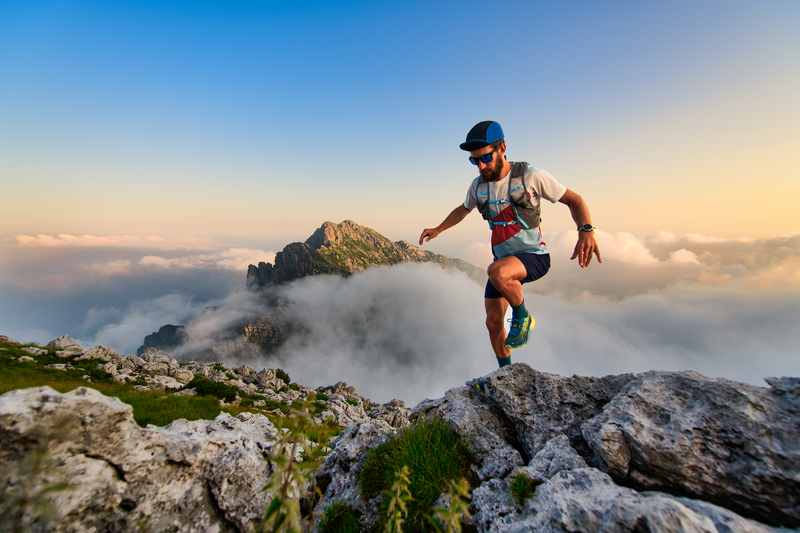
95% of researchers rate our articles as excellent or good
Learn more about the work of our research integrity team to safeguard the quality of each article we publish.
Find out more
REVIEW article
Front. Physiol. , 10 September 2021
Sec. Aquatic Physiology
Volume 12 - 2021 | https://doi.org/10.3389/fphys.2021.734463
This article is part of the Research Topic The Physiological and Molecular Response of Aquatic Animals to Environmental Stresses View all 23 articles
In the past decade, the Deepwater Horizon oil spill triggered a spike in investigatory effort on the effects of crude oil chemicals, most notably polycyclic aromatic hydrocarbons (PAHs), on marine organisms and ecosystems. Oysters, susceptible to both waterborne and sediment-bound contaminants due to their filter-feeding and sessile nature, have become of great interest among scientists as both a bioindicator and model organism for research on environmental stressors. It has been shown in many parts of the world that PAHs readily bioaccumulate in the soft tissues of oysters. Subsequent experiments have highlighted the negative effects associated with exposure to PAHs including the upregulation of antioxidant and detoxifying gene transcripts and enzyme activities such as Superoxide dismutase, Cytochrome P450 enzymes, and Glutathione S-transferase, reduction in DNA integrity, increased infection prevalence, and reduced and abnormal larval growth. Much of these effects could be attributed to either oxidative damage, or a reallocation of energy away from critical biological processes such as reproduction and calcification toward health maintenance. Additional abiotic stressors including increased temperature, reduced salinity, and reduced pH may change how the oyster responds to environmental contaminants and may compound the negative effects of PAH exposure. The negative effects of acidification and longer-term salinity changes appear to add onto that of PAH toxicity, while shorter-term salinity changes may induce mechanisms that reduce PAH exposure. Elevated temperatures, on the other hand, cause such large physiological effects on their own that additional PAH exposure either fails to cause any significant effects or that the effects have little discernable pattern. In this review, the oyster is recognized as a model organism for the study of negative anthropogenic impacts on the environment, and the effects of various environmental stressors on the oyster model are compared, while synergistic effects of these stressors to PAH exposure are considered. Lastly, the understudied effects of PAH photo-toxicity on oysters reveals drastic increases to the toxicity of PAHs via photooxidation and the formation of quinones. The consequences of the interaction between local and global environmental stressors thus provide a glimpse into the differential response to anthropogenic impacts across regions of the world.
As countries develop and human activities expand, so does the amount of waste produced and consequently, the amount of environmental pollution released. The rapid industrial growth of the 21st century has caused a demand for power satisfied primarily by petroleum that in 2020 has contributed to 34.7% of total energy consumption in the United States (United States Energy Information Administration, 2021), As a result, the United States has inadvertently become the largest source of polycyclic aromatic hydrocarbons (PAHs) in the environment either through incomplete combustion of coal, oil, petroleum, and wood (Abdel-Shafy and Mansour, 2016), or directly via oil spills such as the Deepwater Horizon spill (Reddy et al., 2012). The introduction of such chemicals to the environment does not come at no cost, and it is well-known that PAHs are toxic to humans (Abdel-Shafy and Mansour, 2016) and may constitute a possible health risk if bioaccumulated or otherwise present in food sources (Tongo et al., 2017; Rai et al., 2019; Abalaka et al., 2020).
A large body of research has taken interest in the environmental monitoring of the contaminants currently found in the environment by measuring PAH concentrations from the water column and in sediments (Yu et al., 2021). The inclusion of different media is very important to capture the distribution of contaminants because of the differential ability to accumulate contaminants. For example, the sediments at the bottom of the water column may act as a sink for contaminants since both metals and PAHs adsorb onto sediments (Jackim et al., 1977; Geffard et al., 2003), while organisms in the water column such as copepods ingest PAH-contaminated algae that later reaches the seafloor as fecal pellets (Arias et al., 2016). In fact, Guerra-García et al. (2021) considers sediment measurements to be preferable to seawater measurements in terms of characterizing the local pollutant levels due to the dynamic and variable nature of water in contrast to the more static ability of sediments to act as a storage for contaminants.
As for the organisms that live among the pollutants, chemicals enter the organisms’ bodies by diffusion, adsorption, or ingestion (Kelly et al., 2007; Arias et al., 2016; Metian et al., 2020; Shao and Wang, 2020), sometimes accumulating concentrations far exceeding that of either the water or sediments (Cortazar et al., 2008) although this is not always the case (Bustamante et al., 2012a; Hong et al., 2016; Vaezzadeh et al., 2019). Regardless, the use of bioindicators or organisms that accumulate contaminants to evaluate not only the levels but also the effects of environmental pollution is a useful method that likely provides a better representation of the anthropogenic impacts (Galloway et al., 2004). According to Zhou et al. (2008), biomonitoring reveals the integrated effects of the pollutants on the organisms by way of subtle biological changes that are not only sensitive due to the rapid onset of biological response but are also realized at contaminant levels that may be below detection limits due to chronic exposure to low doses. As a result, the bioaccumulation levels and responses in organisms such as algae, zooplankton, crustaceans, mollusks, fish, amphibians, and even marine mammals have been studied in aquatic and marine systems (Zhou et al., 2008; Cullen et al., 2019; Liu et al., 2019).
Gastropods and bivalves are two different classes of mollusks commonly used as bioindicators (O’Connor, 2002; Rittschof and McClellan-Green, 2005; Noh et al., 2018; Pham et al., 2020). Of these two, bivalves are potentially better suited for biomonitoring due to their sedentary lifestyle and filter-feeding. Oysters are especially of interest, due to their contaminant load often far exceeding their bivalve congeners (O’Connor, 2002; Leon et al., 2013; Wang and Lu, 2017; Liu et al., 2019) while displaying measurable signs of contaminant-induced stress. PAH exposure has been linked with increased antioxidant activity (Sarkar et al., 2017), altered immune system activity (Donaghy et al., 2010; Croxton et al., 2012), mutagenicity (Sarker et al., 2018), and larval abnormalities (Vignier et al., 2015) in oysters, which provide a long list of sublethal biomarkers with which to study the effects of such contaminants. Since oysters are of interest for human consumption, there are concerns over health risks due to their accumulation of toxic compounds (Hong et al., 2016; Liu et al., 2019; Wang et al., 2020), while the demand for the food source has been stimulating the growing oyster aquaculture industry (Botta et al., 2020). It is, therefore, no surprise that oysters have been extremely popular as bioindicators in recent decades. In this review, the contributions of various species of oysters (Crassostrea brasiliana, C. rhizophorae, C. tulipa (formerly C. gasar), C. virginica, Magallana (formerly Crassostrea) angulata, M. belcheri, M. bilineata (formerly C. madrasensis), M. gigas, M. hongkongensis, Ostrea edulis, O. equestris, Saccostrea cucullata, and S. glomerata) toward the bioaccumulation and toxicology of PAHs are presented.
Boening (1999) summarized that bioindicator organisms are suggested to: (1) be tolerant to the contaminant and accumulate contaminants without suffering mortality, (2) be either sedentary or have limited range of movement, (3) have a sufficient life span to allow for sampling over a broad range of time, (4) be abundant at the study site, (5) be of sufficient size for chemical analysis, (6) be hardy enough to maintain health through sampling and laboratory procedures, (7) be easy to sample and identify, (8) have high contaminant accumulation levels, and (9) be sensitive to changes in contaminant exposure. While not exhaustive, this list puts together the main concerns with the appropriateness of a bioindicator by highlighting ease of access, ability to reflect environmental change, and ease of robust scientific study. Further research efforts have added criteria for consideration as a bioindicator. (10) Bioindicators should have a low ability to metabolize contaminants (Hamzah-Chaffai, 2014) and (11) would be more useful if they had different life stages (Zhou et al., 2008). Organisms with a high ability to metabolize contaminants would in turn have a high efflux rate of any uptaken contaminants and may not accumulate detectable or appreciable levels of contaminant and lead to false-negative readings. Furthermore, Zhou et al. (2008) noted that having larva and adult stages provides the opportunity to study the effects of contaminants on multiple stages and the physiological processes of metamorphosis. As Boulais et al. (2018) demonstrated, the development and growth of the embryo stage of C. virginica were more sensitive to PAH-contaminated sediment than that of the veliger stage, and thus presents findings novel to studies on strictly adult oysters.
Oysters are palm-sized bivalve organisms, with an irregular shell, and a planktonic larval stage that metamorphoses into a sedentary adult life stage living attached to any hard substrate such as tree roots, pebbles and shells, or other oysters (National Research Council, 2004). As a result, some species such as Magallana gigas (formerly Crassostrea gigas) form vast oyster reefs that cover a wide area sufficient to transform entire benthic landscapes and habitats (Troost, 2010). The sessile nature of oysters results in the bioaccumulation of the contaminants in the immediate vicinity, while the wide-ranging reefs allow an equivalently wide sampling range that monitors the spatial distribution of point-source pollution. Due to the fact that oysters live amongst potentially contaminated sediments, and are filter-feeders that clear particles from large volumes of seawater each day (National Research Council, 2004), oysters are likely exposed to many times more contaminants than either non-filter-feeders or nekton that do not live near the sediments. Despite this, oysters continue to thrive due to their resilience to pollutants with several studies such as dos Reis et al. (2015) reporting no mortalities after exposing C. brasiliana to up to 1,000 μg L–1 phenanthrene (Phe) for up to 10 days, Perić et al. (2020) reporting no mortalities after exposing O. edulis and M. gigas to up to 100 μg L–1 copper and 50 μg L–1 cadmium for up to 4 days, and Aguirre-Rubí et al. (2018b) demonstrating that oysters stayed alive for as long as 10 days without water despite exhibiting possible contaminant-induced digestive gland atrophy and hemocytic infiltration of interstitial connective tissue. Thus their ability to survive despite the pollution while exhibiting measurable responses to it, combined with their distribution, living habits, and multiple life stages make oysters an exceptionally favorable bioindicator organism (Zhou et al., 2008).
PAHs are organic molecules made up of two or more aromatic hydrocarbon rings and have become a staple in the investigation of the effects of oil spills and coastal pollution near urban areas. Originating from sources such as petroleum (petrogenic) and the incomplete combustion of petroleum (pyrogenic), PAHs have become widespread across the globe, especially concentrated near urban centers (Laflamme and Hites, 1978; Wang et al., 1999). As such, they have become convenient proxies for all human activities that rely on fossil fuels. PAHs themselves are characterized by their low solubilities (Sangster, 1989) and are thus usually found adhering to small particles in either marine sediment (Vaezzadeh et al., 2019) or soil (Eker and Hatipoglu, 2019). Due to their semi-volatility, PAHs can be removed via evaporation or photooxidation (Karaca and Tasdemir, 2013; Eker and Hatipoglu, 2019), a process that could become problematic depending on the photoproducts. In general, photooxidation of PAHs yields reactive molecules, such as aldehydes and aromatic ketones known as quinones (Ehrenhauser, 2011), which are highly toxic (reviewed by Lee, 2003) and reminiscent of the compounds created during PAH metabolism (reviewed by Ertl et al., 2016). Therefore, PAHs are often regarded as carcinogenic and of great health risk due to their lipophilicity and ability to readily accumulate into body tissues (Abdel-Shafy and Mansour, 2016). Thus it is crucial to look into how PAHs have accumulated and their effects on the organism.
Of the 16 PAHs recognized by the USEPA, all but the four smallest PAHs Naphthalene (Naph), Acenaphthylene (Acy), Acenaphthene (Ace), and Fluorene (Flu) have log Kow > 4 (Sahu and Pandit, 2003) and are considered hydrophobic or lipophilic (La Guardia et al., 2012). Flu itself is on the borderline with a log Kow = 3.96, whereas the widely accepted value of 4.18 (Sangster, 1989) makes Flu lipophilic. Therefore, among PAHs, solubility generally decreases with increasing molecular weight (Sahu and Pandit, 2003). Oysters tend to concentrate very high levels of PAHs, but the lipophilicity of the organic compounds affects the accumulation patterns. Since Geffard et al. (2003) found that the bioaccumulation factor (ratio of PAH concentration in the body to the PAH concentration in the sediments) of M. gigas larvae was negatively correlated with log Kow, a PAH is more likely to accumulate if it is more soluble.
In general, a 2–3-ring PAH is considered a low-molecular weight (LMW) PAH (Vaezzadeh et al., 2019; Pham et al., 2020; Wang et al., 2020) whereas a PAH having 4–6 rings is considered high-molecular weight (HMW), although Vignier et al. (2018) consider 3–6 rings as HMW and 2–4 rings as being soluble. Due to the difference in solubility, the HMW PAHs are more likely to be adsorbed to the sediment, especially if the sediment contains a large organic fraction (Baumard et al., 1999; La Guardia et al., 2012). In Dalian, China, HMW PAHs were found to accumulate throughout the year into sediments due to a calculated net flux from seawater to sediment (Hong et al., 2016). This could explain why in the water column near Hainan, China, 98% of the PAHs are LMW PAHs with 2–4 rings while 3-ringed (61%) and 4-ringed (28%) PAHs formed the majority of the sediment-bound PAHs (Wang et al., 2020). This highlights a shift toward HMW PAHs in the sediments. Vaezzadeh et al. (2019) found the mangrove sediments of Malaysia to be dominated by 5–6-ring PAHs, making up between 45–80% of the total PAHs found in the sediments. This in turn results in the increased bioavailability of the more soluble LMW PAHs (Baumard et al., 1999), and a greater concentration of LMW PAHs accumulated in the oyster. The PAHs accumulated in M. belcheri were reported to consist of at least 40% LMW PAHs in four out of five different mangrove sites around Malaysia (Vaezzadeh et al., 2019) despite the majority of HMW PAHs in the sediments. Resonating this pattern, the contaminants in both the Magallana spp. oysters and Cymatium spp. gastropods sampled from the Can Gio coastal wetland of Vietnam were enriched in 2–3-ring LMW PAHs, with the smallest PAH, Naph, dominating the PAH composition in oyster whole soft tissue (Pham et al., 2020). The marine oyster O. equestris predominantly accumulated the 3-ringed Phe in the Gulf of Mexico until major flooding of the Mississippi River occurred. However, molecular weight and bioavailability should not be taken as a law. Equal molecular weight pyrene (Pyr) and fluoranthene (Fla), exposed at roughly equal concentrations, still resulted in a soft tissue concentration of Fla exceeding 50% greater than that of pyrene in S. glomerata (Ertl et al., 2016). Increased uptake and/or retention of Fla or increased metabolism and elimination of pyrene potentially due to the observed upregulation of carbonyl reductase (CBR) were proposed to explain such discrepancies.
Despite increased bioavailability due to solubility, uptake of PAHs is not as simple as diffusion. Evidence suggests that the presence of sediments greatly increases PAH uptake. Exposure to PAH-contaminated sediments proved to be almost 100 times as toxic to M. gigas embryos than exposure only to the elutriate of those sediments (Geffard et al., 2001) suggesting that the PAHs in the sediments were more bioavailable than those in the water above in some way. Elutriates are essentially suspensions of “washed off” or decanted sediments and are synonymous with low or high-energy water attenuated fractions (HEWAF, LEWAF) that mix compounds of high log Kow such as crude oil rather than sediments with water (Vignier et al., 2015). In the follow-up study by Geffard et al. (2002), unfiltered elutriate was tested against filtered elutriate. It was found that the unfiltered elutriates still contained over 10 times the total PAH concentration of the filtered elutriates, which was deduced to come from extremely fine suspended sediments > 0.7 μm minimum length. Larvae reared in the fine suspended sediments (unfiltered elutriate) bioaccumulated three times more PAHs in the 25% elutriate-dilution treatment (least dilution, most concentrated treatment) than did larvae reared in filtered seawater with no contaminants, but fed algae reared in the filtered elutriate. Since the filtered elutriate contained just one-tenth of the PAHs in the unfiltered elutriate, the fact that the larvae fed algae only contaminated with filtered elutriate was able to reach one third the PAH concentration of the larvae directly reared in the unfiltered elutriate provides additional support that ingestion is a highly significant mode of PAH accumulation. However, this only occurred at the highest concentration (25% elutriate). The other concentrations (1, 5, and 10% elutriate) still caused 50–100 ng/g dry weight of accumulation in the larvae reared in the unfiltered elutriate, while the larvae reared in clean seawater but fed contaminated algae accumulated close to nothing. Therefore, even though ingestion is an important mechanism for accumulation, it could be extremely limited when the contaminant levels in prey are very low, and the additional PAHs in the unfiltered elutriate may have been accumulated by the larvae through different means. Shao and Wang (2020) showed that even 60-nm nanoparticles would be ingested, while the suspended fine sediments in the unfiltered elutriate from Geffard et al.’s (2002) study were at least 700 nm (0.7 μm). Therefore, it could be the case that non-food particles are being consumed and contributing to the bioaccumulation of PAHs. A study has shown that a diet of particulate matter with less organic content is more likely to be rejected than particles more enriched in organic content by the cockle Cerastoderma edule (Iglesias et al., 1996), which could suggest that particles with enough organic matter may fail to be rejected and instead be consumed alongside food particles. Further research by Geffard et al. (2003, 2004) continues to reinforce the role sediments play in bioaccumulation, and suggests that resuspension of sediments, not unlike the action of elutriation, facilitate the uptake of PAHs and heavy metals.
The PAHs that are taken up, are then allocated to lipid-rich organs. Wang et al. (2020) break down the oyster anatomy into five portions: adductor, gill, gonad, hepatopancreas, and mantle. In other studies, the hepatopancreas is often referred to as the digestive gland (Bustamante et al., 2012b; Vaschenko et al., 2013; dos Reis et al., 2015; Shenai-Tirodkar et al., 2017; Aguirre-Rubí et al., 2018b; Perić et al., 2020; Shao and Wang, 2020) and the adductor is referred to as “muscle” (Bustamante et al., 2012b). The use of “soft tissues” is much more vague as it likely refers to gonads and mantle (Shao and Wang, 2020), non-gill tissue (Chan and Wang, 2019), or even all tissues combined and sometimes called either “total” or “whole” soft tissues (Aguirre-Rubí et al., 2018b; Sezer et al., 2018; Wang et al., 2020). In general, PAHs accumulated in the mantle or hepatopancreas, with gonad or gill next and adductor muscle last (Wang et al., 2020). Bustamante et al. (2012b) found that M. gigas exposed to the 4-ring PAH, pyrene (Pyr), for 24 h had the highest concentrations in the gills, followed by the hepatopancreas, then mantle, and then adductor muscle with twofold reductions at each step except for the last step in which the mantle contained 2.5 times more Pyr than the adductor muscle did directly after exposure. It was suggested that the mantle and especially gills may have high levels of PAHs simply due to their being the most exposed organs to the environment (Bustamante et al., 2012b) because, during a 15-day depuration period, some Pyr was retained in the mantle and hepatopancreas, but was removed from the gills rapidly. The high lipid content of the hepatopancreas and gonad may store lipophilic compounds such as PAHs (Wang et al., 2020), contributing to slower removal and increased bioaccumulation in those tissues. These findings led to the proposal that the mantle and hepatopancreas should be the target organs for biomonitoring data collection (Wang et al., 2020).
Differently sized organs of different species, therefore, result in differential accumulation within the same habitat conditions. Three different species of bivalves, the oyster M. gigas, mussel Mytilus californianus, and clam Corbicula fluminea, transplanted for 90 days within San Francisco Bay, United States, accumulated different levels of PAHs despite controlling for location and time (Oros and Ross, 2005). The oyster M. gigas accumulated the most out of the three species with total PAH ranging from 184–6,899 ng/g dry weight, while the mussel and clam accumulated 21–1,093 and 78–720 ng/g dry weight, respectively. Due to the high lipid content of oysters, this species is thought to be an excellent bioindicator for lipophilic contaminants such as PAHs, polychlorinated biphenyls (PCBs), and organochlorine pesticides (OCPs) (Fisher et al., 2000; Bustamante et al., 2012a; Leon et al., 2013; Trevisan et al., 2016; Aguirre-Rubí et al., 2018a) and even organic metals such as tributyltin (Alzieu et al., 1986; Higuera-Ruiz and Elorza, 2011) and methyl-Hg (Metian et al., 2020).
Once PAHs are taken up by the hepatopancreas, which is the detoxifying and xenobiotic-metabolizing center (Wang et al., 2020) akin to the human liver, many physiological mechanisms begin actively breaking down and detoxifying the potential carcinogen. In a process that will be discussed in the next section in greater detail, PAHs are reduced by stage I metabolic enzymes into water-soluble hydroquinones and later either excreted or conjugated by stage II metabolic enzymes into thio-conjugated o-quinones and subsequently excreted (Ertl et al., 2016). PAH-metabolism ultimately results in its depuration and effective detoxification. Some PAHs may be preferentially metabolized such as Pyr, Benzo[a]pyrene (BaP), and Benzo[a]anthracene (BaA) (Ertl et al., 2016), which may affect the ratios of PAH isomers such as BaP and BeP (Baumard et al., 2001). Since PAH ratios are often used as diagnostic ratios to track pyrogenic or petrogenic sources (Pie et al., 2015), preferential metabolism could lead to false readings and the calculation should be calibrated accordingly.
To assess the effects of a stressor, the researcher must identify biomarkers that would in theory react in some measurable way. In the case of chemical contaminants, most of the endpoints treat the compounds negatively as toxic and expect a weakening of physiology. The anatomy or histology could be directly observed to deviate from the norm, the physiological process could be expected to occur slowly or not at all, and mortality remains a viable effect. Other scientists take a more response-based approach and rely on the organism’s homeostatic mechanisms to alert any changes due to the contaminant. Such mechanisms could be detoxification processes that attack the contaminant or stress responses that either mitigate or repair damage caused by the contaminant. Still, other scientists may take a more abstract approach by measuring changes in gene expression that signal to the observer that the organism is in the process of either a detoxification process or stress response. In the following sections, a few basic biomarkers will be discussed and explained in the terms of an oyster, and a summary of the effects will be listed in Tables 1–3, according to whether the study was a laboratory-controlled test (Tables 1, 2) or an in situ observation of oysters collected from the field (Table 3).
Table 1. A summary of the results of the lab tests on the toxicities of PAHs is organized here, sorted by contaminant.
Table 2. A summary of the results of the lab tests on the toxicities of contaminant mixtures with other pollutants in addition to PAHs is organized here.
Table 3. The summary of the observations of the field collections reflecting the toxicities of heavy metals and PAHs is organized here.
PAHs express their toxicity in the form of oxidative potential. Unlike heavy metals, the toxicity of PAHs comes from the intermediates formed during their breakdown process. As Ertl et al. (2016) summarized, hydrophobic PAHs are transformed during phase I metabolism of xenobiotics by cytochrome P450 (CYP) proteins and CBR into water-soluble hydroquinones that can be excreted. An increase in phase I metabolic protein transcripts and activity upon PAH-exposure thus suggest that a detoxification response has been initiated. Pyr has been found to cause significant initial spikes in the CYP proteins CYP3475C1, CYP2AU1, CYP2-like, and CYP356A transcripts in C. tulipa that dropped back to base levels by 96 h post treatment (Zacchi et al., 2019), while Flu exposure elicited a similar upregulation of CYP2AU2 in M. gigas (dos Reis et al., 2020). Potentially more lasting reactions were observed in C. tulipa individuals exposed to Flu, with significantly elevated levels of CYP2AU1 96 h post treatment, even though other CYP protein transcripts remained insignificantly changed (Zacchi et al., 2019). These results may be exhibiting a species-dependent response, in which “species” could ambiguously refer to either the organism or the PAH, or simple unreliability as biomarkers for PAH toxicity. Following phase I metabolism, the quinones (sometimes known as oxy-PAHs) produced are often even more toxic than their parent PAHs (Sarker et al., 2018; McCarrick et al., 2019) and present a serious electrophilic threat. During phase II metabolism, the conjugation of quinones with glutathione is catalyzed by glutathione S-transferase (GST) in order to detoxify and excrete contaminants via the mercapturate pathway (Cooper and Hanigan, 2018). Other pathways involve the conjugation of xenobiotics into sulfate esters by sulfotransferases (SULT) to facilitate excretion as well (Croom, 2012). Both enzymes experienced the similar, aforementioned short-term upregulation in C. tulipa and M. gigas exposed to Pyr and Flu, respectively (Zacchi et al., 2019; dos Reis et al., 2020). Overall, since the act of removal of PAHs causes its bioactivation as a toxicant, a constant supply of antioxidants is needed to prevent oxidative damage during PAH metabolism. Superoxide dismutase (SOD), catalase (CAT), and glutathione peroxidase (GPx), in addition to detoxification enzymes such as GSTs and SULT are thus potentially useful biomarkers to use when studying the effects of and tracking the accumulation of PAHs.
Indeed, levels of SOD, CAT, and GST in S. cucullata were found to be very closely correlated to total PAH concentrations from various sites in the Arabian Sea (Sarkar et al., 2017). In contrast, neither SOD, CAT, nor GST was found to be upregulated in S. glomerata exposed to dietary Pyr and Fla for 7 days, with GST ultimately being downregulated (Ertl et al., 2016). The phase I metabolic proteins, cytochrome P450 and CBR, were drastically upregulated, indicating active metabolism of the accumulated PAHs and reduction of the quinone intermediates, and it was proposed that preexisting SOD and CAT in the oysters may have been sufficient in clearing the reactive oxygen species (ROS) formed during this process. In addition to cytochrome P450 and CBR, the third group of phase I metabolic enzymes, aldo-keto reductases (AKR), specializing in metabolizing aldehydes and ketones was downregulated instead. This possibly suggests a preferential pathway of CBR reduction (Ertl et al., 2016) or a lack of need for AKRs in PAH breakdown. As for the downregulation of GST, it should be noted that such ambiguous phenomenon was observed in O. edulis and M. gigas by Perić et al. (2020) following the exposure of heavy metals Cu and Cd, and that antioxidant or metabolic enzymes should not be exclusively relied upon as biomarkers of PAH exposure. The lack of any change in CYP, GST, SULT, SOD, CAT, and GPx transcripts and/or activity in the gills of C. brasiliana after Phe exposure (Lima et al., 2018) followed by the downregulation of CYP, GSTΩ and increase in SOD, CAT, and GST activity in the same experiment with C. tulipa (Lima et al., 2019) further promotes caution regarding the reliability of these biomarkers. It is interesting to note that the oysters observed in the field under chronic exposure (Sarkar et al., 2017) returned the more expected results in expression of antioxidant and metabolic proteins, while the oysters under the acute, controlled laboratory exposures exhibited much less expected and more complicated results (Ertl et al., 2016) and may be related to the intertwining of PAH metabolism and toxicity. For example, acutely-exposed oysters may rely on pre-existing SOD, CAT, and GST as needed, since PAHs only exhibit toxicity when transformed, while those chronically-exposed could never maintain a reservoir of SOD, CAT, and GST and must constantly produce them.
In addition to oxidative damage on tissues such as lipid peroxidation due to PAHs, DNA damage was observed in a few studies. PAHs have long been recognized in many studies as a cancer-causing agent in marine systems (Le Goff, 2017). Besides lipids, nucleic acids are a susceptible target of oxidative attack. DNA damage is often characterized by DNA strand breaks or unwinding (Sarkar et al., 2017; Sarker et al., 2018), and by problems during DNA replication involving micronuclei formation (McCarrick et al., 2019). S. cucullata collected off the west coast of India were found to contain DNA strand breaks seemingly proportional to the amount of PAH pollution at each site (Sarkar et al., 2017). The follow-up study by Sarker et al. (2018) expanded the previous study by including multiple abiotic parameters and heavy metal concentrations to rule out confounding variables and found that PAHs contributed to at least 87% of the variation in DNA integrity. Lab results confirm that the effects of BaP on the loss of DNA integrity is dose-dependent (Sarker et al., 2018) and support the hypothesis that PAHs as mutagens in oysters.
Left unchecked, mutagens may cause cancerous tumors and lesions in several different tissues in oysters over chronic exposure. 13.6% of C. virginica exposed to contaminated sediment off the coast of New York at a suspended concentration of 20 mg/L for 30–60 days developed tissue neoplasms including renal cell tumors, water tube and gill filament tumors, gastrointestinal adenocarcinoma, neuroblastoma, circulatory system tumors, and gonadal papillary lesions (Gardner et al., 1991). Although, the sediments contained heavy metals, pesticides, and PCBs in addition to PAHs, the concentration of accumulated non-PAHs in oysters never exceeded 8× the pre-treatment control levels, whereas PAHs accumulated between 6 and 60× the pre-treatment control levels with most being over 20× pre-treatment levels. Due to a lack of available literature on PAH-induced neoplasia in mollusks and the difficulty of attributing etiology in field studies by the aforementioned confounding variables, the reliability of cancerous lesions as a biomarker for PAHs is uncertain thus far. Although neoplasia has been observed in fish from contaminated habitats in many studies (reviewed by Le Goff, 2017), many of the confounding variables remain in the pathogenesis of the neoplasia, such as viruses which are known to cause a wide variety of neoplasia including hemic neoplasia. Longer-term laboratory-controlled experiments in zebrafish (Danio rerio) have revealed a link between petroleum compounds and carcinogenic effects especially on the liver (Larcher et al., 2014), which could equate to similar results if tested on mollusks.
Should the prevalence of neoplasia be due to increased viral infection, alteration of immune function by PAH exposure may be a possible cause. There is evidence to suggest that PAHs are toxic to hemocytes. For example, M. gigas exposed to dietary PAHs on spiked diatoms displayed increases and decreases in different types of hemocytes (Croxton et al., 2012). More specifically, Pyr and BaP exposure increased the proportion of agranular hemocytes while reducing the granular hemocyte proportion in part due to mortality. Hemocyte apoptosis was also observed in C. virginica juveniles that were exposed to a HEWAF of crude oil (Vignier et al., 2018). This could be the cause of reduced phagocytic capacity, which was reported to occur in M. gigas collected in areas affected by oil spill (Auffret et al., 2004). Without distinguishing between the types of hemocytes, Xie et al. (2017) recorded a net decrease in hemocyte count, and the proportion of mobile hemocytes, those involved in phagocytosis, in the pearl oyster Pinctada imbricata (formerly Pinctada martensii) from Pyr exposure. On the other hand, apparent increase in immune system activity was found in M. gigas, that exhibited decreased hemocyte mortality, increased phenoloxidase activity, lysosome count, and the% of non-specific esterase cells upon exposure to a wide variety of PAHs (Bado-Nilles et al., 2008). S. glomerata, having been exposed to Pyr and Flu for 7 days, differentially expressed many unique pattern recognition receptor proteins (Ertl et al., 2016) involved in detecting and neutralizing pathogens, including peptidoglycan recognition proteins, C1q domain-containing proteins, toll-like receptors, c-type lectins, c-type mannose receptors/macrophase mannose receptors, galectin, gram-negative bacteria binding protein, scavenger receptor, 2′–5′-oligoadenylate synthase 1, and fibrinogen-related proteins. In the field, exposure to an oil spill off the coast of Korea was associated with a possible reduction (not statistically significant) in granulocyte population and phagocytic capacity in M. gigas (Donaghy et al., 2010), while chronically exposed M. gigas measured to have elevated PAH tissue concentrations expressed immunotoxicity in the form of hemocytes with impaired mitochondrial function and a 50% reduction in phagocytic function (Auffret et al., 2004).
It follows then, that lab experiments show an increase in both disease transmission and susceptibility with increasing concentration of a combination of PAHs and heterocyclic compounds (Chu and Hale, 1994; Chu et al., 2002). The prevalence of Perkinsus marinus increased both in oysters inoculated with the parasite and in oysters placed nearby without direct inoculation when treated with a less-diluted water-soluble fraction of contaminated sediments from the Elizabeth River near the Chesapeake Bay in the United States (Chu and Hale, 1994). Due to the use of only the water-soluble fraction, this result was achieved using the more soluble low molecular weight PAHs and heterocyclic compounds. In practice, however, this result is much more ambiguous. The prevalence and intensity of P. marinus were not found to significantly correlate with total PAH body-burden but associated with urban land use instead (Wilson et al., 1990). Many other factors were suggested to confound this relationship. For example, high latitude lower temperatures, and low salinities were all associated with reduced infection load. It was proposed that colder conditions reduce reproductive seasons, which in turn reduce efflux of PAHs resulting in greater body burden while being less susceptible to P. marinus (Wilson et al., 1990). Little could be said about the connection between PAHs and infection with P. marinus, and its correlation thus far seems to be coincidental.
Besides changes in immune function, additional physiological consequences arise in oysters exposed to PAHs. The algae clearance rates of both M. gigas adults and C. virginica spat algae clearance rates were found to be reduced in individuals exposed to a PAH mixture and HEWAF of Deepwater Horizon slick oil, respectively (Jeong and Cho, 2007; Vignier et al., 2018). Shell closure, a physiological defense against unfavorable water conditions, was blamed for the reduced feeding among the contaminated oysters (Vignier et al., 2018). Once the valves shut, feeding ceases and the oyster begins to decline in health and experience symptoms reminiscent to that of starvation, especially due to the lack of partially-digested food particles in the alimentary tract (Vignier et al., 2018). Degradation of the alimentary canal was observed, with HEWAF-treated juvenile C. virginica exhibiting digestive tubule atrophy and necrosis, luminal dilation, ulcers, inflammation of the stomach and labial palps, hypertrophic and overrepresented mucous cells in the lumen along with a truncation in the height of luminal epithelial cells (Vignier et al., 2018). Similar digestive tubule atrophy was also observed in C. tulipa and M. gigas exposed to Pyr or crude oil HEWAF, while an increase in mantle mucosal cells was seen in C. tulipa exposed to Pyr and M. gigas exposed to Flu (Luna-Acosta et al., 2017; Zacchi et al., 2019; dos Reis et al., 2020). The increase in mucous cells in the lumen was accompanied by excess mucus as well as hemocyte diapedesis in the alimentary tract as a possible strategy to expel xenobiotics (Luna-Acosta et al., 2017; Vignier et al., 2018). Digestive gland edema was also found in wild C. rhizophorae from a contaminated site in Colombia (Aguirre-Rubí et al., 2018b). Yet, despite the inflammation and erosion of the digestive tract, mortality rates among spat remained low and insignificantly changed due to exposure to the HEWAF of crude oil.
Lysosomal health has been traced in response to PAH-related stress as well. Lysosomes are organelles filled with hydrolytic enzymes and helpful to the detention of xenobiotics (reviewed by Hwang et al., 2008; Wang et al., 2018). Lysosomal enlargement in digestive cells occurred in crude oil HEWAF-exposed M. gigas (Luna-Acosta et al., 2017), potentially indicating storage of accumulated oil or PAHs. The lysosomes in hemocytes, however, were not only observed to increase in number in M. gigas exposed to DiBenzo[a,h]Anthracene (DBahA) (Bado-Nilles et al., 2008), but also experience membrane permeability in a process known as lysosomal destabilization in C. virginica exposed to a mixture of 24 PAHs and C. brasiliana exposed to a variety of chemicals including PAHs in coal tar-based paint (Hwang et al., 2008; Chiovatto et al., 2021). This membrane breach may result in the release of cathepsins into the cytoplasm (reviewed by Wang et al., 2018) that ultimately could be responsible for the hemocyte apoptosis recorded by Vignier et al. (2018) in juvenile C. virginica exposed to crude oil HEWAF. Lastly, since the exposure of M. gigas to the chemical dispersant FINASOL® caused only lysosomal biomarkers to be affected, and did not cause the additional symptoms such as antioxidant activity and digestive tubule atrophy associated with Brut Arabian Light oil HEWAF and CEWAF exposure (Luna-Acosta et al., 2017), changes to lysosomal activity may be very sensitive endpoints to consider for PAH-exposure.
Due to rapid turnover time, high quantities, and high sensitivity to contaminants, the early life stage developmental milestones (embryo, larvae) are useful biomarkers for PAH toxicity assessment. Millions of larvae could be produced rapidly, only taking roughly 24–48 h from zygote to D-shaped larva. Abnormal larval shape is the most common endpoint, described by His et al. (1997) for M. gigas larvae as possessing morphological aberrations in the form of a convex hinge, indented shell margin, incomplete shell, and/or a protruding mantle. Such abnormalities are potentially the result of rapid shell closure that occurs so quickly that, like juvenile oysters, the larvae are left with a protruding mantle or velum (Vignier et al., 2016). The subsequent starvation of the larval oyster could then explain the reduced shell length, larval abnormality, stunted growth, and mortality observed in larval C. virginica exposed to the HEWAF of oil from the Deepwater Horizon spill (Vignier et al., 2015, 2016, 2019). Later-term pediveliger larvae of settlement age exposed to the same HEWAF also experienced a drastic reduction in spatfall or settlement success across all concentrations with no dose-dependency (Vignier et al., 2016). The chemically-enhanced water accommodated fraction (CEWAF) of the oil using the dispersant Corexit exhibited a clear, almost linear, dose-dependency of settlement success, despite potentially greater access to the PAHs (Vignier et al., 2016). Therefore, the dramatic drop in settlement success in the HEWAF assays were either due to a PAH-exclusive effect on physiology or the coating of settlement surfaces with PAHs. The former seems not to be the case, since the presence of the dispersant alone usually resulted in extreme toxicity, often far greater than the HEWAF alone (Vignier et al., 2016, 2019; Volety et al., 2016).
When exposed to PAH-contaminated sediment elutriate during pre-fertilization, M. gigas sperm showed no significant changes in health and fertilization success (Geffard et al., 2001). These findings were corroborated by Volety et al. (2016), who found that neither the survivorship, viability, nor acrosomes of the C. virginica sperm cells were negatively affected by exposure to the HEWAF of crude oil. In fact, it was found that ROS activities had unexpectedly dropped, which was explained by greater metabolic activity occurring and the active detoxification of the ROS. However, exposure of both sperm and oocyte of C. virginica to a HEWAF of crude oil or sediment elutriate resulted in a significant reduction in fertilization success (Vignier et al., 2015; Volety et al., 2016; Boulais et al., 2018). This may suggest that the eggs are susceptible to the toxicity of PAHs. Post-fertilization exposure to unfiltered elutriates produced from contaminated sediment for 24 h resulted in a totality of abnormal larvae (Geffard et al., 2001). The larvae accumulate PAHs so quickly that elongated exposure time did not significantly change the number of abnormal larvae (Geffard et al., 2004). Having contact with the sediment was many times more potent than being in contact with only the unfiltered elutriate (Geffard et al., 2001, 2003, 2004), corroborating the notion that sediments provide a powerful vehicle for the accumulation of contaminants. Other modes of entry, such as ingestion of algae reared in contaminated elutriate, exhibited similar results to non-filtered elutriate (Geffard et al., 2002). Based on the Effective Concentration for 50% abnormality (EC50) values for gametes (267 μg/L, Vignier et al., 2015) and embryos (342 μg/L reported by Vignier et al., 2015; 22.4 g/L reported by Boulais et al., 2018), the gamete is more sensitive than the embryo, and the embryo is more sensitive than the larval stage is to environmental contaminants (Boulais et al., 2018).
Oysters of the order Ostreida are a group of hardy bivalves globally distributed in intertidal and shallow subtidal regions within sheltered bays, mangroves, or estuaries with freshwater input (Gosling, 2003). This subjects them routinely to wide temperature and salinity fluctuations through which the oysters survive. Temperature and salinity ranges may be at least as wide as 3–35°C and 5–40 ppt for M. gigas (Bayne, 2017), and 5–25°C and 16–34 ppt for O. edulis (Hutchinson and Hawkins, 1992; Bayne, 2017). However, optimal ranges for growth and reproduction are much narrower, with ranges constricted to approximately 11–34°C and 22–35 ppt for M. gigas, and 15–20°C and 30–32 ppt for O. edulis (Shatkin et al., 1997; Wiltshire, 2007; Bayne, 2017), beyond which stress response systems activate to maintain homeostasis. Such responses are critical for the survival of oysters and other bivalves under extreme and/or prolonged environmental change.
The environment is also not a static system, especially under the human influence by which rising pCO2 levels are expected to reach 538 ppm by 2,100 under the Relative Concentration Pathway of 4.5 W/m2 of radiative forcing (RCP4.5), the current most conservative climate change scenario predicted by IPCC (2013). At this rate, the global mean surface temperature is expected to increase by at least 1°C with global ocean surface pH dropping below 8 (IPCC, 2013). Under the worst-case scenario RCP8.5, the global mean surface temperature may increase by up to 3°C or more with global ocean surface pH falling below 7.8. These projections imply that organisms will potentially be subject to chronically elevated temperatures in addition to ocean acidification. Increasing frequency of heavy precipitation events and tropical cyclones, deemed to occur more likely than not in the late 21st century (IPCC, 2013), will cause drastic short-term changes in salinity in estuarine and bay systems whether it be massive dilutions due to extreme precipitation and flooding (Edmiston et al., 2008; Munroe et al., 2013) or salinity rises due to storm surge (Huang et al., 2014). Mass die-offs of 55–90% of oysters have already been recorded within the Gulf of Mexico due to prolonged periods of reduced salinity as low as 0 for several weeks (Edmiston et al., 2008; Munroe et al., 2013), so it is clear that additional abiotic factors affect oyster health, and may compound onto concurrent PAH-burden.
The solubility and bioavailability of PAHs, especially that of LMW PAHs, increases with decreasing salinity (Shukla et al., 2007), which may result in the increased uptake of PAHs as demonstrated in fish including tilapia (Oreochromis mossambica, formerly Tilapia mossambica), rainbow trout (Oncorhynchus mykiss), and mummichog or Gulf killifish (Fundulus heteroclitus) at lower salinities (Ramachandran et al., 2006; Shukla et al., 2007). However, such straightforward effects are not always observed, especially if other abiotic factors are involved, as evidenced by the complete lack of correlation between salinity and tissue-concentration of PAHs in oysters collected from different sites (Bebianno et al., 2007). When bivalve mollusks experience osmotic shock, they often close their valves (Kurihara, 2016) to limit water movement, and then attempt to osmoregulate by deamination of free amino acids (reduce internal concentration) or decomposition of proteins (increase internal concentration) (reviewed in Pourmozaffar et al., 2020). Withdrawal and shell closure has also been associated with starvation since the oyster is no longer feeding (Vignier et al., 2018), which may further complicate additional burdens by placing tighter limits on energy reserves for more prolonged exposure times. Furthermore, lower salinities have been associated with a host of negative effects on bivalves such as lipid peroxidation in the Mediterranean mussel (Mytilus galloprovincialis) and littleneck clam (Ruditapes philippinarum) (Velez et al., 2016; Freitas et al., 2017), reduced shell length and thickness of the blue mussel (Mytilus edulis) (Maar et al., 2015), reduction in the body cavity index (ratio of the flesh mass to the internal cavity volume) of C. virginica (Heilmayer et al., 2008), reduced growth rate and size of both O. edulis and O. lurida larvae (Robert et al., 1988; Lawlor and Arellano, 2020), and decreased phagocytosis activity coupled with increased hemocyte mortality in several bivalves (reviewed by Pourmozaffar et al., 2020). This could mean that hypoosmotically-weakened oysters are highly susceptible to PAH-compounded health consequences resulting from the increased accumulation of more-soluble PAHs.
In a thus far rare experiment testing the effects of salinity on Phe exposure on C. brasiliana, strictly increasing bioaccumulation was not observed with decreasing salinity (Zacchi et al., 2017). Instead, the intermediate salinity (25) accumulated the highest amount of Phe (88.4 μg/g dry weight) followed by low salinity (10, 81.5 μg/g dry weight) and then high salinity (35, 72.0 μg/g dry weight) (Zacchi et al., 2017). It may be possible that non-optimal salinities (low and high) have inhibitory effects on the bioaccumulation of PAHs, which would almost serve as a protective measure. However, low salinities still carry the burden of hypoosmotic stress or other mechanisms that negatively affect the health of oysters. The intermediate amount of Phe accumulated at the lowest salinity was associated with elevated CYP isoforms (CYP2AU1 and CYP2-like1) after 24 h of exposure with high levels of CYP2-like1 remaining after 96 h when compared to the control (Zacchi et al., 2017). As CYP proteins are involved in stage I metabolism of PAHs, this shows an increased effort to detoxify Phe at low salinities, possibly due to increased toxicity. As a result, the antioxidant enzymes CAT-like, SOD-like, and two GST proteins GSTm-like and GSTΩ-like were upregulated in the low salinity treatment compared to the two higher salinity treatments as expected. Other than oxidative stress; however, Phe did not seem to inhibit osmoregulation activities and gene expression since amino acid-metabolizing proteins were upregulated with no impact from Phe (Zacchi et al., 2017).
Animals other than oysters have showed increased sensitivity to PAHs at lower salinities as well. The LC50 of the HEWAF of crude oil was found to be significantly reduced, reflecting increased mortalities, at the lower salinities in all three animals tested, grass shrimp (Palaemon pugio, formerly Palaemonetes pugio), sheepshead (Cyprinodon variegatus), and mud snail (Ilyanassa obsoleta, formerly Tritia obsoleta) (DeLorenzo et al., 2021). In fact, there was a clear positive relationship between salinity and LC50. Mussels (Mytilus galloprovincialis) collected at different sites along the south coast of Portugal where salinity varied from 4–34.9 psu exhibited cytochrome P450 levels consistent with what levels would be expected based on the tissue-concentration of PAHs (Bebianno et al., 2007). GST levels from PAH metabolism were found to vary inversely with salinity, which reflects the increased amount of stress at lower salinities. The pattern of GST levels occurred regardless of the tissue-concentration of PAHs, which possibly indicated that the detoxification activity responded to the compounding of low salinity on PAH burden rather than the increasing PAH accumulation at lower salinities. Such apparent changes in toxicity due to reduced salinity were not observed in a number of studies that varied abiotic factors during the exposure of fish to a water attenuated fraction of weathered oil (Serafin et al., 2019; Simning et al., 2019; Allmon et al., 2021). Additionally, Schrandt et al. (2018) found that oil (MC252)-exposed juvenile C. virginica showed a clear interaction between oil exposure and salinity on the survivorship and shell growth. Both survivorship and shell growth diminished with oil exposure. Surprisingly, however, the effects of oil appeared to be exacerbated by the moderate salinity level (18) vs. the low salinity level (8) (Schrandt et al., 2018), despite the moderate salinity level (15–18) being recommended as the optimal salinity for C. virginica (Wallace, 2001). It was suggested that short-term exposure to oil is mitigated by salinity changes due to the shell closure reflex, thus preventing further exposure to oil as well. A discrepancy between short-term and long-term exposures alongside abiotic conditions must then be accounted for in the results from lab experiments, especially in this case in which salinity changes confound rather than compound the effects of contaminant exposure.
Temperature plays a huge role in controlling biological events in oysters such as reproduction and spawning (Bayne, 2017) and subsequent larval swarming (Maathuis et al., 2020). Over small ranges, the temperature has little effect (Matoo et al., 2013), in part due to the wide thermal tolerances of most oysters. But where temperature fluctuations are greater, physiological effects often scale monotonically with temperature, and to a greater degree in contrast to the relationship with salinity. Increasing temperature is not only significantly correlated with growth rate and calcification (Robert et al., 1988; Harney et al., 2016), but also heat duress, during which heat shock proteins (hsp) are upregulated (Yang et al., 2016; Nash and Rahman, 2019; Nash et al., 2019), oxidative stress in both juvenile and adult oysters (Moreira et al., 2017), and reproductive problems marked by cell apoptosis in the gonads (Nash and Rahman, 2019; Nash et al., 2019). Considering the negative effects associated with exposure to high temperatures, the effects could compound with additional PAH exposure. Sampling oysters during the wet and dry season in areas contaminated with PAHs offers a glimpse into how temperature may alter the effects of PAHs. The prevalence of digestive gland oedemas in C. rhizophorae from Colombia was only observed in significantly high numbers in oysters taken from one contaminated site during the wet season, but became ubiquitous across all sites during the dry season (Aguirre-Rubí et al., 2018b). In addition, the number of reproductive anomalies and presence of Nematopsis spp. parasites was elevated in oysters sampled during the wet season from what was considered to be one of the more contaminated sites in Colombia, and even more exaggerated during the dry season (Aguirre-Rubí et al., 2018b). These examples of the effects of pollution becoming more visible or only visible during the dry season provide evidence toward compounding effects of temperature and PAH toxicity. Since it has been found that the pearl oyster Pinctada imbricata (also formerly Pinctada radiata) accumulated a significantly greater amount of Phe at 28 vs 24°C (Jafari et al., 2021—in review), it is very possible that the greater PAH-burden would also cause more deleterious effects.
Dolphin fish (Coryphaena hippurus) larvae exposed to weathered oil HEWAF exhibited very clear dose-dependent effects on the abnormal fluid accumulation in the heart and cardiac output (Perrichon et al., 2018). Edema size, sinus venosus-yolk mass gap, and incidence of intrapericardial hematomas increased with increasing total PAH concentration, whereas heart rate and stroke volume both decreased. Increasing temperature caused a greater sensitivity in stroke volume to total PAH concentration, or in other words, an accelerated effect of the total PAH concentration on the reduction of blood flow. However, the effect of total PAH concentration on the sinus venosus-yolk mass gap was reduced by the increase in temperature (Perrichon et al., 2018), meaning that greater concentrations of PAHs lost some of their potency in ability to cause abnormal swelling. This could be due to a compounded effect of heat stress and oxidative stress from PAH-body burden that caused toxicity to approach its peak lethality, but at the same time, elevated temperature failed to cause more damage. In the aforementioned trio of grass shrimp (Palaemon pugio), sheepshead (Cyprinodon variegatus), and mud snail (Ilyanassa obsoleta), exposure to the HEWAF of Louisiana Sweet crude oil significantly reduced the LC50 of each species when treated at 32°C rather than 25°C (DeLorenzo et al., 2021), apparently lending support to increased toxicity of PAHs at higher temperatures. Unfortunately, available literature on this topic is sparse at the time so there is little else that can be said about the synergistic effects of PAHs and temperature change on a marine organism’s health.
However, heavy metal toxicity is very well-studied and often causes similar effects such as oxidative stress and oxidative damage on oysters (Jo et al., 2008; Shenai-Tirodkar et al., 2017; Alexander et al., 2019). Arsenic (As) exposure in M. gigas larvae contracted the optimal ranges of both temperature and salinity (Moreira et al., 2018). This could be interpreted as either the heavy metals causing non-optimal abiotic conditions such as increased temperature to be even more damaging than before or that those oyster larvae outside their optimal temperature and salinity ranges are more susceptible to As poisoning. The former case seems to be more supported, as it was also shown that cadmium (Cd) exposure in C. virginica caused a sharp increase in mortality at the high temperature of 28°C when individuals not subject to Cd exposure seemed to tolerate the high temperature quite well showing no difference in mortality at any of the different temperature treatments (20, 24, and 28°C) (Lannig et al., 2006). Complementarily, lead (Pb) exposure in mussels (Mytilus galloprovincialis) at higher temperatures appeared to cause a significant inhibition of the release of the antioxidant enzymes SOD, CAT, and GPx (Freitas et al., 2019). Thus, it may be possible to infer that exposure to certain PAHs may also reduce an oyster’s ability to tolerate a wide temperature range by causing more oxidative damage than expected outside the optimal range.
Indeed, such reductions in tolerance could be the case, as the release of hsp70 and fatty acid-binding proteins (FABP) seemed to be somewhat inhibited (although not statistically significant) in the oyster C. brasiliana with Phe exposure at all temperatures tested (18, 24, and 32°C) (Lima et al., 2018). However, even though the accumulation of Phe increased at 32°C compared to at 24 and 18°C, the effect of temperature itself was so great that any consequence of Phe exposure was largely overshadowed. Virtually no differences in both detoxification enzyme and antioxidant enzyme transcript levels and activities were observed between Phe-exposed oysters and uncontaminated oysters at each temperature (Lima et al., 2018). In addition, greater oxidative damage was not observed by Freitas et al. (2019) in the aforementioned Pb-exposed mussels despite the apparent inhibition of release of antioxidant enzymes. It seems that the effects of temperature on PAH toxicity have no obvious pattern. It also may be the case that when interactive effects are observed, it is more likely that the PAH exposure adds to the negative effects of temperature, rather than the temperature changing the toxicity of PAHs. It is clear that although some evidence of the potential for compounding effects between temperature and contaminant exist, the system is dynamic, the synergistic effects are difficult to capture, and that further study is extremely necessary.
There is little doubt that ocean acidification due to rising atmospheric pCO2 levels will have a negative effect on bivalves such as oysters. Mussels and likely other bivalves calcify their shells by controlling the pH directly underneath the shell, creating a region of elevated pH and CO32– concentration that promotes CaCO3 precipitation (Ramesh et al., 2017). This vital activity causes bivalves to be sensitive and often negatively affected by changes in external pH. A CO2-mediated drop in seawater pH has been shown to reduce larval shell growth, cause abnormalities in the larval shell, and ultimately result in a significantly reduced survivorship in many different bivalves including scallops (Pecten maximus) and oysters (M. gigas) (Andersen et al., 2013; Barros et al., 2013; Timmins-Schiffman et al., 2013; Frieder et al., 2017). Due the potentially increased energetic demands of maintaining homeostasis under acidification, the Dynamic Energy Budget model predicts reductions in shell length, flesh weight, and reproductive capacity in adult mussels (Ren et al., 2020). Consistent with this model, stunted growth rate was observed in both juvenile clams (Mya arenaria) and oysters (M. gigas) (Beniash et al., 2010; Zhao et al., 2018), featuring reduced shell mass, soft-tissue mass, and shell strength. Under such an energy-draining abiotic factor, the ability to detoxify contaminants and prevent or repair any oxidative damage is likely to be greatly challenged.
In one of the only currently available pieces of literature on the effects of PAHs on oysters under acidification conditions, Lima et al. (2019) demonstrates that C. tulipa upregulates SOD, CAT, and GST activities despite a reduction in glutathione reductase (GR) activity due to Phe exposure. While reducing the pH to 7.0 and 6.5 and did not cause any change in antioxidant activity besides a slight drop in GR activity in the oysters not subject to Phe contamination, the same drastic pH change resulted in a downregulation of all three enzyme activities down to levels no longer significantly different from that of the uncontaminated oysters. Therefore, low pH may have inhibitory effects on the ability of oysters to detoxify xenobiotics and break down ROS.
There is some evidence that lower pH levels may also have inhibitory effects on the immune response of mollusks. While BaP exposure appears to have reduced granulocyte count and phagocytosis rates in the blood clam Tegillarca granosa, the low pH treatments (7.8 and 7.4) appear to compound the effects of BaP and further reduce granulocyte activity (Su et al., 2017). Furthermore, the immune system signaling pathways were negatively affected by the downregulation of toll-like receptors (TLR1, TLR4, TLR6), myeloid differentiation primary response protein (MyD88), TNF receptor-associated factor 6 (TRAF6), transforming growth factor beta-activated kinase 1 (TAK1), inhibitory κB kinase α (IKKα), and nuclear factor kappa B (NF-κB). Together, these immunomodulating proteins recognize pathogens and signal various immune system pathways that initiate an inflammatory response (Kawasaki and Kawai, 2014). Although the toll-like receptor protein 2 (TLR2) and TAK1-binding protein 2 (TAB2) were upregulated, all other immunomodulatory proteins were downregulated significantly not only by BaP exposure, but also by reduced pH. In fact, reduced pH compounded the effects of BaP and caused a significant and even further downregulation in the oysters exposed to BaP at low pH (7.4) compared to either expression level due to BaP exposure or low pH alone.
Again, the well-studied, toxic effects of heavy metals may be considered to make predictions about the effects of PAHs under acidified conditions. M. gigas oysters exposed to Cd at a the low pH of 7.6 suffered the most oxidative stress in the digestive gland, with elevated SOD, GST, GPx, and lipid peroxidation even though the slightly more modest acidified condition (pH 7.8) saw slightly greater accumulation of Cd (Cao et al., 2018). In a follow-up study, Cao et al. (2019) found that M. gigas individuals exposed to both Cu and low pH (7.6) exhibited several toxic effects in addition to greater oxidative stress and lipid peroxidation than in individuals subject only to Cu contamination. Those oysters suffered gill damage in the form of increased prevalence of vacuolization, cilia erosion, and gill lamina hypertrophy, which could partially be attributed to the increase in accumulation of Cu at the lowest pH tested (7.6). At the same time, the oysters within the low pH, Cu-exposure treatment group had reduced algae clearance rates, respiration rates, and flesh condition beyond that of the oysters receiving only Cu-exposure. This inevitably would result in reduced energy reserves with consequences related to the dynamic energy budget. Evidence for that scenario was found in S. glomerata exposed to Cu at elevated levels of pCO2 (1,000 μatm). This group of oyster individuals maintained physiological activities at levels not significantly different from unexposed individuals; however, the eggs produced by the females were smaller with less lipid content (Scanes et al., 2018). This was hypothesized to be due to a shift in energy allocation from maternal investment to homeostatic maintenance. Surprisingly, the embryos fertilized from the more poorly-developed eggs developed normally; however, such larvae may be at a much greater disadvantage if they were to grow in nature away from the protective laboratory conditions. In summary, increased acidity of the water generally compounds the negative effects of the existing contaminants. With new findings that Phe accumulates in greater quantities in Pinctada imbricata at reduced pH levels (Jafari et al., 2021—in review), it is highly likely that acidification would cause even greater stress in oysters burdened by chronic exposure to PAHs.
One interesting property of PAHs is their potential to be photo-toxic. Not unlike the bioactivation of PAH toxicity during its metabolism, photo-oxidation of PAHs in natural sunlight not only creates ROS immediately, but also breaks them down into toxic quinones (Lee, 2003), which are not only capable of producing ROS but are also more soluble (Ertl et al., 2016) and likely more bioavailable. Lee (2003) reviews what is considered the primary mechanism underlying PAH photo-toxicity: PAHs release a free radical upon sunlight exposure that reacts with oxygen thereby creating ROS. The photo-products such as anthraquinone, benzo[a]anthraquinone, phenanthrenequinone are often significantly more toxic than their parent PAHs were (Lee, 2003). The water-attenuated fractions of weathered oil treated with UV light were shown to be significantly more toxic than untreated oil WAFs (Finch et al., 2016; DeLorenzo et al., 2021). In particular, Finch et al. (2016) demonstrated and isolated UV-light as the effective component of sunlight that induces phototoxicity. The effective concentration at which 10% of the C. virginica larvae or sperm were found to be abnormal (EC10) dropped drastically with increasing intensity of UV light exposure on the water-attenuated fraction. Different oils gave different values of EC10 (Finch et al., 2016), which suggests that oil composition and the presence of compounds that are more likely to be photo-toxic play an important role in the potential photo-toxicity of a given oil film. This discrepancy between oils was observed by Pelletier et al. (1997), in which one of the fuel oils with lower PAH composition, did not display photo-toxicity in contrast to the other three oils tested. The lethal concentrations (LC50) of the oils to brine shrimp (Americamysis bahia) translated to the UV-treated oil as being drastically more toxic than the fluorescent light-treated oil (control), ranging from 4× to upward of 80× more toxic, with similar jumps in toxicity being recorded for the survival and development of the dwarf surf clam (Mulinia lateralis) (Pelletier et al., 1997).
UV-treatment of individual PAHs boasted an exaggerated 10 s of 1,000 s of times more toxic than fluorescently-treated PAHs (control) were tested to be (Pelletier et al., 1997) and is graphically represented in Figure 1. The toxicity of Pyr and BaP reached similar levels after UV-exposure, needing only 5 μg/L of either PAH to cause complete failure in the larval cohort, whereas doses of 1 μg/L of either PAH saw UV-exposure as the difference between having approximately 80% abnormal (UV-exposure) or 80% (no UV-exposure) normal D-shaped larvae (Lyons et al., 2002). In the mussel (Mytilus galloprovincialis), four PAHs: Pyr, Phe, Fla, and chrysene (Chr) were tested (Okay and Karacik, 2008). Phe and Chr were found to be especially photo-toxic as UV exposure yielded an approximately 60–80% drop in filtration rate (Phe only) and neutral red retention (measure of lipid membrane integrity) in the hemocytes. In contrast, neither the feeding rate nor the neutral red retention dropped significantly after UV exposure in both Pyr and Flu (Okay and Karacik, 2008), but it is clear that PAH-accumulation under UV exposure has massive potential for harm compared to simply accumulating PAHs by themselves.
Figure 1. The PAH-induced stress hierarchy. PAHs induce bodily stress above that of normal uncontaminated conditions. Salinity and Temperature may add to or mitigate the stress of PAH-burden. Photo-oxidized PAHs are far more toxic than their parent PAHs, and their increased potential to cause harm is represented by the break in the vertical bar.
The oysters of the genera Crassostrea, Magallana, Ostrea, and Saccostrea play a vital role in our understanding of the distribution and potency of pollutants that result from anthropogenic activities. Oysters are a model bioindicator organism capable of accumulating large concentrations of contaminants while being easy to collect data from, and providing data that is easy to conceptualize. They provide valuable insight into how PAHs affect marine ecosystems. The negative consequences of the bioaccumulation of PAHs are likely to be felt by all species, but it is our choice of bioindicator that reveals this information. Oysters have revealed that PAHs cause oxidative damage, DNA strand breaks, dampened immune systems, reduced fertilization rates, larval abnormalities, changes in body mass, and fierce metabolic and antioxidant responses, while abiotic conditions such as temperature, salinity, pH, and especially UV-light could increase these effects. Across the many studies, some biomarkers such as disease prevalence or antioxidant and detoxification response have some ambiguity in the results, and caution should be applied during their interpretation. All possibilities of exposure duration, stage of response, and confounding factors should be considered carefully. Due to this caveat, it is important to realize that the health problems observed in wild oysters may not necessarily reflect the stressor of interest due to the existence of a myriad of other contaminants and abiotic conditions and their synergistic effects, and the chronic nature of the exposure. The environment is extremely complex and wrought with confounding factors. This makes it nearly impossible to pinpoint the exact cause without a controlled lab test. However, wild oysters will never be exposed to controlled conditions in the wild, and thus, more synergistic effects must be studied. At this time, most synergistic effects of coexposure to changes in abiotic conditions and PAHs are thus far poorly explored, especially with more ambiguous interactions such as between temperature changes and PAH exposure. Further study should take more synergistic effects involving abiotic conditions into consideration, and may consider using less common biomarkers involving histology to observe tissue damage and neoplasia. Alternatively, novel biomarkers could be explored as well, such as the heart rate of oyster cardiac muscle cells and the gene expression of stress-related proteins pioneered by Xu et al. (2018).
NG did the literature review and drafted the manuscript. WX edited and finalized the manuscript. All authors contributed to the article and approved the submitted version.
This manuscript was prepared under the support of the National Science Foundation (1903719). The fund for the open access publication fee is from the Texas A&M University—Corpus Christi.
The authors declare that the research was conducted in the absence of any commercial or financial relationships that could be construed as a potential conflict of interest.
All claims expressed in this article are solely those of the authors and do not necessarily represent those of their affiliated organizations, or those of the publisher, the editors and the reviewers. Any product that may be evaluated in this article, or claim that may be made by its manufacturer, is not guaranteed or endorsed by the publisher.
We would like to thank Leisha Martin at TAMU-Corpus Christi for her assistance in manuscript preparation.
Abalaka, S. E., Enem, S. I., Idoko, I. S., Sani, N. A., Tenuche, O. Z., Ejeh, S. A., et al. (2020). Heavy metals bioaccumulation and health risks with associated histopathological changes in Clarias gariepinus from the kado fish market, abuja, nigeria. J. Health Pollut. 10:200602. doi: 10.5696/2156-9614-10.26.200602
Abdel-Shafy, H. I., and Mansour, M. S. M. (2016). A review on polycyclic aromatic hydrocarbons: source, environmental impact, effect on human health and remediation. Egypt. J. Pet. 25, 107–123. doi: 10.1016/j.ejpe.2015.03.011
Aguirre-Rubí, J. R., Luna-Acosta, A., Etxebarria, N., Soto, M., Espinoza, F., Ahrens, M. J., et al. (2018a). Chemical contamination assessment in mangrove-lined Caribbean coastal systems using the oyster Crassostrea rhizophorae as biomonitor species. Environ. Sci. Pollut. Res. 25, 13396–13415. doi: 10.1007/s11356-017-9159-2
Aguirre-Rubí, J. R., Luna-Acosta, A., Ortiz-Zarragoitia, M., Zaldibar, B., Izagirre, U., Ahrens, M. J., et al. (2018b). Assessment of ecosystem health disturbance in mangrove-lined Caribbean coastal systems using the oyster Crassostrea rhizophorae as sentinel species. Sci. Total Environ. 618, 718–735. doi: 10.1016/j.scitotenv.2017.08.098
Alexander, T. C., Han, F. X., Arslan, Z., and Tchounwou, P. B. (2019). Toxicity of As in Crassostrea virginica (Gmelin, 1791) from the Northern Gulf of Mexico at the presence of Zn and its antioxidant defense mechanisms. Ecotoxicol. Environ. Saf. 172, 514–522. doi: 10.1016/j.ecoenv.2019.02.009
Allmon, E., Serafin, J., Chen, S., Rodgers, M. L., Griffit, R., Bosker, T., et al. (2021). Effects of polycyclic aromatic hydrocarbons and abiotic stressors on Fundulus grandis cardiac transcriptomics. Sci. Total Environ. 752:142156. doi: 10.1016/j.scitotenv.2020.142156
Alzieu, C., Sanjuan, J., Deltreil, J. P., and Borel, M. (1986). Tin contamination in arcachon bay – effects on oyster shell anomalies. Mar. Pollut. Bull. 17, 494–498.
Andersen, S., Grefsrud, E. S., and Harboe, T. (2013). Effect of increased pCO2 level on early shell development in great scallop (Pecten maximus Lamarck) larvae. Biogeosciences 10, 6161–6184. doi: 10.5194/bg-10-6161-2013
Arias, A. H., Souissi, A., Roussin, M., Ouddane, B., and Souissi, S. (2016). Bioaccumulation of PAHs in marine zooplankton: an experimental study in the copepod Pseudodiaptomus marinus. Environ. Earth Sci. 75:691. doi: 10.1007/s12665-016-5472-1
Auffret, M., Duchemin, M., Rousseau, S., Boutet, I., Tanguy, A., Moraga, D., et al. (2004). Monitoring of immunotoxic responses in oysters reared in areas contaminated by the “Erika” oil spill. Aquat. Living Resour. 17, 297–302. doi: 10.1051/alr:2004035
Bado-Nilles, A., Gagnaire, B., Thomas-Guyon, H., Le Floch, S., and Renault, T. (2008). Effects of 16 pure hydrocarbons and two oils on haemocyte and haemolymphatic parameters in the Pacific oyster, Crassostrea gigas (Thunberg). Toxicol. Vitro. 22, 1610–1617. doi: 10.1016/j.tiv.2008.04.011
Barros, P., Sobral, P., Range, P., Chícaro, L., and Matias, D. (2013). Effects of sea-water acidification on fertilization and larval development of the oyster Crassostrea gigas. J. Exp. Mar. Bio. Ecol. 440, 200–206. doi: 10.1016/j.jembe.2012.12.014
Baumard, P., Budzinski, H., Garrigues, P., Dizer, H., and Hansen, P. D. (1999). Polycyclic aromatic hydrocarbons in recent sediments and mussels (Mytilus edulis) from the Western Baltic Sea: occurrence, bioavailability and seasonal variations. Mar. Environ. Res. 47, 17–47. doi: 10.1016/S0141-1136(98)00105-6
Baumard, P., Budzinski, H., Garrigues, P., Raoux, C., Bellocq, J., and Thompson, S. (2001). “Chapter 6. Comparative study of sediment and mussel aromatic compound content in European coastal environments. Relationship with specific biomarkers,” in Biomarkers in Marine Organisms, eds P. Garrigues, H. Barth, C. H. Walker, and J. F. Narbonne (Amsterdam: Elsevier Science), 131–177.
Bebianno, M. J., Lopes, B., Guerra, L., Hoarau, P., and Ferreira, A. M. (2007). Glutathione S-transferases and cytochrome P450 activities in Mytilus galloprovincialis from the South coast of Portugal: effects of abiotic factors. Environ. Int. 33, 550–558.
Beniash, E., Ivanina, A., Lieb, N. S., Kurochkin, I., and Sokolova, I. M. (2010). Elevated level of carbon dioxide affects metabolism and shell formation in oysters Crassostrea virginica. Mar. Ecol. Prog. Ser. 419, 95–108. doi: 10.3354/meps08841
Boening, D. W. (1999). An evaluation of bivalves as biomonitors of heavy metals pollution in marine waters. Environ. Monit. Assess. 55, 459–470. doi: 10.1023/A:1005995217901
Botta, R., Asche, F., Borsum, J. S., and Camp, E. V. (2020). A review of global oyster aquaculture production and consumption. Mar. Policy 117:103952. doi: 10.1016/j.marpol.2020.103952
Boulais, M., Vignier, J., Loh, A. N., Chu, F. L. E., Lay, C. R., Morris, J. M., et al. (2018). Sublethal effects of oil-contaminated sediment to early life stages of the Eastern oyster, Crassostrea virginica. Environ. Pollut. 243, 743–751. doi: 10.1016/j.envpol.2018.09.017
Bustamante, J., Arana, G., de Diego, A., and Madariaga, J. M. (2012a). The use of SPMDs and implanted oysters for monitoring PAHs and PCBs in an aquatic environment in the estuary of Urdaibai (Western Pyrenees). Environ. Eng. Manag. J. 11, 1707–1714. doi: 10.30638/eemj.2012.211
Bustamante, P., Luna-Acosta, A., Clemens, S., Cassi, R., Thomas-Guyon, H., and Warnau, M. (2012b). Bioaccumulation and metabolization of 14C-pyrene by the Pacific oyster Crassostrea gigas exposed via seawater. Chemosphere 87, 938–944. doi: 10.1016/j.chemosphere.2012.01.049
Cao, R., Liu, Y., Wang, Q., Dong, Z., Yang, D., Liu, H., et al. (2018). Seawater acidification aggravated cadmium toxicity in the oyster Crassostrea gigas: metal bioaccumulation, subcellular distribution and multiple physiological responses. Sci. Total Environ. 642, 809–823. doi: 10.1016/j.scitotenv.2018.06.126
Cao, R., Zhang, T., Li, X., Zhao, Y., Wang, Q., and Yang, D. (2019). Seawater acidification increases copper toxicity: a multi-biomarker approach with a key marine invertebrate, the pacific oyster Crassostrea gigas. Aquat. Toxicol. 210, 167–178. doi: 10.1016/j.aquatox.2019.03.002
Chan, C. Y., and Wang, W.-X. (2019). Biomarker responses in oysters Crassostrea hongkongensis in relation to metal contamination patterns in the Pearl River Estuary, southern China. Environ. Pollut. 251, 264–276. doi: 10.1016/j.envpol.2019.04.140
Chiovatto, A. C. L., de Godoi, A. V. O., Zanardi-Lamardo, E., Duarte, F. A., DelValls, T. A., Pereira, C. D. S., et al. (2021). Effects of substances released from a coal tar-based coating used to protect harbor structures on oysters. Mar. Pollut. Bull. 166:112221. doi: 10.1016/j.marpolbul.2021.112221
Chu, F.-L. E., and Hale, R. C. (1994). Relationship between pollution and susceptibility to infectious disease in the eastern oyster, Crassostrea virginica. Mar. Environ. Res. 38, 243–256. doi: 10.1016/0141-1136(94)90026-4
Chu, F.-L. E., Volety, A. K., Hale, R. C., and Huang, Y. (2002). Cellular responses and disease expression in oysters (Crassostrea virginica) exposed to suspended field - contaminated sediments. Mar. Environ. Res. 53, 17–35. doi: 10.1016/S0141-1136(01)00104-0
Cooper, A. J. L., and Hanigan, M. H. (2018). “Metabolism of glutathione s-conjugates: multiple pathways,” in Comprehensive Toxicology, ed. C. A. McQueen (Amsterdam: Elsevier Science), 363–406.
Cortazar, E., Bartolomé, L., Arrasate, S., Usobiaga, A., Raposo, J. C., Zuloaga, O., et al. (2008). Distribution and bioaccumulation of PAHs in the UNESCO protected natural reserve of Urdaibai, Bay of Biscay. Chemosphere 72, 1467–1474. doi: 10.1016/j.chemosphere.2008.05.006
Croom, E. (2012). “Chapter Three – Metabolism of Xenobiotics of Human Environments,” in Progress in Molecular Biology and Translational Science, ed. E. Hodgson (Cambridge, MA: Academic Press), 31–88.
Croxton, A. N., Wikfors, G. H., and Schulterbrandt-Gragg, R. D. (2012). Immunomodulation in eastern oysters, Crassostrea virginica, exposed to a PAH-contaminated, microphytobenthic diatom. Aquat. Toxicol. 118-119, 27–36. doi: 10.1016/j.aquatox.2012.02.023
Cullen, J. A., Marshall, C. D., and Hala, D. (2019). Integration of multi-tissue PAH and PCB burdens with biomarker activity in three coastal shark species from the northwestern Gulf of Mexico. Sci. Total Environ. 650, 1158–1172. doi: 10.1016/j.scitotenv.2018.09.128
DeLorenzo, M. E., Key, P. B., Chung, K. W., Aaby, K., Hausman, D., Jean, C., et al. (2021). Multi-stressor effects of ultraviolet light, temperature, and salinity on Louisiana Sweet crude oil toxicity in larval estuarine organisms. Arch. Environ. Contam. Toxicol. 80, 461–473. doi: 10.1007/s00244-021-00809-3
Donaghy, L., Hong, H.-K., Lee, H.-J., Jun, J.-C., Park, Y.-J., and Choi, K.-S. (2010). Hemocyte parameters of the Pacific oyster Crassostrea gigas a year after the Hebei Spirit oil spill off the west coast of Korea. Helgol. Mar. Res. 64, 349–355. doi: 10.1007/s10152-010-0190-7
dos Reis, I. M. M., Mattos, J. J., Garcez, R. C., Zacchi, F. L., Miguelão, T., Flores-Nunes, F., et al. (2015). Histological responses and localization of the cytochrome P450 (CYP2AU1) in Crassostrea brasiliana exposed to phenanthrene. Aquat. Toxicol. 169, 79–89. doi: 10.1016/j.aquatox.2015.10.011
dos Reis, I. M. M., Siebert, M. N., Zacchi, F. L., Mattos, J. J., Fores-Nunes, F., de Toledo-Silva, G., et al. (2020). Differential responses in the biotransformation systems of the oyster Crassostrea gigas (Thunberg, 1789) elicited by pyrene and fluorene: Molecular, biochemical and histological approach – Part II. Aquat. Toxicol. 226:105565. doi: 10.1016/j.aquatox.2020.105565
Edmiston, H. L., Fahrny, S. A., Lamb, M. S., Levi, L. K., Wanat, J. M., Avant, J. S., et al. (2008). Tropical storm and hurricane impacts on a gulf coast estuary: APALACHICOLA Bay, Florida. J. Coastal. Res. 8, 38–49. doi: 10.2112/SI55-009.1
Ehrenhauser, F. S. (2011). Photochemical reaction products of polycyclic aromatic hydrocarbons adsorbed at an air-water interface. LSU Doctoral Dissertations. Baton Rouge: Louisiana State University, 530.
Eker, G., and Hatipoglu, M. (2019). Effect of UV wavelength, temperature and photocatalyst on the removal of PAHs from industrial soil with photodegradation applications. Environ. Technol. 40, 3793–3803. doi: 10.1080/09593330.2018.1491635
Ertl, N. G., O’Connor, W. A., Brooks, P., Keats, M., and Elizur, A. (2016). Combined exposure to pyrene and fluoranthene and their molecular effects on the Sydney rock oyster, Saccostrea glomerata. Aquat. Toxicol. 177, 136–145. doi: 10.1016/j.aquatox.2016.05.012
Finch, B. E., Stefansson, E. S., Langdon, C. J., Pargee, S. M., Blunt, S. M., Gage, S. J., et al. (2016). Photo-enhanced toxicity of two weathered Macondo crude oils to early life stages of the eastern oyster (Crassostrea virginica). Mar. Pollut. Bull. 113, 316–323. doi: 10.1016/j.marpolbul.2016.10.008
Fisher, W. S., Oliver, L. M., Winstead, J. T., and Long, E. R. (2000). A survey of oysters Crassostrea virginica from Tampa Bay, Florida: associations of internal defense measurements with contaminant burdens. Aquat. Toxicol. 51, 115–138. doi: 10.1016/S0166-445X(00)00082-5
Freitas, R., de Marchi, L., Bastos, M., Moreira, A., Velez, C., Chiesa, S., et al. (2017). Effects of seawater acidification and salinity alterations on metabolic, osmoregulation and oxidative stress markers in Mytilus galloprovincialis. Ecol. Indic. 79, 54–62. doi: 10.1016/j.ecolind.2017.04.003
Freitas, R., Leite, C., Pinto, J., Costa, M., Monteira, R., and Henriques, B. (2019). The influence of temperature and salinity on the impacts of lead in Mytilus galloprovincialis. Chemosphere 235, 403–412. doi: 10.1016/j.chemosphere.2019.05.221
Frieder, C. A., Applebaum, S. L., Pan, T.-C., Hedgecock, D., and Manahan, D. T. (2017). Metabolic cost of calcification in bivalve larvae under experimental ocean acidification. ICES J. Mar. Sci. 74, 941–954. doi: 10.1093/icesjms/fsw213
Galloway, T. S., Brown, R. J., Browne, M. A., Dissanayake, A., Lowe, D., Jones, M. B., et al. (2004). A multibiomarker approach to environmental assessment. Environ. Sci. Technol. 38, 1723–1731. doi: 10.1021/es030570
Gardner, G. R., Yevich, P. P., Harshbarger, J. C., and Malcom, A. R. (1991). Carcinogenicity of Black Rock Harbor sediment to the eastern oyster and trophic transfer of Black Rock Harbor carcinogens from the blue mussel to the winter flounder. Environ. Health Perspect. 90, 53–66. doi: 10.1289/ehp.90-1519497
Geffard, O., Budzinski, H., Augagneur, S., Seaman, M. N. L., and His, E. (2001). Assessment of sediment contamination by spermiotoxicity and embryotoxicity bioassays with sea urchins (Paracentrotus lividus) and oysters (Crassostrea gigas). Environ. Toxicol. Chem. 20, 1605–1611. doi: 10.1002/etc.5620200727
Geffard, O., Budzinski, H., and His, E. (2002). The effects of elutriates from PAH and heavy metal polluted sediments on Crassostrea gigas (Thunberg) embryogenesis, larval growth and bio-accumulation by the larvae of pollutants from sedimentary origin. Ecotoxicology 11, 403–416. doi: 10.1023/A:1021024415695
Geffard, O., Budzinski, H., and His, E. (2004). The effects of decanted sediments on embryogenesis in oysters (Crassostrea gigas). Environ. Toxicol. Chem. 23, 1655–1661. doi: 10.1897/03-206
Geffard, O., Geffard, A., His, E., and Budzinski, H. (2003). Assessment of the bioavailability and toxicity of sediment-associated polycyclic aromatic hydrocarbons and heavy metals applied to Crassostrea gigas embryos and larvae. Mar. Pollut. Bull. 46, 481–490. doi: 10.1016/S0025-326X(02)00451-4
Le Goff, J. (2017). “Carcinogenicity of Petrogenic PAHs,” in Petrogenic Polycyclic Aromatic Hydrocarbons in the Aquatic Environment: Analysis, Synthesis, Toxicity and Environmental Impact, eds D. M. Pampanin and M. O. Sydnes (Sharjah, UAE: Bentham Science Publishing), 65–110.
Gosling, E. (2003). Bivalve Molluscs: Biology, Ecology and Culture. Oxford: Blackwell Publishing Ltd.
Guerra-García, J. M., Navarro-Barranco, C., Martínez-Laiz, G., Moreira, J., Giráldez, I., Morales, E., et al. (2021). Assessing environmental pollution levels in marinas. Sci. Total Environ. 762:144169. doi: 10.1016/j.scitotenv.2020.144169
Hamzah-Chaffai, A. (2014). Usefulness of bioindicators and biomarkers in pollution biomonitoring. Int. J. Biotechnol. Wellness Ind. 3, 19–26. doi: 10.6000/1927-3037.2014.03.01.4
Harney, E., Artigaud, S., Le Souchu, P., Miner, P., Corporeau, C., Essid, H., et al. (2016). Non-additive effects of ocean acidification in combination with warming on the larval proteome of the Pacific oyster, Crassostrea gigas. J. Proteom. 135, 151–161. doi: 10.1016/j.jprot.2015.12.001
Heilmayer, O., Digialleonardo, J., Qian, L., and Roesijadi, G. (2008). Stress tolerance of a subtropical Crassostrea virginica population to the combined effects of temperature and salinity. Estuar. Coast. Shelf Sci. 79, 179–185. doi: 10.1016/j.ecss.2008.03.022
Higuera-Ruiz, R., and Elorza, J. (2011). Shell thickening and chambering in the oyster Crassostrea gigas and anthropogenic influence of tributyltin contamination. Environ. Technol. 32, 583–591. doi: 10.1080/09593330.2010.506201
His, E., Seaman, M. N. L., and Beiras, R. (1997). A simplification the bivalve embryogenesis and larval development bioassay method for water quality assessment. Water Res. 31, 351–355. doi: 10.1016/S0043-1354(96)00244-8
Hong, W.-J., Jia, H., Li, Y.-F., Sun, Y., Liu, X., and Wang, L. (2016). Polycyclic aromatic hydrocarbons (PAHs) and alkylated PAHs in the coastal seawater, surface sediment and oyster from Dalian, Northeast China. Ecotoxicol. Environ. Saf. 128, 11–20. doi: 10.1016/j.ecoenv.2016.02.003
Hwang, H.-M., Wade, T. L., and Sericano, J. L. (2008). Residue-response relationship between PAH body burdens and lysosomal membrane destabilization in eastern oysters (Crassostrea virginica) and toxicokinetics of PAHs. J. Environ. Sci. Health A. 43, 1373–1380. doi: 10.1080/10934520802232006
Huang, W., Hagen, S. C., Bacopoulos, P., and Teng, F. (2014). Sea-level rise effects on hurricane-induced salinity transport in Apalachicola Bay. J. Coastal. Res. 4, 49–56. doi: 10.2112/SI68-007.1
Hutchinson, S., and Hawkins, L. E. (1992). Quantification of the physiological-responses of the european flat oyster ostrea-edulis l to temperature and salinity. J. Mollus. Stud. 58, 215–226. doi: 10.1093/mollus/58.2.215
Iglesias, J. I. P., Urrutia, M. B., Navarro, E., Alvarez-Jorna, P., Larretxea, X., Bougrier, S., et al. (1996). Variability of feeding processes in the cockle Cerastoderma edule (L.) in response to changes in seston concentration and composition. J. Exp. Mar. Biol. Ecol. 197, 121–143. doi: 10.1016/0022-0981(95)00149-2
IPCC. (2013). “Summary for Policymakers,” in Climate Change 2013: The Physical Science Basis, eds T. F. Stocker, D. Qin, G.-K. Plattner, M. Tignor, S. K. Allen, J. Boschung, et al. (Cambridge, UK: Cambridge University Press), 203.
Jackim, E., Morrison, G., and Steele, R. (1977). Effects of environmental factors on radiocadmium uptake by four species of marine bivalves. Mar. Biol. 40, 303–308. doi: 10.1007/BF00395722
Jafari, F., Naeemi, A. S., Noorinezhad, M., and Sohani, M. M. (2021). The effect of global warming and acidification on PAHs bioaccumulation in pearl oyster Pinctada radiate. Res. Square [preprint].
Jeong, W. G., and Cho, S. M. (2005). The effects of polycyclic aromatic hydrocarbon exposure on the fertilization and larval development of the Pacific oyster, Crassostrea gigas. J. Shellfish Res. 24, 209–213.
Jeong, W. G., and Cho, S. M. (2007). Long-term effect of polycyclic aromatic hydrocarbon on physiological metabolisms of the Pacific oyster, Crassostrea gigas. Aquaculture 265, 343–350. doi: 10.1016/j.aquaculture.2007.02.021
Jo, P. G., Choi, Y. K., and Choi, C. Y. (2008). Cloning and mRNA expression of antioxidant enzymes in the Pacific oyster, Crassostrea gigas in response to cadmium exposure. Comp. Biochem. Physiol. C Toxicol. Parmacol. 147, 460–469. doi: 10.1016/j.cbpc.2008.02.001
Karaca, G., and Tasdemir, Y. (2013). Effects of temperature and photocatalysts on removal of polycyclic aromatic hydrocarbons (PAHs) from automotive industry sludge. Polycycl. Aromat. Comp. 33, 380–395. doi: 10.1080/10406638.2013.782880
Kawasaki, T., and Kawai, T. (2014). Toll-like receptor signaling pathways. Front. Immunol. 5:461. doi: 10.3389/fimmu.2014.00461
Kelly, B., Ikonomou, M., Blair, J. D., Morin, A. E., and Gobas, F. (2007). Food web-specific biomagnifican of persistent organic pollutants. Science 317, 236–239. doi: 10.1126/science.1138275
Kurihara, T. (2016). Tolerance of the bivalve Trapezium liratum (Reeve, 1843) to decrease in salinity. Plankton Benthos Res. 12, 44–52. doi: 10.3800/pbr.12.44
La Guardia, M. J., Hale, R. C., Harvey, E., Mainor, T. M., and Ciparis, S. (2012). In situ accumulation of HBCD, PBDEs, and several alternative flame-retardants in the bivalve (Corbicula fluminea) and Gastropod (Elimia proxima). Environ. Sci. Technol. 46, 5798–5805. doi: 10.1021/es3004238
Laflamme, R. E., and Hites, R. A. (1978). The global distribution of polycyclic aromatic hydrocarbons in recent sediments. Geochim. Cosmochim. Acta. 42, 289–303. doi: 10.1016/0016-7037(78)90182-5
Lannig, G., Flores, J. F., and Sokolova, I. M. (2006). Temperature-dependent stress response in oysters, Crassostrea virginica: pollution reduces temperature tolerance in oysters. Aquat. Toxicol. 79, 278–287. doi: 10.1016/j.aquatox.2006.06.017
Larcher, T., Perrichon, P., and Vignet, C. (2014). Chronic dietary exposure of zebrafish to PAH mixtures results in carcinogenic but not genotoxic effects. Environ. Sci. Pollut. Res. 21, 13833–13849. doi: 10.1007/s11356-014-2923-7
Lawlor, J. A., and Arellano, S. M. (2020). Temperature and salinity, not acidification, predict near-future larval growth and larval habitat suitability of Olympia oysters in the Salish Sea. Sci. Rep. 10:13787. doi: 10.1038/s41598-020-69568-w
Lee, R. F. (2003). Photo-oxidation and photo-toxicity of crude and refined oils. Spill. Sci. Tech. Bull. 8, 157–162. doi: 10.1016/S1353-2561(03)00015-X
Leon, V. M., Moreno-González, R., González, E., Martínez, F., García, V., and Campillo, J. A. (2013). Interspecific comparison of polycyclic aromatic hydrocarbons and persistent organochlorines bioaccumulation in bivalves from a Mediterranean coastal lagoon. Sci. Total Environ. 463-464, 975–987. doi: 10.1016/j.scitotenv.2013.06.075
Lima, D., Mattos, J. J., Piazza, R. S., Righetti, B. P. H., Monteiro, J. S., Grott, S. C., et al. (2019). Stress responses in Crassostrea gasar exposed to combined effects of acute pH changes and phenanthrene. Sci. Total Environ. 678, 585–593. doi: 10.1016/j.scitotenv.2019.04.450
Lima, D., Zacchi, F. L., Mattos, J. J., Fores-Nunes, F., de Miranda Gomes, C. H. A., de Mello, A. C. P., et al. (2018). Molecular and cellular effects of temperature in oysters Crassostrea brasiliana exposed to phenanthrene. Chemosphere 209, 307–318. doi: 10.1016/j.chemosphere.2018.06.094
Liu, Q., Xu, X., Zeng, J., Shi, X., Liao, Y., Du, P., et al. (2019). Heavy metal concentrations in commercial marine organisms from Xiangshan Bay, China, and the potential health risks. Mar. Pollut. Bull. 141, 215–226. doi: 10.1016/j.marpolbul.2019.02.058
Luna-Acosta, A., Bustamante, P., Thomas-Guyon, H., Zaldibar, B., Izagirre, U., and Marigómez, I. (2017). Integrative biomarker assessment of the effects of chemically and mechanically dispersed crude oil in Pacific oysters, Crassostrea gigas. Sci. Total Environ. 598, 713–721. doi: 10.1016/j.scitotenv.2017.04.001
Lyons, B. P., Pascoe, C. K., and McFadzen, I. R. B. (2002). Phototoxicity of pyrene and benzo[a]pyrene to embryo-larval stages of the pacific oyster Crassostrea gigas. Mar. Environ. Res. 54, 627–631. doi: 10.1016/s0141-1136(02)00124-1
Maar, M., Saurel, C., Landes, A., Dolmer, P., and Petersen, J. K. (2015). Growth potential of blue mussels (M. edulis) exposed to different salinities evaluated by a Dynamic Energy Budget model. J. Mar. Syst. 148, 48–55. doi: 10.1016/j.jmarsys.2015.02.003
Maathuis, M. A. M., Coolen, J. W. P., van der Have, T., and Kamermans, P. (2020). Factors determining the timing of swarming of European flat oyster (Ostrea edulis L.) larvae in the Dutch Delta area: implications for flat oyster restoration. J. Sea Res. 156:101828. doi: 10.1016/j.seares.2019.101828
Matoo, O. B., Ivanina, A. V., Ullstad, C., Beniash, E., and Sokolova, I. M. (2013). Interactive effects of elevated temperature and CO2 levels on metabolism and oxidative stress in two common marine bivalves (Crassostrea virginica and Mercenaria mercenaria). Comp. Biochem. Physiol. A Mol. Integr. Physiol. 164, 545–553. doi: 10.1016/j.cbpa.2012.12.025
McCarrick, S., Cunha, V., Zapletal, O., Vondracek, J., and Dreij, K. (2019). In vitro and in vivo genotoxicity of oxygenated polycyclic aromatic hydrocarbons. Environ. Pollut. 246, 678–687. doi: 10.1016/j.envpol.2018.12.092
Metian, M., Pouil, S., Dupuy, C., Teyssié, J.-L., Warnau, M., and Bustamante, P. (2020). Influence of food (ciliate and phytoplankton) on the trophic transfer of inorganic and methyl-mercury in the Pacific cupped oyster Crassostrea gigas. Environ. Pollut. 257:113503. doi: 10.1016/j.envpol.2019.113503
Moreira, A., Figuiera, E., Pecora, I. L., Soares, A. M. V. M., and Freitas, R. (2017). Biochemical alterations in native and exotic oyster species in Brazil in response to increasing temperature. Comp. Biochem. Physiol. C Toxicol. Pharmacol. 191, 183–193. doi: 10.1016/j.cbpc.2016.10.008
Moreira, A., Freitas, R., Figueira, E., Ghirardini, A. V., Soares, A. M. V. M., Radaelli, M., et al. (2018). Combined effects of arsenic, salinity and temperature on Crassostrea gigas embryotoxicology. Ecotoxicol. Environ. Saf. 147, 251–259. doi: 10.1016/j.ecoenv.2017.08.043
Munroe, D., Tabatabai, A., Burt, I., Bushek, D., Powell, E. N., and Wilkin, J. (2013). Oyster mortality in Delaware Bay: impacts and recovery from Hurricane Irene and Tropical Storm Lee. Estuar. Coast Shelf Sci. 135, 209–219. doi: 10.1016/j.ecss.2013.10.011
Nash, S., Johnstone, J., and Rahman, M. S. (2019). Elevated temperature attenuates ovarian functions and induces apoptosis and oxidative stress in the American oyster, Crassostrea virginica: potential mechanisms and signaling pathways. Cell Stress Chaparones 24, 957–967. doi: 10.1007/s12192-019-01023-w
Nash, S., and Rahman, M. S. (2019). Short-term heat stress impairs testicular functions in the American oyster, Crassostrea virginica: molecular mechanisms and induction of oxidative stress and apoptosis in spermatogenic cells. Mol. Reprod. Dev. 86, 1444–1458. doi: 10.1002/mrd.23268
National Research Council. (2004). Nonnative Oysters in the Chesapeake Bay. Washington, DC: The National Academies Press.
Noh, J., Kim, H., Lee, C., Yoon, S. J., Chu, S., Kwon, B.-O., et al. (2018). Bioaccumulation of polycyclic aromatic hydrocarbons (PAHs) by the marine clam, Mactra veneriformis, chronically exposed to oil-suspended particulate matter aggregates. Environ. Sci. Technol. 52, 7910–7920. doi: 10.1021/acs.est.7b06692
O’Connor, T. P. (2002). National distribution of chemical concentrations in mussels and oysters in the USA. Mar. Environ. Res. 53, 117–143. doi: 10.1016/S0141-1136(01)00116-7
Okay, O. S., and Karacik, B. (2008). Bioconcentration and phototoxicity of selected PAHs to marine mussel Mytilus galloprovincialis. J. Environ. Sci. Heal. A. 43, 1234–1242. doi: 10.1080/10934520802177763
Oliver, L. M., Fisher, W. S., Winstead, J. T., Hemmer, B. L., and Long, E. R. (2001). Relationships between tissue contaminants and defense-related characteristics of oysters (Crassostrea virginica) from five Florida bays. Aquat. Toxicol. 55, 203–222. doi: 10.1016/s0166-445x(01)00161-8
Oros, D. R., and Ross, J. R. M. (2005). Polycyclic aromatic hydrocarbons in bivalves from the San Francisco estuary: spatial distributions, temporal trends, and sources (1993-2001). Mar. Environ. Res. 60, 466–488. doi: 10.1016/j.marenvres.2005.02.001
Pelletier, M. C., Burgess, R. M., Ho, K. T., Kuhn, A., McKinney, R. A., and Ryba, S. A. (1997). Phototoxicity of individual polycyclic aromatic hydrocarbons and petroleum to marine invertebrate larvae and juveniles. Environ. Toxicol. Chem. 16, 2190–2199. doi: 10.1002/etc.5620161029
Perić, L., Perusco, V. S., and Nerlović, V. (2020). Differential response of biomarkers in the native European flat oyster Ostrea edulis and the non-indigenous Pacific oyster Crassostrea gigas co-exposed to cadmium and copper. J. Exp. Mar. Biol. Ecol. 523:151271. doi: 10.1016/j.jembe.2019.151271
Perrichon, P., Mager, E. M., Pasparakis, C., Stieglitz, J. D., Benetti, D. D., Grosell, M., et al. (2018). Combined effects of elevated temperature and Deepwater Horizon oil exposure on the cardiac performance of larval mahi-mahi, Coryphaena hippurus. PLoS One 13:e0203949. doi: 10.1371/journal.pone.0203949
Pham, L. T., Hoang, T. T. T., Tu, L. C. T., Tran, Y. H. T., Le, B. D., Nguyen, D. V., et al. (2020). Bioaccumulation and health risk assessment of polycyclic aromatic hydrocarbons in oyster (Crassostrea sp.) and gastropod (Cymatium sp.) species from the Can Gio Coastal Wetland in Vietnam. Mar. Freshwater Res. 71, 617–626. doi: 10.1071/MF19055
Pie, H. V., Heyes, A., and Mitchelmore, C. L. (2015). Investigating the use of oil platform marine fouling invertebrates as monitors of oil exposure in the Northern Gulf of Mexico. Sci. Total Environ. 508, 553–565. doi: 10.1016/j.scitotenve.2014.11.050
Pourmozaffar, S., Jahromi, S. T., Rameshi, H., Sadeghi, A., Bagheri, T., Behzadi, S., et al. (2020). The role of salinity in physiological responses of bivalves. Rev. Aquac. 12, 1548–1566. doi: 10.1111/raq.12397
Rai, N., Sjoberg, V., Forsberg, G., Karlsson, S., Olsson, P. E., and Jass, J. (2019). Metal contaminated soil leachates from an art glass factory elicit stress response, alter fatty acid metabolism and reduce lifespan in Caenorhabditis elegans. Sci. Total Environ. 651, 2218–2227. doi: 10.1016/j.scitotenv.2018.10.067
Ramachandran, S. D., Sweezey, M. J., Hodson, P. V., Boudreau, M., Courtenay, S. C., Lee, K., et al. (2006). Influence of salinity and fish species on PAH uptake from dispersed crude oil. Mar. Pollut. Bull. 52, 1182–1189. doi: 10.1016/j.marpolbul.2006.02.009
Ramesh, K., Hu, M. Y., Thomsen, J., Bleich, M., and Melzner, F. (2017). Mussel larvae modify calcifying fluid carbonate chemistry to promote calcification. Nat. Commun. 8:1709. doi: 10.1038/s41467-017-01806-8
Reddy, C. M., Arey, J. S., Seewald, J. S., Sylva, S. P., Lemkau, K. L., Nelson, R. K., et al. (2012). Composition and fate of gas and oil released to the water column during the Deepwater Horizon oil spill. Proc. Natl. Acad. Sci. U.S.A. 109, 20229–20234. doi: 10.1073/pnas.1101242108
Ren, J. S., Ragg, N. L. C., Cummings, V. J., and Zhang, J. (2020). Ocean acidification and dynamic energy budget models: Parameterisation and simulations for the green-lipped mussel. Eco. Model. 426:109069. doi: 10.1016/j.ecolmodel.2020.109069
Rittschof, D., and McClellan-Green, P. (2005). Molluscs as multidisciplinary models in environment toxicology. Mar. Pollut. Bull. 50, 369–373. doi: 10.1016/j.marpolbul.2005.02.008
Robert, R., His, E., and Dinet, A. (1988). Combined effects of temperature and salinity on fed and starved larvae of the European flat oyster Ostrea edulis. Mar. Biol. 97, 95–100. doi: 10.1007/BF00391249
Sahu, S. K., and Pandit, G. G. (2003). Estimation of octanol-water partition coefficients for polycyclic aromatic hydrocarbons using reverse-phase HPLC. J. Liq. Chrom. Relat. Tech. 26, 135–146. doi: 10.1081/JLC-120017158
Sangster, J. (1989). Octanol-water partition coefficients of simple organic compounds. Phys. Chem. Ref. Data. 18:1111. doi: 10.1063/1.555833
Sarkar, A., Bhagat, J., Sarker, M. S., Gaitonde, D. C. S., and Sarker, S. (2017). Evaluation of the impact of bioaccumulation of PAH from the marine environment on DNA integrity and oxidative stress in marine rock oyster (Saccostrea cucullata) along the Arabian sea coast. Ecotoxicology 26, 1105–1116. doi: 10.1007/s10646-017-1837-9
Sarker, S., Vashistha, D., Sarker, M. S., and Sarkar, A. (2018). DNA damage in marine rock oyster (Saccostrea cucullata) exposed to environmentally available PAHs and heavy metals along the Arabian Sea coast. Ecotoxcol. Environ. Saf. 151, 132–143. doi: 10.1016/j.ecoenv.2018.01.004
Scanes, E., Parker, L. M., O’Connor, W. A., Gibbs, M. C., and Ross, P. M. (2018). Copper and ocean acidification interact to lower maternal investment, but have little effect on adult physiology of the Sydney rock oyster Saccostrea glomerata. Aquat. Toxicol. 203, 51–60. doi: 10.1016/j.aquatox.2018.07.020
Schrandt, M., Powers, S., Rikard, F. S., Thongda, W., and Peatman, E. (2018). Short-term low salinity mitigates effects of oil and dispersant on juvenile eastern oysters: A laboratory experiment with implications for oil spill response activities. PLoS One. 13:e0203485. doi: 10.1371/journal.pone.0203485
Serafin, J., Guffey, S. C., Bosker, T., Griffit, R. J., de Guise, S., Perkins, C., et al. (2019). Combined effects of salinity temperature, hypoxia, and Deepwater Horizon oil on Fundulus grandis larvae. Ecotoxicol. Environ. Saf. 181, 106–113. doi: 10.1016/j.ecoenv.2019.05.059
Sezer, N., Kiliç, Ö, Metian, M., and Belivermiş, M. (2018). Effects of ocean acidification on 109Cd, 57Co, and 134Cs bioconcentration by the European oyster (Ostrea edulis): Biokinetics and tissue-to-subcellular partitioning. J. Environ. Radioact. 192, 376–384. doi: 10.1016/j.jenvrad.2018.07.011
Shao, Z., and Wang, W.-X. (2020). Biodynamics of silver nanoparticles in an estuarine oyster revealed by 110mAgNP tracing. Environ. Sci. Technol. 54, 965–974. doi: 10.1021/acs.est.9b04241
Shatkin, G., Shumway, S. E., and Hawes, R. (1997). Considerations regarding the possible introduction of the Pacific oyster, Crassostrea gigas, to the Gulf of Maine: a review of global experience. J. Shellfish Res. 16, 463–477.
Shenai-Tirodkar, P. S., Gauns, M. U., Mujawar, M. W. A., and Ansari, Z. A. (2017). Antioxidant responses in gills and digestive gland of oyster Crassostrea madrasensis (Preston) under lead exposure. Ecotoxicol. Environ. Saf. 142, 87–94. doi: 10.1016/j.ecoenv.2017.03.056
Shukla, P., Gopalani, M., Ramteke, D. S., and Wate, S. R. (2007). Influence of salinity on PAH uptake from water soluble fraction of crude oil in Tilapia mossambica. Bull. Environ. Contam. Toxicol. 79, 601–605. doi: 10.1007/s00128-007-9272-x
Simning, D., Sepulveda, M., de Gulse, S., Bosker, T., and Griffitt, R. J. (2019). The combined effects of salinity, hypoxia, and oil exposure on survival and gene expression in developing sheepshead minnows, Cyprinodon variegatus. Aquat. Toxicol. 214:105234. doi: 10.1016/j.aquatox.2019.105234
Su, W., Zha, S., Wang, Y., Shi, W., Xiao, G., Chai, X., et al. (2017). Benzo[a]pyrene exposure under future ocean acidification scenarios weakens the immune responses of blood clam, Telligarca granosa. Fish Shellfish Immunol. 63, 465–470. doi: 10.1016/j.fsi.2017.02.046
Timmins-Schiffman, E., O’Donnell, M. J., Friedman, C. S., and Roberts, S. B. (2013). Elevated pCO2 causes developmental delay in early larval pacific oysters, Crassostrea gigas. Mar. Biol. 160, 1973–1982. doi: 10.1007/s00227-012-2055-x
Tongo, I., Ogbeide, O., and Ezemonye, L. (2017). Human health risk assessment of polycyclic aromatic hydrocarbons (PAHs) in smoked fish species from markets in Southern Nigeria. Toxicol. Rep. 4, 55–61. doi: 10.1016/j.toxrep.2016.12.006
Trevisan, R., Flores-Nunes, F., Dolores, E. S., Mattos, J. J., Piazza, C. E., Sasaki, S. T., et al. (2016). Thiol oxidation of hemolymph proteins in oysters Crassostrea brasiliana as markers of oxidative damage induced by urvan sewage exposure. Environ. Toxicol. Chem. 36, 1833–1845. doi: 10.1002/etc.3543
Troost, K. (2010). Causes and effects of a highly successful marine invasion: Case-study of the introduced Pacific oyster Crassostrea gigas in continental NW European estuaries. J. Sea Res. 64, 145–165. doi: 10.1016/j.seares.2010.02.004
Vaezzadeh, V., Zakaria, M. P., Bong, C. W., Masood, N., Magam, S. M., and Alkhadher, S. (2019). Mangrove oyster (Crassostrea belcheri) as a biomonitor species for bioavailability of polycyclic aromatic hydrocarbons (PAHs) from sediment of the west coast of peninsular Malaysia. Polycycl. Aromat. Compd. 39, 470–485. doi: 10.1080/10406638.2017.1348366
Vaschenko, M. A., Hsieh, H.-L., and Radashevsky, V. I. (2013). Gonadal state of the oyster Crassostrea angulata cultivated in Taiwan. J. Shellfish Res. 32, 471–482. doi: 10.2983/035.032.0227
Velez, C., Figueira, E., Soares, A. M. V. M., and Freitas, R. (2016). Combined effects of seawater acidification and salinity changes in Ruditapes philippinarum. Aquat. Toxicol. 176, 141–150. doi: 10.1016/j.aquatox.2016.04.016
Vignier, J., Donaghy, L., Soudant, P., Chu, F. L. E., Morris, J. M., Carney, M. W., et al. (2015). Impacts of Deepwater Horizon oil and associated dispersant on early development of the eastern oyster Crassostrea virginica. Mar. Pollut. Bull. 100, 426–437. doi: 10.1016/j.marpolbul.2015.08.011
Vignier, J., Rolton, A., Soudant, P., Chu, F. L. E., Robert, R., and Volety, A. K. (2018). Evaluation of toxicity of Deepwater Horizon slick oil on spat of the oyster Crassostrea virginica. Environ. Sci. Pollut. Res. 25, 1176–1190. doi: 10.1007/s11356-017-0476-2
Vignier, J., Rolton, A., Soudant, P., Chu, F. L. E., Robert, R., and Volety, A. K. (2019). Interactions between Crassostrea virginica larvae and Deepwater Horizon oil: toxic effects via dietary exposure. Environ. Pollut. 246, 544–551. doi: 10.1016/j.envpol.2018.12.057
Vignier, J., Soudant, P., Chu, F. L. E., Morris, J. M., Carney, M. W., Lay, C. R., et al. (2016). Lethal and sub-lethal effects of deepwater horizon slick oil and dispersant on oyster (crassostrea virginica) larvae. Mar. Environ. Res. 120, 20–31. doi: 10.1016/j.marenvres.2016.07.006
Volety, A., Boulais, M., Donaghy, L., Vignier, J., Loh, A. N., and Soudant, P. (2016). Application of flow cytometry to assess deepwater horizon oil toxicity on the eastern oyster Crassostrea virginica spermatozoa. J. Shellfish Res. 35, 91–99. doi: 10.2983/035.035.0100
Wallace, R. K. (2001). Cultivating the eastern oyster, Crassostrea virginica. Stoneville, MS: SRAC Publication, 432.
Wang, F., Gómez-Sintes, R., and Boya, P. (2018). Lysosomal membrane permeabilization and cell death. Traffic. 19, 918–931. doi: 10.1111/tra.12613
Wang, H., Huang, W., Gong, Y., Chen, C., Zhang, T., and Diao, X. (2020). Occurrence and potential health risks assessment of polycyclic aromatic hydrocarbons (PAHs) in different tissues of bivalves from Hainan Island, China. Food Chem. Toxicol. 136:111108. doi: 10.1016/j.fct.2019.111108
Wang, W. X., and Lu, G. Y. (2017). “Heavy metals in bivalve mollusks,” in Chemical Contaminants and Residues in Food, 2nd Edn, eds A. Cartus and D. Schrenk (Sawston: Woodhead Publishing), 553–594.
Wang, Z., Fingas, M., Shu, Y. Y., Sigouin, L., Landriault, M., Lambert, P., et al. (1999). Quantitative characterization of PAHs in burn residue and soot samples and differentiation of pyrogenic PAHs from petrogenic PAHs - The 1994 Mobile Burn Study. Environ. Sci. Technol. 33, 3100–3109. doi: 10.1021/es990031y
Wilson, E. A., Powell, E. N., Craig, M. A., Wade, T. L., and Brooks, J. M. (1990). The distribution of Perkinsus marinus in Gulf Coast oysters: its relationship with temperature, reproduction, and pollutant body burden. Int. Revue. Ges. Hydrobiol. 75, 533–550. doi: 10.1002/iroh.19900750408
Wiltshire, K. H. (2007). Ecophysiological tolerances of the Pacific oyster, Crassostrea gigas, with regard to the potential spread of populations in South Australian waters. West Beach, SA: SARDI Aquatic Sciences.
Xie, J., Zhao, C., Han, Q., Zhou, H., Li, Q., and Diao, X. (2017). Effects of pyrene exposure on immune response and oxidative stress in the pearl oyster, Pinctada martensii. Fish Shellfish Immunol. 63, 237–244. doi: 10.1016/j.fsi.2017.02.032
Xu, W., Vebrosky, E. N., Richards, M. L., and Armbrust, K. L. (2018). Evaluation of dichloran phototoxicity using primary cardiomyocyte culture from Crassostrea virginica. Sci. Total Environ. 628-629, 1–10. doi: 10.1016/j.scitotenv.2018.02.022
Yang, C.-Y., Sierp, M. T., Abbott, C. A., Li, Y., and Qin, J. G. (2016). Responses to thermal and salinity stress in wild and farmed Pacific oysters Crassostrea gigas. Comp. Biochem. Physiol. A. 201, 22–29. doi: 10.1016/j.cbpa.2016.06.024
Yu, H. Y., Liu, Y. F., Han, C. X., Fang, H., Weng, J. H., Shu, X. Q., et al. (2021). Polycyclic aromatic hydrocarbons in surface waters from the seven main river basins of China: Spatial distribution, source apportionment, and potential risk assessment. Sci. Total Environ. 752:141764. doi: 10.1016/j.scitotenv.2020.141764
Zacchi, F. L., de Lima, D., Flores-Nunes, F., Mattos, J. J., Luchmann, K. H., Gomes, C. H. A. D. M., et al. (2017). Transcriptional changes in oysters Crassostrea brasiliana exposed to phenanthrene at different salinities. Aquat. Toxicol. 183, 94–103. doi: 10.1016/j.aquatox.2016.12.016
Zacchi, F. L., dos Reis, I. M. M., Siebert, M. N., Mattos, J. J., Flores-Nunes, F., de Toledo-Silva, G., et al. (2019). Differential responses in the biotransformation systems of the oyster Crassostrea gasar (Adanson, 1757) elicited by pyrene and fluorene: molecular, biochemical and histological approach – Part I. Aquat. Toxicol. 216:105318. doi: 10.1016/j.aquatox.2019.105318
Zhao, L., Milano, S., Walliser, E. O., and Schöne, B. R. (2018). Bivalve shell formation in a naturally CO2-enriched habitat: unraveling the resilience mechanisms from elemental signatures. Chemosphere 203, 132–138. doi: 10.1016/j.chemosphere.2018.03.180
Keywords: polycyclic aromatic hydrocarbons, oyster, bioaccumulation, environmental impact, host response
Citation: Gan N, Martin L and Xu W (2021) Impact of Polycyclic Aromatic Hydrocarbon Accumulation on Oyster Health. Front. Physiol. 12:734463. doi: 10.3389/fphys.2021.734463
Received: 01 July 2021; Accepted: 03 August 2021;
Published: 10 September 2021.
Edited by:
Jianmin Zhao, Yantai Institute of Coastal Zone Research, Chinese Academy of Sciences (CAS), ChinaReviewed by:
Linbao Zhang, Chinese Academy of Fishery Sciences (CAFS), ChinaCopyright © 2021 Gan, Martin and Xu. This is an open-access article distributed under the terms of the Creative Commons Attribution License (CC BY). The use, distribution or reproduction in other forums is permitted, provided the original author(s) and the copyright owner(s) are credited and that the original publication in this journal is cited, in accordance with accepted academic practice. No use, distribution or reproduction is permitted which does not comply with these terms.
*Correspondence: Wei Xu, d2VpLnh1QHRhbXVjYy5lZHU=
Disclaimer: All claims expressed in this article are solely those of the authors and do not necessarily represent those of their affiliated organizations, or those of the publisher, the editors and the reviewers. Any product that may be evaluated in this article or claim that may be made by its manufacturer is not guaranteed or endorsed by the publisher.
Research integrity at Frontiers
Learn more about the work of our research integrity team to safeguard the quality of each article we publish.