckel, J. D. (2020). Gone caving: roles of the transcriptional regulators YAP and TAZ in skeletal development. Curr. Osteoporos. Rep. 18, 526–540. doi: 10.1007/s11914-020-00605-3
Keune, J. A., Branscum, A. J., Wong, C. P., Iwaniec, U. T., and Turner, R. T. (2019). Effect of leptin deficiency on the skeletal response to hindlimb unloading in adult male mice. Sci. Rep. 9:9336. doi: 10.1038/s41598-019-45587-0
Keune, J. A., Wong, C. P., Branscum, A. J., Iwaniec, U. T., and Turner, R. T. (2017). Bone marrow adipose tissue deficiency increases disuse-induced bone loss in male mice. Sci. Rep. 7:46325. doi: 10.1038/srep46325
Kim, K. M., Choi, Y. J., Hwang, J.-H., Kim, A. R., Cho, H. J., Hwang, E. S., et al. (2014). Shear stress induced by an interstitial level of slow flow increases the osteogenic differentiation of mesenchymal stem cells through TAZ activation. PLoS ONE 9:e92427. doi: 10.1371/journal.pone.0092427
Kitase, Y., Barragan, L., Qing, H., Kondoh, S., Jiang, J. X., Johnson, M. L., et al. (2010). Mechanical induction of PGE2 in osteocytes blocks glucocorticoid-induced apoptosis through both the β-catenin and PKA pathways. J. Bone Miner. Res. 25, 2657–2668. doi: 10.1002/jbmr.168
Klein-Nulend, J., Bacabac, R. G., and Bakker, A. D. (2012). Mechanical loading and how it affects bone cells: the role of the osteocyte cytoskeleton in maintaining our skeleton. Eur. Cell Mater. 24, 278–291. doi: 10.22203/eCM.v024a20
Knothe Tate, M. L., Steck, R., Forwood, M. R., and Niederer, P. (2000). In vivo demonstration of load-induced fluid flow in the rat tibia and its potential implications for processes associated with functional adaptation. J. Exp. Biol. 203, 2737–2745. doi: 10.1242/jeb.203.18.2737
Kodama, Y., Umemura, Y., Nagasawa, S., Beamer, W. G., Donahue, L. R., Rosen, C. R., et al. (2000). Exercise and mechanical loading increase periosteal bone formation and whole bone strength in C57BL/6J mice but not in C3H/Hej mice. Calcif. Tissue Int. 66, 298–306. doi: 10.1007/s002230010060
Komiya, Y., and Habas, R. (2008). Wnt signal transduction pathways. Organogenesis 4, 68–75. doi: 10.4161/org.4.2.5851
Komori, T. (2015). Animal models for osteoporosis. Eur. J. Pharmacol. 759, 287–294. doi: 10.1016/j.ejphar.2015.03.028
Komori, T. (2020). Functions of osteocalcin in bone, pancreas, testis, and muscle. Int. J. Mol. Sci. 21:7513. doi: 10.3390/ijms21207513
Krishnan, V., Bryant, H. U., and MacDougald, O. A. (2006). Regulation of bone mass by Wnt signaling. J. Clin. Invest. 116, 1202–1209. doi: 10.1172/JCI28551
Kwon, R. Y., Temiyasathit, S., Tummala, P., Quah, C. C., and Jacobs, C. R. (2010). Primary cilium-dependent mechanosensing is mediated by adenylyl cyclase 6 and cyclic AMP in bone cells. FASEB J. 24, 2859–2868. doi: 10.1096/fj.09-148007
Lau, R. Y., and Guo, X. (2011). A review on current osteoporosis research: with special focus on disuse bone loss. J. Osteoporos. 2011:293808. doi: 10.4061/2011/293808
Lee, N. K., Sowa, H., Hinoi, E., Ferron, M., Ahn, J. D., Confavreux, C., et al. (2007). Endocrine regulation of energy metabolism by the skeleton. Cell 130, 456–469. doi: 10.1016/j.cell.2007.05.047
Lerner, U. H., and Ohlsson, C. (2015). The WNT system: background and its role in bone. J. Intern. Med. 277, 630–649. doi: 10.1111/joim.12368
Li, X., Ominsky, M. S., Niu, Q.-T., Sun, N., Daugherty, B., D'Agostin, D., et al. (2008). Targeted deletion of the sclerostin gene in mice results in increased bone formation and bone strength. J. Bone Miner. Res. 23, 860–869. doi: 10.1359/jbmr.080216
Li, X., Zhang, Y., Kang, H., Liu, W., Liu, P., Zhang, J., et al. (2005). Sclerostin binds to LRP5/6 and antagonizes canonical Wnt signaling. J. Biol. Chem. 280, 19883–19887. doi: 10.1074/jbc.M413274200
Lin, C., Jiang, X., Dai, Z., Guo, X., Weng, T., Wang, J., et al. (2009). Sclerostin mediates bone response to mechanical unloading through antagonizing Wnt/beta-catenin signaling. J. Bone Miner. Res. 24, 1651–1661. doi: 10.1359/jbmr.090411
Linkhart, T. A., Linkhart, S. G., Kodama, Y., Farley, J. R., Dimai, H. P., Wright, K. R., et al. (1999). Osteoclast formation in bone marrow cultures from two inbred strains of mice with different bone densities. J. Bone Miner. Res. 14, 39–46. doi: 10.1359/jbmr.1999.14.1.39
Linossier, M.-T., Amirova, L. E., Thomas, M., Normand, M., Bareille, M.-P., Gauquelin-Koch, G., et al. (2017). Effects of short-term dry immersion on bone remodeling markers, insulin and adipokines. PLoS ONE 12:e0182970. doi: 10.1371/journal.pone.0182970
Liu, X., Hou, W., He, L., Han, F., Lu, M., Lu, X., et al. (2019). AMOT130/YAP pathway in topography-induced BMSC osteoblastic differentiation. Colloids Surf. B 182:110332. doi: 10.1016/j.colsurfb.2019.06.061
Lloyd, S. A., Lewis, G. S., Zhang, Y., Paul, E. M., and Donahue, H. J. (2012). Connexin 43 deficiency attenuates loss of trabecular bone and prevents suppression of cortical bone formation during unloading. J. Bone Miner. Res. 27, 2359–2372. doi: 10.1002/jbmr.1687
Lloyd, S. A., Loiselle, A. E., Zhang, Y., and Donahue, H. J. (2013). Connexin 43 deficiency desensitizes bone to the effects of mechanical unloading through modulation of both arms of bone remodeling. Bone 57, 76–83. doi: 10.1016/j.bone.2013.07.022
Lyons, J. S., Joca, H. C., Law, R. A., Williams, K. M., Kerr, J. P., Shi, G., et al. (2017). Microtubules tune mechanotransduction through NOX2 and TRPV4 to decrease sclerostin abundance in osteocytes. Sci. Signal 10:5748. doi: 10.1126/scisignal.aan5748
Macias, B. R., Aspenberg, P., and Agholme, F. (2013). Paradoxical Sost gene expression response to mechanical unloading in metaphyseal bone. Bone 53, 515–519. doi: 10.1016/j.bone.2013.01.018
Maher, R. L., Barbash, S. M., Lynch, D. V., and Swoap, S. J. (2015). Group housing and nest building only slightly ameliorate the cold stress of typical housing in female C57BL/6J mice. Am. J. Physiol. 308, R1070–R1079. doi: 10.1152/ajpregu.00407.2014
Marie, P. J., Haý, E, and Saidak, Z. (2014). Integrin and cadherin signaling in bone: role and potential therapeutic targets. Trends Endocrinol. Metab. 25, 567–575. doi: 10.1016/j.tem.2014.06.009
Martin, S. A., Philbrick, K. A., Wong, C. P., Olson, D. A., Branscum, A. J., Jump, D. B., et al. (2019). Thermoneutral housing attenuates premature cancellous bone loss in male C57BL/6J mice. Endocr. Connect. 8, 1455–1467. doi: 10.1530/EC-19-0359
Maurel, D. B., Duan, P., Farr, J., Cheng, A.-L., Johnson, M. L., and Bonewald, L. F. (2016). Beta-catenin haplo insufficient male mice do not lose bone in response to hindlimb unloading. PLoS ONE 11:e0158381. doi: 10.1371/journal.pone.0158381
Mera, P., Laue, K., Ferron, M., Confavreux, C., Wei, J., Galán-Díez, M., et al. (2016). Osteocalcin signaling in myofibers is necessary and sufficient for optimum adaptation to exercise. Cell Metab. 23, 1078–1092. doi: 10.1016/j.cmet.2016.05.004
Metzger, C. E., Brezicha, J. E., Elizondo, J. P., Narayanan, S. A., Hogan, H. A., and Bloomfield, S. A. (2017). Differential responses of mechanosensitive osteocyte proteins in fore- and hindlimbs of hindlimb-unloaded rats. Bone 105, 26–34. doi: 10.1016/j.bone.2017.08.002
Moorer, M. C., and Riddle, R. C. (2018). Regulation of osteoblast metabolism by Wnt signaling. Endocrinol. Metab. 33, 318–330. doi: 10.3803/EnM.2018.33.3.318
Morey, E. R. (1979). Spaceflight and bone turnover: correlation with a new rat model of weightlessness. BioScience 29, 168–172. doi: 10.2307/1307797
Morey-Holton, E., Globus, R. K., Kaplansky, A., and Durnova, G. (2005). The hindlimb unloading rat model: literature overview, technique update and comparison with space flight data. Adv. Space Biol. Med. 10, 7–40. doi: 10.1016/S1569-2574(05)10002-1
Morey-Holton, E. R., and Globus, R. K. (1998). Hindlimb unloading of growing rats: a model for predicting skeletal changes during space flight. Bone 22, 83S−88S. doi: 10.1016/S8756-3282(98)00019-2
Morey-Holton, E. R., and Globus, R. K. (2002). Hindlimb unloading rodent model: technical aspects. J. Appl. Physiol. 92, 1367–1377. doi: 10.1152/japplphysiol.00969.2001
Mosialou, I., Shikhel, S., Liu, J.-M., Maurizi, A., Luo, N., He, Z., et al. (2017). MC4R-dependent suppression of appetite by bone-derived lipocalin 2. Nature. 543, 385–90. doi: 10.1038/nature21697
Niziolek, P. J., Bullock, W., Warman, M. L., and Robling, A. G. (2015). Missense mutations in LRP5 associated with high bone mass protect the mouse skeleton from disuse- and ovariectomy-induced osteopenia. PLoS ONE 10:e0140775. doi: 10.1371/journal.pone.0140775
Obernier, J. A., and Baldwin, R. L. (2006). Establishing an appropriate period of acclimatization following transportation of laboratory animals. ILAR J. 47, 364–369. doi: 10.1093/ilar.47.4.364
Oganov, V. S., Grigor'ev, A. I., Voronin, L. I., Rakhmanov, A. S., Bakulin, A. V., Schneider, V. S., et al. (1992). Bone mineral density in cosmonauts after flights lasting 4.5-6 months on the Mir orbital station. Aviakosmicheskaia Ekol Meditsina Aerosp Environ Med. 26, 20–4.
Ono, T., Hayashi, M., Sasaki, F., and Nakashima, T. (2020). RANKL biology: bone metabolism, the immune system, and beyond. Inflamm. Regen. 40:2. doi: 10.1186/s41232-019-0111-3
Paik, J., and Scott, L. J. (2020). Romosozumab: a review in postmenopausal osteoporosis. Drugs Aging 37, 845–855. doi: 10.1007/s40266-020-00793-8
Panciera, T., Azzolin, L., Cordenonsi, M., and Piccolo, S. (2017). Mechanobiology of YAP and TAZ in physiology and disease. Nat. Rev. Mol. Cell Biol. 18, 758–770. doi: 10.1038/nrm.2017.87
Pasieka, A. M., and Rafacho, A. (2016). Impact of glucocorticoid excess on glucose tolerance: clinical and preclinical evidence. Metabolites 6:24. doi: 10.3390/metabo6030024
Patel, J. J., Utting, J. C., Key, M. L., Orriss, I. R., Taylor, S. E. B., Whatling, P., et al. (2012). Hypothermia inhibits osteoblast differentiation and bone formation but stimulates osteoclastogenesis. Exp. Cell Res. 318, 2237–2244. doi: 10.1016/j.yexcr.2012.06.021
Plotkin, L. I., Gortazar, A. R., Davis, H. M., Condon, K. W., Gabilondo, H., Maycas, M., et al. (2015). Inhibition of osteocyte apoptosis prevents the increase in osteocytic receptor activator of nuclear factor κB ligand (RANKL) but does not stop bone resorption or the loss of bone induced by unloading. J. Biol. Chem. 290, 18934–18942. doi: 10.1074/jbc.M115.642090
Price, C., Zhou, X., Li, W., and Wang, L. (2011). Real-time measurement of solute transport within the lacunar-canalicular system of mechanically loaded bone: Direct evidence for load-induced fluid flow. J. Bone Miner. Res. 26, 277–285. doi: 10.1002/jbmr.211
Qin, L., Liu, W., Cao, H., and Xiao, G. (2020). Molecular mechanosensors in osteocytes. Bone Res. 8, 1–24. doi: 10.1038/s41413-020-0099-y
Racine, H. L., Meadows, C. A., Ion, G., and Serrat, M. A. (2018). Heat-induced limb length asymmetry has functional impact on weight bearing in mouse hindlimbs. Front. Endocrinol. 9 :289. doi: 10.3389/fendo.2018.00289
Rittweger, J., Simunic, B., Bilancio, G., De Santo, N. G., Cirillo, M., Biolo, G., et al. (2009). Bone loss in the lower leg during 35 days of bed rest is predominantly from the cortical compartment. Bone 44, 612–618. doi: 10.1016/j.bone.2009.01.001
Roach, H. I., Mehta, G., Oreffo, R. O. C., Clarke, N. M. P., and Cooper, C. (2003). Temporal analysis of rat growth plates: cessation of growth with age despite presence of a physis. J. Histochem. Cytochem. 51, 373–383. doi: 10.1177/002215540305100312
Robinson, J. A., Chatterjee-Kishore, M., Yaworsky, P. J., Cullen, D. M., Zhao, W., Li, C., et al. (2006). Wnt/beta-catenin signaling is a normal physiological response to mechanical loading in bone. J. Biol. Chem. 281, 31720–31728. doi: 10.1074/jbc.M602308200
Robling, A. G., Niziolek, P. J., Baldridge, L. A., Condon, K. W., Allen, M. R., Alam, I., et al. (2008). Mechanical stimulation of bone in vivo reduces osteocyte expression of Sost/sclerostin. J. Biol. Chem. 283, 5866–5875. doi: 10.1074/jbc.M705092200
Rucci, N., Capulli, M., Piperni, S. G., Cappariello, A., Lau, P., Frings-Meuthen, P., et al. (2015). Lipocalin 2: a new mechanoresponding gene regulating bone homeostasis. J. Bone Miner. Res. 30, 357–368. doi: 10.1002/jbmr.2341
Saxena, R., Pan, G., Dohm, E. D., and McDonald, J. M. (2011). Modeled microgravity and hindlimb unloading sensitize osteoclast precursors to RANKL-mediated osteoclastogenesis. J. Bone Miner. Metab. 29, 111–122. doi: 10.1007/s00774-010-0201-4
Sen, B., Xie, Z., Uzer, G., Thompson, W. R., Styner, M., Wu, X., et al. (2015). Intranuclear actin regulates osteogenesis. Stem Cells 33, 3065–3076. doi: 10.1002/stem.2090
Shahnazari, M., Kurimoto, P., Boudignon, B. M., Orwoll, B. E., Bikle, D. D., and Halloran, B. P. (2012). Simulated spaceflight produces a rapid and sustained loss of osteoprogenitors and an acute but transitory rise of osteoclast precursors in two genetic strains of mice. Am. J. Physiol. 303, E1354–E1362. doi: 10.1152/ajpendo.00330.2012
Simske, S. J., Guerra, K. M., Greenberg, A. R., and Luttges, M. W. (1992). The physical and mechanical effects of suspension-induced osteopenia on mouse long bones. J. Biomech. 25, 489–499. doi: 10.1016/0021-9290(92)90089-J
Simske, S. J., Luttges, M. W., and Wachtel, H. (1990). Age dependent development of osteopenia in the long bones of tail-suspended mice. Biomed. Sci. Instrum. 26, 87–94.
Škop, V., Guo J, Liu, N., Xiao, C., Hall, K. D., Gavrilova, O., et al. (2020). Mouse thermoregulation: introducing the concept of the thermoneutral point. Cell Rep. 31:107501. doi: 10.1016/j.celrep.2020.03.065
Somerville, J. M., Aspden, R. M., Armour, K. E., Armour, K. J., and Reid, D. M. (2004). Growth of C57Bl/6 mice and the material and mechanical properties of cortical bone from the tibia. Calcif. Tissue Int. 74, 469–475. doi: 10.1007/s00223-003-0101-x
Spatz, J. M., Ellman, R., Cloutier, A. M., Louis, L., van Vliet, M., Suva, L. J., et al. (2013). Sclerostin antibody inhibits skeletal deterioration due to reduced mechanical loading. J. Bone Miner. Res. 28, 865–874. doi: 10.1002/jbmr.1807
Spatz, J. M., Wein, M. N., Gooi, J. H., Qu, Y., Garr, J. L., Liu, S., et al. (2015). The Wnt Inhibitor Sclerostin Is Up-regulated by Mechanical Unloading in Osteocytes in Vitro. J Biol Chem 290, 16744–16758. doi: 10.1074/jbc.M114.628313
Speacht, T. L., Krause, A. R., Steiner, J. L., Lang, C. H., and Donahue, H. J. (2018). Combination of hindlimb suspension and immobilization by casting exaggerates sarcopenia by stimulating autophagy but does not worsen osteopenia. Bone 110, 29–37. doi: 10.1016/j.bone.2018.01.026
Speakman, J. R., and Keijer, J. (2012). Not so hot: optimal housing temperatures for mice to mimic the thermal environment of humans. Mol. Metab. 2, 5–9. doi: 10.1016/j.molmet.2012.10.002
Steczina, S., Tahimic, C. G. T., Pendleton, M., M'Saad, O., Lowe, M., Alwood, J. S., et al. (2020). Dietary countermeasure mitigates simulated spaceflight-induced osteopenia in mice. Sci. Rep. 10:6484. doi: 10.1038/s41598-020-63404-x
Steffen, J. M., and Musacchia, X. J. (1987). Disuse atrophy, plasma corticosterone, and muscle glucocorticoid receptor levels. Aviat. Space Environ. Med. 58, 996–1000.
Stein, T. P., Schulter, M. D., and Boden, G. (1994). Development of insulin resistance by astronauts during spaceflight. Aviat. Space Environ. Med. 65, 1091–1096.
Sugiyama, F., Wu, J., Fujioka, M., Ezaki, J., Takeda, K., Miyaura, C., et al. (2006). Soybean isoflavones preserve bone mass in hindlimb-unloaded mice. J. Bone Miner. Metab. 24, 439–446. doi: 10.1007/s00774-006-0711-2
Sun, W., Chi, S., Li, Y., Ling, S., Tan, Y., Xu, Y., et al. (2019). The mechanosensitive Piezo1 channel is required for bone formation. eLife 8:e47454. doi: 10.7554/eLife.47454
Tahimic, C. G. T., Paul, A. M., Schreurs, A.-S., Torres, S. M., Rubinstein, L., Steczina, S., et al. (2019). Influence of social isolation during prolonged simulated weightlessness by hindlimb unloading. Front. Physiol. 10:1147. doi: 10.3389/fphys.2019.01147
Tanaka-Kamioka, K., Kamioka, H., Ris, H., and Lim, S.-S. (1998). Osteocyte shape is dependent on actin filaments and osteocyte processes are unique actin-rich projections. J. Bone Miner. Res. 13, 1555–1568. doi: 10.1359/jbmr.1998.13.10.1555
Tousen, Y., Ichimaru, R., Kondo, T., Inada, M., Miyaura, C., and Ishimi, Y. (2020). The combination of soy isoflavones and resveratrol preserve bone mineral density in hindlimb-unloaded mice. Nutrients 12 :2043. doi: 10.3390/nu12072043
Vico, L., Collet, P., Guignandon, A., Lafage-Proust, M.-H., Thomas, T., Rehailia, M., et al. (2000). Effects of long-term microgravity exposure on cancellous and cortical weight-bearing bones of cosmonauts. Lancet 355, 1607–1611. doi: 10.1016/S0140-6736(00)02217-0
Wagner, E. B., Granzella, N. P., Saito, H., Newman, D. J., Young, L. R., and Bouxsein, M. L. (2010). Partial weight suspension: a novel murine model for investigating adaptation to reduced musculoskeletal loading. J. Appl. Physiol. 109, 350–357. doi: 10.1152/japplphysiol.00014.2009
Wang, L., You, X., Lotinun, S., Zhang, L., Wu, N., and Zou, W. (2020). Mechanical sensing protein PIEZO1 regulates bone homeostasis via osteoblast-osteoclast crosstalk. Nat. Commun. 11:282. doi: 10.1038/s41467-019-14146-6
Wang, Y., Zhao, W., Shi, J., Wang, J., Hao, J., Pang, X., et al. (2019). Intestinal microbiota contributes to altered glucose metabolism in simulated microgravity mouse model. FASEB J. 33, 10140–10151. doi: 10.1096/fj.201900238RR
Weinbaum, S., Cowin, S. C., and Zeng, Y. (1994). A model for the excitation of osteocytes by mechanical loading-induced bone fluid shear stresses. J. Biomech. 27, 339–360. doi: 10.1016/0021-9290(94)90010-8
Xia, X., Batra, N., Shi, Q., Bonewald, L. F., Sprague, E., and Jiang, J. X. (2010). Prostaglandin promotion of osteocyte gap junction function through transcriptional regulation of connexin 43 by glycogen synthase kinase 3/beta-catenin signaling. Mol. Cell Biol. 30, 206–219. doi: 10.1128/MCB.01844-08
Xiong, J., Almeida, M., and O'Brien, C. A. (2018). The YAP/TAZ transcriptional co-activators have opposing effects at different stages of osteoblast differentiation. Bone 112, 1–9. doi: 10.1016/j.bone.2018.04.001
Xiong, J., and O'Brien, C. A. (2012). Osteocyte RANKL: New insights into the control of bone remodeling. J. Bone Miner. Res. 27, 499–505. doi: 10.1002/jbmr.1547
Xiong, J., Piemontese, M., Onal, M., Campbell, J., Goellner, J. J., Dusevich, V., et al. (2015). Osteocytes, not osteoblasts or lining cells, are the main source of the RANKL required for osteoclast formation in remodeling bone. PLoS ONE 10:e0138189. doi: 10.1371/journal.pone.0138189
Yang, J., Li, J., Cui, X., Li, W., Xue, Y., Shang, P., et al. (2020). Blocking glucocorticoid signaling in osteoblasts and osteocytes prevents mechanical unloading-induced cortical bone loss. Bone 130:115108. doi: 10.1016/j.bone.2019.115108
Yang, J., Yang, Z., Li, W., Xue, Y., Xu, H., Li, J., et al. (2017). Glucocorticoid: A potential role in microgravity-induced bone loss. Acta Astronaut. 140, 206–212. doi: 10.1016/j.actaastro.2017.08.007
Yavropoulou, M. P., and Yovos, J. G. (2016). The molecular basis of bone mechanotransduction. J. Musculoskelet. Neuronal. Interact. 16, 221–236. doi: 10.1172/JCI140214
Keywords: hindlimb unloading, osteocyte, mechanotransduction, bone loss, disuse osteopenia
Citation: Garg P, Strigini M, Peurière L, Vico L and Iandolo D (2021) The Skeletal Cellular and Molecular Underpinning of the Murine Hindlimb Unloading Model. Front. Physiol. 12:749464. doi: 10.3389/fphys.2021.749464
Received: 29 July 2021; Accepted: 23 September 2021;
Published: 19 October 2021.
Edited by:
Rhonda Prisby, University of Texas at Arlington, United StatesReviewed by:
Meghan E. McGee-Lawrence, Medical College of Georgia, United StatesGiacomina Brunetti, University of Bari Aldo Moro, Italy
Copyright © 2021 Garg, Strigini, Peurière, Vico and Iandolo. This is an open-access article distributed under the terms of the Creative Commons Attribution License (CC BY). The use, distribution or reproduction in other forums is permitted, provided the original author(s) and the copyright owner(s) are credited and that the original publication in this journal is cited, in accordance with accepted academic practice. No use, distribution or reproduction is permitted which does not comply with these terms.
*Correspondence: Laurence Vico, dmljb0B1bml2LXN0LWV0aWVubmUuZnI=