- 1Exercise and Sport Sciences Laboratory, Department of Sport, Exercise, Recreation, and Kinesiology, East Tennessee State University, Johnson City, TN, United States
- 2Department of Rehabilitative Sciences, East Tennessee State University, Johnson City, TN, United States
- 3Integrative Muscle Physiology Laboratory, Department of Health and Exercise Science, Appalachian State University, Boone, NC, United States
- 4Department of Biology, Appalachian State University, Boone, NC, United States
- 5Department of Physiology, Faculty of Medicine and Nursing, University of the Basque Country, Leioa, Spain
- 6Exercise Science Laboratory, School of Kinesiology, Faculty of Medicine, Universidad Finis Terrae, Santiago, Chile
Before major athletic events, a taper is often prescribed to facilitate recovery and enhance performance. However, it is unknown which taper model is most effective for peaking maximal strength and positively augmenting skeletal muscle. Thus, the purpose of this study was to compare performance outcomes and skeletal muscle adaptations following a step vs. an exponential taper in strength athletes. Sixteen powerlifters (24.0 ± 4.0 years, 174.4 ± 8.2 cm, 89.8 ± 21.4 kg) participated in a 6-week training program aimed at peaking maximal strength on back squat [initial 1-repetition-maximum (1RM): 174.7 ± 33.4 kg], bench press (118.5 ± 29.9 kg), and deadlift (189.9 ± 41.2 kg). Powerlifters were matched based on relative maximal strength, and randomly assigned to either (a) 1-week overreach and 1-week step taper or (b) 1-week overreach and 3-week exponential taper. Athletes were tested pre- and post-training on measures of body composition, jumping performance, isometric squat, and 1RM. Whole muscle size was assessed at the proximal, middle, and distal vastus lateralis using ultrasonography and microbiopsies at the middle vastus lateralis site. Muscle samples (n = 15) were analyzed for fiber size, fiber type [myosin-heavy chain (MHC)-I, -IIA, -IIX, hybrid-I/IIA] using whole muscle immunohistochemistry and single fiber dot blots, gene expression, and microRNA abundance. There were significant main time effects for 1RM squat (p < 0.001), bench press (p < 0.001), and deadlift, (p = 0.024), powerlifting total (p < 0.001), Wilks Score (p < 0.001), squat jump peak-power scaled to body mass (p = 0.001), body mass (p = 0.005), fat mass (p = 0.002), and fat mass index (p = 0.002). There were significant main time effects for medial whole muscle cross-sectional area (mCSA) (p = 0.006) and averaged sites (p < 0.001). There was also a significant interaction for MHC-IIA fiber cross-sectional area (fCSA) (p = 0.014) with post hoc comparisons revealing increases following the step-taper only (p = 0.002). There were significant main time effects for single-fiber MHC-I% (p = 0.015) and MHC-IIA% (p = 0.033), as well as for MyoD (p = 0.002), MyoG (p = 0.037), and miR-499a (p = 0.033). Overall, increases in whole mCSA, fCSA, MHC-IIA fCSA, and MHC transitions appeared to favor the step taper group. An overreach followed by a step taper appears to produce a myocellular environment that enhances skeletal muscle adaptations, whereas an exponential taper may favor neuromuscular performance.
Introduction
Before major competitions, a taper is often prescribed as the final stage of training aimed at decreasing physiological and psychological fatigue to achieve optimal preparedness (Mujika and Padilla, 2003; Travis et al., 2020c). A taper is typically constructed via reducing the amount of training, primarily through decreasing overall training volume-load and manipulating intensity over 1–4 weeks (Mujika and Padilla, 2003; Pritchard et al., 2015; Travis et al., 2020c). The manner in which work is reduced can be accomplished using different taper models including step, linear, and exponential with fast- or slow-decay (Mujika and Padilla, 2003). While the majority of the tapering literature has focused on endurance sport performances, the current literature for tapering and peaking maximal strength is scant.
The evidence for tapering and peaking maximal strength primarily consists of observational (Bazyler et al., 2017, 2018; Travis et al., 2020b) and qualitative research (Pritchard et al., 2016, 2020; Grgic and Mikulic, 2017; Winwood et al., 2018). To date, only two studies have experimentally compared tapering strategies aimed at improving maximal strength: (a) +5% vs. −10% intensity manipulation with a ∼70% volume-load reduction using a step taper (Pritchard et al., 2019), and (b) a step vs. an exponential taper with a ∼54% volume-load reduction while maintaining intensity (Seppänen and Häkkinen, 2020). In summary, these data suggest that a higher intensity taper and an exponential taper over 2-weeks may produce favorable outcomes. However, based on a recent systematic review, the optimal tapering duration for peaking maximal strength may be ≤2 weeks where volume-load is reduced by half while maintaining or reducing intensity (Travis et al., 2020c). Interestingly, survey results from 364 United States and Canadian powerlifters revealed lifters most frequently used a 7–10 day step taper with a 41–50% reduction in volume-load, but varied intensity manipulation (−30 to +10%) (Travis et al., 2021). Tapering for maximal strength appears to be more sensitive to volume-load reductions and possibly the duration of a pre-competition taper, rather than intensity manipulations. Nonetheless, the manner in which volume-load is reduced (e.g., step-fashion, exponentially decayed) over a 1 or 3-week duration with the aim of peaking maximal strength requires further examination.
According to Luden et al. (2010), the majority of tapering literature has focused on targeting taper-induced physiological adaptations relative to cardiovascular, metabolic, and neuromuscular parameters in an attempt to identify physiological factors supporting the ergogenic effect of tapering. However, these aforementioned parameters do not appear to adequately explain enhanced performance adaptations and these characteristics relatively remain unchanged with endurance athletes. Conversely, skeletal muscle size, force, and power characteristics appear to be more sensitive to tapered training with endurance and strength athletes, which could likely potentiate positive taper-induced training outcomes (Murach et al., 2014). Unfortunately, skeletal muscle adaptations at the whole muscle and single fiber levels as a result of tapering are poorly understood and should be considered per the relationships between altered training volume and muscle size (Haun et al., 2019; Travis et al., 2020a). For instance, if the taper duration is too long, negative skeletal muscle adaptations could provide evidence for avoiding long duration tapers (e.g., 3-week exponential taper) for strength athletes due to insufficient stimuli. However, skeletal muscle adaptations are often associated with interpretations that are speculative due to measurement limitations such as ultrasonography. Thus, additional assumptions are made regarding deeper levels of skeletal muscle constituents (e.g., muscle fiber size, myosin, and actin protein concentrations), which are also poorly understood and require further investigation.
Studies with strength athletes have demonstrated whole muscle cross-sectional area (mCSA) is maintained or slightly decreased during or after a taper possibly due to sustained reductions in training volume (Häkkinen et al., 1991; Zaras et al., 2014, 2016; Bazyler et al., 2017, 2018; Suarez et al., 2019; Seppänen and Häkkinen, 2020; Travis et al., 2020b). This is understandable given the direct relationship between training volume and muscle size (Schoenfeld et al., 2014; Haun et al., 2019). While performances can vary as a result of whole muscle maintenance or loss, it is unclear what underlying cellular or molecular changes are taking place at the muscle fiber level with strength-based tapers. Skeletal muscle is highly plastic, which is evidenced by changes in muscle fiber composition in response to different training stimuli (D’Souza et al., 2018; Travis et al., 2020a). Specifically, tapering has been shown to favor myosin-heavy chain (MHC)-IIA isoforms by increasing fiber cross-sectional area (fCSA), peak force, and power output (Trappe et al., 2000b; Neary et al., 2003; Luden et al., 2010; Murach et al., 2014), albeit in endurance athletes. Considering MHC-IIA muscle fibers are the most abundant and largest fibers in powerlifters (Prince et al., 1976; MacDougall et al., 1982; Tesch et al., 1984; Kadi et al., 1999; Fry et al., 2003; Eriksson et al., 2005; Machek et al., 2020), creating a cellular environment that enhances IIA fiber content may be warranted. Unfortunately, it is unknown whether similar muscular adaptations occur in strength athletes following a taper. Further, additional myocellular constituents (e.g., sarcoplasm, gene expression, and protein abundance) driving whole muscle and single fiber adaptations in strength athletes during a taper are still unclear.
When assessing taper-induced MHC isoform shifts, there are concurrent MHC isogenes [e.g., myosin-heavy chain 7 (MYH7), myosin-heavy chain 2 (MYH2), myosin-heavy chain 1 (MYH1)] (McDermott and Bonen, 1991) that encode different types of molecular motors (Goldspink, 2002). Over a decade ago, evidence was provided to support the mechanisms underlying skeletal muscle regulation which was linked to vast arrays of muscle-specific genes encoding for proteins that required specialized functions for contractile apparatuses, enzymes, receptors, and ion channels (Goldspink, 2002; van Rooij et al., 2008). Downstream from gene expression, collections of muscle-specific microRNAs (miR) (i.e., MyomiR) (McCarthy, 2011) mediated by cell proliferation, differentiation, contractility, and stress responses were identified. miR inhibits translation and promotes messenger RNA (mRNA) degradation. Thus, the interactions between genes and miR are vital for understanding molecular mechanisms that influence skeletal muscle adaptations.
Prior work by D’Souza et al. (2017) characterized powerlifters’ muscle phenotype via gene and miR expression suggesting that powerlifters possess unique expression profiles, compared to other populations. However, only one study has attempted to assess these molecular markers during a training protocol with powerlifters, albeit aimed at enhancing muscle hypertrophy (Bjørnsen et al., 2019a). Contrary to the notion that exercise gene response and adaptative potential are attenuated as training status improves, findings by Murach et al. (2014) suggest transcriptional flexibility in MHC-I and -IIA at the gene level can be observed after tapering in collegiate distance runners. Nonetheless, changes in gene and miR expression have not been studied in strength athletes following a taper. Given the influence of muscular adaptations on maximal strength outcomes (MacDougall et al., 1982; Brechue and Abe, 2002; Schoenfeld et al., 2014; Abe et al., 2018a,b), genes mediating muscle phenotype [i.e., SRY-box transcription factor 6 (SOX6), myosin-heavy gene 7 (MYH7), myosin-heavy gene 2 (MYH2), and myosin-heavy gene 1 (MYH1)], and regulating myogenesis [i.e., protein Pax7 (PAX7), Myostatin (MSTN), myogenic differentiation 1 (MyoD), and myogenin (MyoG)] may elucidate tapering-induced muscular adaptations at the molecular level (McCarthy, 2011; D’Souza et al., 2017; Bjørnsen et al., 2019a). In addition to gene expression, examining muscle-specific miRs highly expressed in powerlifters (i.e., miR-133a, -206, -486, and -499a), and indicative of catabolic gene inhibition (i.e., miR-23 and miR-451) may enhance our understanding of the gene-miR interactions with subsequent muscular adaptations following tapering. Whether taper models commonly used by strength athletes exhibit differences in gene expression and corresponding muscle phenotype remains to be investigated.
Currently, it is unclear which tapering model is most effective for peaking maximal strength and positively augmenting skeletal muscle. Additionally, beyond macroscopic assessments, molecular measurements are needed to further understand taper-induced adaptations. Thus, the purpose of this study was to compare performance outcomes and skeletal muscle adaptations following a 6-week peaking program using a step or exponential taper in strength athletes.
Materials and Methods
Ethical Approval and Participant Screening
A total of 16 powerlifters (14 males and 2 females; 24.2 ± 4.0 years; 174.4 ± 8.2 cm; 89.8 ± 21.4 kg) read and signed an informed consent document before beginning the study. This protocol was reviewed and approved by the East Tennessee State Institutional Review Board and complied with the Helsinki Declaration (approved protocol # 0191.15f). Inclusion criteria were as follows: (1) 18–35 years old; (2) free from injury/illness that would hinder participation; (3) regularly trained the powerlifting movements (i.e., back squat, bench press, and deadlift) over the last year; and (4) could demonstrate a 1-repetition-maximum (1RM) strength level ≥1.5× body mass on back squat and deadlift, and ≥1× body mass on bench press. Participants completed all testing and training sessions, and there were no dropouts.
Experimental Design
An experimental design was used to compare a 1-week step vs. a 3-week exponential taper completed during a 6-week peaking program. All participants were familiarized with testing procedures over 4-weeks prior to beginning the study. Before any training, participants underwent a 1RM testing session where they were ranked based on calculated Wilks Score (i.e., coefficient used to compare relative strength across differing body mass and between sex) (Vanderburgh and Batterham, 1999). Matched pairs were randomly assigned to either the step or exponential taper by an assistant unaffiliated with the study. All participants trained 3 days per week at the same time of day. At the end of each training session, participants provided session rating of perceived exertion (1–10) and duration (min), which were used to calculate weekly training monotony and strain (Borg, 1962; Pyne et al., 2000). Participants were instructed to arrive at the laboratory in a fully rested, hydrated state, refrain from training and stimulants, and complete a 48-h dietary log prior to the first testing session to be replicated prior to the second testing session. Participants completed two testing sessions: 1 week prior to any training (T1) and 1 week post-taper (T2) (Figure 1).
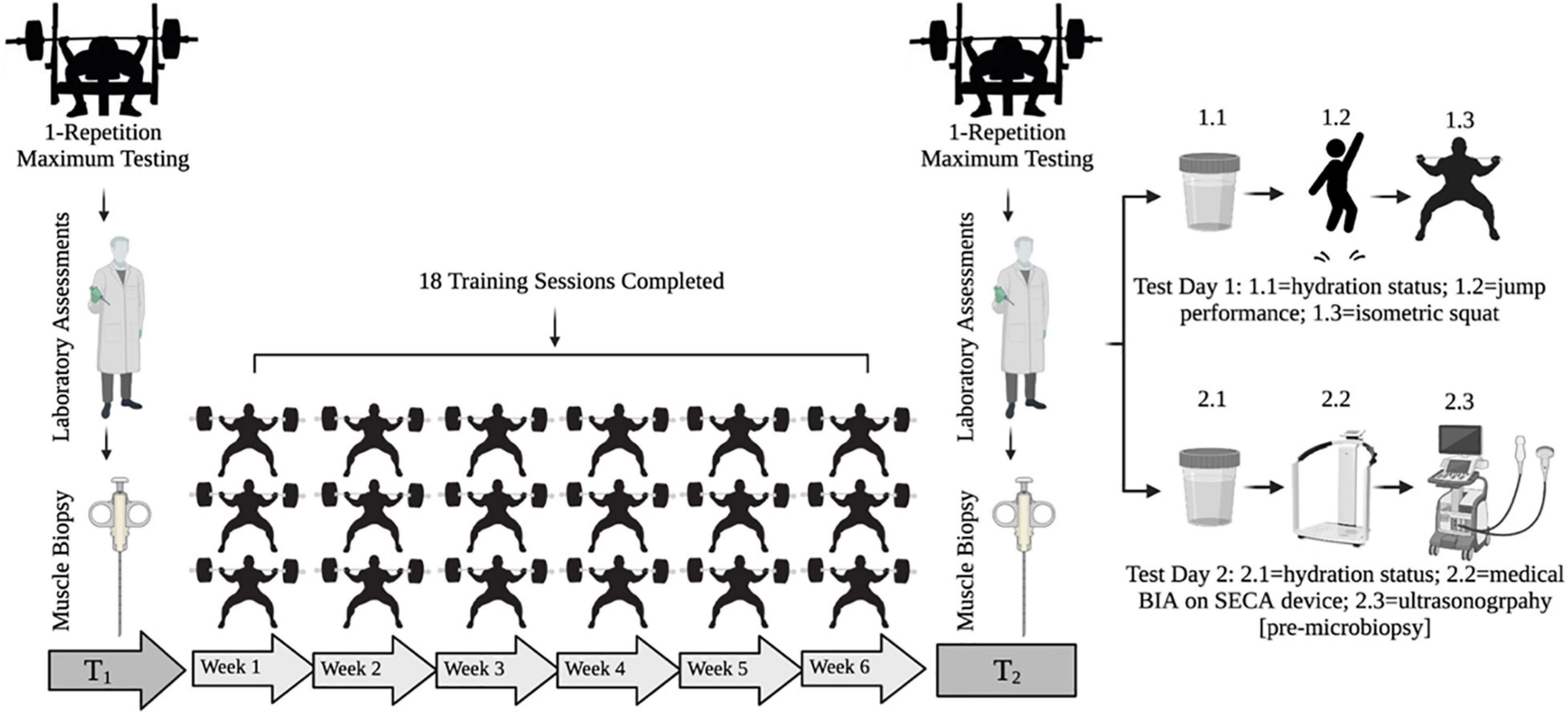
Figure 1. Testing timeline. T1 = pre-training testing week. T2 = post-taper testing week. BIA = bioelectrical impedance analysis.
Training Program
An experimental design was used to compare a 1-week step vs. a 3-week exponential taper completed during a 6-week peaking program. All participants were familiarized with testing procedures over 4-weeks prior to beginning the study. Before any training, participants underwent a 1RM testing session where they were ranked based on calculated Wilks Score (i.e., coefficient used to compare relative strength across differing body mass and between sex) (Vanderburgh and Batterham, 1999). Matched pairs were randomly assigned to either the step or exponential taper by an assistant unaffiliated with the study. All participants trained 3 days per week at the same time of day. At the end of each training session, participants provided session rating of perceived exertion (1–10) and duration (min), which were used to calculate weekly training monotony and strain (Borg, 1962; Pyne et al., 2000; Foster et al., 2001). Participants were instructed to arrive at the laboratory in a fully rested, hydrated state, refrain from training and stimulants, and complete a 48-h dietary log prior to the first testing session to be replicated prior to the second testing session. Participants completed two testing sessions: 1 week prior to any training (T1) and 1 week post-taper (T2) (Tables 1, 2).
Laboratory Testing Procedures
Hydration Assessment
Hydration status was evaluated at the start of each laboratory assessment using a refractometer (ATAGO 4410 PAL-10S, Tokyo, Japan). If urine-specific gravity was ≥1.020, the participant was required to drink water for at least 20 min before hydration status was reassessed. Participants were not allowed to continue testing until urine-specific gravity reached <1.020.
Jump Performance Assessment
Participants performed a standardized dynamic warm-up to prepare for squat jumps (SJ). Unloaded SJs were performed on dual uniaxial force plates affixed side by side with a sampling frequency of 1,000 Hz (Rice Lake Weighing Systems, Rice Lake, WI, United States). The SJ was performed with a near weightless plastic pipe placed across the shoulders to eliminate arm swing. The tester instructed each participant to squat down to a 90° knee angle, which was confirmed with a handheld goniometer. Two warm-ups were completed at 50% and 75% maximal effort. When commanded, each participant was instructed to “step on the force plate,” receive the “ready position” and hold the 90° squat position until the force-time trace was stable for at least 2 s. The tester then shouted “3, 2, 1, jump!” and the participant executed a maximal effort jump. Using the live SJ jump-height (SJH) metric, at least two jumps were performed within a range of ≤2 cm. All jump trials were recorded and analyzed using a custom program (LabView, 2018, National Instruments Co., Austin, TX, United States). Variables of interest (SJH: interclass correlation coefficient (ICC) = 0.96, coefficient of variation (CV) = 2.55%; peak power allometrically scaled for body mass (PPa) (ICC = 0.96, CV = 2.08%) yielded repeated measurement values consistent with previous reports from our laboratory (Bazyler et al., 2018; Travis et al., 2020b).
Isometric Squat Assessment
Participants were positioned in a custom designed power rack that allows fixation of the bar at any height as described previously (Bazyler et al., 2015). Each participant’s bar height was determined by a 90° knee angle confirmed with a handheld goniometer referencing the greater trochanter, lateral epicondyle, and lateral malleolus for the appropriate isometric squat (ISQ) position. The same investigator measured bar height and knee angle, which was replicated at each testing session. Kinetic variables were measured on dual uniaxial force plates affixed side by side with a sampling frequency of 1,000 Hz. Prior to maximal testing, two warm-up trials were provided at 50 and 75% maximal effort. At least two maximal effort trials were administered. Additional trials were provided if isometric peak force (IPF) values were ≥100 N. The ISQ trials with the two highest IPF values were analyzed using a custom program (LabView, 2018, National Instruments Co., Austin, TX, United States). Test–retest reliability for ISQ IPF was nearly perfect (ICC = 0.99, CV = 1.97%).
One-Repetition-Maximum Assessment
Participants underwent two 1RM testing sessions at T1 and T2 in the form of mock competitions aimed at achieving a true 1RM. Both mock competitions were supervised and performed in accordance with USA Powerlifting (USAPL) rules while using validated 1RM and attempt selection procedures (Zourdos et al., 2016; USAPL and Administrators, 2019; Travis et al., 2020d). The primary investigator determined load increases for each attempt for all participants under the following conditions: (a) a rating of perceived exertion of 10 being recorded and the investigator determined that any load increase would not result in a successful attempt or the participant failing on any subsequent attempt thereafter or (b) a recorded rating of perceived exertion of 9 or 9.5 and then the participant failing on the subsequent attempt with a load increase of ≤2.5 kg.
Skeletal Muscle Measurements
Body Composition Assessment
A medical body composition analyzer (SECA mBCA 515 v1.1 Hamburg, Germany) using bioelectrical impedance analysis (BIA) was used to determine body mass, fat mass (FM), fat free mass (FFM), fat mass index (FMI), fat free mass index (FFMI), total body water (TBW) [i.e., composed of extracellular water (ECW) and intracellular water], and skeletal muscle mass. Impedance was measured at frequencies ranging from 1 to 1,000 kHz (Peine et al., 2013). The measurement scanning sequence was performed segmentally in accordance with the user instruction manual in the following order: right arm, left arm, right leg, left leg, trunk, right body side, and left body side (Peine et al., 2013). Test–retest reliability was nearly perfect for all SECA variables with an ICC = 0.98 to 0.99 and CV = 1.76 to 3.41% (Bosy-Westphal et al., 2013, 2017; Peine et al., 2013; Jensen et al., 2019).
Ultrasonography
Anatomical mCSA of the right vastus lateralis was assessed using 2D ultrasonography (LOGIQ P6, General Electric Healthcare, Wauwatosa, WI, United States). A 7.5 MHz ultrasound probe was covered with water-soluble transmission gel to aid acoustic coupling. Depression of the skin was avoided while collecting the cross-sectional image. Sampling locations of interest were mCSA1/3 (proximal), mCSA1/2 (middle), and mCSA2/3 (distal) of femur length, which was determined by the distance between the greater trochanter and the lateral epicondyle of the femur. Each sub-region was measured, recorded, and marked with a permanent marker to guide biopsy procedures relative to the area of interest. Each participant laid on their left side in a recovery position with hips perpendicular to the examination table in the axial plane. The mean of three images from each sub-section was used for analysis. For analysis, mCSA was measured by tracing the inter-muscular interface around each muscle cross-sectional image using a secondary software (Image-J Fiji version 2.0.0-rc-68/1.52g, National Institutes of Health, Bethesda, MD, United States) (Schindelin et al., 2015). mCSA from individual sites (mCSA1/3, mCSA1/2, and mCSA2/3) and the mean of three sites (mCSAavg) were analyzed to assess changes in regional and whole muscle size, respectively. Test–retest reliability was nearly perfect (ICC: 0.99; CV = 0.98%) and agrees with previous reports published from the same technician (Travis et al., 2020b).
Muscle Biopsy
Following ultrasound scans, local anesthesia (1% xylocaine, Hospira, Inc., Lake Forest, IL, United States) was injected subcutaneously in the right vastus lateralis at the site corresponding to the mCSA1/2 femur length ultrasound marking. Muscle biopsy samples were obtained using a 14G × 9 cm biopsy instrument with a 13G × 3.9 cm co-axial introducer (MCXS1409LX SuperCoreTM Semi-Automatic Biopsy Instrument, Argon Medical Devices, Frisco, TX, United States). After placing the introducer needle, 5–6 passes were performed with the biopsy needle extracting approximately 15–20 mg of muscle tissue per pass. As a result, muscle samples totaled approximately 75–120 mg, which were separated from connective and adipose tissue. Approximately four fascicles were removed from the total muscle sample and mounted in duplicate on corks in tragacanth gum/optimal cutting temperature mixture (Thermo Fisher Scientific Inc., Waltham, MA, United States) and frozen in liquid N2-cooled isopentane for histological analyses. The remaining tissues were then weighed, equally divided into 3–4 CryoTube® vials (Nunc®, Roskilde, Denmark), flash frozen in liquid nitrogen, and stored in −80°C for subsequent analyses. These procedures were implemented at T1 and T2.
Immunohistochemical Analysis
Cork mounted samples were cut into 10 μm cross-sections using a cryostat (Model Microm HM 505; Thermo Fisher Scientific Inc.) and mounted on positively charged microscope slides (FisherbrandTM Superfrost Plus; Fisher Scientific, Pittsburgh, PA, United States). Section quality and tissue integrity were assessed using hematoxylin and eosin staining. MHC fiber type immunofluorescent detection was performed using published methods with modifications for human skeletal muscle (Lawrence et al., 2021). Muscle sections were blocked with 10% normal goat serum in 1 × PBS and incubated overnight (12 h) in 4°C with a primary antibody (1°Ab) cocktail obtained from the Developmental Studies Hybridoma Bank (DSHB) (Iowa City, IA, United States) containing MHC-IIA (SC-71 dilution 1:25), MHC-IIX (6H1 dilution 1:100), and Laminin (28E dilution 1:12.5). MHC-I was left unstained to optimize the immunofluorescent signal to noise ratio and to minimize non-specific binding in the background of images. After an overnight incubation, a 3 × 5-min wash at room temperature was completed using PBS/0.1% Triton-X100 prior to and following a 1 h incubation at room temperature with the secondary antibody (2°Ab) cocktail with specific fluorophores directed to each 1°Ab. These included MHC-IIA/Alexa Fluor 350 (IgG1 dilution 1:100; Invitrogen #A21120), MHC-IIX/Alexa Fluor 488 (IgM dilution 1:500; Invitrogen #A21042), and Laminin/Alexa Fluor 555 (IgG2a dilution 1:250 #A21137). Slides were then mounted with an anti-fade mounting medium (Vectashield HardsetTM; Vector Laboratories, Burlingame, CA, United States) prior to microscopy imaging. Multi-channel 5 × 5 tile scanned images of entire cross-sections were captured with an incubated confocal laser scanning microscope (Zeiss LSM 880 with Airyscan; Zeiss International, Oberkochen, Germany). Images were processed for fCSA and MHC content using ImageJ software (ImageJ 1.53a, Java 1.8.0_172_64-bit, National Institutes of Health, Bethesda, MD, United States). Due to the lack of MHC-IIX fibers, only MHC-I and MHC-IIA fibers were used for the final analysis.
Single Fiber Analysis
Single Fiber Isolation and Permeabilization
Approximately 25–30 mg of tissue from each biopsy was placed in skinning solution for later analyses of permeabilized single muscle fibers to assess MHC content. Our chemical based skinning solution contained (in mM) 125 potassium propionate, 2.0 EGTA, 4.0 ATP, 1.0 MgCl2, and 20.0 imidazole (pH 7.0) and 50% (vol/vol) glycerol as previously described (Trappe et al., 2000b). A total of 105 ± 4 single muscle fibers that were 1–3 mm in length were isolated from tissue bundles using jeweler’s forceps. The number of single fibers used for analysis is justified by recent work from Murach et al. (2016) showing that (a) single fiber phenotyping results are the same for 25 vs. 125 fibers; and (b) false discovery rate was 0% beyond 25 fibers. Thus, any analysis >25 fibers can reliably estimate fiber type distribution of a larger sampling of fibers (Murach et al., 2016). Single fibers were placed into individually labeled 0.6-mL microcentrifuge tubes containing 10 μL of sodium dodecyl sulfate (SDS) loading buffer (0.125 M Tris–HCl, 10% glycerol, 4% SDS, 4 M urea, 10% 2-mercaptoethanol, 0.001% bromophenol blue, pH 6.8 diluted 2:1 with 1× Tris–HCl [pH 6.8]) (Christiansen et al., 2019). Samples remained in SDS loading buffer at room temperature for at least 2 h prior to immunoblotting and then placed in −20° for storage (Figure 2).
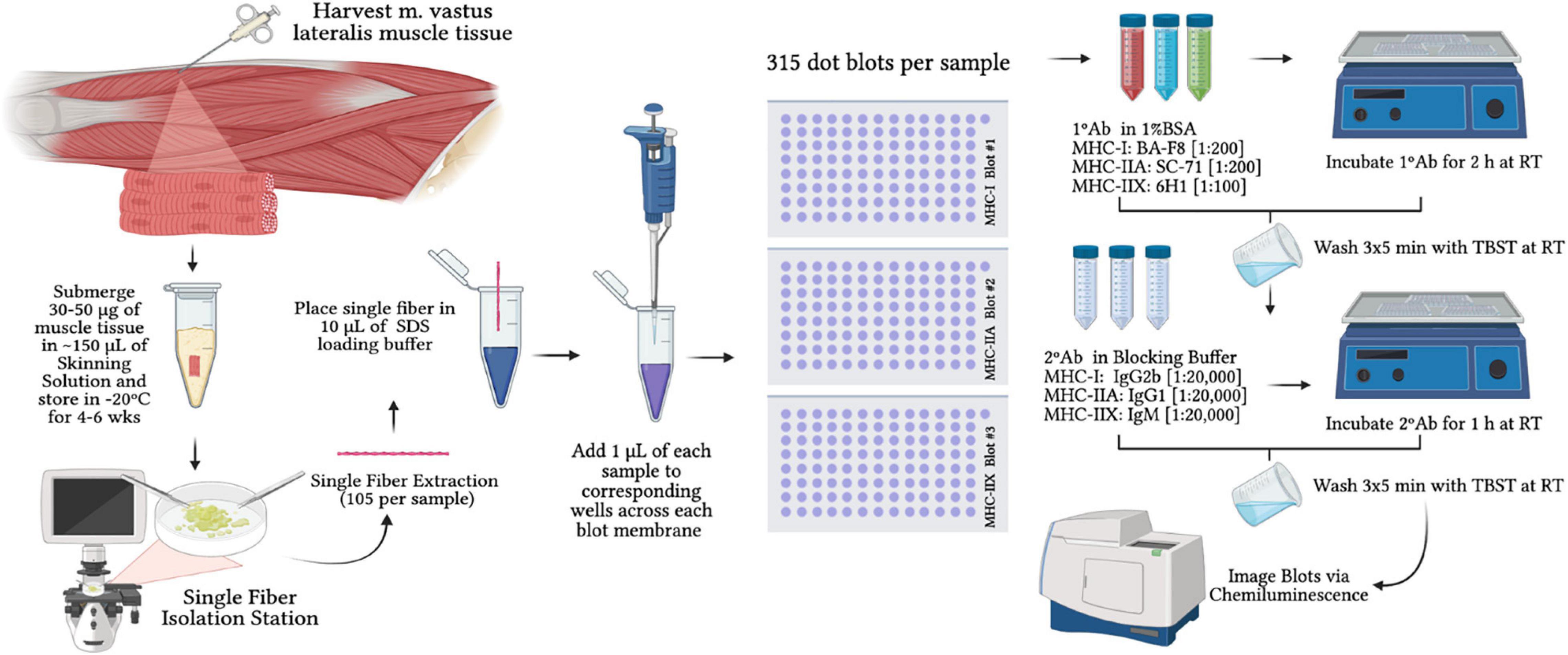
Figure 2. Schematic of single fiber isolation and dot blot timeline for myosin-heavy chain analysis. SDS = sodium dodecyl sulfate; MHC-I Blot #1 = immunoblot treated for myosin-heavy chain I; MHC-IIA Blot #2 = immunoblot treated for myosin-heavy chain IIA; MHC-IIX Blot #3 = immunoblot treated for myosin-heavy chain IIX; 1°Ab = primary antibody; 2°Ab = secondary antibody; 1%BSA = 1% bovine serum albumin; RT = room temperature.
Single Fiber Phenotyping
An immunoblotting dot blot protocol previously described (Christiansen et al., 2019; Lamboley et al., 2020) was modified and used to determine pure MHC-I, -IIA, -IIX, and hybrid-I/IIA content of single fibers. Hybrid MHC-IIA/IIX fiber type determination was not possible with this method due to MHC-IIX antibody overstaining and non-specific binding as explained by Christiansen et al. (2019) To begin dot blotting, samples were solubilized by vortexing for ∼5 s. Three PVDF membranes cut to 8 cm × 13 cm were activated with 100% methanol and equilibrated in transfer buffer. The wet membranes were placed on top of a single piece of dry filter paper and a 96 well wafer was affixed atop each membrane (Figure 2). For each sample per subject, 1 μL of each fiber was applied to the same corresponding well of each wet membrane and allowed to dry. The dry membranes were then reactivated with 100% methanol and equilibrated in transfer buffer (25 mM Tris, 192 mM glycine, pH 8.3 and 20% methanol). Per our modifications, each blot was placed inside individual PerfectWesternTM blot boxes (GenHunter Corporation, Nashville, TN, United States) to optimize each MHC signal, avoid Ab cross-activity, and to eliminate stripping of proteins. Each blot was quick washed for ∼30 s three times in Tris-buffered saline containing 0.1% Tween20 (TBST), and then placed in blocking buffer (5% non-fat dry milk in TBST) for 5 min. Following blocking, membranes were rinsed with TBST and incubated individually for 2 h with gentle rocking with 1°Ab obtained from DSHB diluted in 1% BSA/PBST. Each 1°Ab corresponded to labeled blot boxes for blot box #1 for MHC-I (BA-F8 dilution 1:200), blot box #2 for MHC-IIA (SC-71 dilution 1:200), and blot box #3 for MHC-IIX (6H1 dilution 1:100). After 1°Ab incubations, membranes were washed 3 × 5 min with TBST and then incubated in goat anti-mouse IgG horseradish peroxidase 2°Ab at room temperature while gentle rocking for 1 h. Each 2°Ab was obtained from Invitrogen and diluted in blocking buffer corresponding to blot box #1 for MHC-I (IgG2b; diluted 1:20,000), blot box #2 for MHC-IIA (IgG1; diluted 1:20,000), and blot box #3 for MHC-IIX (IgM; diluted in 1:20,000). Lastly, the 3 × 5 min wash was repeated and membranes were individually exposed to clarity enhanced chemiluminescence reagent (SuperSignalTM West Dura Extended Duration Substrate; Thermo Fisher Scientific Inc.) for molecular imaging (ChemiDocTM XRS; Bio-Rad Laboratories, Inc.) using Immun-Star HRP settings in ImageLabTM software (Bio-Rad Laboratories, Inc.).
It was possible to determine pure MHC-I, -IIA, and -IIX content per each blot probed for specific 1° Ab and 2°Ab as well as hybrid MHC-I/IIA (Figure 3). If no MHC protein was present, the corresponding well for all 3 blots produced a blank which may have indicated an unsuccessful collection of a fiber. Additionally, a process of elimination was used to determine MHC content as described previously (Christiansen et al., 2019). Christiansen et al. (2019) determined that the quantification of MHC-I, -IIA, -IIX, and -I/IIA is a reliable and valid phenotyping method compared to Western Blots using a total of 40 single fibers. In this study, we compared the fiber types of ∼3,000 fibers from immunohistochemical (IHC) cross-sections to ∼3,000 single fibers which produced a very strong relationship between the two methods (r2 = 0.94) per pure fiber content. It appeared that the pure MHC output was similar between each method; however, the dot blot protocol produced higher sensitivity for I/IIA hybrid fibers whereas IHC output showed greater sensitivity for revealing possible IIA/IIX hybrid fibers. Our methodology is in agreeance with previous literature showing that single fiber phenotyping with strength and power athletes can accurately assess the presence of pure and hybrid fibers compared to the common over- and under-estimations produced from traditional IHC analyses (Serrano et al., 2019). A recent study by Lamboley et al. (2020) also used dot blot phenotyping to confirm force output for MHC-I and MHC-II muscle fibers and suggest that the data of both methods were in 100% agreement.
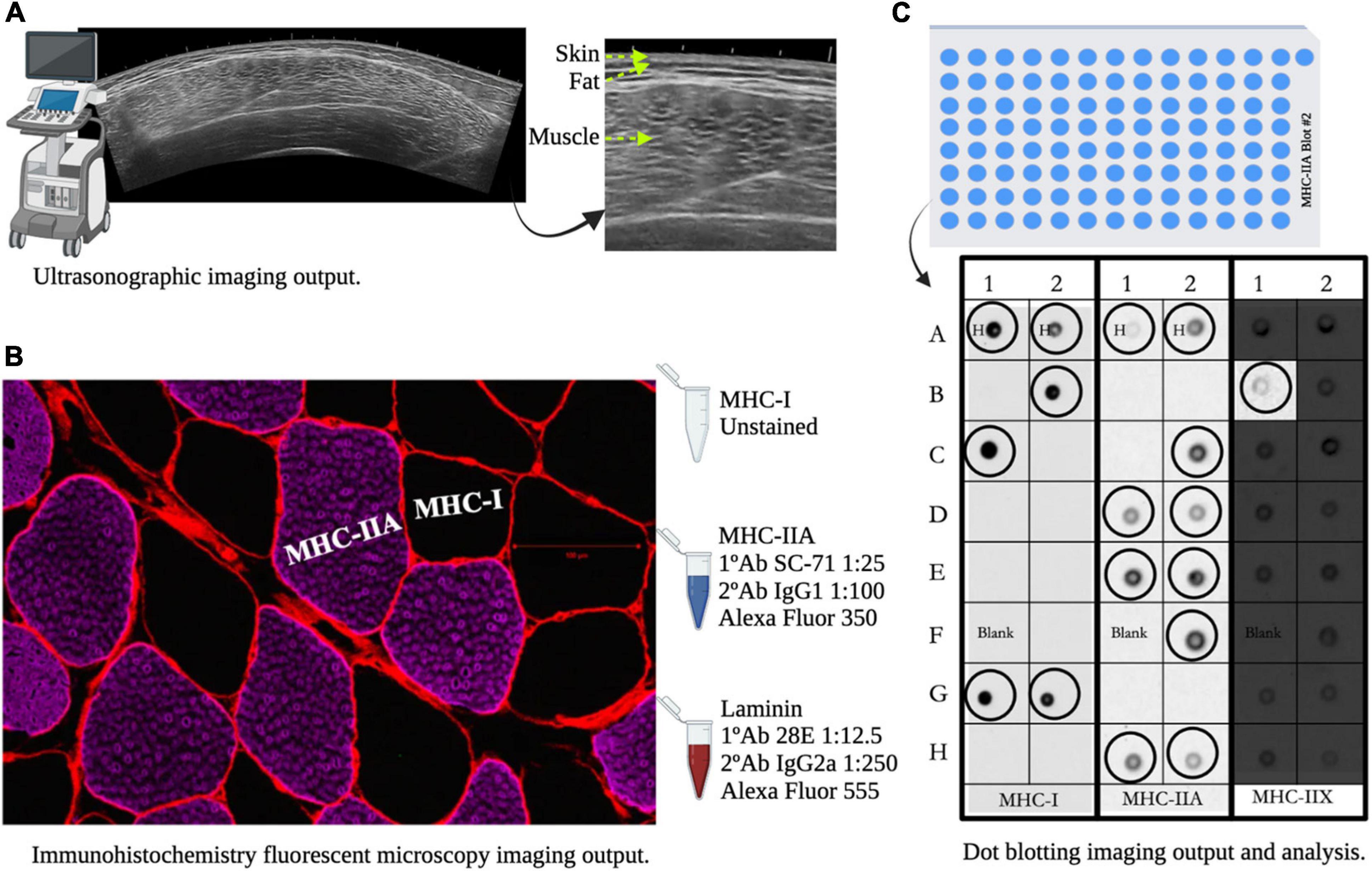
Figure 3. Muscle measurements for B-mode ultrasonography whole muscle imaging, immunohistochemical muscle fiber analysis, and single fiber dot blot analysis. Panel (A) shows image differences between skin, subcutaneous fat, and skeletal muscle mass of the vastus lateralis. Panel (B) shows cell border staining (Laminin), unstained myosin-heavy chain (MHC)-I fibers (Black) and fibers that positively stained for MHC-IIA (Magenta). Panel (C) shows dot blotting output and analysis procedures: circled dot = fiber detected, H = hybrid MHC-I/IIA detection, and Blank = example of no fiber detection.
Muscle Messenger RNA and MicroRNA Analyses
Total RNA was extracted from ∼20 mg of tissue from the vastus lateralis biopsy using the AllPrep® DNA/RNA/miRNA Universal Kit (QIAGEN GmbH, Hilden, Germany) following the manufacturer’s instructions. Briefly, frozen muscle samples were homogenized on ice in Lysing Matrix D tubes (MP Biomedicals, Irvine, CA, United States) using the Cool Prep 24 homogenizer (MP Biomedicals) followed by RNA purification. RNA quality was verified spectrophotometrically using the A260/A280 ratio ≥1.8 determined using a Nanodrop 2000 (Thermo Scientific, Rockford, IL, United States). Reverse transcription of RNA-to-complimentary DNA (cDNA) was conducted using a High-Capacity reverse transcription kit (Life Technologies, Carlsbad, CA, United States) performed with a GeneAmp® PCR System 9700 (Applied Biosystems; Foster City, CA, United States). cDNA was quantified using a nanodrop and aliquots were stored at −20°C for real time-polymerase chain reaction (RT-PCR) analysis. Genes and miRNAs of interest were based on previous literature demonstrating significant roles in hypertrophy, strength, and fiber type changes relative to a given training stimulus or controls (D’Souza et al., 2017, 2019; Bjørnsen et al., 2019a). Genes selected for analysis were the following: PAX7, MSTN, MyoD, MyoG, SOX6, MYH7, MYH2, and MYH1 (Supplementary Table 1). Additionally, endoplasmic reticulum membrane protein complex subunit 7 (EMC7), charged multivesicular body protein 2A (CHMP2A), and chromosome 1 open reading frame 43 (C1orf43) were used as housekeeping genes for reference (Eisenberg and Levanon, 2013; D’Souza et al., 2017). Target miRNAs selected for quantitation were miR-23a-5p, -133a-3p, -206, -451a, -486-5p, -499a-3p, while miR-186-5p and -361-5p were used for reference (TaqMan® Advanced miRNA Assays, Thermo Fisher Scientific) (Vandesompele et al., 2002; McCarthy, 2011; D’Souza et al., 2017). All mRNA primer sequences were designed using a BLAST software and determined based on previously published primer reports (Supplementary Table 2) (D’Souza et al., 2017).
Quantitative RT-PCR (qPCR) analysis of mRNA for target genes was conducted using Power SYBRTM Green I Master Mix (Applied Biosystems) using gene specific primers. Additionally, target miRNAs analyses were conducted using TaqMan® Fast Advanced Master Mix (Applied Biosystems). Samples and reagents were loaded in a MicroAmp Fast-Optical 96 Well Reaction Plate and run in triplicate. Plates were analyzed on a 7500 Fast RT-PCR System (Applied Biosystems). Relative mRNA levels were normalized to CT calculations from housekeeping genes. Standard and melting curves were performed for every target to confirm primer efficiency and single-product amplification. The abundance of mRNA was measured using the 2–ΔΔCT method (Livak and Schmittgen, 2001; Schmittgen and Livak, 2008).
Statistical Analyses
The results are presented as mean values and standard deviations (mean ± SD). A 2 × 2 repeated measures analysis of variance (ANOVA; Group × Time) was used to test all performance and physiological variables. Significant main effects were followed by post hoc pairwise comparisons using a Holm-Bonferroni adjustment. Effect sizes were determined using Hedge’s g (g) and classified as small ≤0.20, medium between >0.20 and <0.60, and large >0.60 (Hedges, 1980). Alpha level for significance was defined as p ≤ 0.05. All analyses were performed using IBM SPSS Statistics v.27 (SPSS Inc., Chicago, IL, United States) and Microsoft Excel v16.49 (Microsoft Corporation, Redmond, WA, United States).
Results
Training Volume-Load, Monotony, and Strain
There was a significant group by time interaction (p < 0.001), and main time effect (p < 0.001) for training volume-load. Post hoc analyses revealed volume-load during weeks 2–5 was significantly greater than week 1 volume load within each group (p < 0.05). However, week 6 volume-load was significantly less than week 1 volume-load in the step (p < 0.001) and exponential taper group (p < 0.001). Further, volume-load was significantly different between groups during week 3 (p = 0.019) and week 5 (p = 0.001) corresponding to the overreach week during the exponential and step taper, respectively. However, total volume-load completed during the 6-week program (step taper: 213,323 ± 50,066 kg vs. exponential taper: 203,568 ± 35,260 kg) was not significantly different between groups (Figures 4A,B).
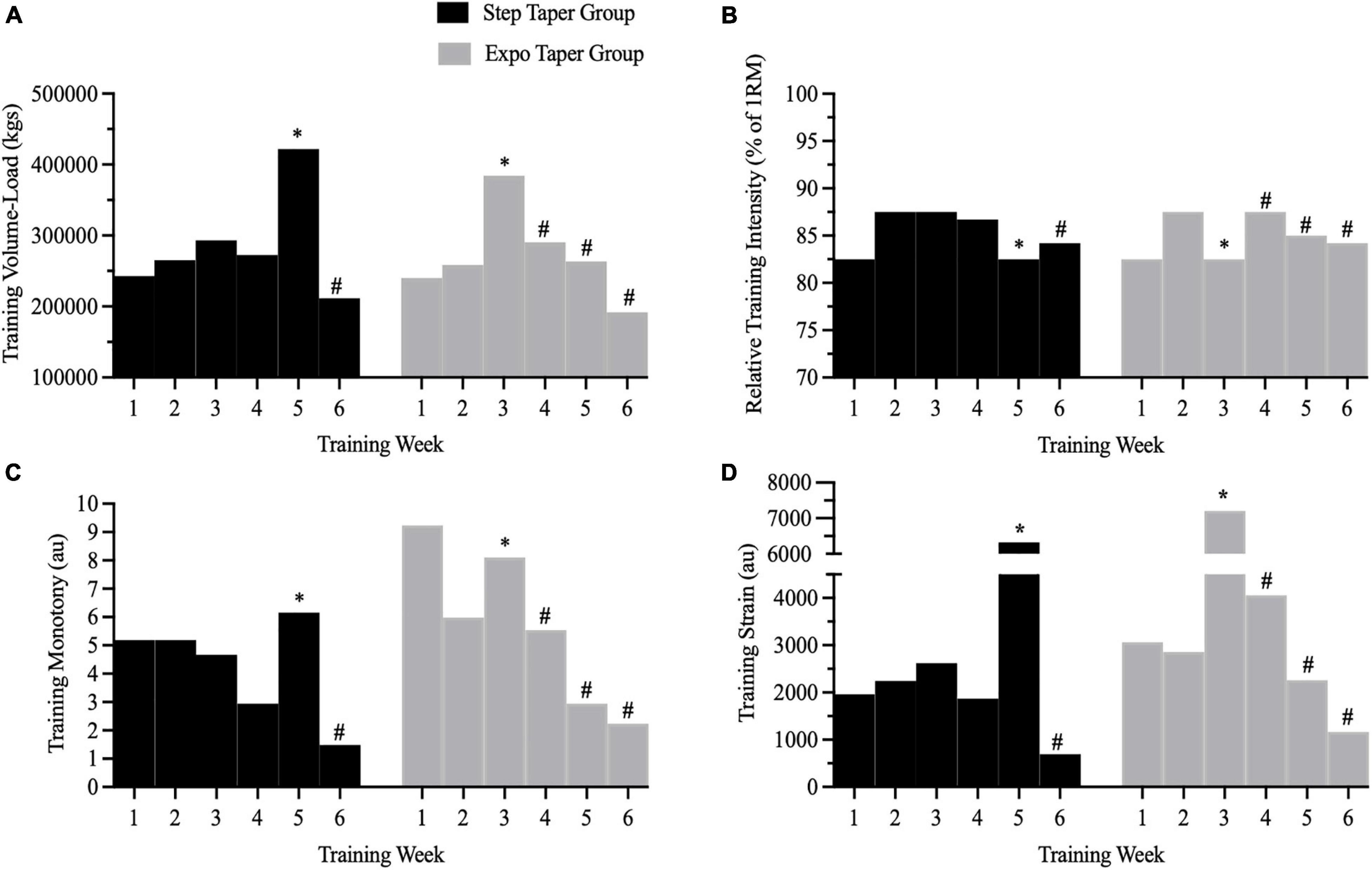
Figure 4. Training metrics from all work completed across the 6-week peaking program. Panels represent (A) training volume-load, (B) relative training intensity, (C) training monotony, and (D) training strain. ∗ = planned overreach week; # = taper weeks.
There was a significant group by time interaction (p = 0.016), and main time effect (p < 0.001) for training monotony. Post hoc analyses revealed training monotony was significantly lower during weeks 2 (p = 0.023), 4 (p = 0.021), 5 (p = 0.025), and 6 (p < 0.001) compared to week 1 in the exponential taper group. However, only training monotony during week 6 (p = 0.023) was lower than week 1 in the step taper group. Further, training monotony was significantly greater during week 5 in the step taper group compared to the exponential taper group (p = 0.027). Total training monotony during the 6-week program was not significantly different between groups (Figure 4C).
There was a significant group by time interaction (p < 0.001), and main time effect (p < 0.001) for training strain. Post hoc analyses revealed training strain was significantly greater during week 3 (p = 0.002), and lower during week 6 (p = 0.006) compared to week 1 in the exponential taper group. Training strain was significantly greater during week 5 (p = 0.007), and lower during week 6 (p = 0.05) compared to week 1 in the step taper group. Further, training strain was significantly greater during week 3 (p = 0.009) in the exponential taper group compared to the step taper group, but vice-versa during week 5 (p = 0.028) corresponding to the overreach weeks in each group. Total training strain during the 6-week program was not significantly different between groups (Figure 4D). There were no other significant main effects for training volume-load, monotony, and strain.
Performance Assessments
For maximal strength, there were significant main time effects for back squat 1RM (p < 0.001), bench press 1RM (p < 0.001), deadlift 1RM (p = 0.024), powerlifting total (p < 0.001), and Wilks Score (p < 0.001) (Figure 5A and Table 3). Post hoc pairwise comparisons revealed statistically significant increases in back squat 1RM (p = 0.002, g = 0.37; p < 0.001, g = 0.54), bench press 1RM (p < 0.001, g = 0.38; p < 0.001, g = 0.35), powerlifting total (p = 0.003, g = 0.25; p < 0.001, g = 0.48), and Wilks Score (p = 0.003, g = 0.36; p < 0.001, g = 0.55) following the step-taper and the exponential taper, respectively. However, deadlift 1RM (p = 0.009, g = 0.48) significantly increased following the exponential taper only. There was as a significant main time effect for SJ PPa (p = 0.001), but not for SJH or ISQ IPFa (Table 3). Post hoc pairwise comparisons revealed statistically significant increases for SJ PPa (p = 0.001, g = 0.84) following the exponential taper only. No significant interactions or main effects were observed for any other performance measure.
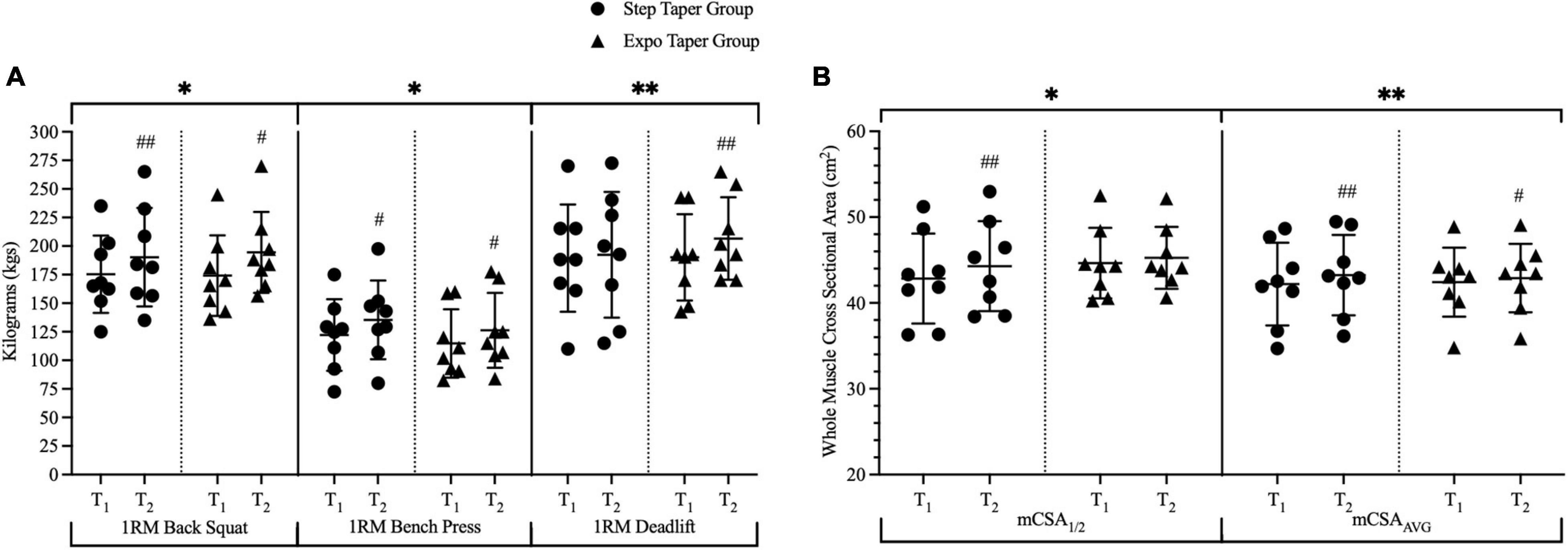
Figure 5. Changes over time for 1-repetition-maximum performances and whole muscle cross-sectional area. Panel (A) represents all lifts that were completed during 1RM testing and panel (B) represents the significant changes in whole muscle measurements via ultrasound. Data is represented by means ± standard deviations. Significant main time effects: ∗p ≤ 0.05, ∗∗p ≤ 0.001. Significant group change from baseline: #p ≤ 0.05, ##p ≤ 0.001. T1 = pre-training; T2 = post-taper; 1RM = 1-repetition-maximum; mCSA = muscle cross-sectional area; mCSA1/2 = medial vastus lateralis measurement; mCSAAVG = average of three sites for vastus lateralis measurements.
Body Composition Assessments
For body composition assessments, there were significant main time effects for body mass (p = 0.005), FM (p = 0.002), and FMI (p = 0.002) (Table 3). Post hoc pairwise comparisons revealed statistically significant increases in the step taper group for body mass (p = 0.021, g = 0.08), FM (p = 0.005, g = 0.08), and FMI (p = 0.010, g = 0.08), but only significant increases in the exponential taper group for body mass (p = 0.047, g = 0.05) and FMI (p = 0.038, g = 0.05). No significant interactions or main effects were observed for any other body composition measure.
Skeletal Muscle Assessments
At the whole muscle level, there were significant main time effects for mCSA1/2 (p = 0.006) and mCSAavg (p < 0.001) (Figure 5B). Post hoc pairwise comparisons revealed significant increases in mCSA1/2 (p = 0.007, g = 0.26) and mCSAavg (p < 0.001, g = 0.21) following the step taper, and significant increases in mCSAavg (p = 0.047, g = 0.11) following the exponential taper. Main time effects for mCSA1/3 (p = 0.077, g = 0.13) and mCSA2/3 (p = 0.067, g = 0.11) exhibited small effect sizes, but did not reach significance. There were no significant interactions or main effects observed for other whole muscle measurements.
At the muscle fiber level using IHC analysis, there was a significant group by time interaction for MHC-IIA fCSA (p = 0.014) (Figure 6A). There were also significant main time effects for fCSAavg (p = 0.020) and MHC-IIA fCSA (p = 0.010). Post hoc pairwise comparisons revealed statistically significant increases in fCSAavg (p = 0.010, g = 0.90) and MHC-IIA fCSA (p = 0.002, g = 1.07) only following the step taper.
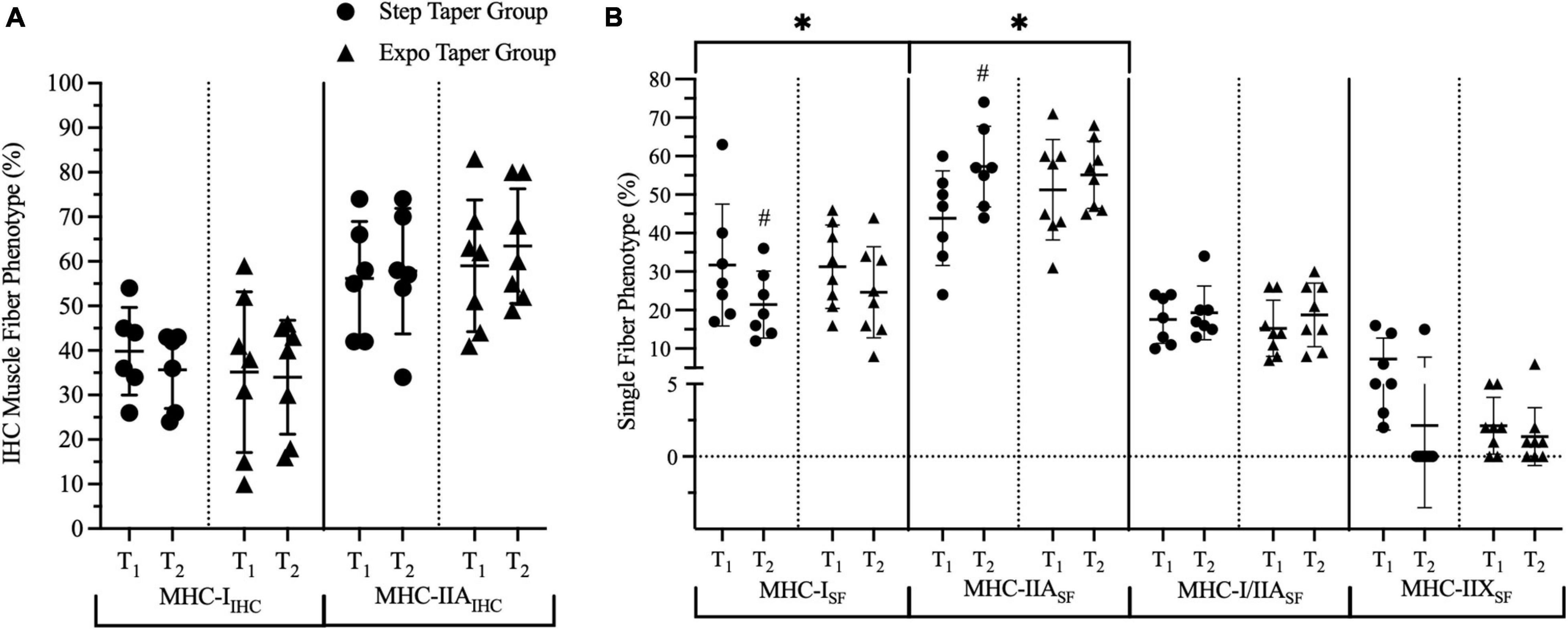
Figure 6. Phenotype composition based on myosin-heavy chain content. Panels represent fiber composition per (A) immunohistochemistry for type I and IIA fibers and (B) single fiber analyses for type I, IIA, I/IIA, and IIX. Data is represented by means ± standard deviations. Significant main time effects: ∗p ≤ 0.05. Significant group change from baseline: #p ≤ 0.05. T1 = pre-training; T2 = post-taper; IHC = immunohistochemistry; SF = single fiber; MHC = myosin-heavy chain; MHC-I = myosin-heavy chain I; MHC-IIA = myosin-heavy chain IIA; MHC-I/IIA = myosin-heavy chain I/IIA hybrid; MHC-IIX = myosin-heavy chain IIX.
At the isolated single muscle fiber level using immunoblot dot blotting analysis, there were significant main time effects for MHC-ISF% (p = 0.015) and MHC-IIASF% (p = 0.033) (Figure 6B). Post hoc pairwise comparisons revealed a significant decrease in MHCISF% (p = 0.037, g = 0.78), and a significant increase in MHC-IIASF% (p = 0.023, g = 1.11) only following the step taper. The main time effect for MHC-IIXSF% (p = 0.087, g = 0.63) exhibited a large effect size, but did not reach statistical significance.
At the molecular level using mRNA and miRNA analyses, there were significant main time effects for MyoD (p = 0.002), MyoG (p = 0.037), and miR-499a (p = 0.033) (Figure 7). Post hoc pairwise comparisons revealed significant decreases in MyoD (p = 0.002, g = 1.60) only following the step taper. Main time effects for Sox6 (p = 0.053, g = 0.65), MYH1 (p = 0.08, g = 0.49), MSTN (p = 0.053, g = 0.39), and miR-486 (p = 0.06, g = 0.99) exhibited moderate to large effect sizes, but did not reach statistical significance. No additional significant myocellular changes were observed.
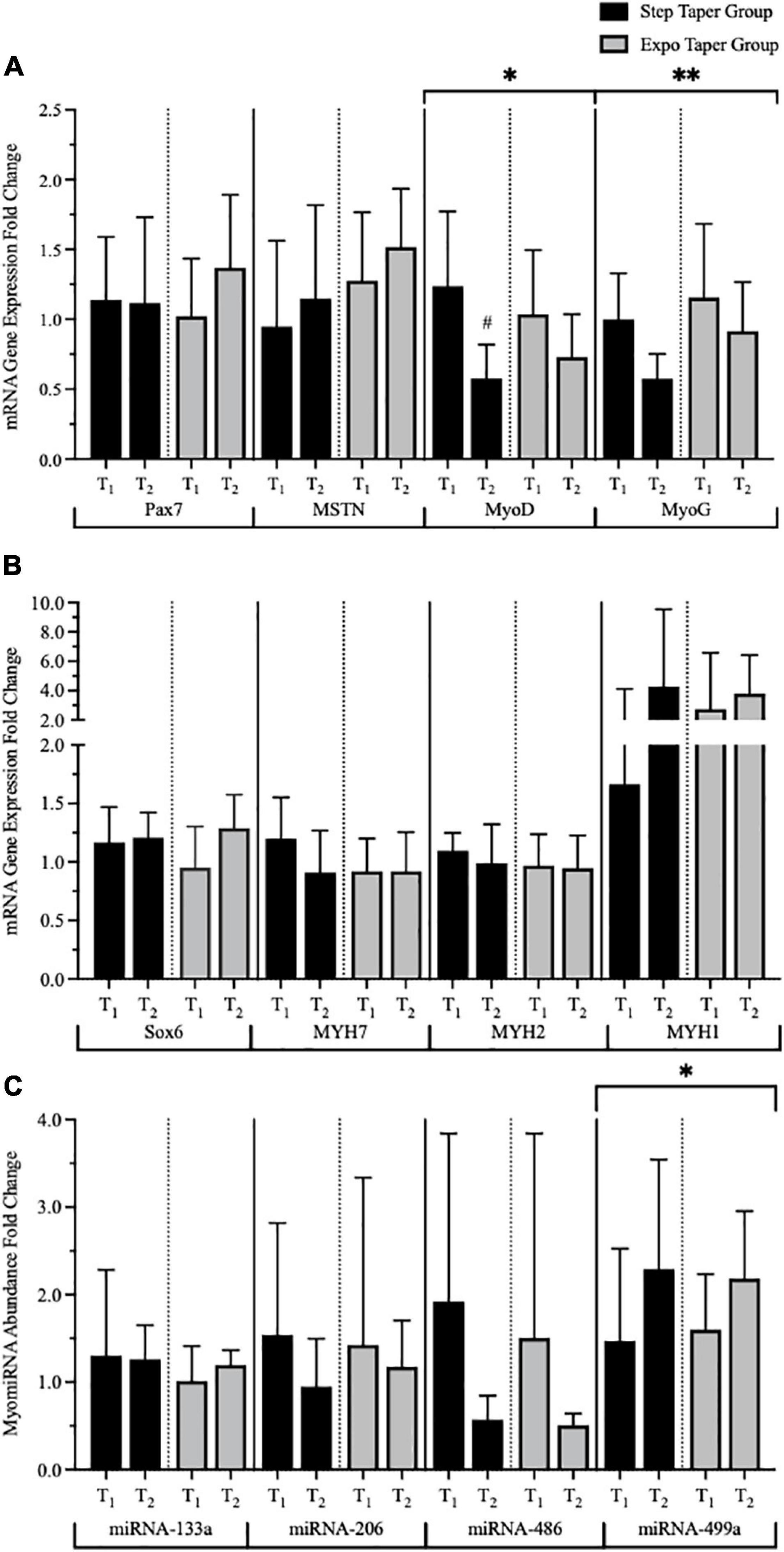
Figure 7. Messenger RNA (mRNA) gene expression and myomiRNA abundance over time. Panels represent (A) myogenic mRNA gene expression, (B) phenotype mRNA gene expression, and (C) myomiR abundance. Data is represented by means ± standard deviations. Significant main time effects: ∗p ≤ 0.05, ∗∗p ≤ 0.001. Significant group change from baseline: #p ≤ 0.05. T1 = pre-training; T2 = post-taper; Pax7 = Paired Box 7; MSTN = Myostatin; MyoD = Myogenic Differentiation 1; MyoG = Myogenin; Sox6 = SRY-Box Transcription Factor 6; MYH7 = β-myosin-heavy chain for slow twitch skeletal muscle; MYH2 = myosin-heavy chain 2; MYH1 = striated muscle myosin-heavy chain 1. miRNA = microRNA.
Discussion
This is the first study to compare performance changes coupled with skeletal muscle adaptations at the whole muscle, muscle fiber, and molecular levels between two tapering models in powerlifters. Our main findings indicate equated volume-loads over 6-weeks produced similar outcomes following the step and exponential tapers with some exceptions. Changes in 1RM performance were similar between taper models; however, changes in deadlift 1RM favored the exponential taper. Secondary performance assessments (i.e., SJH and ISQ IPF) did not appear to be as sensitive to the peaking program compared to 1RMs. Skeletal muscle can be positively augmented based on mCSA increases following both tapers, but single fiber MHC phenotype changes appear to favor the step taper. However, the overall lack of differences between taper models is likely attributed to the similar total workload completed by both groups, whereas the distribution of work may explain the favorable muscular adaptations observed in the step taper group. Thus, the findings from these two taper models warrant further discussion.
Tapering prior to competition has been shown to improve competition and laboratory-based performances (Mujika et al., 2002; Mujika and Padilla, 2003; Seppänen and Häkkinen, 2020; Travis et al., 2020b,c) along with enhancing physiological factors (Trappe et al., 2000a; Neary et al., 2003; Coutts et al., 2007; Murach et al., 2014; Zaras et al., 2014; Bazyler et al., 2018; Travis et al., 2020b). A systematic review by Travis et al. (2020c) and an experimental study by Seppänen and Häkkinen (2020) provide evidence to suggest a ∼50% volume-load reduction may be ideal to enhance or maintain maximal strength. However, volume-load reductions typically follow a normal training period or a planned overreach microcycle, both of which may influence the magnitude of performance improvement. To taper effectively, the current evidence supports using a planned overreach period prior to tapering. However, this assumes a sufficient stimulus and recovery period is provided to elicit a super-compensation effect (Aubry et al., 2014; Seppänen and Häkkinen, 2020; Travis et al., 2020b; Williams et al., 2020). Training programs implementing a 100–200% increase in volume-load followed by a taper have been shown to improve competition and laboratory based performances (i.e., bench press, snatch, clean-and-jerk, and jump height), and biochemical markers of training stress (i.e., cortisol, creatine kinase) in weightlifters, powerlifters, and track and field throwers (Warren et al., 1992; Fry et al., 1993; Stone and Fry, 1998; Williams et al., 2020). Similarly, the current results indicate 1RM strength improvements can be achieved following a 1-week planned overreach with a 3-week exponential taper or a 1-week planned overreach with a 1-week step taper in strength athletes.
While 1RMs for back squat (step: 8%Δ vs. expo: 10%Δ) and bench press (step: 10%Δ vs. expo: 9%Δ) improved similarly in both groups, 1RM deadlift performance favored the 3-week exponential taper (8%Δ) compared to the step taper group (1%Δ). Prior exposures to intensified training, such as overreaching microcycles, seem to reduce the likelihood of performance decrements in experienced athletes possibly due to a repeated bout effect (Stone and Fry, 1998; Pistilli et al., 2008). Nonetheless, it is possible the 1-week step taper did not provide sufficient recovery time for some athletes following the planned overreach week, particularly for deadlift. Interestingly, back squat, bench press, and deadlift exhibit similar recovery patterns in strength-trained males following four sets of each lift performed to failure using 80% 1RM (Belcher et al., 2019), and eight sets of two repetitions of back squat and deadlift performed at 95%1RM (Barnes et al., 2019). Nonetheless, these studies did not examine the cumulative effects of repeated training sessions on deadlift performance. Further, anecdotal reports from powerlifters (Pritchard et al., 2016; Grgic and Mikulic, 2017) and strongman competitors (Winwood et al., 2018) claim the deadlift requires a longer recovery period between final training and competition compared to the other lifts. Thus, a 3-week exponential taper may be warranted following a planned overreach to facilitate recovery-adaptation and enhance performance concurrently across each power lift.
Furthermore, SJ PPa (step: 4%Δ vs. expo: 9%Δ) was the only laboratory-based performance measure that increased following the 6-week peaking phase, specifically following the exponential taper. Although peak power calculations are heavily influenced by body mass (Cormie et al., 2007), when allometrically scaled for body mass, SJ PPa still improved following the exponential taper. This finding likely reflects an improved ability to generate high ground reaction forces relative to the athlete’s body mass. This is reflected by strong relationships observed between 1RM back squat scaled for body mass and jumping performance (Jacobson, 2015). Thus, it is likely that improvements in lower body maximal strength contributed to the increased SJ PPa following the exponential taper. Interestingly, improvements in SJH and SJ PPa have been repeatedly observed in weightlifters following an overreach and 3-week exponential taper (Bazyler et al., 2018; Travis et al., 2018, 2020b). Nonetheless, this study did not observe increases in SJH following either taper model. This discrepancy may be attributed to the different training programs employed by powerlifters compared to weightlifters, particularly in the current study and prior studies with weightlifters from our laboratory. Notably, weightlifters commonly train with movements (e.g., snatch, clean and jerk, clean pulls, and mid-thigh pulls) emphasizing “triple extension” of the hips, knees, and ankle joints, which exhibit a high degree of task specificity to jumping. Additionally, it is also possible the 3-week exponential taper provided greater recovery time to enhance jumping ability following the overreach compared to the 1-week step taper. Nonetheless, it appears the “transfer of training effect” from the powerlifting-oriented training in the current study to SJ performance is smaller than that observed previously in weightlifters during a taper (Bazyler et al., 2018; Travis et al., 2020b).
Despite improvements in 1RM squat, there were no improvements in ISQ IPF following either taper. A strong relationship (r = 0.84) has been observed between 1RM back squat and ISQ IPF at a 90° knee angle (Bazyler et al., 2015). ISQ IPF has also been shown to improve concurrently with 1RM back squat following 7 weeks of back squat training in strength-trained males (Bazyler et al., 2014). These discrepancies may be explained by the higher initial relative strength levels of participants in the current study (squat to body mass ratio: 1.94 ± 0.34) compared to the aforementioned study (1.73 ± 0.19). It is well established that changes in maximal strength following strength training are inversely related to initial relative strength levels (Ahtiainen et al., 2003; Ishida et al., 2020, 2021). Thus, given the higher degree of task specificity of back squat training to 1RM back squat compared to isometric squats, it is possible 1RMs provide a more sensitive measure to assess changes in maximal strength, particularly for subjects with greater initial relative strength. Nonetheless, differences in training program design, selection and order of testing procedures could also explain the differences in outcomes for ISQ IPF between studies.
There is a direct relationship between increases in mCSA and the ability to produce force (Häkkinen et al., 1991; Gabriel et al., 2006; Schoenfeld et al., 2014; Enoka and Duchateau, 2017; Seppänen and Häkkinen, 2020). Muscular adaptations are highly influenced by the prescription of training variables such as volume-load and training intensity (Schoenfeld et al., 2014; Bjørnsen et al., 2019a; Haun et al., 2019; Travis et al., 2020a). In the current study, it appears that training volume-load prescription was sufficient to elicit hypertrophic adaptations at the whole muscle level in conjunction with 1RM strength improvements. Schoenfeld et al. (2014) demonstrated similar improvements in biceps brachii muscle thickness in strength-trained males following equated volumes of bodybuilding-styled and powerlifting-styled training over 8 weeks; however, improvements in maximal strength favored the powerlifting-styled training. Similarly, meta-analytic results from Schoenfeld et al. (2017) demonstrate improvements in maximal strength are best achieved with high loads (>60% 1RM) compared to low loads (≤60% 1RM) while muscle hypertrophy can be achieved across a broad spectrum of loads. Thus, our results for 1RM and whole muscle size changes are consistent with previous studies when volume-loads are equated between training programs, and heavy loads are used in training. However, studies with weightlifters, throwers, and strength-trained individuals have reported no changes or small decreases in vastus lateralis mCSA following a taper (Häkkinen et al., 1991; Zaras et al., 2014, 2016; Bazyler et al., 2017, 2018; Suarez et al., 2019; Seppänen and Häkkinen, 2020; Travis et al., 2020b). Nonetheless, these studies have typically implemented either: (a) smaller increases in volume-load during the overreach (<150%) or (b) normal training followed by a 1–4 week taper consisting of larger reductions (≥50%) in volume-load. Despite matched reductions in volume-load (−50%) during the final week of the study, in the current study, mCSA increases were relatively larger across all measurement sites (proximal: 1.8% vs. 1.0%, middle: 3.2% vs. 1.4%, distal: 2.2% vs. 0.95%, and average: 2.4% vs. 1.1%) in the step taper compared to the exponential taper group. Changes in fCSAavg (8.6% vs. 1.7%) and MHC-IIA fCSA (11.0% vs. 0.33%) were even more pronounced favoring the step taper group. These results may be due to the timing of the overreach relative to post-training testing. Specifically, the overreach may have provided a greater hypertrophic stimulus closer to post-training testing in the step-taper (2 weeks prior) compared to the exponential taper (4 weeks prior). Indeed, previous studies observing decreases in vastus lateralis mCSA have attributed the decreases in muscle size to the prolonged reduction in volume-load during 3-week exponential tapers. Nonetheless, there was still a small, significant increase in mCSAavg following the exponential taper, which may partly be due to the larger overreach (+150%) implemented in the current study compared to previous studies (+20 to 40%) (Thomas and Busso, 2005; Le Meur et al., 2013; Aubry et al., 2014; Bazyler et al., 2018; Travis et al., 2020b). Thus, these results suggest that a 1-week planned overreach followed by a 3-week exponential taper or a 1-week step taper produces significant improvements in strength athletes’ vastus lateralis whole muscle size provided the overreaching stimulus is sufficient leading into the taper.
It is well established that resistance training produces increases in skeletal muscle fCSA (Haun et al., 2019). Haun et al. (2019) recently investigated the mechanisms associated with fCSA hypertrophy after 6 weeks of high-volume training. In brief, myosin and actin content decreased despite enhanced fCSA, yet an accretion of sarcoplasmic proteins appeared to explain the observed changes. The authors purported that observed hypertrophy was attributed to sarcoplasmic hypertrophic adaptations as a result of high training volume. In our study, it is unlikely the observed fCSA hypertrophy was due to sarcoplasmic changes considering the training protocols aimed to reduce volume-load. Decades ago, Stone et al. (1983) suggested that it is possible to produce sarcoplasmic expansion and metabolic conditioning via higher-volume/lower-load training preceding lower-volume/higher-load training that will, in turn, produce more favorable strength outcomes. In agreement, Haun et al. (2019) suggest training with higher loads can proportionally increase myofibrillar protein levels and fCSA, which would, in turn, enhance ultrastructural hypertrophy leading to increases in maximal strength. Although fCSA constituents were not measured in the current study, it is plausible that increases in myofibrillar protein levels could have contributed to the observed increases in fCSA. Future investigations should examine the constituents of muscle fiber size changes following a taper in strength athletes.
Skeletal muscle is a highly plastic tissue that shows a remarkable ability to adapt to imposed demands (Schoenfeld et al., 2014), particularly at the muscle fiber level. Previous work from 1976 to 2005 (Prince et al., 1976; MacDougall et al., 1982; Tesch et al., 1984; Kadi et al., 1999; Fry et al., 2003; Eriksson et al., 2005) and two recent studies (Bjørnsen et al., 2019a; Machek et al., 2020) have characterized the fiber types of powerlifters. Powerlifters typically demonstrate the highest expression of MHC-IIA isoforms followed by MHC-I isoforms to a lesser degree, and depending on training status, potentially little to no MHC-IIX isoforms. At baseline, our data from biopsy tissue IHC analyses and isolated single fiber analyses agree with the current literature (Prince et al., 1976; MacDougall et al., 1982; Tesch et al., 1984; Kadi et al., 1999; Fry et al., 2003; Eriksson et al., 2005; Bjørnsen et al., 2019a; Machek et al., 2020). However, the isolated single fiber analyses appeared to produce a higher sensitivity yield for accurate quantitation of pure phenotype expression along with accurately identifying hybrid MHC-I/IIA expression. A recent study by Serrano et al. (2019) using both techniques, characterized the muscle fiber types of elite weightlifters and demonstrated that the single fiber method confidently identified the hybrid isoforms, whereas homogenate analyses did not. Thus, to accurately quantify fiber type at baseline and changes across both tapering and peaking protocols, the isolated single muscle fiber analysis was used in our study. Our single fiber dot blotting technique objectively identified MHC shifts toward MHC-IIA (i.e., MHC-I → MHC-I/IIA → MHC-IIA ← MHC-IIA/IIX ← MHC-IIX) from T1 to T2. The MHC shift observed in this study reflects a fiber type transition taking place in as little as 6 weeks, moving toward a preferential fiber type as a result of an effective peaking program. This is a novel finding considering no other published work has demonstrated this phenomenon with powerlifters peaking for competition. Acute training activates a distinct MHC-IIA transcriptome that results in a training-induced increase in MHC-IIA fCSA at the single fiber level (Murach et al., 2014). Murach et al. (2014) indicate an increase in MHC-IIA single fiber fCSA can augment the capacity of MHC-IIA fibers to quickly grow and improve contractile function in the lateral gastrocnemius of distance runners during a taper. Thus, the observed increases in MHC-IIA fCSA, at the whole muscle fiber level, and MHC-IIASF% may explain the performance improvements observed, particularly following the step taper.
At the myocellular level, mRNA up- and down-regulations drive gene expression and miR abundance that can produce observable MHC isoform adaptations and growth beginning with single fibers. One of the most important gene-miR interactions is the up-regulation of SOX6 (which approached significance in the current study; p = 0.053), and the significant down-regulation of miR-499a. The post-transcriptional mechanisms between the SOX6 and miR-499a interaction are directly related to fiber type regulation, which have been confirmed by McCarthy (2011) and Bjørnsen et al. (2019a) The interaction observed between SOX6 and miR-499a as a result of the training stimulus provided in this study also confirms our single fiber MHC fiber typing quantitation (i.e., post-taper shift toward MHC-IIASF). Furthermore, the transcriptional repressor SOX6 and miR-499a have been shown to regulate muscle mass, and in part directly influence MSTN. Interestingly, our results showed an up-regulation of MSTN, which approached significance (p = 0.053) while other myogenic factors MyoD and MyoG were significantly down-regulated. It is important to note that increased muscle mass can occur regardless of MSTN expression levels (Bjørnsen et al., 2019b). Paradoxically, MSTN mRNA expression is greater in larger muscle fibers (Carlson et al., 1999). Thus, increases in MSTN expression may correspond to the fCSA increases observed in the present study. Additionally, myogenic markers have been shown to play a significant role in MHC composition (Mozdziak et al., 1998). In mature muscle, MyoD and MyoG typically possess low expression levels (Mozdziak et al., 1998). However, acute bouts of resistance training can significantly increase expression of MyoD and MyoG mRNA corresponding to increases in MHC-I, -IIA, and -IIX mRNA expression (Willoughby and Nelson, 2002). Nonetheless, decreases in resting MyoD and MyoG expression following the taper may reflect a molecular adaptation of a muscle that already achieved full recovery prior to the T2 biopsy. Despite the limited molecular changes following the taper, our data aligns with other reports on gene expression and miRNA abundance changes in resting conditions in powerlifters (D’Souza et al., 2017; Bjørnsen et al., 2019a). However, these results should be interpreted with caution considering molecular measurements are transient, and these data may not fully reflect the myocellular response immediately post-taper. Recent findings by Vann et al. (2021) demonstrate the transient nature of molecular assessments even with biopsy measurements taking place 24 h post-intervention.
There are a few limitations that should be considered when interpreting the results of this study. First, it is unknown whether edema contributed to mCSA changes measured via ultrasonography following the taper. Nevertheless, ultrasound images were collected at least 72 h following 1RM testing at both testing time points. While we could have implemented additional measurements such as echo intensity in an attempt to identify muscle swelling, the validity of such measurement is questionable (Yitzchaki et al., 2019). Second, we did not account for sarcoplasmic myofibrillar protein content, which could have differentially influenced the fCSA measurements. Additionally, we did not control for total caloric or macronutrient intake (e.g., protein and carbohydrate consumption) throughout the study, which could have influenced our molecular muscle measurements, particularly at the gene and miR levels (Roy and Tarnopolsky, 1998; Machek et al., 2020). Nonetheless, dietary intake was standardized in the 48 h prior to both muscle biopsy time points. Also, it was not possible to standardize subjects’ training prior to the 6-week peaking phase; however, all subjects consistently trained for powerlifting over the year leading up to the study. Lastly, it is important to consider the muscle tissue analyses only reflected a specific snapshot in time from when the tissue was extracted. Specifically, after the taper, athletes rested for 2 days before 1RM assessments followed by 3 days of rest before muscle biopsies. Therefore, it is possible that our muscle tissue results are more reflective of a “tapered post-competition” rested state. Nevertheless, future investigators may repeat our study design and replace or precede 1RM assessments with a muscle biopsy to assess the skeletal muscle environment in a peaked state.
Conclusion
Overall, this study provides novel evidence toward an enhanced neuromuscular profile following tapering in strength athletes. Increases in powerlifting performance following the step and exponential tapers appeared to be mediated by whole muscle, single muscle fiber, and myocellular adaptations. Specifically, increases in mCSA, fCSA, and MHC-IIA fCSA favored the step taper. Increases in MHC-IIA content with concomitant decreases in MHC-I and -IIX content were also observed following the step taper. These myosin isoform shifts toward the MHC-IIA phenotype appear to be related to changes in underlying myocellular signaling (i.e., Sox6 up-regulation and miRNA-499a down-regulation) responsible for fiber-type transitions. Thus, planning an overreach close to competition, followed by a short, step taper may support a more favorable environment to induce fast-twitch fiber adaptations compared to an overreach planned further from competition followed by an exponential taper. Nonetheless, it is possible that the 1-week step taper did not provide sufficient recovery time for some athletes following the overreach, particularly for deadlift and squat jump performance. This study also provides direct evidence for short-term skeletal muscle plasticity at all measurable levels, and subsequent potentiating effects on maximal strength performance following a taper in strength athletes. Based on these findings, we recommended strength athletes use a 1-week overreach where volume-load is increased by ≥150% followed by a step or an exponential taper where training volume-load is reduced by ∼50% over a duration of 1–3 weeks to promote a myocellular environment favorable to fast-twitch skeletal muscle adaptations and to enhance maximal strength.
Data Availability Statement
The original contributions presented in the study are included in the article/Supplementary Material, further inquiries can be directed to the corresponding author/s.
Ethics Statement
This study involving human participants was reviewed and approved by East Tennessee State University Institutional Review Board. The participants provided their written informed consent to participate in this study.
Author Contributions
SKT and KZ performed the experiments and designed all primers. SKT, KZ, and CB analyzed the data. SKT, IM, and CB drafted the manuscript. All authors critically evaluated and contributed to the manuscript.
Funding
This study was funded by the National Strength and Conditioning Association per the Graduate Student Research Grant for Doctoral Students (#20-162) and per the Young Investigator Research Grant (#20-161). Additional funding was obtained from the East Tennessee State University Research Development Committee (#21-010M).
Conflict of Interest
The authors declare that the research was conducted in the absence of any commercial or financial relationships that could be construed as a potential conflict of interest.
Publisher’s Note
All claims expressed in this article are solely those of the authors and do not necessarily represent those of their affiliated organizations, or those of the publisher, the editors and the reviewers. Any product that may be evaluated in this article, or claim that may be made by its manufacturer, is not guaranteed or endorsed by the publisher.
Supplementary Material
The Supplementary Material for this article can be found online at: https://www.frontiersin.org/articles/10.3389/fphys.2021.735932/full#supplementary-material
References
Abe, T., Buckner, S. L., Dankel, S. J., Jessee, M. B., Mattocks, K. T., Mouser, J. G., et al. (2018a). Skeletal muscle mass in human athletes: what is the upper limit? Am. J. Hum. Biol. 30:e23102. doi: 10.1002/ajhb.23102
Abe, T., Buckner, S. L., Mattocks, K. T., Jessee, M. B., Dankel, S. J., Mouser, J. G., et al. (2018b). Skeletal muscle mass and architecture of the World’s strongest raw powerlifter: a case study. Asian J. Sports Med. 9:e61763 doi: 10.5812/asjsm.61763
Ahtiainen, J. P., Pakarinen, A., Alen, M., Kraemer, W. J., and Häkkinen, K. (2003). Muscle hypertrophy, hormonal adaptations and strength development during strength training in strength-trained and untrained men. Eur. J. Appl. Physiol. 89, 555–563. doi: 10.1007/s00421-003-0833-3
Aubry, A., Hausswith, C., Louis, J., Coutts, A. J., and Meur, L. E. Y. (2014). Functional overreaching: the key to peak perfomrance during the taper? Funct. Overreaching Key Peak Perform. Taper 46, 1769–1777.
Barnes, M. J., Miller, A., Reeve, D., and Stewart, R. J. C. (2019). Acute neuromuscular and endocrine responses to two different compound exercises: squat vs. deadlift. J. Strength Cond. Res. 33, 2381–2387. doi: 10.1519/JSC.0000000000002140
Bazyler, C. D., Beckham, G. K., and Sato, K. (2015). The use of the isometric squat as a measure of strength and explosiveness. J. Strength Cond. Res. 29, 1386–1392. doi: 10.1519/JSC.0000000000000751
Bazyler, C. D., Mizuguchi, S., Harrison, A. P., Sato, K., Kavanaugh, A. A., DeWeese, B. H., et al. (2017). Changes in muscle architecture, explosive ability, and track and field throwing performance throughout a competitive season and after a taper. J. Strength Cond. Res. 31, 2785–2793. doi: 10.1519/JSC.0000000000001619
Bazyler, C. D., Mizuguchi, S., Zourdos, M. C., Sato, K., Kavanaugh, A. A., DeWeese, B. H., et al. (2018). Characteristics of a National level female weightlifter peaking for competition: a case study. J. Strength Cond. Res. 32, 3029–3038. doi: 10.1519/JSC.0000000000002379
Bazyler, C. D., Sato, K., Wassinger, C. A., Lamont, H. S., and Stone, M. H. (2014). The efficacy of incorporating partial squats in maximal strength training. J. Strength Cond. Res. 28, 3024–3032. doi: 10.1519/JSC.0000000000000465
Belcher, D. J., Sousa, C. A., Carzoli, J. P., Johnson, T. K., Helms, E., Visavadiya, N. P., et al. (2019). Time course of recovery is similar for the back squat, bench press, and deadlift in well-trained males. Appl. Physiol. Nutr. Metab. 44, 1033–1042 doi: 10.1139/apnm-2019-0004
Bjørnsen, T., Wernbom, M., Kirketeig, A., Paulsen, G., Samnøy, L., Bækken, L., et al. (2019a). Type 1 muscle fiber hypertrophy after blood flow-restricted training in powerlifters. Med. Sci. Sports Exerc. 51, 288–298. doi: 10.1249/MSS.0000000000001775
Bjørnsen, T., Wernbom, M., Løvstad, A., Paulsen, G., D’Souza, R. F., Cameron-Smith, D., et al. (2019b). Delayed myonuclear addition, myofiber hypertrophy, and increases in strength with high-frequency low-load blood flow restricted training to volitional failure. J. Appl. Physiol. (1985) 126, 578–592. doi: 10.1152/japplphysiol.00397.2018
Bosy-Westphal, A., Jensen, B., Braun, W., Pourhassan, M., Gallagher, D., and Müller, M. J. (2017). Quantification of whole-body and segmental skeletal muscle mass using phase-sensitive 8-electrode medical bioelectrical impedance devices. Eur. J. Clin. Nutr. 71, 1061–1067. doi: 10.1038/ejcn.2017.27
Bosy-Westphal, A., Schautz, B., Later, W., Kehayias, J. J., Gallagher, D., and Müller, M. J. (2013). What makes a BIA equation unique? Validity of eight-electrode multifrequency BIA to estimate body composition in a healthy adult population. Eur. J. Clin. Nutr. 67, S14–S21. doi: 10.1038/ejcn.2012.160
Brechue, W. F., and Abe, T. (2002). The role of FFM accumulation and skeletal muscle architecture in powerlifting performance. Eur. J. Appl. Physiol. 86, 327–336.
Carlson, C. J., Booth, F. W., and Gordon, S. E. (1999). Skeletal muscle myostatin mRNA expression is fiber-type specific and increases during hindlimb unloading. Am. J. Physiol. 277, R601–R606. doi: 10.1152/ajpregu.1999.277.2.r601
Christiansen, D., MacInnis, M. J., Zacharewicz, E., Xu, H., Frankish, B. P., and Murphy, R. M. (2019). A fast, reliable and sample-sparing method to identify fibre types of single muscle fibres. Sci. Rep. 9:6473. doi: 10.1038/s41598-019-42168-z
Cormie, P., McBride, J. M., and McCaulley, G. O. (2007). The influence of body mass on calculation of power during lower-body resistance exercises. J. Strength Cond. Res. 21, 1042–1049. doi: 10.1519/R-21636.1
Coutts, A., Reaburn, P., Piva, T. J., and Murphy, A. (2007). Changes in selected biochemical, muscular strength, power, and endurance measures during deliberate overreaching and tapering in rugby league players. Int. J. Sports Med. 28, 116–124. doi: 10.1055/s-2006-924145
D’Souza, R. F., Bjørnsen, T., Zeng, N., Aasen, K. M. M., Raastad, T., Cameron-Smith, D., et al. (2017). MicroRNAs in muscle: characterizing the powerlifter phenotype. Front. Physiol. 8:383. doi: 10.3389/fphys.2017.00383
D’Souza, R. F., Zeng, N., Markworth, J. F., Figueiredo, V. C., Roberts, L. A., Raastad, T., et al. (2018). Divergent effects of cold water immersion versus active recovery on skeletal muscle fiber type and angiogenesis in young men. Am. J. Physiol. Regul. Integr. Comp. Physiol. 314, R824–R833. doi: 10.1152/ajpregu.00421.2017
D’Souza, R. F., Zeng, N., Poppitt, S. D., Cameron-Smith, D., and Mitchell, C. J. (2019). Circulatory microRNAs are not effective biomarkers of muscle size and function in middle-aged men. Am. J. Physiol. Cell Physiol. 316, C293–C298. doi: 10.1152/ajpcell.00395.2018
Eisenberg, E., and Levanon, E. Y. (2013). Human housekeeping genes, revisited. Trends Genet. 29, 569–574. doi: 10.1016/j.tig.2013.05.010
Enoka, R. M., and Duchateau, J. (2017). Rate coding and the control of muscle force. Cold Spring Harb. Perspect. Med. 7:a029702 doi: 10.1101/cshperspect.a029702
Eriksson, A., Kadi, F., Malm, C., and Thornell, L.-E. (2005). Skeletal muscle morphology in power-lifters with and without anabolic steroids. Histochem. Cell Biol. 124, 167–175. doi: 10.1007/s00418-005-0029-5
Foster, C., Florhaug, J. A., Franklin, J., et al. (2001). A new approach to monitoring exercise training. J. Strength Cond. Res. 15, 109–115.
Fry, A. C., Kraemer, W. J., Stone, M. H., Warren, B. J., Kearney, J. T., Maresh, C. M., et al. (1993). endocrine and performance responses to high volume training and amino acid supplementation in elite junior weightlifters. Int. J. Sport Nutr. 3, 306–322. doi: 10.1123/ijsn.3.3.306
Fry, A. C., Webber, J. M., Weiss, L. W., Harber, M. P., Vaczi, M., and Pattison, N. A. (2003). Muscle fiber characteristics of competitive power lifters. J. Strength Cond. Res. 17, 402–410.
Gabriel, D. A., Kamen, G., and Frost, G. (2006). Neural adaptations to resistive exercise: mechanisms and recommendations for training practices. Sports Med. 36, 133–149. doi: 10.2165/00007256-200636020-00004
Goldspink, G. (2002). Gene expression in skeletal muscle. Biochem. Soc. Trans. 30, 285–290. doi: 10.1042/bst0300285
Grgic, J., and Mikulic, P. (2017). Tapering practices of croatian open-class powerlifting champions. J. Strength Cond. Res. 31, 2371-2378. doi: 10.1519/JSC.0000000000001699
Häkkinen, K., Kallinen, M., Komi, P. V., and Kauhanen, H. (1991). Neuromuscular adaptations during short-term “normal” and reduced training periods in strength athletes. Electromyogr. Clin. Neurophysiol. 31, 35–42.
Haun, C. T., Vann, C. G., Osburn, S. C., Mumford, P. W., Roberson, P. A., Romero, M. A., et al. (2019). Muscle fiber hypertrophy in response to 6 weeks of high-volume resistance training in trained young men is largely attributed to sarcoplasmic hypertrophy. BioRxiv [Preprint]. doi: 10.1101/596049
Hedges, L. V. (1980). Combining the Results of Experiments Using Different Scales of Measurement. Available online at: https://www.scholars.northwestern.edu/en/publications/combining-the-results-of-experiments-using-different-scales-of-me (accessed March 18, 2021).
Ishida, A., Rochau, K., Findlay, K. P., Devero, B., Duca, M., and Stone, M. H. (2020). Effects of an initial muscle strength level on sports performance changes in collegiate soccer players. Sports (Basel) 8:127 doi: 10.3390/sports8090127
Ishida, A., Travis, S. K., and Stone, M. H. (2021). Short-term periodized programming may improve strength, power, jump kinetics, and sprint efficiency in soccer. J. Funct. Morphol. Kinesiol. 6:45. doi: 10.3390/jfmk6020045
Jacobson, B. H. (2015). Comparison of allometric scaling methods for normalizing strength, power, and speeed in American football players. J. Sports Med. Phys. Fitness 55, 684–690.
Jensen, B., Braun, W., Geisler, C., Both, M., Klückmann, K., Müller, M. J., et al. (2019). Limitations of fat-free mass for the assessment of muscle mass in obesity. Obes. Facts 12, 307–315. doi: 10.1159/000499607
Kadi, F., Eriksson, A., Holmner, S., Butler-Browne, G. S., and Thornell, L.-E. (1999). Cellular adaptation of the trapezius muscle in strength-trained athletes. Histochem. Cell Biol. 111, 189–195. doi: 10.1007/s004180050348
Lamboley, C. R., Rouffet, D. M., Dutka, T. L., McKenna, M. J., and Lamb, G. D. (2020). Effects of high-intensity intermittent exercise on the contractile properties of human type I and type II skeletal muscle fibers. J. Appl. Physiol. 128, 1207–1216. doi: 10.1152/japplphysiol.00014.2020
Lawrence, M. M., Zwetsloot, K. A., Arthur, S. T., Sherman, C. A., Huot, J. R., Badmaev, V., et al. (2021). Phytoecdysteroids do not have anabolic effects in skeletal muscle in sedentary aging mice. Int. J. Environ. Res. Public Health 18:370. doi: 10.3390/ijerph18020370
Le Meur, Y., Pichon, A., Schaal, K., Schmitt, L., Louis, J., Gueneron, J., et al. (2013). Evidence of parasympathetic hyperactivity in functionally overreached athletes. Med. Sci. Sports Exerc. 45, 2061–2071. doi: 10.1249/MSS.0b013e3182980125
Livak, K. J., and Schmittgen, T. D. (2001). Analysis of relative gene expression data using real-time quantitative PCR and the 2(-Delta Delta C(T)) Method. Methods 25, 402–408. doi: 10.1006/meth.2001.1262
Luden, N., Hayes, E., Galpin, A., Minchev, K., Jemiolo, B., Raue, U., et al. (2010). Myocellular basis for tapering in competitive distance runners. J. Appl. Physiol. 108, 1501–1509. doi: 10.1152/japplphysiol.00045.2010
MacDougall, J. D., Sale, D. G., Elder, G. C. B., and Sutton, J. R. (1982). Muscle ultrastructural characteristics of elite powerlifters and bodybuilders. Eur. J. Appl. Physiol. 48, 117–126. doi: 10.1007/BF00421171
Machek, S. B., Hwang, P. S., Cardaci, T. D., Wilburn, D. T., Bagley, J. R., Blake, D. T., et al. (2020). Myosin heavy chain composition, creatine analogues, and the relationship of muscle creatine content and fast-twitch proportion to wilks coefficient in powerlifters. J. Strength Cond. Res. 34, 3022–3030. doi: 10.1519/JSC.0000000000003804
McCarthy, J. J. (2011). The myomir network in skeletal muscle plasticity. Exerc. Sport Sci. Rev. 39, 150–154. doi: 10.1097/JES.0b013e31821c01e1
McDermott, J. C., and Bonen, A. (1991). The regulation of myosin gene transcription in skeletal muscle: effects of altered functional demand. Can. J. Sport Sci. 16, 210–222.
Mozdziak, P. E., Greaser, M. L., and Schultz, E. (1998). Myogenin, MyoD, and myosin expression after pharmacologically and surgically induced hypertrophy. J. Appl. Physiol. 84, 1359–1364. doi: 10.1152/jappl.1998.84.4.1359
Mujika, I., and Padilla, S. (2003). Scientific bases for precompetition tapering strategies. Med. Sci. Sports Exerc. 35, 1182–1187. doi: 10.1249/01.MSS.0000074448.73931.11
Mujika, I., Padilla, S., and Pyne, D. (2002). Swimming performance changes during the final 3 weeks of training leading to the Sydney 2000 Olympic Games. Int. J. Sports Med. 23, 582–587. doi: 10.1055/s-2002-35526
Murach, K., Raue, U., Wilkerson, B., Minchev, K., Jemiolo, B., Bagley, J., et al. (2014). Single muscle fiber gene expression with run taper. PLoS One 9:e108547. doi: 10.1371/journal.pone.0108547
Murach, K. A., Bagley, J. R., McLeland, K. A., Arevalo, J. A., Ciccone, A. B., Malyszek, K. K., et al. (2016). Improving human skeletal muscle myosin heavy chain fiber typing efficiency. J. Muscle Res. Cell Motil. 37, 1–5. doi: 10.1007/s10974-016-9441-9
Neary, J. P., Martin, T. P., and Quinney, H. A. (2003). Effects of taper on endurance cycling capacity and single muscle fiber properties. Med. Sci. Sports Exerc. 35, 1875–1881. doi: 10.1249/01.MSS.0000093617.28237.20
Peine, S., Knabe, S., Carrero, I., Brundert, M., Wilhelm, J., Ewert, A., et al. (2013). Generation of normal ranges for measures of body composition in adults based on bioelectrical impedance analysis using the seca mBCA. Int. J. Body Compos. Res. 11:67.
Pistilli, E. E., Kaminsky, D. E., Totten, L. M., and Miller, D. R. (2008). Incorporating one week of planned overreaching into the training program of weightlifters. Strength Cond. J. 30, 39-44 doi: 10.1519/SSC.0b013e31818ee78c
Prince, F. P., Hikida, R. S., and Hagerman, F. C. (1976). Human muscle fiber types in power lifters, distance runners and untrained subjects. Pflugers Arch. 363, 19–26. doi: 10.1007/BF00587397
Pritchard, H., Keogh, J., Barnes, M., and McGuigan, M. (2015). Effects and mechanisms of tapering in maximizing muscular strength. Strength Cond. J. 37, 72-83 doi: 10.1519/SSC.0000000000000125
Pritchard, H. J., Barnes, M. J., Stewart, R. J., Keogh, J. W., and McGuigan, M. R. (2019). Higher- versus lower-intensity strength-training taper: effects on neuromuscular performance. Int. J. Sports Physiol. Perform. 14, 458–463. doi: 10.1123/ijspp.2018-0489
Pritchard, H. J., Keogh, J. W., and Winwood, P. W. (2020). Tapering practices of elite CrossFit athletes. Int. J. Sports Sci. Coach.15, 753-761. doi: 10.1177/1747954120934924
Pritchard, H. J., Tod, D. A., Barnes, M. J., Keogh, J. W., and McGuigan, M. R. (2016). Tapering practices of New Zealand’s elite raw powerlifters. J. Strength Cond. Res. 30, 1796–1804. doi: 10.1519/JSC.0000000000001292
Pyne, D. B., Gleeson, M., McDonald, W. A., Clancy, R. L. C. P. Jr., and Fricker, P. A. (2000). Training strategies to maintain immunocompetence in athletes. Int. J. Sports Med. 21, 51–60. doi: 10.1055/s-2000-1452
Roy, B. D., and Tarnopolsky, M. A. (1998). Influence of differing macronutrient intakes on muscle glycogen resynthesis after resistance exercise. J. Appl. Physiol. 84, 890–896. doi: 10.1152/jappl.1998.84.3.890
Schindelin, J., Rueden, C. T., Hiner, M. C., and Eliceiri, K. W. (2015). The imagej ecosystem: an open platform for biomedical image analysis. Mol. Reprod. Dev. 82, 518–529. doi: 10.1002/mrd.22489
Schmittgen, T. D., and Livak, K. J. (2008). Analyzing real-time PCR data by the comparative C(T) method. Nat. Protoc. 3, 1101–1108. doi: 10.1038/nprot.2008.73
Schoenfeld, B. J., Grgic, J., Ogborn, D., and Krieger, J. W. (2017). Strength and hypertrophy adaptations between low- vs. high-load resistance training: a systematic review and meta-analysis. J. Strength Cond. Res. 31, 3508–3523. doi: 10.1519/JSC.0000000000002200
Schoenfeld, B. J., Ratamess, N. A., Peterson, M. D., Contreras, B., Sonmez, G. T., and Alvar, B. A. (2014). Effects of different volume-equated resistance training loading strategies on muscular adaptations in well-trained men. J. Strength Cond. Res. 28, 2909–2918. doi: 10.1519/JSC.0000000000000480
Seppänen, S., and Häkkinen, K. (2020). Step vs. two-phase gradual volume reduction tapering protocols in strength training: effects on neuromuscular performance and serum hormone concentrations. J. Strength Cond. Res. doi: 10.1519/JSC.0000000000003939 [Epub ahead of print]
Serrano, N., Colenso-Semple, L. M., Lazauskus, K. K., Siu, J. W., Bagley, J. R., Lockie, R. G., et al. (2019). Extraordinary fast-twitch fiber abundance in elite weightlifters. PLoS One 14:e0207975. doi: 10.1371/journal.pone.0207975
Stone, M. H., Wilson, D., Rozenek, R., and Newton, H. (1983). Anaerobic capacity: physiological basis. Strength Cond. J. 5, 40–40.
Stone, M., and Fry, A. (1998). “Increased training volume in strength/power athletes,” in Overtraining in Sport, eds R. B. Kreider, A C. Fry, and M. L. O’Toole (Champaign: Human Kinetics), 87–105.
Suarez, D. G., Mizuguchi, S., Hornsby, W. G., Cunanan, A. J., Marsh, D. J., and Stone, M. H. (2019). Phase-specific changes in rate of force development and muscle morphology throughout a block periodized training cycle in weightlifters. Sports 7:129. doi: 10.3390/sports7060129
Tesch, P. A., Thorsson, A., and Kaiser, P. (1984). Muscle capillary supply and fiber type characteristics in weight and power lifters. J. Appl. Physiol. 56, 35–38. doi: 10.1152/jappl.1984.56.1.35
Thomas, L., and Busso, T. (2005). A theoretical study of taper characteristics to optimize performance. Med. Sci. Sports Exerc. 37, 1615–1621.
Trappe, S., Williamson, D., Godard, M., Porter, D., Rowden, G., and Costill, D. (2000b). Effect of resistance training on single muscle fiber contractile function in older men. J. Appl. Physiol. 89, 143–152. doi: 10.1152/jappl.2000.89.1.143
Trappe, S., Costill, D., and Thomas, R. (2000a). Effect of swim taper on whole muscle and single muscle fiber contractile properties. Med. Sci. Sports Exerc. 32, 48–56.
Travis, S. K., Goodin, J. R., Beckham, G. K., and Bazyler, C. D. (2018). Identifying a test to monitor weightlifting performance in competitive male and female weightlifters. Sports 6:46. doi: 10.3390/sports6020046
Travis, S. K., Mujika, I., Gentles, J. A., Stone, M. H., and Bazyler, C. D. (2020c). Tapering and peaking maximal strength for powerlifting performance: a review. Sports 8:125. doi: 10.3390/sports8090125
Travis, S. K., Mizuguchi, S., Stone, M. H., Sands, W. A., and Bazyler, C. D. (2020b). Preparing for a national weightlifting championship: a case series. J. Strength Cond. Res. 34, 1842–1850. doi: 10.1519/JSC.0000000000003312
Travis, S. K., Ishida, A., Taber, C. B., Fry, A. C., and Stone, M. H. (2020a). Emphasizing task-specific hypertrophy to enhance sequential strength and power performance. J. Funct. Morphol. Kinesiol. 5:76. doi: 10.3390/jfmk5040076
Travis, S. K., Zourdos, M. C., and Bazyler, C. D. (2020d). Weight selection attempts of elite classic powerlifters. Percept. Mot. Skills 128, 507-521. doi: 10.1177/0031512520967608
Travis, S. K., Pritchard, H. J., Mujika, I., Gentles, J. A., Stone, M. H., and Bazyler, C. D. (2021). Characterizing the tapering practices of United States and Canadian powerlifters. J. Strength Cond. Res. Epub ahead of print
USAPL and Administrators (2019). USA Powerlifting Technical Rules. Available online at: https://www.usapowerlifting.com/wp-content/uploads/2021/04/USAPL-Rulebook-v2021.1.pdf
van Rooij, E., Liu, N., and Olson, E. N. (2008). MicroRNAs flex their muscles. Trends Genet. 24, 159–166. doi: 10.1016/j.tig.2008.01.007
Vanderburgh, P. M., and Batterham, A. M. (1999). Validation of the Wilks powerlifting formula. Med. Sci. Sports Exerc. 31, 1869–1875.
Vandesompele, J., De Preter, K., Pattyn, F., Poppe, B., Van Roy, N., De Paepe, A., et al. (2002). Accurate normalization of real-time quantitative RT-PCR data by geometric averaging of multiple internal control genes. Genome Biol. 3:research0034.1. doi: 10.1186/gb-2002-3-7-research0034
Vann, C. G., Haun, C. T., Osburn, S. C., Romero, M. A., Roberson, P. A., Mumford, P. W., et al. (2021). Molecular differences in skeletal muscle after 1 week of active vs. passive recovery from high-volume resistance training. J. Strength Cond. Res. 35, 2102-2113. doi: 10.1519/JSC.0000000000004071
Warren, B. J., Stone, M. H., Kearney, J. T., Fleck, S. J., Johnson, R. L., Wilson, G. D., et al. (1992). Performance measures, blood lactate and plasma ammonia as indicators of overwork in elite junior weightlifters. Int. J. Sports Med. 13, 372–376. doi: 10.1055/s-2007-1021283
Williams, T. D., Esco, M. R., Fedewa, M. V., and Bishop, P. A. (2020). bench press load-velocity profiles and strength after overload and taper microcyles in male powerlifters. J. Strength Cond. Res. 34, 3338–3345. doi: 10.1519/JSC.0000000000003835
Willoughby, D. S., and Nelson, M. J. (2002). Myosin heavy-chain mRNA expression after a single session of heavy-resistance exercise. Med. Sci. Sports Exerc. 34, 1262–1269. doi: 10.1097/00005768-200208000-00006
Winwood, P. W., Dudson, M. K., Wilson, D., Mclaren-Harrison, J. K. H., Redjkins, V., Pritchard, H. J., et al. (2018). Tapering practices of strongman athletes. J. Strength Cond. Res. 32, 1181–1196. doi: 10.1519/JSC.0000000000002453
Yitzchaki, N., Kuehne, T. E., Mouser, J. G., and Buckner, S. L. (2019). Can changes in echo intensity be used to detect the presence of acute muscle swelling? Physiol. Meas. 40:045002. doi: 10.1088/1361-6579/ab122a
Zaras, N. D., Stasinaki, A. E., Krase, A. A., Methenitis, S. K., Karampatsos, G. P., Georgiadis, G. V., et al. (2014). Effects of tapering with light vs. heavy loads on track and field throwing performance. J. Strength Cond. Res. 28, 3484-95. doi: 10.1519/JSC.0000000000000566
Zaras, N. D., Stasinaki, A.-N. E., Methenitis, S. K., Krase, A. A., Karampatsos, G. P., Georgiadis, G. V., et al. (2016). Rate of force development, muscle architecture, and performance in young competitive track and field throwers. J. Strength Cond. Res. 30, 81–92. doi: 10.1519/JSC.0000000000001048
Keywords: powerlifting, muscle biopsy, fiber typing, gene expression, mRNA, resistance training, myosin heavy chain, maximal strength
Citation: Travis SK, Zwetsloot KA, Mujika I, Stone MH and Bazyler CD (2021) Skeletal Muscle Adaptations and Performance Outcomes Following a Step and Exponential Taper in Strength Athletes. Front. Physiol. 12:735932. doi: 10.3389/fphys.2021.735932
Received: 03 July 2021; Accepted: 21 September 2021;
Published: 21 October 2021.
Edited by:
Ferdinando Iellamo, University of Rome Tor Vergata, ItalyReviewed by:
Giuseppe D’Antona, University of Pavia, ItalyCarlo Castagna, University of Rome Tor Vergata, Italy
Copyright © 2021 Travis, Zwetsloot, Mujika, Stone and Bazyler. This is an open-access article distributed under the terms of the Creative Commons Attribution License (CC BY). The use, distribution or reproduction in other forums is permitted, provided the original author(s) and the copyright owner(s) are credited and that the original publication in this journal is cited, in accordance with accepted academic practice. No use, distribution or reproduction is permitted which does not comply with these terms.
*Correspondence: S. Kyle Travis, dHJhdmlzc2tAZXRzdS5lZHU=