- Department of Physiology and Biomedical Engineering, Mayo Clinic, Rochester, MN, United States
Previously, we reported that in airway smooth muscle (ASM), the cytosolic Ca2+ ([Ca2+]cyt) and force response induced by acetyl choline (ACh) are increased by exposure to the pro-inflammatory cytokine tumor necrosis factor α (TNFα). The increase in ASM force induced by TNFα was not associated with an increase in regulatory myosin light chain (rMLC20) phosphorylation but was associated with an increase in contractile protein (actin and myosin) concentration and an enhancement of Ca2+ dependent actin polymerization. The sensitivity of ASM force generation to elevated [Ca2+]cyt (Ca2+ sensitivity) is dynamic involving both the shorter-term canonical calmodulin-myosin light chain kinase (MLCK) signaling cascade that regulates rMLC20 phosphorylation and cross-bridge recruitment as well as the longer-term regulation of actin polymerization that regulates contractile unit recruitment and actin tethering to the cortical cytoskeleton. In this study, we simultaneously measured [Ca2+]cyt and force responses to ACh and explored the impact of 24-h TNFα on the dynamic relationship between [Ca2+]cyt and force responses. The temporal delay between the onset of [Ca2+]cyt and force responses was not affected by TNFα. Similarly, the rates of rise of [Ca2+]cyt and force responses were not affected by TNFα. The absence of an impact of TNFα on the short delay relationships between [Ca2+]cyt and force was consistent with the absence of an effect of [Ca2+]cyt and force on rMLC20 phosphorylation. However, the integral of the phase-loop plot of [Ca2+]cyt and force increased with TNFα, consistent with an impact on actin polymerization and, contractile unit recruitment and actin tethering to the cortical cytoskeleton.
Introduction
Agonists such as acetyl choline (ACh) induce a cytosolic Ca2+ ([Ca2+]cyt) and force response in airway smooth muscle (ASM). Previously, we and others showed that the regulation of [Ca2+]cyt in response to ACh stimulation is dynamic as reflected by small amplitude localized [Ca2+]cyt transients (Ca2+ sparks) that fuse into larger amplitude [Ca2+]cyt oscillations that propagate through the cell and summate into larger global responses (Prakash et al., 1997; Pabelick et al., 1999; Sieck et al., 2001). As ACh stimulation increases, the amplitudes of localized [Ca2+]cyt oscillations are not affected, but the frequency and propagation velocity of [Ca2+]cyt oscillations increase leading to greater summation into a larger global [Ca2+]cyt response in ASM cells. The coupling of [Ca2+]cyt to a contractile response is also dynamic with initial force responses to elevated [Ca2+]cyt being delayed by 500–800 ms in porcine ASM (Prakash et al., 1997; Pabelick et al., 1999; Sieck et al., 2001). Furthermore, a maximum steady state force response in ASM is not achieved until 2–4 min after elevation of [Ca2+]cyt. Thus, the dynamic sensitivity of force to elevated [Ca2+]cyt is complex involving both short-term and long-term responses (Figure 1).
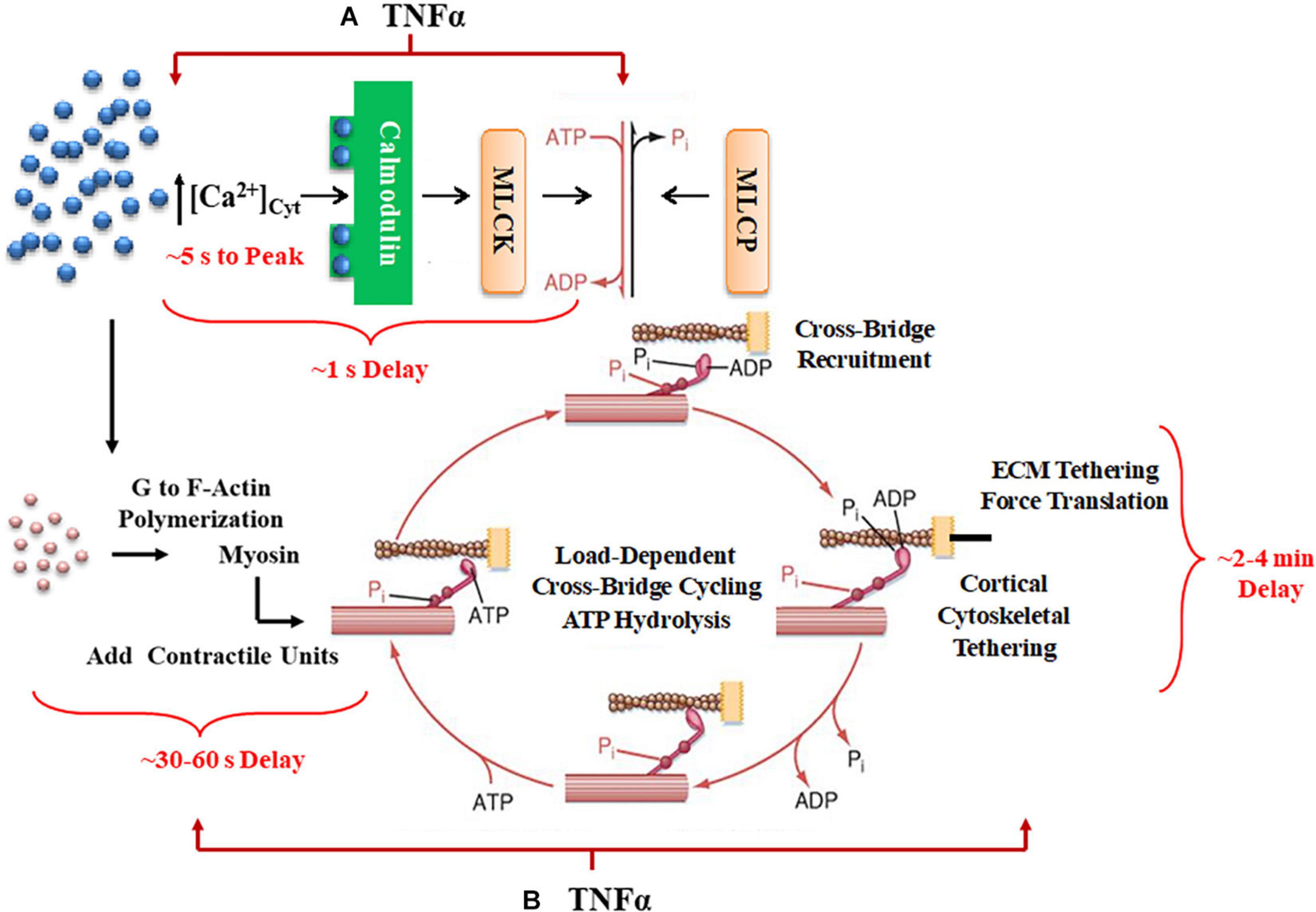
Figure 1. A summary schematic illustrates how TNFα would affect not only short-term time delays reflecting rMLC20 phosphorylation (A) but also would influence longer-term delays in contractile unit recruitment and internal loading in ASM. (B) Activation of ligand-gated or voltage-gated receptors channels by an agonist gives rise to an increase in [Ca2+]cyt concentration. The increased Ca2+ binds to calmodulin (CaM) and then this Ca2+-CaM activates myosin light chain kinase (MLCK) phosphorylating rMLC20, which in turn allows cross-bridge (actin interaction with myosin) recruitment. When cross-bridges are formed, force is generated and transferred to the sarcolemma through the cortical cytoskeletal (actin filaments, dense bodies, and intermediate filaments) that links the contractile units to the plasma membrane. In turn, the plasma membrane of ASM cells attaches to the extracellular matrix (ECM). Cross-bridge cycling is driven by the hydrolysis of ATP. The increase in [Ca2+]cyt concentration also enhances G to F-actin polymerization which contributes to the number of contractile units. Myosin light chain phosphatase (MLCP) dephosphorylates rMLC20, which is believed to play a role in modulating the Ca2+ sensitivity of force generation in ASM.
In smooth muscle, the initial (short-term) coupling of elevated [Ca2+]cyt to a contractile response involves Ca2+ binding to calmodulin (CaM), Ca2+-CaM activating myosin light chain kinase (MLCK), which phosphorylates the regulatory myosin light chain (rMLC20) allowing cross-bridge recruitment and cycling (de Lanerolle and Paul, 1991; Zimmermann et al., 1995; Sieck et al., 2001; Dimopoulos et al., 2007). The level of rMLC20 phosphorylation is also regulated by dephosphorylation via MLC phosphatase (Figure 1). Generally, the sensitivity of the force response to [Ca2+]cyt is thought to be influenced primarily by RhoA signaling through an effect on rMLC20 dephosphorylation (Zhang et al., 2012; Seow and An, 2020). The temporal delay in this canonical signaling cascade is ∼500–800 ms in porcine ASM (Sieck et al., 2001); however, the time required to reach the peak [Ca2+]cyt is ∼ 5 s, and the time to reach the maximum level of rMLC20 phosphorylation is ∼30 s (Zhang et al., 2012).
Force generation in ASM is also affected by mobilization of de novo contractile units via polymerization of actin and myosin filaments and by internal and external loading of cross-bridges (Figure 1). In a previous study, we reported that 24-h exposure of porcine ASM to tumor necrosis factor α (TNFα) increases force generation in response to ACh stimulation by increasing the number of contractile units (actin and myosin concentration) (Dogan et al., 2017). However, TNFα exposure did not affect the extent of rMLC20 phosphorylation. The dynamic nature of force in ASM is also influenced by internal loading of cross-bridges due to the tethering of filamentous actin to the cortical cytoskeleton. As actin filaments are tethered, internal loading increases and cross-bridge cycling rate decreases as reflected by a slowing in the rate of ATP hydrolysis. This tethering process in ASM appears to be much slower with a steady state of ATP hydrolysis reached only after ∼5 min (Delmotte et al., 2020). Thus, the dynamic relationships between [Ca2+]cyt and force responses to ACh stimulation provide insight into the influence of inflammation as mediated by TNFα.
In the present study, we simultaneously measured [Ca2+]cyt and force responses to ACh stimulation in porcine ASM to determine the impact of 24-h TNFα exposure. The dynamic Ca2+ sensitivity of force generation in ASM was assessed using phase-loop plots of [Ca2+]cyt and force responses as previously in ASM (Han et al., 2021) and cardiomyocytes (Spurgeon et al., 1992; Schaible et al., 2016, 2018; Han et al., 2018). We hypothesized that TNFα exposure does not impact short-term time delays reflecting rMLC20 phosphorylation but does affect longer-term delays in contractile unit recruitment and internal loading in ASM.
Materials and Methods
Porcine Airway Smooth Muscle Preparation
Porcine tracheas from both female and male pigs were obtained from a local abattoir, with exemption from Institutional Animal Care and Use Committee (IACUC) approval. During transport to the lab, tracheas were kept in ice-cold physiologic saline solution (composition in mM: 118.99 NaCl, 1.17 MgSO4, 1.18 KH2PO4, 4.7 KCl, 2.5 CaCl2, 0.03 EDTA, 5.5 dextrose, 25 HEPES, and pH 7.4). The ASM layer from each trachea was dissected under a binocular microscope, and eight strips (∼0.8 × 4 mM) were cut as previously described (Fredberg et al., 1996; Sieck et al., 1998, 2001, 2019; Jones et al., 1999a; Dogan et al., 2017). The ASM strips were separated into two groups: one group exposed to TNFα (100 ng/ml; Cell Sciences, MA, United States) for 24 h at room temperature and a second group exposed to physiological saline for the same period (control). Four of the ASM strips (two TNFα treated and two controls) were used for duplicate measurements of simultaneous [Ca2+]cyt and force responses to ACh stimulation (EC50; 2.6 μM for control and 1.3 μM for TNFα). The second set of four ASM strips (two TNFα treated and two controls) were used to examine ACh-induced changes in rMLC20 phosphorylation.
Simultaneous Measurements of [Ca2+]cyt and Force Responses to ACh Stimulation
To measure [Ca2+]cyt, the ASM strips were incubated for 4 h (at 25°C) in physiological saline solution containing the acetoxymethyl ester of fura-2 (fura-2 AM; 5 μM) dissolved in dimethyl sulfoxide (DMSO) as well as 0.02% Pluronic (F-127; Molecular Probes) to facilitate efficient cytosolic loading of fura-2 AM. The ASM strips were then mounted using stainless steel microforceps between a micropositioner (Mitutoyo America Corp., Aurora, IL, United States) to adjust muscle length and an isometric force transducer (KG4; Scientific Instruments, GmbH, Heidelberg, Germany) in a 0.2-ml quartz cuvette (Guth Muscle Research System; Scientific Instruments GmbH, Heidelberg, Germany) (Guth and Wojciechowski, 1986; Sieck et al., 2019) that was continuously perfused with physiological saline solution aerated with 95% O2 and 5% CO2. The ASM strips were perfused for 30 min to wash out any excess fura-2 AM. The length of the ASM strip was then adjusted over a 1-h period to achieve a sustained preload force of 10 mN.
Fura-2 fluorescence ion the ASM strip was excited using a mercury high-pressure lamp (75 W) as a light source at alternating (every 2 ms) wavelengths of 340 and 380 nm restricted by using a rotating filter wheel as previously described (Lorenz et al., 1999; Han et al., 2010). Emitted fluorescence was detected at 510 nm (restricted using a bandpass filter) using a photomultiplier tube. The ratio of emitted fluorescence at 340 and 380 nm excitation wavelengths (F340/F380) was used to determine [Ca2+]cyt based on a calibration equation described by Grynkiewicz et al. (1985); Todoroki-Ikeda et al. (2000), Han et al. (2010), and Sieck et al., 2019. Duplicate measurements of isometric [Ca2+]cyt and force responses of ASM strips to ACh concentration (EC50) were digitized (1 kHz sampling rate) using LabChart8 (AD Instruments, Colorado Springs, CO, United States). The duplicate measurements were subsequently averaged for each trachea.
Measurement of rMLC20 Phosphorylation in Response to ACh Stimulation
As previously described (Sieck et al., 2019), rMLC20 phosphorylation was detected using a Phos-tagTM sodium dodecyl sulfate-polyacrylamide (SDS-PAGE) gel with Zn2+ (Takeya et al., 2008; Walsh et al., 2011; Sieck et al., 2019; Han et al., 2021) (Wako Chemicals Inc., Richmond, VA, United States) and standard western blotting. In each of the TNFα treated and control groups, one ASM strip was not exposed to ACh and was thus used to determine the baseline level of rMLC20 phosphorylation. The second ASM strip in each group was stimulated with ACh concentration (EC50) for 30 s, a time period previously found to induce maximum rMLC20 phosphorylation (Parkman et al., 2001). The ASM strips were then flash-frozen in 10% trichloroacetic acid/10 mM dithiothreitol in pre-chilled acetone. Electrophoresis of the Phos-tagTM gels was performed at 20 mA for 1 h 50 min in running buffer (pH 7.4; 100 mM Tris-base, 100 mM 3-morpholinopropane-1-sulfonic acid, 0.1% SDS, 5 mM Sodium Bisulfate) at the room temperature. The gel was immersed in 25 mM Tris, 192 mM Glycine, 10% methanol (v/v) containing 10 mM EDTA to remove Zn2+ for 30 min and proteins were transferred to polyvinylidene difluoride membrane, which was fixed with 0.5% formaldehyde in PBS for 45 min (Takeya et al., 2008) and blocked with 5% dry milk in TBST. Using a standard western blotting technique, the membrane was incubated with primary (1:2000 dilution, rabbit polyclonal anti-rMLC20; sc-15370; Santa Cruz, CA, United States) and secondary antibody (1:10,000 dilution, goat anti-rabbit IgG-HRP conjugate; Santa Cruz, CA, United States). Unphosphorylated rMLC20 and phosphorylated rMLC20 (p-rMLC20) were detected by enhanced chemiluminescence (SuperSignal West Dura Extended Duration Substrate; Thermo Fisher Scientific, Rockford, IL, United States) and imaged on ChemiDoc MP Image System (Bio-Rad Laboratories, Hercules, CA, United States) and analyzed using an Image-Lab-Software (version 6.0.1, Bio-Rad Laboratories, Hercules, CA, United States) (Figure 5C).
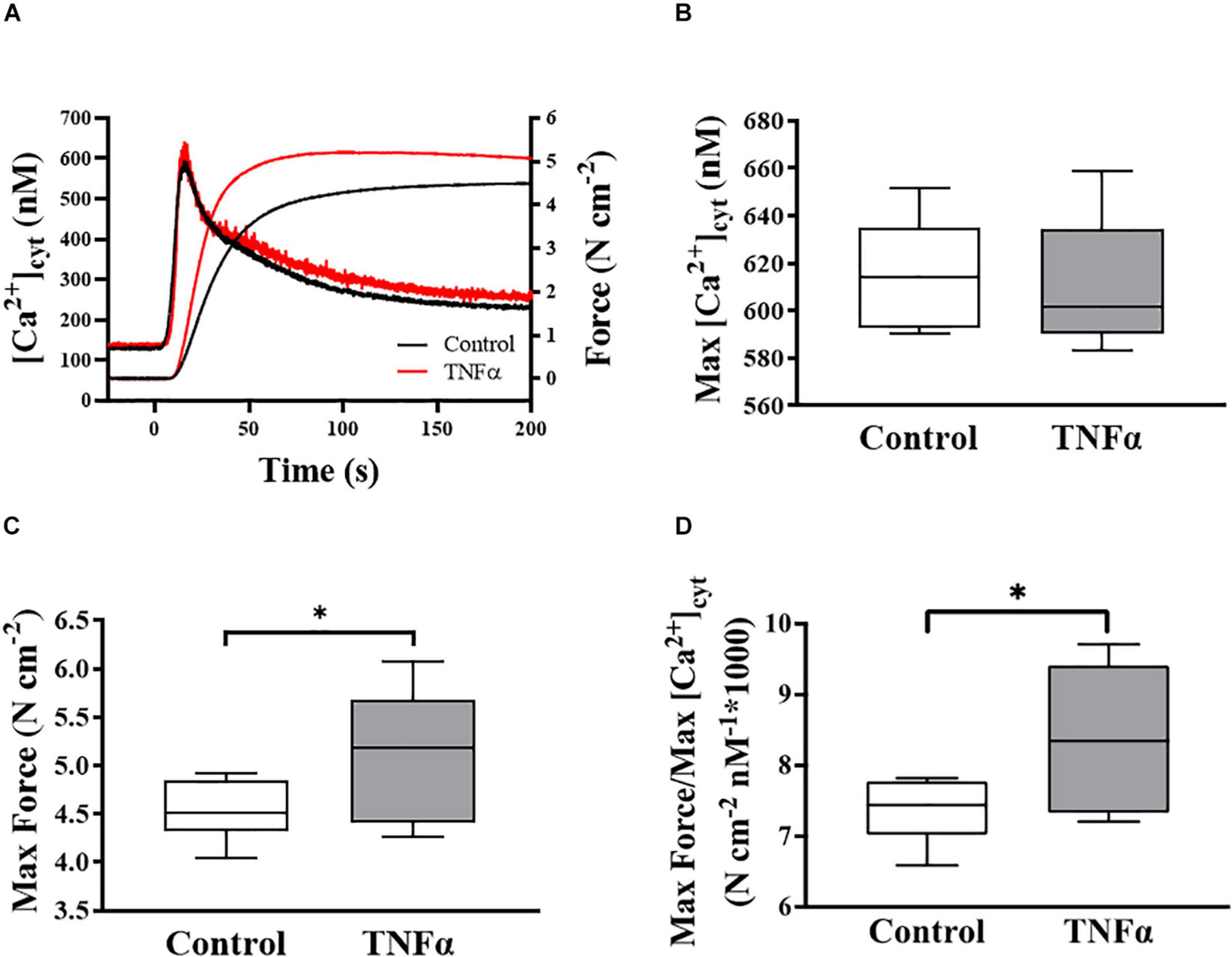
Figure 2. Representative tracings of simultaneous measurements of [Ca2+]cyt and force responses of ASM to ACh stimulation (EC50) (A). Maximum specific force (C) and static Ca2+ sensitivity of ASM significantly increased (D) with TNFα exposure, while there was no significant change in maximum [Ca2+]cyt (B) (p > 0.05), compared to controls [summarized as medians and interquartile range (IQR)]. Data were analyzed using a one-way repeated measure ANOVA. Significance was considered at p < 0.05 [∗compared with TNFα; n = 6 (number of animals)].
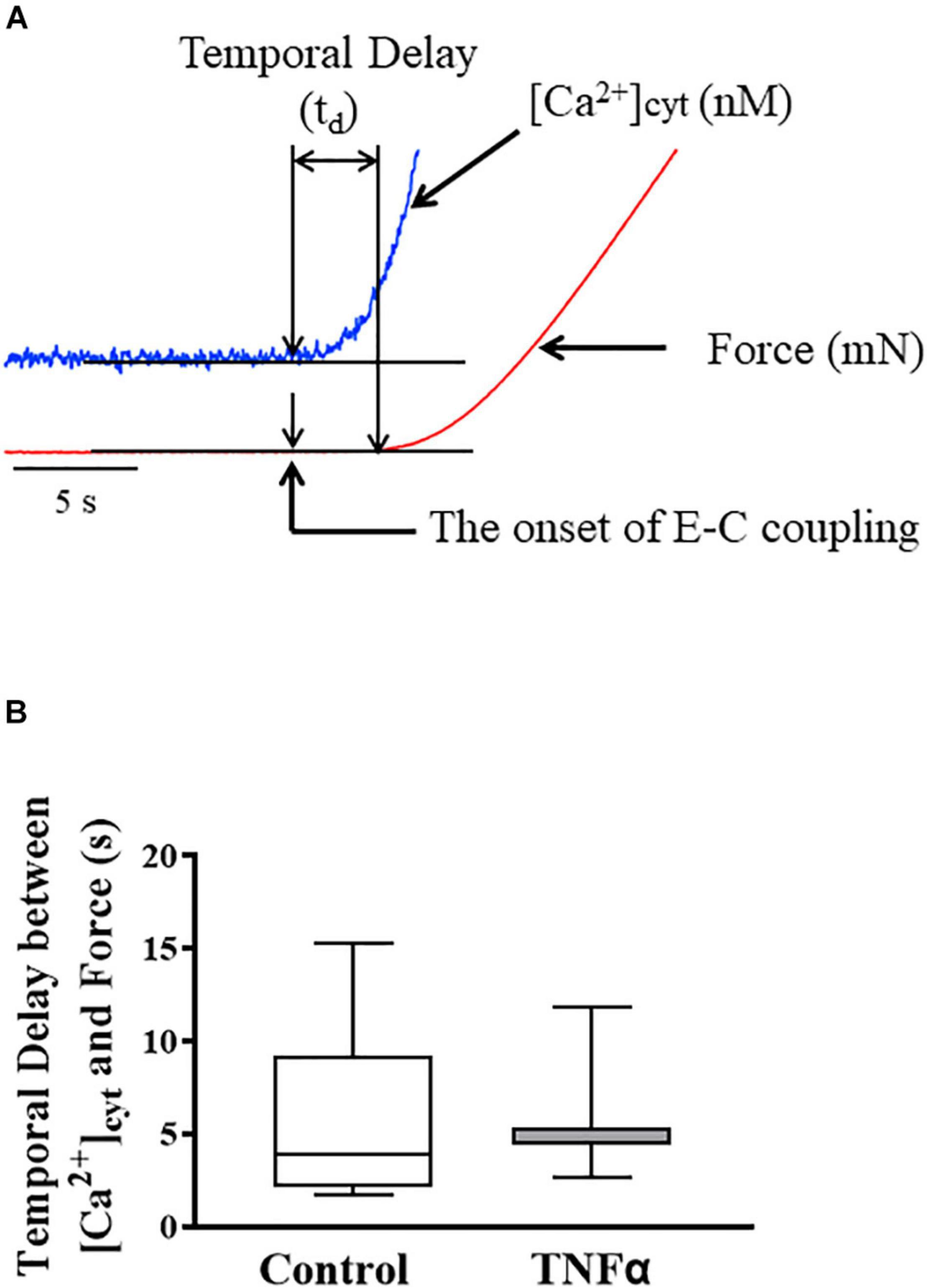
Figure 3. Representative tracings of [Ca2+]cyt (blue) and force (red) response of ASM to ACh stimulation (A). The temporal delay (td) between the onset of [Ca2+]cyt and force responses was not dependent on TNFα (B). A one-way repeated measure ANOVA was used to analyze the results; p > 0.05, n = 6 (number of animals) and summarized as medians and interquartile range (IQR).
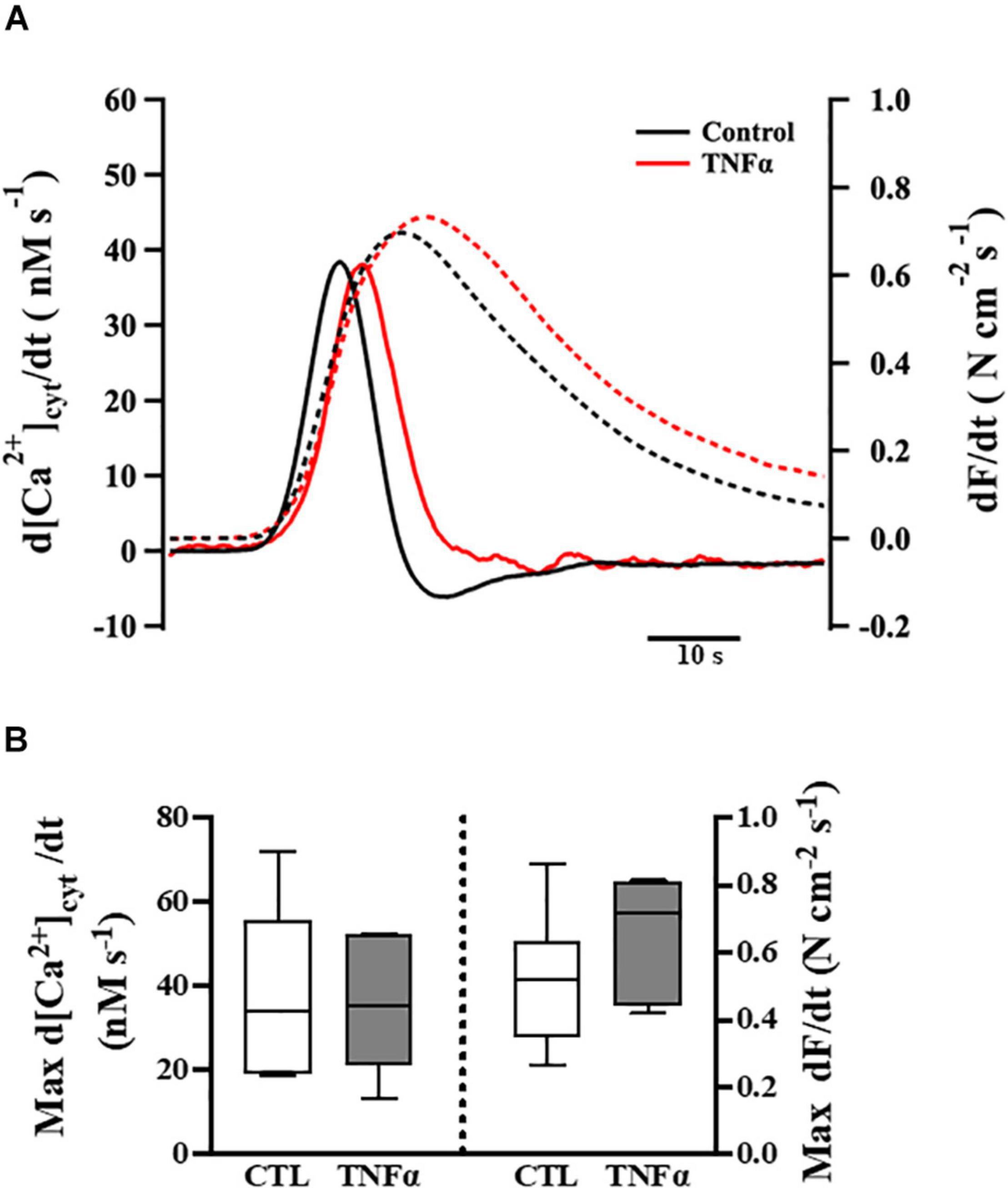
Figure 4. Tumor necrosis factor α exposure had no significant effects on the rates of rise of both the [Ca2+]cyt and force responses of ASM to ACh stimulation (EC50), compared to controls. Representative tracings to show the first derivatives of [Ca2+]cyt (solid lines) and force (dashed lines) responses to ACh stimulation (EC50) (A). The maximum d[Ca2+]cyt/dt and maximum dForce/dt at ACh concentration (EC50) are summarized as medians and interquartile range (IQR) (B). A one-way repeated measure ANOVA was used to analyze results; p > 0.05, n = 6 (number of animals).
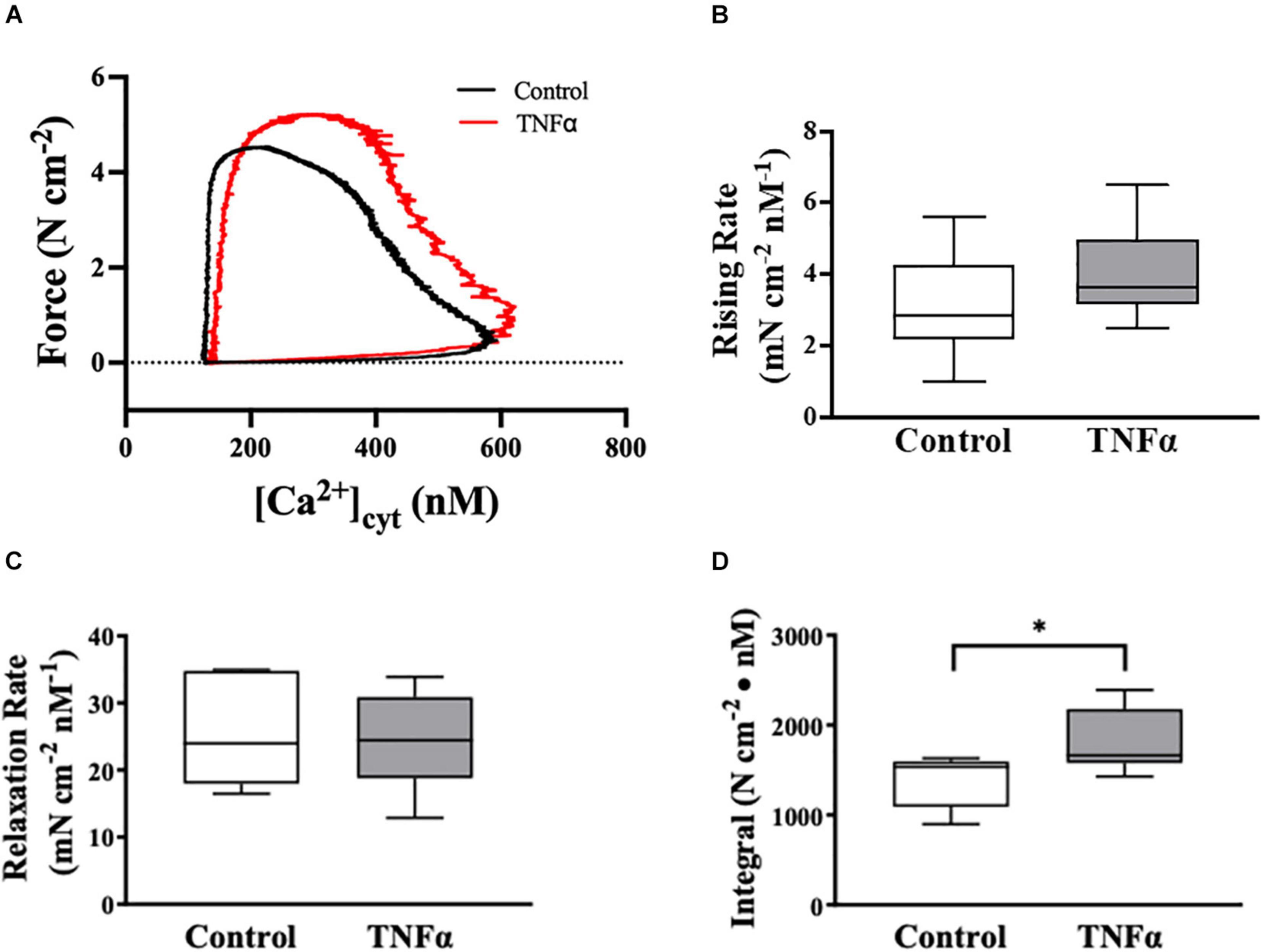
Figure 5. Tumor necrosis factor αsignificantly increased the integral of the rising phase of the phase-loop plots of ACh-induced [Ca2+]cyt and force as compared to controls. Representative tracings of phase-loop plots at ACh concentration (EC50) are presented in (A). Both rising rate and relaxation rate of the phase-loop plots in TNFα treated group were not different than untreated controls [p > 0.05, n = 6 (number of animals)]. (B,C) The integral of the rising phase of the phase-loop plots of ACh-induced [Ca2+]cyt and force was summarized and found to increase significantly with TNFα [∗p < 0.05, n = 6 (number of animals)] (D).
Statistical Analysis
Four ASM strips were used from each animal (n = 6) for the analysis of [Ca2+]cyt and force responses induced by ACh stimulation with (two strips) and without (two strips) 24-h exposure to TNFα. The two independent measurements of [Ca2+]cyt and force responses to ACh were then averaged to provide a single measurement per treatment per animal. In a separate set of four ASM strips from each animal (n = 6), baseline (no ACh stimulation) and ACh-induced changes in rMLC20 phosphorylation were analyzed with (two strips – one baseline and one ACh stimulated) and without (two strips – one baseline and one ACh stimulated) 24-h exposure to TNFα. Results were analyzed using a Student t-test (JMP Pro software; JMP, RRID: SCR_014242). Data are summarized as means ± SD, with significance established at p < 0.05.
Results
Effects of TNFα on ACh-Induced [Ca2+]cyt and Force Responses
In each of six animals (n = 6), the duplicate measurements of ASM [Ca2+]cyt and force responses to ACh stimulation were comparable (<10% variance between the two measurements) and averaged to obtain a single value for summary analysis. In each ASM strip, ACh stimulation induced a biphasic [Ca2+]cyt response reaching an initial peak by ∼5 s and then declining to a sustained plateau after ∼2–3 min. The isometric force response to ACh stimulation was delayed and slower with maximum specific force reached after ∼1–2 min. Compared to control (untreated) ASM strips, 24-h exposure to TNFα (100 ng/ml) increased the peak force response to ACh by ∼18% compared to control (Figures 2A,C; p < 0.05), whereas the peak [Ca2+]cyt response to ACh was unaffected by TNFα (Figures 2A,B). The ratio of maximum specific force to maximum [Ca2+]cyt increased by ∼18% in ASM strips exposed to 24-h TNFα (p < 0.01; Figure 2D). These results indicate that static Ca2+ sensitivity of force generation in ASM is increased by TNFα.
TNFα Does Not Affect the Temporal Delay Between ACh-Induced [Ca2+]cyt and Force Responses
In each ASM strip, [Ca2+]cyt and isometric force responses to ACh stimulation were simultaneously recorded (Figure 3A). The ACh-induced elevation of [Ca2+]cyt always preceded the force response by ∼3–5 s (Figure 3A). The fura-2 fluorescence signal of [Ca2+]cyt was noisier affecting the signal to noise level and detection of the onset of the ACh-induced response. Yet, the signal to noise ratio of the [Ca2+]cyt responses was less than 10%, which was sufficient to determine the onset of the [Ca2+]cyt response within 10 ms with a 1 kHz sampling rate. The noise level of the mechanical force signal was much lower, and thus did not affect the detection of the onset of the ACh force response. The temporal delay between the onset of ACh-induced [Ca2+]cyt and force responses was ∼4–5 s corresponding to the time to reach a peak [Ca2+]cyt response. Importantly, compared to control (untreated) ASM strips, 24-h TNFα exposure had no impact on the temporal delay between the onset of ACh-induced [Ca2+]cyt and force responses (Figure 3B).
TNFα Does Not Affect the Rate of Rise of ACh-Induced [Ca2+]cyt and Force Responses
The temporal characteristics of the ACh-induced [Ca2+]cyt and force responses in ASM strips were determined by calculating the first derivatives of [Ca2+]cyt and force responses (i.e., d[Ca2+]cyt/dt and dForce/dt) (Figure 4A). The maximum ACh-induced d[Ca2+]cyt/dt was ∼40 nM [Ca2+]cyt s–1. Compared to control (untreated) ASM strips, 24-h TNFα exposure had no significant effect on the rate of rise in ACh-induced [Ca2+]cyt response (Figure 4B). The maximum ACh-induced dForce/dt was much slower at ∼0.6 N cm–2 s–1. Although peak force was higher in TNFα treated ASM strips, the maximum dForce/dt was not different than untreated controls (Figure 4B).
TNFα Impacts the Phase-Loop Plots of ACh-Induced [Ca2+]cyt and Force Responses
The dynamic coupling of ACh-induced [Ca2+]cyt and force responses in ASM strips was evaluated using phase-loop plots (Figure 5A). The ascending limb of the phase loop plot showed that little force developed as [Ca2+]cyt increased to a peak value that was similar between 24-h TNFα treated and untreated control ASM strips. The rising rate of the phase-loop plots of ACh-induced [Ca2+]cyt and force responses was similar between TNFα and control ASM strips (Figure 5B). Subsequently force increased as [Ca2+]cyt decreased. Importantly, the ACh-induced force response in TNFα treated ASM strips was greater compared to untreated control ASM strips. The relaxation rate of the phase-loop plots of ACh-induced [Ca2+]cyt and force responses was also similar between TNFα and control ASM strips (Figure 5C). To provide an overall assessment of the dynamic coupling of ACh-induced [Ca2+]cyt and force responses, the integral of the phase-loop plots was calculated. Compared to control (untreated) ASM strips, TNFα significantly increased the integral of the phase-loop plot (p < 0.05, Figure 5D).
TNFα Does Not Affect the Extent of ACh-Induced rMLC20 Phosphorylation
In a separate set of ASM strips, the extent of rMLC20 phosphorylation (ratio of p-rMLC20 to total rMLC20) induced by 30 s ACh stimulation was examined in ASM strips using western blot analysis (Figure 6). The 30 s time period for ACh stimulation was selected as we have previously shown that the maximum extent of rMLC20 phosphorylation is reached by this time (Dogan et al., 2017). ACh stimulation significantly increased the extent of rMLC20 phosphorylation in both 24-h TNFα treated and untreated control ASM strips (Figures 6B,C), compared to the baseline (no ACh stimulation). Compared to control ASM strips, 24-h TNFα exposure had no significant effect on the extent of ACh-induced rMLC20 phosphorylation (Figures 6B,C), although the ACh-induced force generated by TNFα treated ASM strips after 30 s was significantly greater than that of control ASM strips (p < 0.05; Figure 6A). Thus, the increase in ACh-induced ASM force following TNFα exposure was unrelated to the extent of rMLC20 phosphorylation. These results are consistent with previous studies showing that TNFα increases ASM force generation due to increased contractile protein content (greater number of contractile units) and enhanced cytoskeletal remodeling (actin polymerization) resulting in increased tethering of contractile elements to the plasma membrane and force translation to the extracellular matrix (ECM) rather than changes in rMLC20 phosphorylation (Jones et al., 1999a; Zhang et al., 2015; Dogan et al., 2017; Sieck et al., 2019).
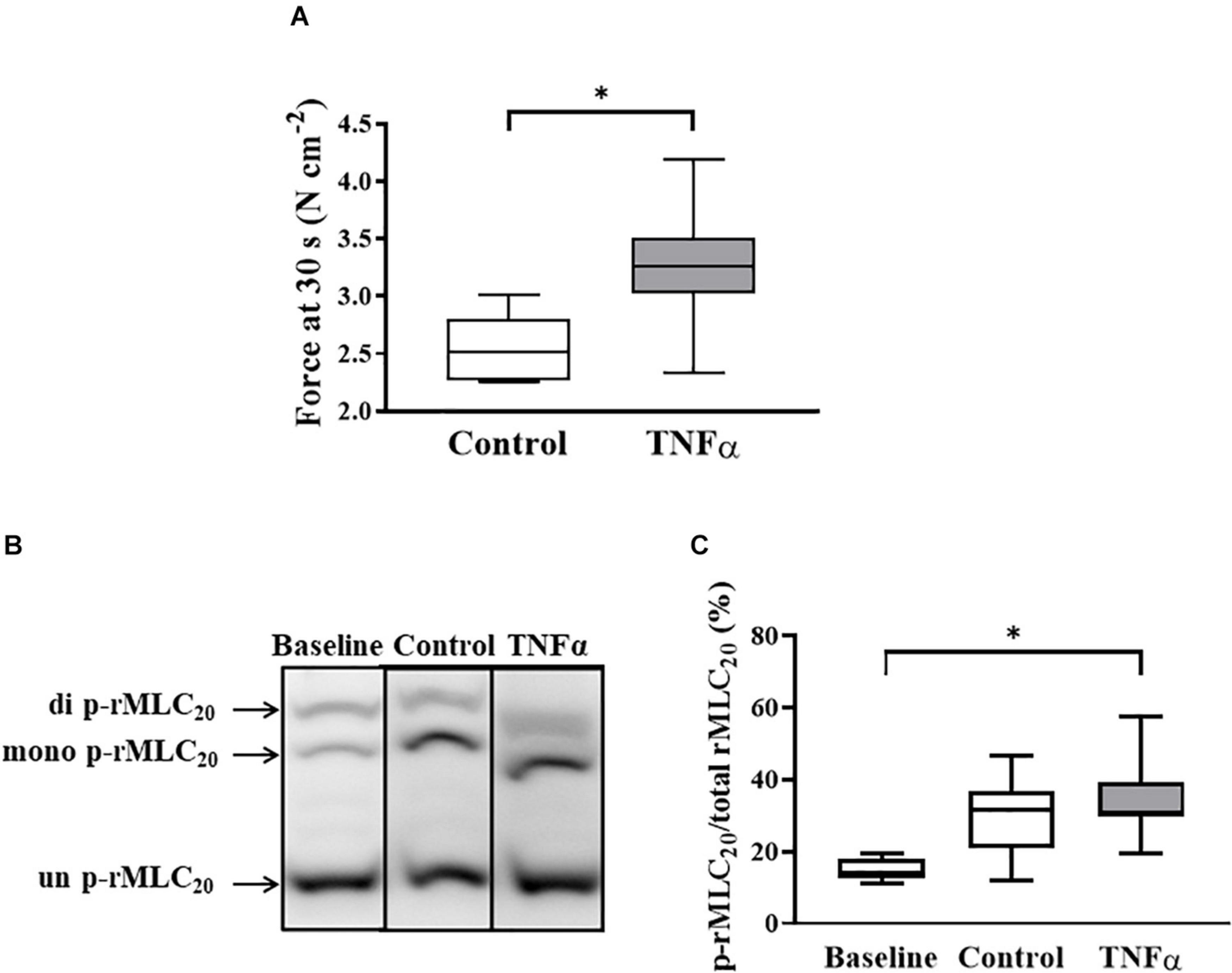
Figure 6. Tumor necrosis factor α had no significant effect on rMLC20 phosphorylation in ASM stimulated at ACh concentration (EC50) [p > 0.05, n = 6 (number of animals)] (C), even though ACh stimulation significantly increased the extent of rMLC20 phosphorylation in both 24-h TNFα treated and untreated control ASM strips (B,C), compared to the baseline (no ACh stimulation). However, force generated in ASM after 30 s at ACh stimulation (EC50) significantly increased with TNFα exposure, compared to controls [∗p < 0.05, n = 6 (number of animals)] (A). Representative western blots to show phosphorylated (single- and double-p-rMLC20) and un-phosphorylated (un-p-rMLC20) rMLC20 using a modified Phos-tagTM gels (B).
Discussion
The present study demonstrates that in porcine ASM, 24-h TNFα exposure significantly increases the force response induced by ACh stimulation while not affecting the [Ca2+]cyt response. Thus, by definition the Ca2+ sensitivity of force generation in ASM is increased by TNFα exposure. To further explore the impact of TNFα on the dynamic nature of Ca2+ sensitivity in ASM, the present study simultaneously measured [Ca2+]cyt and force responses to ACh stimulation. The initial coupling of [Ca2+]cyt and force responses involves the canonical signaling cascade that regulates rMLC20 phosphorylation and cross-bridge recruitment. An effect of TNFα on this regulatory signaling cascade would have been reflected by a reduced temporal delay in the shorter-term coupling of ACh-induced [Ca2+]cyt and force responses. However, the results of the present study showed no effect of TNFα on the temporal delay between the onset of [Ca2+]cyt and force responses or on the rate of rise in either parameter. Previous results from our lab indicated that 24-h TNFα exposure increases contractile protein concentration in ASM (Dogan et al., 2017; Sieck et al., 2019) and the tethering of actin to the cortical cytoskeleton (Sieck et al., 2019). The coupling between elevated [Ca2+]cyt, contractile unit recruitment and internal loading in ASM involves much longer time delays that are affected by TNFα exposure but independent of rMLC20 phosphorylation.
Does the Canonical rMLC20 Phosphorylation Pathway Regulate Ca2+ Sensitivity in ASM?
It is commonly thought that an increase in Ca2+ sensitivity of force generation in ASM is mediated by increasing the extent of rMLC20 phosphorylation via agonist-induced activation of rho kinase (ROCK)-dependent inhibition of myosin light chain phosphatase (MLCP) (de Lanerolle and Paul, 1991; Jones et al., 1994a, 1999a; Somlyo and Somlyo, 1994; Karaki et al., 1997; Sieck et al., 1998; Todoroki-Ikeda et al., 2000; Sakamoto et al., 2003; Sakai et al., 2017; Alvarez-Santos et al., 2020). However, we and others previously reported that the extent of rMLC20 phosphorylation induced by ACh stimulation reaches a maximum after ∼30 s or less and does not change thereafter (Jones et al., 1999a; Mehta and Gunst, 1999; Zhang et al., 2015; Dogan et al., 2017; Sieck et al., 2019; Seow and An, 2020; Han et al., 2021). This timeframe does not match the time for force generation in ASM, which reaches a maximum only after 60–120 s. Moreover, the extent of rMLC20 phosphorylation does not change with increasing ACh concentration, even though force increases (Dogan et al., 2017; Sieck et al., 2019; Han et al., 2021). Thus, while [Ca2+]cyt-dependent rMLC20 phosphorylation does mediate cross-bridge recruitment and the onset of a force response in ASM, it does not appear to regulate the longer-term time course or amplitude of the force response. In the present study, even at the lower EC50 concentration of ACh (1.3 vs 2.6 μM in TNFα treated vs untreated control, respectively), the force response in TNFα treated ASM was greater while the extent of rMLC20 phosphorylation was unaffected. In agreement, there was also no effect of TNFα exposure on the temporal delay between the onset of [Ca2+]cyt and force responses or on their rates of rise after ACh stimulation. These results indicate that neither the extent nor the rate of rMLC20 phosphorylation in ASM is affected by TNFα exposure. Thus, we conclude that ACh-dependent rMLC20 phosphorylation is not the primary mechanism through which Ca2+ sensitivity of force generation increases after 24-h TNFα exposure.
Short-Term Dynamic Coupling of ACh-Induced [Ca2+]cyt and Force Responses
In ASM, muscarinic stimulation induces a biphasic elevation of [Ca2+]cyt with a peak level reach in ∼5 s followed by a slow decline to steady state after ∼120–180 s. The initial elevation of [Ca2+]cyt in response to ACh stimulation is due to several sources of Ca2+ including inositol triphosphate receptor (IP3R) and ryanodine receptor (RyR) mediated sarcoplasmic reticulum (SR) Ca2+ release as well as extracellular Ca2+ influx via L-type, voltage-gated Ca2+ channels (Takuwa et al., 1987; Jones et al., 1994b). Both the IP3R and RyR channels are Ca2+ sensitive resulting in Ca2+-induced SR Ca2+ release (CICR) and a more rapid accelerating elevation of [Ca2+]cyt (Kannan et al., 1997; Sieck et al., 1997; Prakash et al., 2000; Pabelick et al., 2001; Bai et al., 2009). ACh stimulation activates phospholipase C and IP3 production, and IP3 mediates the opening of IP3R channels in the SR. In contrast, there is no second messenger agonist to mediate the opening of RyR channels in the SR. Instead, with the initial elevation of [Ca2+]cyt due to extracellular Ca2+ influx or IP3R mediated SR Ca2+ release, CICR mediates the opening of RyR channels in ASM. However, ACh-induced production of cyclic ADP ribose increases the sensitivity of RyR to CICR (Kannan et al., 1996; Hsuan et al., 1999; White et al., 2000; Deshpande et al., 2004). In porcine ASM, we previously showed that ACh stimulation induces localized constant amplitude [Ca2+]cyt oscillations that propagate through the cell (Prakash et al., 1997; Pabelick et al., 1999; Sieck et al., 2001). The global elevation of [Ca2+]cyt in response to muscarinic stimulation reflects the spatial-temporal integration of these propagating [Ca2+]cyt oscillations (Prakash et al., 1997; Pabelick et al., 1999; Sieck et al., 2001). While the amplitude of localized [Ca2+]cyt oscillations is constant, both [Ca2+]cyt oscillation frequency and propagation velocity are increased by increasing ACh concentration. The time course of the spatial/temporal summation of ACh-induced [Ca2+]cyt oscillations in ASM cells is ∼700 ms, roughly matching the short-term delay in rMLC20 phosphorylation and cross-bridge recruitment (Figure 1; Prakash et al., 1997; Pabelick et al., 1999; Sieck et al., 2001). Measurements of the time delay between the onset of [Ca2+]cyt and force responses as well as the first derivatives of these responses (i.e., d[Ca2+]cyt/dt and dForce/dt) reflect the short-term dynamic coupling mediated by rMLC20 phosphorylation and cross-bridge recruitment (Figures 3A,B). Importantly, 24-h TNFα exposure did not affect these measures of short-term coupling of ACh-induced [Ca2+]cyt and force responses.
Long-Term Dynamic Coupling of ACh-Induced [Ca2+]cyt and Force Responses
Unlike the [Ca2+]cyt response, the ACh-induced force response in ASM is not biphasic but develops at a much slower rate reaching a plateau in force only after 60–120 s. As shown in Figure 1, short-term force generation in ASM depends on [Ca2+]cyt/calmodulin-mediated activation of MLCK and rMLC20 phosphorylation and cross-bridge recruitment. The phosphorylation of rMLC20 is also affected by MLCP and dephosphorylation; however, we and others have shown that the maximum level of ACh-induced rMLC20 phosphorylation in ASM is reached and sustained after ∼30 s or less, well before the plateau in ACh-induced force (Washabau et al., 1991; Dogan et al., 2017; Sieck et al., 2019; Han et al., 2021). Importantly, ACh-induced force generation in ASM increases by the addition of de novo contractile units due to [Ca2+]cyt-dependent polymerization of actin and myosin, and to the tethering of filamentous actin to the cortical cytoskeleton for force translation to the ECM (Figure 1; Gunst and Tang, 2000; Sieck et al., 2001; Murphy and Rembold, 2005; Ijpma et al., 2010, 2011; Sieck and Gransee, 2012; Delmotte et al., 2020; Seow and An, 2020; Wang et al., 2020). It can be assumed that by ∼30 s after ACh stimulation, when the maximum extent of rMLC20 phosphorylation is achieved, the recruitment of available cross-bridges does not account for additional force generation. Thus, from 30 to ∼120 s after ACh stimulation when ASM force plateaus, the addition of de novo contractile units or the tethering of actin filaments to the cortical cytoskeleton are the major contributors to force generation.
The cycling of cross-bridges in ASM is associated with ATP hydrolysis, and is dependent on load (Jones et al., 1999a; Delmotte et al., 2020). The tethering of actin filaments to the cortical cytoskeleton via dense bodies allows ASM force transmission to the ECM (Jones et al., 1999b; Mehta and Gunst, 1999; Gunst and Fredberg, 2003; Gunst et al., 2003; Gunst and Zhang, 2008; Zhang and Gunst, 2008; Chitano et al., 2017; Dogan et al., 2017; Tang, 2018; Seow and An, 2020; Wang et al., 2020). At the same time, the tethering of contractile units increases internal loading and slows cross-bridge cycling rate (Dogan et al., 2017; Sieck et al., 2019; Han et al., 2021). In permeabilized ASM strips maximally activated by Ca2+ (pCa 4.0), we simultaneously measured force and ATP hydrolysis rate using an NADH-linked fluorescence technique (Delmotte et al., 2020). The maximal increase in ATP hydrolysis rate occurred during the initial 30–60 s with the rMLC20 phosphorylation-mediated recruitment of cross-bridges and the de novo addition of contractile units. Thereafter, the increase in ATP hydrolysis rate slowed reflecting the tethering of contractile units to the cortical cytoskeleton and internal loading with peak ATP hydrolysis rate reached after ∼120 s (Delmotte et al., 2020). Thereafter, ATP hydrolysis rate decreased with further tethering of contractile units and increased internal loading until a plateau was reached after ∼300–400 s.
The polymerization of monomeric globular (G) actin to filamentous (F) actin is Ca2+ dependent and increases with ACh stimulation (Hirshman and Emala, 1999; Jones et al., 1999b; Mehta and Gunst, 1999; Sieck et al., 2019). Zhang et al. reported that ACh-induced actin polymerization in ASM is regulated by RhoA activation, which enhances the assembly of the adhesome within the cortical cytoskeleton (Zhang et al., 2012; Seow and An, 2020). In support, we found that the extent of actin polymerization and tethering to the cortical cytoskeleton increases with time and with ACh concentration (Brandenburg et al., 2019). Furthermore, inhibiting actin polymerization greatly reduces ASM force generation (Jones et al., 1999b; Mehta and Gunst, 1999; Sieck et al., 2019; Delmotte et al., 2020; Han et al., 2021), reflecting the disruption of both the contribution of additional contractile units and force translation due to the tethering of contractile elements to the cortical cytoskeleton.
Integral of Phase-Loop Plots of [Ca2+]cyt and Force Responses to ACh Stimulation
In the heart, phase-loop plots of [Ca2+]cyt and force responses evoked by electrical stimulation have been used to assess Ca2+ sensitivity of cardiomyocytes based on the [Ca2+]cyt at which 50% relaxation occurs (Schaible et al., 2016; Tveita et al., 2019). This assessment reflects the off-rate of Ca2+ binding to troponin-C (TnC) and thin filament regulation of cross-bridge attachment/detachment in cardiomyocytes. In contrast, the coupling of [Ca2+]cyt and force responses in ASM is far more complex; however, phase-loop plot of ACh-induced [Ca2+]cyt and force responses still provides valuable physiological insight. The rising phase of the phase-loop plot most likely reflects rMLC20 phosphorylation and cross-bridge recruitment, while the relaxation rate most likely reflects rMLC20 dephosphorylation. Importantly, both the rising phase and the relaxation rate of the phase-loop plots of ACh-induced [Ca2+]cyt and force responses were not affected by 24-h TNFα exposure. In contrast, the integral of the ACh-induced [Ca2+]cyt and force phase-loop plot increased significantly after 24-h TNFα.
Conclusion
We conclude that 24-h TNFα exposure increases the Ca2+ sensitivity of ASM force generation through an effect on the long-term dynamic coupling of ACh-induced [Ca2+]cyt and force responses. Our results indicate that the increase in Ca2+ sensitivity induced by TNFα exposure is not dependent on rMLC20 phosphorylation or dephosphorylation. Instead, TNFα exposure increases actin and myosin concentration in ASM leading to the recruitment of additional contractile units. In addition, TNFα exposure increases actin tethering to cortical cytoskeleton and force translation to the ECM.
Data Availability Statement
The raw data supporting the conclusions of this article will be made available by the authors, without undue reservation.
Ethics Statement
The animal study was reviewed and approved by the Institutional Animal Care and Use Committee (IACUC) Mayo Clinic.
Author Contributions
Y-SH, PD, and GS contributed the conception and design of the study, analyzed the data, performed the statistical analysis, and wrote the manuscript. Y-SH collected the data. All authors approved the final version of the manuscript and agreed to be accountable for all aspects of the work in ensuring that questions related to the accuracy or integrity of any part of the work were appropriately investigated and resolved.
Funding
This work was supported by the National Institutes of Health (NIH) RO1HL157984 to GS.
Conflict of Interest
The authors declare that the research was conducted in the absence of any commercial or financial relationships that could be construed as a potential conflict of interest.
Publisher’s Note
All claims expressed in this article are solely those of the authors and do not necessarily represent those of their affiliated organizations, or those of the publisher, the editors and the reviewers. Any product that may be evaluated in this article, or claim that may be made by its manufacturer, is not guaranteed or endorsed by the publisher.
References
Alvarez-Santos, M. D., Alvarez-Gonzalez, M., Estrada-Soto, S., and Bazan-Perkins, B. (2020). Regulation of myosin light-chain phosphatase activity to generate airway smooth muscle hypercontractility. Front. Physiol. 11:701. doi: 10.3389/fphys.2020.00701
Bai, Y., Edelmann, M., and Sanderson, M. J. (2009). The contribution of inositol 1,4,5-trisphosphate and ryanodine receptors to agonist-induced Ca2+ signaling of airway smooth muscle cells. Am. J. Physiol. Lung Cell. Mol. Physiol. 297, L347–L361.
Brandenburg, J. E., Fogarty, M. J., and Sieck, G. C. (2019). A critical evaluation of current concepts in cerebral palsy. Physiology 34, 216–229. doi: 10.1152/physiol.00054.2018
Chitano, P., Wang, L., Tin, G. Y. Y., Ikebe, M., Pare, P. D., and Seow, C. Y. (2017). Smooth muscle function and myosin polymerization. J. Cell Sci. 130, 2468–2480.
de Lanerolle, P., and Paul, R. J. (1991). Myosin phosphorylation/dephosphorylation and regulation of airway smooth muscle contractility. Am. J. Physiol. 261, L1–L14.
Delmotte, P., Han, Y. S., and Sieck, G. C. (2020). Cytoskeletal remodeling slows cross-bridge cycling and ATP hydrolysis rates in airway smooth muscle. Physiol. Rep. 8:e14561.
Deshpande, D. A., Dogan, S., Walseth, T. F., Miller, S. M., Amrani, Y., Panettieri, R. A., et al. (2004). Modulation of calcium signaling by interleukin-13 in human airway smooth muscle: role of CD38/cyclic adenosine diphosphate ribose pathway. Am. J. Respir. Cell Mol. Biol. 31, 36–42. doi: 10.1165/rcmb.2003-0313oc
Dimopoulos, G. J., Semba, S., Kitazawa, K., Eto, M., and Kitazawa, T. (2007). Ca2+-dependent rapid Ca2+ sensitization of contraction in arterial smooth muscle. Circ. Res. 100, 121–129.
Dogan, M., Han, Y. S., Delmotte, P., and Sieck, G. C. (2017). TNFalpha enhances force generation in airway smooth muscle. Am. J. Physiol. Lung Cell Mol. Physiol. 312, L994–L1002.
Fredberg, J. J., Jones, K. A., Nathan, M., Raboudi, S., Prakash, Y. S., Shore, S. A., et al. (1996). Friction in airway smooth muscle: mechanism, latch, and implications in asthma. J. Appl. Physiol. 81, 2703–2712. doi: 10.1152/jappl.1996.81.6.2703
Grynkiewicz, G., Poenie, M., and Tsien, R. Y. (1985). A new generation of Ca2+ indicators with greatly improved fluoresence properites. J. Biol. Chem. 260, 3440–3450. doi: 10.1016/s0021-9258(19)83641-4
Gunst, S. J., and Fredberg, J. J. (2003). The first three minutes: smooth muscle contraction, cytoskeletal events, and soft glasses. J. Appl. Physiol. 95, 413–425. doi: 10.1152/japplphysiol.00277.2003
Gunst, S. J., and Tang, D. D. (2000). The contractile apparatus and mechanical properties of airway smooth muscle. Eur. Respir. J. 15, 600–616. doi: 10.1034/j.1399-3003.2000.15.29.x
Gunst, S. J., Tang, D. D., and Opazo Saez, A. (2003). Cytoskeletal remodeling of the airway smooth muscle cell: a mechanism for adaptation to mechanical forces in the lung. Respir. Physiol. Neurobiol. 137, 151–168. doi: 10.1016/s1569-9048(03)00144-7
Gunst, S. J., and Zhang, W. (2008). Actin cytoskeletal dynamics in smooth muscle: a new paradigm for the regulation of smooth muscle contraction. Am. J. Physiol. Cell Physiol. 295, C576–C587.
Guth, K., and Wojciechowski, R. (1986). Instruments and techniques: perfusion cuvette for the simultaneous measurement of mechanical, optical and energetic parameters of skinned muscle fibres. Pflugers Arch. 407, 552–557. doi: 10.1007/bf00657515
Han, Y. S., Delmotte, P. F., Arteaga, G. M., and Sieck, G. C. (2021). Dynamic cytosolic Ca(2+) and force responses to muscarinic stimulation in airway smooth muscle. Am. J. Physiol. Lung Cell Mol. Physiol. 321, L91–L101.
Han, Y. S., Schaible, N., Tveita, T., and Sieck, G. (2018). Discontinued stimulation of cardiomyocytes provides protection against hypothermia-rewarming-induced disruption of excitation-contraction coupling. Exp. Physiol. 103, 819–826. doi: 10.1113/ep086774
Han, Y. S., Tveita, T., Prakash, Y. S., and Sieck, G. C. (2010). Mechanisms underlying hypothermia-induced cardiac contractile dysfunction. Am. J. Physiol. Heart Circ. Physiol. 298, H890–H897.
Hirshman, C. A., and Emala, C. W. (1999). Actin reorganization in airway smooth muscle cells involves Gq and Gi-2 activation of Rho. Am. J. Physiol. 277, L653–L661.
Hsuan, S. L., Kannan, M. S., Jeyaseelan, S., Prakash, Y. S., Malazdrewich, C., Abrahamsen, M. S., et al. (1999). Pasteurella haemolytica leukotoxin and endotoxin induced cytokine gene expression in bovine alveolar macrophages requires NF-kappaB activation and calcium elevation. Microb. Pathog. 26, 263–273. doi: 10.1006/mpat.1998.0271
Ijpma, G., Al-Jumaily, A. M., Cairns, S. P., and Sieck, G. C. (2010). Logarithmic superposition of force response with rapid length changes in relaxed porcine airway smooth muscle. Am. J. Physiol. Lung Cell. Mol. Physiol. 299, L898–L904.
Ijpma, G., Al-Jumaily, A. M., Cairns, S. P., and Sieck, G. C. (2011). Myosin filament polymerization and depolymerization in a model of partial length adaptation in airway smooth muscle. J. Appl. Physiol. 111, 735–742. doi: 10.1152/japplphysiol.00114.2011
Jones, K. A., Lorenz, R. R., Prakash, Y. S., Sieck, G. C., and Warner, D. O. (1999a). ATP hydrolysis during contraction of permeabilized airway smooth muscle. Am. J. Physiol. 277, L334–L342.
Jones, K. A., Perkins, W. J., Lorenz, R. R., Prakash, Y. S., Sieck, G. C., and Warner, D. O. (1999b). F-actin stabilization increases tension cost during contraction of permeabilized airway smooth muscle in dogs. J. Physiol. 519(Pt 2), 527–538. doi: 10.1111/j.1469-7793.1999.0527m.x
Jones, K. A., Lorenz, R. R., Warner, D. O., Katusic, Z. S., and Sieck, G. C. (1994a). Changes in cytosolic cGMP and calcium in airway smooth muscle relaxed by 3-morpholinosydnonimine. Am. J. Physiol. 266, L9–L16.
Jones, K. A., Wong, G. Y., Lorenz, R. R., Warner, D. O., and Sieck, G. C. (1994b). Effects of halothane on the relationship between cytosolic calcium and force in airway smooth-muscle. Am. J. Physiol. 266, L199–L204.
Kannan, M. S., Fenton, A. M., Prakash, Y. S., and Sieck, G. C. (1996). Cyclic ADP-ribose stimulates sarcoplasmic reticulum calcium release in porcine coronary artery smooth muscle. Am. J. Physiol. 270, H801–H806.
Kannan, M. S., Prakash, Y. S., Brenner, T., Mickelson, J. R., and Sieck, G. C. (1997). Role of ryanodine receptor channels in Ca2+ oscillations of porcine tracheal smooth muscle. Am. J. Physiol. 272, L659–L664.
Karaki, H., Ozaki, H., Hori, M., Mitsui-Saito, M., Amano, K., Harada, K., et al. (1997). Calcium movements, distribution, and functions in smooth muscle. Pharmacol. Rev. 49, 157–230.
Lorenz, R. R., Warner, D. O., and Jones, K. A. (1999). Hydrogen peroxide decreases Ca(2+) sensitivity in airway smooth muscle by inhibiting rMLC phosphorylation. Am. J. Physiol. 277, L816–L822.
Mehta, D., and Gunst, S. J. (1999). Actin polymerization stimulated by contractile activation regulates force development in canine tracheal smooth muscle. J. Physiol. 519(Pt 3), 829–840. doi: 10.1111/j.1469-7793.1999.0829n.x
Murphy, R. A., and Rembold, C. M. (2005). The latch-bridge hypothesis of smooth muscle contraction. Can. J. Physiol. Pharmacol. 83, 857–864. doi: 10.1139/y05-090
Pabelick, C. M., Hrometz, S. L., Prakash, Y. S., Warner, D. O., and Sieck, G. C. (1999). Comparison of sevoflurane and isoflurane effects on calcium regulation in airway smooth muscle. Anesthesiology 91, U240–U240.
Pabelick, C. M., Sieck, G. C., and Prakash, Y. S. (2001). Invited review: significance of spatial and temporal heterogeneity of calcium transients in smooth muscle. J. Appl. Physiol. 91, 488–496. doi: 10.1152/jappl.2001.91.1.488
Parkman, H. P., Garbarino, R., and Ryan, J. P. (2001). Myosin light chain phosphorylation correlates with contractile force in guinea pig gallbladder muscle. Dig. Dis. Sci. 46, 176–181.
Prakash, Y. S., Kannan, M. S., and Sieck, G. C. (1997). Regulation of intracellular calcium oscillations in porcine tracheal smooth muscle cells. Am. J. Physiol. 272, C966–C975.
Prakash, Y. S., Pabelick, C. M., Kannan, M. S., and Sieck, G. C. (2000). Spatial and temporal aspects of ACh-induced [Ca2+]i oscillations in porcine tracheal smooth muscle. Cell Calcium 27, 153–162. doi: 10.1054/ceca.1999.0106
Sakai, H., Suto, W., Kai, Y., and Chiba, Y. (2017). Mechanisms underlying the pathogenesis of hyper-contractility of bronchial smooth muscle in allergic asthma. J. Smooth Muscle Res. 53, 37–47. doi: 10.1540/jsmr.53.37
Sakamoto, K., Hori, M., Izumi, M., Oka, T., Kohama, K., Ozaki, H., et al. (2003). Inhibition of high K+-induced contraction by the ROCKs inhibitor Y-27632 in vascular smooth muscle: possible involvement of ROCKs in a signal transduction pathway. J. Pharmacol. Sci. 92, 56–69. doi: 10.1254/jphs.92.56
Schaible, N., Han, Y. S., Hoang, T., Arteaga, G., Tveita, T., and Sieck, G. (2016). Hypothermia/rewarming disrupts excitation-contraction coupling in cardiomyocytes. Am. J. Physiol. Heart Circ. Physiol. 310, H1533–H1540.
Schaible, N., Han, Y. S., Tveita, T., and Sieck, G. C. (2018). Role of superoxide ion formation in hypothermia/rewarming induced contractile dysfunction in cardiomyocytes. Cryobiology 81, 57–64. doi: 10.1016/j.cryobiol.2018.02.010
Seow, C. Y., and An, S. S. (2020). The force awakens in the cytoskeleton: the saga of a shape-shifter. Am. J. Respir. Cell Mol. Biol. 62, 550–551. doi: 10.1165/rcmb.2019-0462ed
Sieck, G. C., Dogan, M., Young-Soo, H., Osorio Valencia, S., and Delmotte, P. (2019). Mechanisms underlying TNFalpha-induced enhancement of force generation in airway smooth muscle. Physiol. Rep. 7:e14220.
Sieck, G. C., and Gransee, H. M. (2012). Respiratory Muscles Structure, Function & Regulation, Colloquium Series on Integrated Systems Physiology, From Molecule to Function to Disease. San Rafael, CL: Morgan & Claypool Life Sciences.
Sieck, G. C., Han, Y. S., Pabelick, C. M., and Prakash, Y. S. (2001). Temporal aspects of excitation-contraction coupling in airway smooth muscle. J. Appl. Physiol. 91, 2266–2274. doi: 10.1152/jappl.2001.91.5.2266
Sieck, G. C., Han, Y. S., Prakash, Y. S., and Jones, K. A. (1998). Cross-bridge cycling kinetics, actomyosin ATPase activity and myosin heavy chain isoforms in skeletal and smooth respiratory muscles. Comp. Biochem. Physiol. B Biochem. Mol. Biol. 119, 435–450. doi: 10.1016/s0305-0491(98)00005-4
Sieck, G. C., Kannan, M. S., and Prakash, Y. S. (1997). Heterogeneity in dynamic regulation of intracellular calcium in airway smooth muscle cells. Can. J. Physiol. Pharmacol. 75, 878–888. doi: 10.1139/y97-103
Somlyo, A. P., and Somlyo, A. V. (1994). Signal transduction and regulation in smooth muscle. Nature 372, 231–236. doi: 10.1038/372231a0
Spurgeon, H. A., duBell, W. H., Stern, M. D., Sollott, S. J., Ziman, B. D., Silverman, H. S., et al. (1992). Cytosolic calcium and myofilaments in single rat cardiac myocytes achieve a dynamic equilibrium during twitch relaxation. J. Physiol. 447, 83–102. doi: 10.1113/jphysiol.1992.sp018992
Takeya, K., Loutzenhiser, K., Shiraishi, M., Loutzenhiser, R., and Walsh, M. P. (2008). A highly sensitive technique to measure myosin regulatory light chain phosphorylation: the first quantification in renal arterioles. Am. J. Physiol. Renal 294, F1487–F1492.
Takuwa, Y., Takuwa, N., and Rasmussen, H. (1987). Measurement of cytoplasmic free Ca2+ concentration in bovine tracheal smooth muscle using aequorin. Am. J. Physiol. 253(6 Pt 1), C817–C827.
Tang, D. D. (2018). The Dynamic Actin Cytoskeleton in Smooth Muscle. Adv. Pharmacol. 81, 1–38. doi: 10.1016/bs.apha.2017.06.001
Todoroki-Ikeda, N., Mogami, K., Kusuda, T., Yamamoto, K., Miyake, T., Sato, M., et al. (2000). Sphingosylphosphorylcholine induces Ca(2+)-sensitization of vascular smooth muscle contraction: possible involvement of rho-kinase. FEBS Lett. 482, 85–90. doi: 10.1016/s0014-5793(00)02046-9
Tveita, T., Arteaga, G. M., Han, Y. S., and Sieck, G. C. (2019). Cardiac troponin-I phosphorylation underlies myocardial contractile dysfunction induced by hypothermia rewarming. Am. J. Physiol. Heart Circ. Physiol. 317, H726–H731.
Walsh, M. P., Thornbury, K., Cole, W. C., Sergeant, G., Hollywood, M., and McHale, N. (2011). Rho-associated kinase plays a role in rabbit urethral smooth muscle contraction, but not via enhanced myosin light chain phosphorylation. Am. J. Physiol. Renal Physiol. 300, F73–F85.
Wang, Y., Wang, R., and Tang, D. D. (2020). Ste20-like kinase-mediated control of actin polymerization is a new mechanism for thin filament-associated regulation of airway smooth muscle contraction. Am. J. Respir. Cell. Mol. Biol. 62, 645–656. doi: 10.1165/rcmb.2019-0310oc
Washabau, R. J., Wang, M. B., and Ryan, J. P. (1991). Myosin light chain phosphorylation and contraction of guinea pig gallbladder smooth muscle. Am. J. Physiol. 261, G952–G957.
White, T., Johnson, S., Walseth, T., Hon, L., Graeff, R., Munshi, C., et al. (2000). Subcellular localization of cyclic ADP ribosyl cyclase and cyclic ADP ribose hydrolase activities in porcine airway smooth muscle. Biochim. Biophys. Acta 1498, 64–71. doi: 10.1016/s0167-4889(00)00077-x
Zhang, W., and Gunst, S. J. (2008). Interactions of airway smooth muscle cells with their tissue matrix: implications for contraction. Proc. Am. Thorac. Soc. 5, 32–39. doi: 10.1513/pats.200704-048vs
Zhang, W., Huang, Y., and Gunst, S. J. (2012). The small GTPase RhoA regulates the contraction of smooth muscle tissues by catalyzing the assembly of cytoskeletal signaling complexes at membrane adhesion sites. J. Biol. Chem. 287, 33996–34008. doi: 10.1074/jbc.m112.369603
Zhang, W., Huang, Y., Wu, Y., and Gunst, S. J. (2015). A novel role for RhoA GTPase in the regulation of airway smooth muscle contraction. Can. J. Physiol. Pharmacol. 93, 129–136. doi: 10.1139/cjpp-2014-0388
Zimmermann, B., Somlyo, A. V., Ellis-Davies, G. C., Kaplan, J. H., and Somlyo, A. P. (1995). Kinetics of prephosphorylation reactions and myosin light chain phosphorylation in smooth muscle. Flash photolysis studies with caged calcium and caged ATP. J. Biol. Chem. 270, 23966–23974. doi: 10.1074/jbc.270.41.23966
Keywords: inflammatory cytokines, dynamic Ca2+ sensitivity, airway smooth muscle, phase-loop plots, myosin light chain, temporal delay
Citation: Han Y-S, Delmotte P and Sieck GC (2021) Effects of TNFα on Dynamic Cytosolic Ca2 + and Force Responses to Muscarinic Stimulation in Airway Smooth Muscle. Front. Physiol. 12:730333. doi: 10.3389/fphys.2021.730333
Received: 24 June 2021; Accepted: 09 July 2021;
Published: 30 July 2021.
Edited by:
Chun Y. Seow, University of British Columbia, CanadaReviewed by:
Dale Tang, Albany Medical College, United StatesSteven S. An, Rutgers Institute for Translational Medicine and Science, United States
Copyright © 2021 Han, Delmotte and Sieck. This is an open-access article distributed under the terms of the Creative Commons Attribution License (CC BY). The use, distribution or reproduction in other forums is permitted, provided the original author(s) and the copyright owner(s) are credited and that the original publication in this journal is cited, in accordance with accepted academic practice. No use, distribution or reproduction is permitted which does not comply with these terms.
*Correspondence: Gary C. Sieck, Sieck.Gary@mayo.edu
†These authors have contributed equally to this work and share first authorship