- 1Laboratório de Sinalização Celular e Expressão Gênica, Departamento de Biologia Geral e Aplicada, Instituto de Biociências, UNESP, Rio Claro, Brazil
- 2Institute for Integrative Biology of the Cell (I2BC), CEA, CNRS, Université Paris-Saclay, Gif-sur-Yvette, France
Animal experimentation is limited by unethical procedures, time-consuming protocols, and high cost. Thus, the development of innovative approaches for disease treatment based on alternative models in a fast, safe, and economic manner is an important, yet challenging goal. In this paradigm, the fruit-fly Drosophila melanogaster has become a powerful model for biomedical research, considering its short life cycle and low-cost maintenance. In addition, biological processes are conserved and homologs of ∼75% of human disease-related genes are found in the fruit-fly. Therefore, this model has been used in innovative approaches to evaluate and validate the functional activities of candidate molecules identified via in vitro large-scale analyses, as putative agents to treat or reverse pathological conditions. In this context, Drosophila offers a powerful alternative to investigate the molecular aspects of liver diseases, since no effective therapies are available for those pathologies. Non-alcoholic fatty liver disease is the most common form of chronic hepatic dysfunctions, which may progress to the development of chronic hepatitis and ultimately to cirrhosis, thereby increasing the risk for hepatocellular carcinoma (HCC). This deleterious situation reinforces the use of the Drosophila model to accelerate functional research aimed at deciphering the mechanisms that sustain the disease. In this short review, we illustrate the relevance of using the fruit-fly to address aspects of liver pathologies to contribute to the biomedical area.
Introduction
In modern societies, obesity has become a prevalent problem; according to the World Health Organization (WHO), more than 600 million adults were obese in 2016 (WGO, 2020). Consequently, metabolic dysfunctions have considerably increased, including hepatic diseases, which are one of the top 10 causes of death worldwide (Asrani et al., 2019; WHO, 2020; Powell et al., 2021). These diseases classified as acute or chronic may progress to irreversible damage and malfunction of the liver, while therapeutic strategies are still limited. To address this problem, innovative drugs and natural products have been explored; however, clinical trials have their own limitations, considering patient safety against the adverse side effects of pharmaceutical products, extensive time required for analyses, and ethical issues. Thus, to help investigating new compounds in preclinical stages, the Drosophila melanogaster model has become of utmost importance. Drosophila genetics has been used for studying many biological processes and is now a powerful alternative model to investigate metabolic changes associated with disease settings. The fruit-fly has a short life cycle, which enables analyses of the effects of drugs on various metabolic routes in a short time (Tennessen et al., 2014; Men et al., 2016; Lee et al., 2020). Furthermore, relevant biological processes and disease-related genes are conserved between humans and Drosophila (Pandey and Nichols, 2011; Ugur et al., 2016), despite the flies lower genomic complexity compared to that of humans. In this minireview we discuss the advantages offered by the fruit-fly model to investigate hepatic dysfunctions in a translational perspective.
The Burden of Hepatic Diseases Needs Translational Science
The liver is a critical organ for the maintenance of body homeostasis; it controls several molecular and metabolic routes and also acts in detoxification processes (Ramos et al., 2021). In metabolic disorders, lipids may accumulate in the hepatocytes contributing to the establishment of fatty liver, also known as hepatic steatosis. This clinical condition, which happens when triacylglycerols (TAGs) represent at least 5% of the liver weight (Ikura, 2014; Powell et al., 2021; Ramos et al., 2021), can remain asymptomatic for several years or progress to more deleterious stages. Non-alcoholic fatty liver disease (NAFLD) is one of the most common forms of liver pathologies affecting ∼25% of the world population (Younossi et al., 2018). In addition, drug abuse, environmental contaminants, imbalanced diet, and viral infections may also contribute to the development of NAFLDs (Byrne and Targher, 2015). More dramatically, NAFLD favors the development of chronic hepatitis that potentially progresses to cirrhosis, increasing the risk for HCC (Friedman et al., 2018; Powell et al., 2021). The worldwide panel of liver pathologies also comprises chronic viral hepatitis, alcoholic liver disease, metabolic and cholestatic liver dysfunctions (Asrani et al., 2019; WGO, 2020). Although NAFLD was identified more than 50 years ago (Parise, 2019; Sanyal, 2019), the therapeutic options to treat or at least to control these diseases rely on long-term procedures and are still limited to weight loss and diet modification, stressing the critical need for innovative strategies based on fast and reliable preclinical tests.
Translational science aims at using the scientific discoveries from preclinical models to set up the bases for clinical trials (Gilliland et al., 2016; van Erk et al., 2021). Knowledge on liver pathologies has now increased, leading to the characterization of the biochemical mechanisms underlying disease progression and to the identification of specific biomarkers (Friedman et al., 2018; Samuel and Shulman, 2018; Eslam and George, 2020). Advances in our understanding of lipid and sugar metabolism along with omics studies and mechanistic investigations, have revealed important regulatory functions for the transcription factors sterol-regulatory-element-binding-proteins (SREBPs), carbohydrate-responsive-element-binding-protein (ChREBP), liver-X-receptors (LXRs), and peroxisome-proliferator-activated-receptors (PPARs). In addition, the patatin-like-phospholipase-domain-containing-3 (PNPLA3), a triacylglycerol-lipase, has been shown to contribute to the control of energy expenditure or storage; PNPLA3 expression is regulated by nutritional sources, especially carbohydrates (Bruschi et al., 2017). Furthermore, the mechanistic-target-of-rapamycin (mTOR), which coordinates cell growth at the organismal level, is implicated in metabolic-related disease (Saxton and Sabatini, 2017; Ji et al., 2021). Other studies have demonstrated that epigenetic processes have been linked to liver diseases, but also that the genetic profile of an individual is directly correlated to the severity of the disease, since different alleles encode metabolic enzymes with variable functional activities (Anstee et al., 2019; Ramos et al., 2021). These findings open unexplored fields in the search for innovative therapeutics, including oligonucleotide usage to reduce through RNA-interference (RNAi) the levels of molecules relevant for liver homeostasis. In this context, translational research provides a safe way to test innovative drugs at a large scale using animal models. Rodents have long been used in preclinical trials, despite the time-consuming analyses, while unpredictable side-effects of the drugs in humans cannot be excluded (Bryda, 2013; Van Norman, 2019). Thus, alternative models provide a golden solution, considering that many societies limit animal experimentation to protect them against cruelty (Doke and Dhawale, 2015; Freires et al., 2017).
The Fruit-Fly Model
In this paradigm, the fruit-fly has emerged as a powerful system to study pathological conditions. The Drosophila life-cycle comprises four developmental stages: embryonic, larval, pupal, and adult. Development from embryo to adult takes about 10 days and adult lifespan 1–2 months (Johnson and Stolzing, 2019), allowing metabolic investigations on hundreds of offspring either at juvenile (larva and pupa) or adult stages. Given its powerful genetics, the Drosophila model has proved an efficient strategy for the study of several types of human pathologies, including metabolic (Perrimon et al., 2016; Musselman and Kuhnlein, 2018; Baenas and Wagner, 2019), neurological (Coll-Tane et al., 2019; Link and Bellen, 2020; Mariano et al., 2020; Salazar et al., 2020), cardiac (Birse et al., 2010; Piazza and Wessells, 2011; Diop et al., 2015; Guida et al., 2019), digestive (Musselman and Kuhnlein, 2018; Nayak and Mishra, 2019), and nephrocytic (Millet-Boureima et al., 2018; Rani and Gautam, 2018) comorbidities. In addition, tumor models may be induced in larvae or adult flies by genome manipulation (Pagliarini and Xu, 2003; Igaki et al., 2006; Dong et al., 2007; Gonzalez, 2013; Hirabayashi et al., 2013; Samji et al., 2021). The fruit-fly enables functional large-scale analysis to validate relevant molecules and biomarkers in a faster way than with rodent-based studies (Fernandez-Hernandez et al., 2016; Richardson and Portela, 2018; Bossen et al., 2019; Papanikolopoulou et al., 2019; Bangi, 2020).
Most of the genes and metabolic routes involved in human hepatic diseases are conserved in Drosophila (Table 1). The conservation between fly and mammalian genes is of utmost interest for translational studies aiming for a deeper understanding of cellular dysfunctions for which, investigation is technically restricted in mammalian models and impossible in humans.
In contrast to mammals, most invertebrates do not contain an organ equivalent to the liver, although hepatic functions are conserved. In insects, the fat body (FB) has long been considered as the liver counterpart (Li et al., 2019), although recent studies suggest that the oenocytes also accomplish hepatic-related functions (Gutierrez et al., 2007; Storelli et al., 2019). In Drosophila, the FB is an organ that spreads throughout the entire organism and oenocytes are groups of cells located underneath the abdominal external cuticle. In humans, dietary nutrients are transferred to the liver through a portal system and lipids are transported through the lymph stream as chylomicrons. Drosophila has an open circulatory system, so that nutrients crossing the intestinal epithelium enter the haemolymph, and thus, the body-wide distribution of the FB favors nutrient uptake (Figure 1A). Further, given its eating sources (mostly rotting fruits), the fruit-fly needs a powerful detoxification system that involves the gut, the FB and the oenocytes (Yang et al., 2007; Iredale, 2010; Huang et al., 2019). Over the past two decades, several studies on metabolic hepatic dysfunctions have taken advantage of the fly model (Hader et al., 2003; Villanueva et al., 2019; Ghosh et al., 2020; Hofbauer et al., 2020; Liao et al., 2020) to describe the functional activities of molecules relevant for human pathologies (Perrimon et al., 2016; Ugur et al., 2016; Heier and Kuhnlein, 2018; Hmeljak and Justice, 2019).
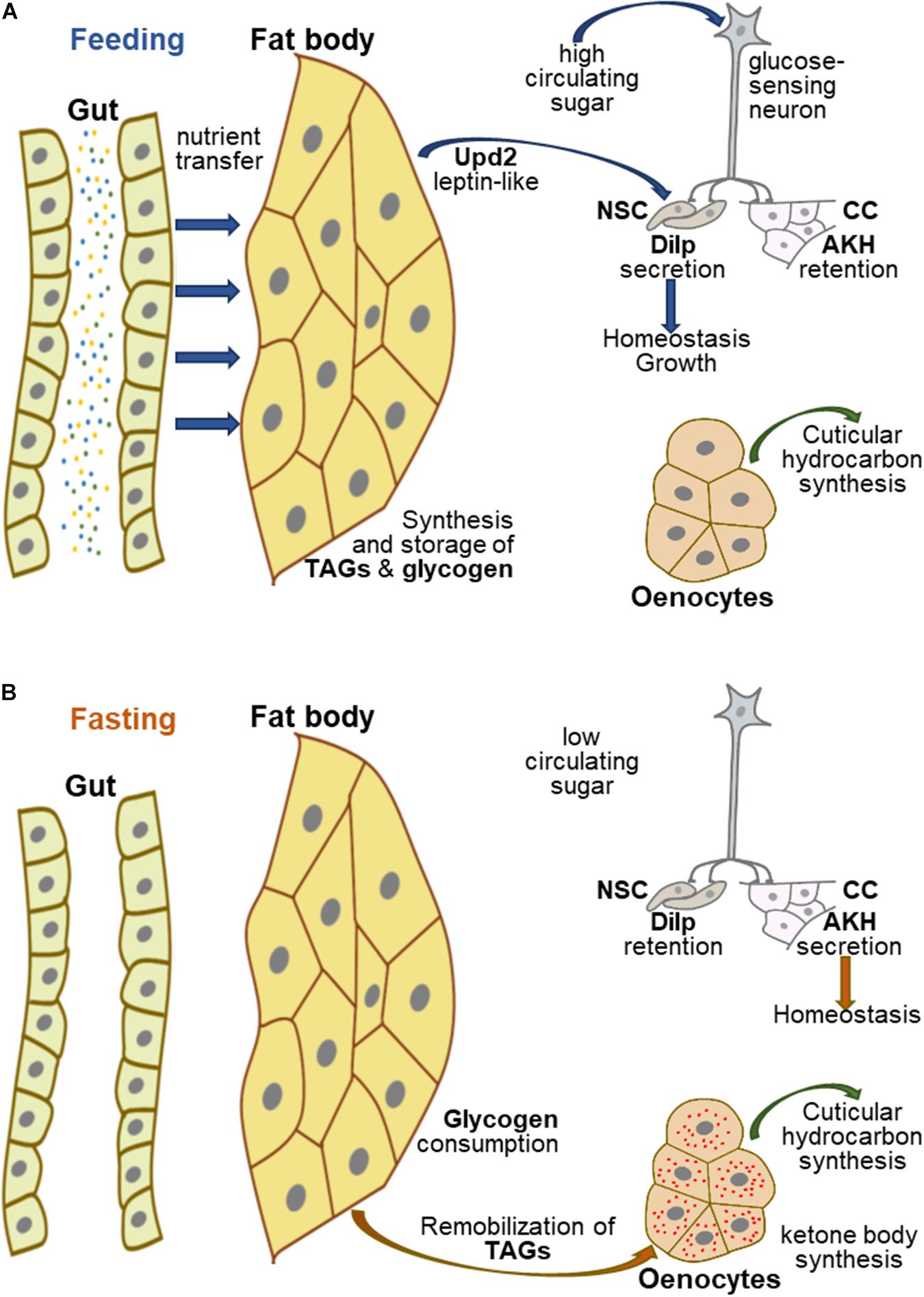
Figure 1. Hepatic-like homeostasis in Drosophila. (A) In fed Drosophila, nutrients are transferred to the appropriate organs, in particular the FB, which acts as a nutrient sensor to coordinate organismal growth and homeostasis. The FB synthesizes and stores glycogen and TAGs, and secretes several proteins, including Upd2, which can be replaced experimentally by mammalian leptin; Upd2 and glucose-sensing neurons contribute to regulating the secretion of Dilps and AKH to control growth and homeostasis. (B) In fasting Drosophila, FB glycogen and TAGs are remobilized, the latter being transferred to the oenocytes to produce ketone bodies, while glucose sensing neurons potentiate AKH secretion and Dilp retention in neurosecretory cells to maintain homeostasis.
Homeostatic Dysfunctions in Non-Alcoholic Fatty Liver Disease
Type 2 diabetes mellitus (T2DM) is another metabolic-related disease progressing exponentially. The T2DM hallmark is the development of insulin resistance, due to impaired response to the hormone or its reduced production by pancreatic β-cells (Boland et al., 2017; Li et al., 2021), thereby resulting in high glucose levels, increased general oxidative stress, vascular problems, and serious secondary perturbations in the physiology and the metabolism of the body (Chatterjee et al., 2017; Galicia-Garcia et al., 2020). In diabetic patients, lipid metabolism is modified, and the liver may accumulate fatty acids (FAs), which facilitates the establishment of NAFLD (Sanyal, 2019; Kuchay et al., 2020).
In NAFLD, the metabolism of both lipid and glucose in hepatic cells is disrupted (Shen et al., 2017; Sanyal, 2019). The accumulation of lipids in the hepatocytes results from changes in lipid uptake, de novo synthesis of FAs, β-oxidation and export of very-low-density-lipoproteins (VLDL) (Ipsen et al., 2018; Sanyal, 2019), which together affect the serum levels of TAG and cholesterol and may provoke instability of body homeostasis. Conversely, these changes in lipid metabolism, which are in part connected to insulin resistance in the hepatic tissue, reinforce the persistence of liver dysfunction, indicating that cellular metabolism is integrated at the organismal level (Chao et al., 2019; Sanyal, 2019). Under a normal and healthy homeostatic environment, hepatic cells use pyruvate to produce energy through the citric-acid cycle in mitochondria. During this process the exceeding energy is exported from the mitochondria as citrate to generate palmitic acid that can be used as a precursor of membrane lipids or esterified to TAGs and stored in cytosolic lipid droplets (LDs) (Currie et al., 2013; Ramos et al., 2021). These hepatic lipid stores can be remobilized through the β-oxidation pathway in response to energy demands, or exported from the liver in water-soluble VLDL particles comprising apolipoproteins, cholesterol and phospholipids (Ipsen et al., 2018). However, under conditions of metabolic dysfunction, excess LDs may accumulate in the liver and trigger the NAFLD, which favors lipotoxicity and, subsequently, the development of inflammatory processes and an increase in the levels of reactive oxygen species. Together, these perturbations provoke mitochondrial dysfunction and disease progression, leading to insulin resistance, T2DM and metabolic syndrome (Rosato et al., 2019).
Binding of insulin to its cognate receptor activates the mTOR signaling network (Yoon, 2017; Meng et al., 2021), which acts through a feedback loop to dampen the insulin response by inactivating insulin-receptor-substrate-1 (IRS1) and repressing the expression of the insulin receptor (InR) and insulin-like-growth-factor-1 (IGF1). Therefore, in the context of metabolic dysfunction, the mTOR signaling network is poorly activated and the subsequent absence of the negative feedback loop sustains an auto-amplification of the insulin resistance process. In this pathological condition, a number of regulatory proteins are synthesized by the hepatocytes to counteract homeostasis disruption, including Notch-receptor-2, insulin-like-growth-factor-binding-proteins (IGFBPs), the potassium-inwardly rectifying-channel-subfamily-J-member-11, chemokines and kinases (Tamarai et al., 2019).
The PPAR nuclear receptors that can act as lipid sensors to regulate metabolic homeostasis are potential targets for the development of drug therapy against NAFLD (Liss and Finck, 2017). The human genome encodes three PPAR members (α, β/δ, and γ), which are differentially expressed in tissues, although each of them may impinge on liver metabolism. PPARα is largely expressed in the liver where it controls several aspects of FA homeostasis; it is frequently reduced in patients with steatosis. PPARβ/γ is mainly expressed in muscle cells and but at lower levels in adipocytes and hepatocytes. PPARγ, which is mainly expressed in adipose tissue, is upregulated in the NAFLD pathological condition to dampen dysfunction of lipid and glucose metabolism. However, these unbalanced production of PPARs may contribute to insulin resistance and metabolic syndrome (Liss and Finck, 2017; Corrales et al., 2018), supporting the use of PPAR agonists to treat NAFLD (Seo et al., 2008; Yoo et al., 2021). The utilization Glucagon-likepeptide-1 (GLP-1) receptor agonists is another therapeutic strategy currently explored in clinical trials (Dai et al., 2020; Lv et al., 2020). GLP-1 is secreted by enteroendocrine cells after meal ingestion to potentiate insulin secretion and suppress glucagon production. The secreted bioactive forms act systemically, since the GLP-1 receptor (GLP-1R) is expressed in a number of tissues (Dai et al., 2020). The utilization of GLP-1R agonists exhibiting a longer half-life compare to that of the genuine hormone has been approved for T2DM treatment (Andersen et al., 2018). These agonists have positive effects on steatogenesis in T2DM patients (Teshome et al., 2020), but controversial results on their benefits for NAFLD have as yet restrained their use (Lv et al., 2020). Finally, a number of molecules secreted by the adipose tissue may affect inflammatory processes, insulin resistance and NAFLD progression. Adiponectin is one such factor that is down-regulated in response to hepatic stress and constitutes a promising target to treat steatosis, since studies demonstrated that increased levels of this adipokine ameliorate NAFLD (Scherer et al., 1995; Shabalala et al., 2020). Given the diversity of signals that may induce metabolic deregulations in hepatic cells, genetic investigations using alternative in vivo models are needed to decipher the organ interconnections that elicit the deleterious condition of NAFLD.
Homeostasis and Metabolism in Drosophila
In Drosophila, the FB synthesizes and stores glycogen and TAG-containing LDs (Figure 1A; Gronke et al., 2003; Garrido et al., 2015; Yamada et al., 2018). In the gut, dietary lipids are hydrolyzed to glycerol, free FAs and monoacylglycerols and taken up into the enterocytes through a molecular mechanism poorly characterized as yet (Miguel-Aliaga et al., 2018; Holtof et al., 2019; Toprak et al., 2020). In the enterocytes, these molecules are converted to diacylglycerols that are loaded in the haemolymph on apolipophorin particles, functioning as lipid vehicles to the FB (Palm et al., 2012). However, FB cells deficient for fatty-acid-synthase fail to accumulate LDs, indicating that de novo FA synthesis is essential for lipid storage in these cells (Garrido et al., 2015). Conversely, fasting induces mobilization and consumption of lipid stores from the FB. The perilipins Lsd1 and Lsd2 envelop LDs and protect them from lipolysis, whereas the lipase, Brummer, catalyzes TAG hydrolysis (Gronke et al., 2005, 2007; Bi et al., 2012). Concurrent to fasting-induced lipid hydrolysis, oenocytes accumulate LDs (Figure 1B), suggesting that remobilized lipid stores efflux from the FB and are taken up and oxidized in the oenocytes (Gutierrez et al., 2007). This process is similar to the remobilization of lipid stores from adipocytes to hepatocytes induced by fasting in mammals (Iredale, 2010; Yamada et al., 2020). Congruently, enzymes responsible for ketone body biogenesis are highly expressed in oenocytes (Huang et al., 2019), although it has not been formally demonstrated whether other tissues (FB, muscles) may also perform β-oxidation to supply energy demand (Parvy et al., 2012). This energy mobilization process is regulated by target-of-rapamycin (TOR), which is present in two distinct complexes, TORC1 and TORC2; the former directly responds to nutrients, whereas the latter is a component of the insulin signaling pathway. The intermediates of this signaling network are conserved in the fruit-fly (Table 1), although the TORC1 and insulin/TORC2 signaling branches can work independently in most Drosophila tissues, including the FB (Devilliers et al., 2021).
The FB also acts as a nutrient sensor to coordinate overall body growth and homeostasis through the production of insulin-like-peptides (Dilps) by a cluster of neurosecretory cells (NSCs) (Colombani et al., 2003). Moreover, similar to mammals, sugar metabolism in flies is modulated by an insulin/glucagon-like axis (Figures 1A,B; Oh et al., 2019). The Drosophila genome encodes eight Dilps (Brogiolo et al., 2001), Dilp2 and Dilp5, which are produced in the NSCs, are the major regulators of sugar homeostasis (Rulifson et al., 2002). Genetic ablation of the NSCs results in an overall reduced body size and in an increase in the levels of glucose and trehalose (disaccharide of glucose) that is the main circulating sugar in insects. This phenotype resembles the metabolic defects provoked by insulin deficiency in Type-I-diabetic patients. Conversely, the Drosophila adipokinetic hormone (AKH) produced by the neuroendocrine corpora cardiaca (CC) controls circulating sugar levels in a glucagon-like manner (Kim and Rulifson, 2004). Both NSCs and CC secrete their products into the haemolymph, close to the Drosophila heart equivalent, thereby favoring hormone distribution throughout the entire body. Secretion of AKH in Drosophila by the CC depends on an ATP-K + -dependent channel that directly responds to circulating sugar levels (Kim and Rulifson, 2004). A pair of glucose-sensing neurons plays a pivotal role in coordinating NSC and CC functions by activating Dilp secretion, while inhibiting AKH secretion (Figures 1A,B; Oh et al., 2019). Furthermore, secretion of Dilps by the NSCs strongly relies on FB messengers that relay nutritional cues (Colombani et al., 2003; Delanoue et al., 2016). The JAK-STAT ligand Unpaired2, is one such messenger, which can be functionally replaced using molecular genetic tools by human leptin, showing that as in mammals, fat cells produce an hormone in response to nutrient load to control feeding physiology (Rajan and Perrimon, 2012). Therefore, although the mammalian liver equivalent is not a discrete organ in Drosophila, hepatic functions and dysfunctions are closely conserved: the FB appears to be in charge of hepatic functions related to feeding, whereas the oenocytes accomplish functions related to fasting. Further, both in mammals and flies, integration of the TOR signaling network at the organismal level is central in controlling lipid and sugar metabolism in response to the nutritional status (Schmitt et al., 2015; Sanguesa et al., 2019).
Most of the mammalian transcriptional regulators involved in NAFLD are conserved in Drosophila (Table 1). Mondo, the ChREBP/MondoA homolog, regulates several metabolic routes in response to dietary sugar (Mattila et al., 2015). The Drosophila SREBP regulates the expression of genes required for FA synthesis (Kunte et al., 2006). However, consistent with insect sterol auxotrophy (Clark and Block, 1959), SREBP activity is not regulated by sterols, but by phosphatidylethanolamine (Dobrosotskaya et al., 2002). The best homologs of LXRs and PPARs are the Ecdysone receptor (EcR) and the nuclear receptor Eip75B, respectively (Parvy et al., 2014). Ecdysone is a steroid hormone that controls developmental transitions, whereas Eip75B is an intermediate of the ecdysone signaling, whose activity depends on nitric-oxide-synthase. Surprisingly, manipulating this signaling pathway in the ecdysone-producing gland, results in a dramatic changes in LD accumulation in FB cells, indicating that this signaling pathway also impinge on lipid homeostasis (Caceres et al., 2011). In summary, the metabolic routes and most of the regulatory genes that play a critical role in NAFLD are conserved in Drosophila. The activities of some regulatory gene products varies as compared to that of their mammalian counterparts, but remain connected to basal metabolism. Importantly, the phenotypes induced by loss-of-function of these genes can be used as reference criteria to monitor the efficiency and the adverse effects of drug compounds in preclinical trials.
Perspectives
Alternative models have largely contributed to our understanding of biological processes. Recent studies have shed light on potential targets for drug therapy, which need physiological validation prior to clinical trials. Thanks to a plethora of genetics tools to direct in a tissue-specific manner either over-expression or RNAi-inactivation of a gene of interest (Ugur et al., 2016; Senturk and Bellen, 2018), the fruit-fly model emerges as a powerful alternative for large-scale analyses. Collections of transgenic lines targeting a vast majority of the Drosophila genes, with a particular focus on the orthologs of disease-related-human genes, are available from Stock centers (Dietzl et al., 2007; Heigwer et al., 2018). This approach validated the functions of relevant proteins, such as CDGSH-iron-sulfur-domain-containing-protein-2, whose haplo-insufficiency causes NAFLD and promotes HCC development (Shen et al., 2017). This approach also identified genes that contribute to fat deposition (Pospisilik et al., 2010; Hoffmann et al., 2013) and whose dysregulation in humans can lead to obesity, diabetes, and NAFLD (Graham and Pick, 2017). Liver diseases are a burden in modern societies, especially NAFLD and HCC; in vivo investigations using Drosophila in translational approaches will be useful to validate enzymes and other molecules crucial for body homeostasis, increasing the chance to develop innovative therapeutic strategies.
Author Contributions
KCMM and JM contributed equally to the content of the work. Both authors contributed to the article and approved the submitted version.
Funding
This study was supported by the research grants from Fundação de Amparo à Pesquisa do Estado de São Paulo (FAPESP—2018/05286-3; 2019/16406-2) to KCMM and from the CNRS (PRC2744 CNRS-FAPESP) to JM.
Conflict of Interest
The authors declare that the research was conducted in the absence of any commercial or financial relationships that could be construed as a potential conflict of interest.
Publisher’s Note
All claims expressed in this article are solely those of the authors and do not necessarily represent those of their affiliated organizations, or those of the publisher, the editors and the reviewers. Any product that may be evaluated in this article, or claim that may be made by its manufacturer, is not guaranteed or endorsed by the publisher.
Acknowledgments
We thank Anne-Marie Pret for manuscript editing, the Fundação de Amparo à Pesquisa do Estado de São Paulo (FAPESP—2018/05286-3; 2019/16406-2) and the CNRS (PRC2744 CNRS-FAPESP).
References
Andersen, A., Lund, A., Knop, F. K., and Vilsboll, T. (2018). Glucagon-like peptide 1 in health and disease. Nat. Rev. Endocrinol. 14, 390–403. doi: 10.1038/s41574-018-0016-2
Anstee, Q. M., Reeves, H. L., Kotsiliti, E., Govaere, O., and Heikenwalder, M. (2019). From NASH to HCC: current concepts and future challenges. Nat. Rev. Gastroenterol. Hepatol. 16, 411–428. doi: 10.1038/s41575-019-0145-7
Asrani, S. K., Devarbhavi, H., Eaton, J., and Kamath, P. S. (2019). Burden of liver diseases in the world. J. Hepatol. 70, 151–171. doi: 10.1016/j.jhep.2018.09.014
Baenas, N., and Wagner, A. E. (2019). Drosophila melanogaster as an alternative model organism in nutrigenomics. Genes Nutr. 14:14. doi: 10.1186/s12263-019-0641-y
Bangi, E. (2020). Strategies for functional interrogation of big cancer data using drosophila cancer models. Int. J. Mol. Sci. 21:21113754. doi: 10.3390/ijms21113754
Bi, J., Xiang, Y., Chen, H., Liu, Z., Gronke, S., Kuhnlein, R. P., et al. (2012). Opposite and redundant roles of the two Drosophila perilipins in lipid mobilization. J. Cell Sci. 125, 3568–3577. doi: 10.1242/jcs.101329
Birse, R. T., Choi, J., Reardon, K., Rodriguez, J., Graham, S., Diop, S., et al. (2010). High-fat-diet-induced obesity and heart dysfunction are regulated by the TOR pathway in Drosophila. Cell Metab. 12, 533–544. doi: 10.1016/j.cmet.2010.09.014
Bohni, R., Riesgo-Escovar, J., Oldham, S., Brogiolo, W., Stocker, H., Andruss, B. F., et al. (1999). Autonomous control of cell and organ size by CHICO, a Drosophila homolog of vertebrate IRS1-4. Cell 97, 865–875. doi: 10.1016/s0092-8674(00)80799-0
Boland, B. B., Rhodes, C. J., and Grimsby, J. S. (2017). The dynamic plasticity of insulin production in beta-cells. Mol. Metab. 6, 958–973. doi: 10.1016/j.molmet.2017.04.010
Bossen, J., Uliczka, K., Steen, L., Pfefferkorn, R., Mai, M. M., Burkhardt, L., et al. (2019). An EGFR-Induced drosophila lung tumor model identifies alternative combination treatments. Mol. Cancer Ther. 18, 1659–1668. doi: 10.1158/1535-7163.MCT-19-0168
Brogiolo, W., Stocker, H., Ikeya, T., Rintelen, F., Fernandez, R., and Hafen, E. (2001). An evolutionarily conserved function of the Drosophila insulin receptor and insulin-like peptides in growth control. Curr. Biol. 11, 213–221. doi: 10.1016/s0960-9822(01)00068-9
Bruschi, F. V., Tardelli, M., Claudel, T., and Trauner, M. (2017). PNPLA3 expression and its impact on the liver: current perspectives. Hepat. Med. 9, 55–66. doi: 10.2147/HMER.S125718
Bryda, E. C. (2013). The Mighty Mouse: the impact of rodents on advances in biomedical research. Mo Med. 110, 207–211.
Byrne, C. D., and Targher, G. (2015). NAFLD: a multisystem disease. J. Hepatol. 62, S47–S64. doi: 10.1016/j.jhep.2014.12.012
Caceres, L., Necakov, A. S., Schwartz, C., Kimber, S., Roberts, I. J., and Krause, H. M. (2011). Nitric oxide coordinates metabolism, growth, and development via the nuclear receptor E75. Genes Dev. 25, 1476–1485. doi: 10.1101/gad.2064111
Chao, H. W., Chao, S. W., Lin, H., Ku, H. C., and Cheng, C. F. (2019). Homeostasis of glucose and lipid in non-alcoholic fatty liver disease. Int. J. Mol. Sci. 20:20020298. doi: 10.3390/ijms20020298
Chatterjee, S., Khunti, K., and Davies, M. J. (2017). Type 2 diabetes. Lancet 389, 2239–2251. doi: 10.1016/S0140-6736(17)30058-2
Clark, A. J., and Block, K. (1959). The absence of sterol synthesis in insects. J. Biol. Chem. 234, 2578–2582.
Coll-Tane, M., Krebbers, A., Castells-Nobau, A., Zweier, C., and Schenck, A. (2019). Intellectual disability and autism spectrum disorders ‘on the fly’: insights from Drosophila. Dis. Model Mech. 12:39180. doi: 10.1242/dmm.039180
Colombani, J., Raisin, S., Pantalacci, S., Radimerski, T., Montagne, J., and Leopold, P. (2003). A nutrient sensor mechanism controls Drosophila growth. Cell 114, 739–749. doi: 10.1016/s0092-8674(03)00713-x
Corrales, P., Vidal-Puig, A., and Medina-Gomez, G. (2018). PPARs and metabolic disorders associated with challenged adipose tissue plasticity. Int. J. Mol. Sci. 19, 19072124. doi: 10.3390/ijms19072124
Currie, E., Schulze, A., Zechner, R., Walther, T. C., and Farese, R. V. Jr. (2013). Cellular fatty acid metabolism and cancer. Cell Metab. 18, 153–161. doi: 10.1016/j.cmet.2013.05.017
Dai, Y., He, H., Li, S., Yang, L., Wang, X., Liu, Z., et al. (2020). Comparison of the efficacy of glucagon-like peptide-1 receptor agonists in patients with metabolic associated fatty liver disease: updated systematic review and meta-analysis. Front. Endocrinol. 11:622589. doi: 10.3389/fendo.2020.622589
Delanoue, R., Meschi, E., Agrawal, N., Mauri, A., Tsatskis, Y., McNeill, H., et al. (2016). Drosophila insulin release is triggered by adipose Stunted ligand to brain Methuselah receptor. Science 353, 1553–1556. doi: 10.1126/science.aaf8430
Devilliers, M., Garrido, D., Poidevin, M., Rubin, T., Le Rouzic, A., and Montagne, J. (2021). Differential metabolic sensitivity of insulin-like-response- and mTORC1-dependent overgrowth in drosophila fat cells. Genetics 2021:10. doi: 10.1093/genetics/iyaa010
Dietzl, G., Chen, D., Schnorrer, F., Su, K. C., Barinova, Y., Fellner, M., et al. (2007). A genome-wide transgenic RNAi library for conditional gene inactivation in Drosophila. Nature 448, 151–156. doi: 10.1038/nature05954
Diop, S. B., Bisharat-Kernizan, J., Birse, R. T., Oldham, S., Ocorr, K., and Bodmer, R. (2015). PGC-1/Spargel counteracts high-fat-diet-induced obesity and cardiac lipotoxicity downstream of TOR and Brummer ATGL Lipase. Cell Rep. 10, 1572–1584. doi: 10.1016/j.celrep.2015.02.022
Dobrosotskaya, I. Y., Seegmiller, A. C., Brown, M. S., Goldstein, J. L., and Rawson, R. B. (2002). Regulation of SREBP processing and membrane lipid production by phospholipids in Drosophila. Science 296, 879–883. doi: 10.1126/science.1071124
Doke, S. K., and Dhawale, S. C. (2015). Alternatives to animal testing: A review. Saudi Pharm. J. 23, 223–229. doi: 10.1016/j.jsps.2013.11.002
Dong, J., Feldmann, G., Huang, J., Wu, S., Zhang, N., Comerford, S. A., et al. (2007). Elucidation of a universal size-control mechanism in Drosophila and mammals. Cell 130, 1120–1133. doi: 10.1016/j.cell.2007.07.019
Eslam, M., and George, J. (2020). Genetic contributions to NAFLD: leveraging shared genetics to uncover systems biology. Nat. Rev. Gastroenterol. Hepatol. 17, 40–52. doi: 10.1038/s41575-019-0212-0
Fernandez-Hernandez, I., Scheenaard, E., Pollarolo, G., and Gonzalez, C. (2016). The translational relevance of Drosophila in drug discovery. EMBO Rep. 17, 471–472. doi: 10.15252/embr.201642080
Freires, I. A., Sardi, J. C., de Castro, R. D., and Rosalen, P. L. (2017). Alternative animal and non-animal models for drug discovery and development: bonus or burden? Pharm. Res. 34, 681–686. doi: 10.1007/s11095-016-2069-z
Friedman, S. L., Neuschwander-Tetri, B. A., Rinella, M., and Sanyal, A. J. (2018). Mechanisms of NAFLD development and therapeutic strategies. Nat. Med. 24, 908–922. doi: 10.1038/s41591-018-0104-9
Galicia-Garcia, U., Benito-Vicente, A., Jebari, S., Larrea-Sebal, A., Siddiqi, H., Uribe, K. B., et al. (2020). Pathophysiology of type 2 diabetes mellitus. Int. J. Mol. Sci. 21:21176275. doi: 10.3390/ijms21176275
Garrido, D., Rubin, T., Poidevin, M., Maroni, B., Le Rouzic, A., Parvy, J. P., et al. (2015). Fatty acid synthase cooperates with glyoxalase 1 to protect against sugar toxicity. PLoS Genet. 11:e1004995. doi: 10.1371/journal.pgen.1004995
Ghosh, A. C., Tattikota, S. G., Liu, Y., Comjean, A., Hu, Y., Barrera, V., et al. (2020). Drosophila PDGF/VEGF signaling from muscles to hepatocyte-like cells protects against obesity. Elife 9:56969. doi: 10.7554/eLife.56969
Gilliland, C. T., Zuk, D., Kocis, P., Johnson, M., Hay, S., Hajduch, M., et al. (2016). Putting translational science on to a global stage. Nat. Rev. Drug Discov. 15, 217–218. doi: 10.1038/nrd.2016.33
Gonzalez, C. (2013). Drosophila melanogaster: a model and a tool to investigate malignancy and identify new therapeutics. Nat Rev Cancer 13, 172–183. doi: 10.1038/nrc3461
Graham, P., and Pick, L. (2017). Drosophila as a model for diabetes and diseases of insulin resistance. Curr. Top. Dev. Biol. 121, 397–419. doi: 10.1016/bs.ctdb.2016.07.011
Gronke, S., Beller, M., Fellert, S., Ramakrishnan, H., Jackle, H., and Kuhnlein, R. P. (2003). Control of fat storage by a Drosophila PAT domain protein. Curr. Biol. 13, 603–606. doi: 10.1016/s0960-9822(03)00175-1
Gronke, S., Mildner, A., Fellert, S., Tennagels, N., Petry, S., Muller, G., et al. (2005). Brummer lipase is an evolutionary conserved fat storage regulator in Drosophila. Cell Metab. 1, 323–330. doi: 10.1016/j.cmet.2005.04.003
Gronke, S., Muller, G., Hirsch, J., Fellert, S., Andreou, A., Haase, T., et al. (2007). Dual lipolytic control of body fat storage and mobilization in Drosophila. PLoS Biol. 5:e137. doi: 10.1371/journal.pbio.0050137
Guida, M. C., Birse, R. T., Dall’Agnese, A., Toto, P. C., Diop, S. B., Mai, A., et al. (2019). Intergenerational inheritance of high fat diet-induced cardiac lipotoxicity in Drosophila. Nat. Commun. 10:193. doi: 10.1038/s41467-018-08128-3
Gutierrez, E., Wiggins, D., Fielding, B., and Gould, A. P. (2007). Specialized hepatocyte-like cells regulate Drosophila lipid metabolism. Nature 445, 275–280. doi: 10.1038/s41467-019-08492-8
Hader, T., Muller, S., Aguilera, M., Eulenberg, K. G., Steuernagel, A., Ciossek, T., et al. (2003). Control of triglyceride storage by a WD40/TPR-domain protein. EMBO Rep. 4, 511–516. doi: 10.1038/sj.embor.embor837
Havula, E., Teesalu, M., Hyotylainen, T., Seppala, H., Hasygar, K., Auvinen, P., et al. (2013). Mondo/ChREBP-Mlx-regulated transcriptional network is essential for dietary sugar tolerance in Drosophila. PLoS Genet. 9:e1003438. doi: 10.1371/journal.pgen.1003438
Heier, C., and Kuhnlein, R. P. (2018). Triacylglycerol Metabolism in Drosophila melanogaster. Genetics 210, 1163–1184. doi: 10.1534/genetics.118.301583
Heigwer, F., Port, F., and Boutros, M. (2018). RNA Interference (RNAi) Screening in Drosophila. Genetics 208, 853–874. doi: 10.1534/genetics.117.300077
Hirabayashi, S., Baranski, T. J., and Cagan, R. L. (2013). Transformed Drosophila cells evade diet-mediated insulin resistance through wingless signaling. Cell 154, 664–675. doi: 10.1016/j.cell.2013.06.030
Hmeljak, J., and Justice, M. J. (2019). From gene to treatment: supporting rare disease translational research through model systems. Dis. Model Mech. 12:39271. doi: 10.1242/dmm.039271
Hofbauer, H. F., Heier, C., Sen Saji, A. K., and Kuhnlein, R. P. (2020). Lipidome remodeling in aging normal and genetically obese Drosophila males. Insect. Biochem. Mol. Biol. 2020:103498. doi: 10.1016/j.ibmb.2020.103498
Hoffmann, J., Romey, R., Fink, C., and Roeder, T. (2013). Drosophila as a model to study metabolic disorders. Adv. Biochem. Eng. Biotechnol. 135, 41–61. doi: 10.1007/10_2013_196
Holtof, M., Lenaerts, C., Cullen, D., and Vanden Broeck, J. (2019). Extracellular nutrient digestion and absorption in the insect gut. Cell Tissue Res. 377, 397–414. doi: 10.1007/s00441-019-03031-9
Honegger, B., Galic, M., Kohler, K., Wittwer, F., Brogiolo, W., Hafen, E., et al. (2008). Imp-L2, a putative homolog of vertebrate IGF-binding protein 7, counteracts insulin signaling in Drosophila and is essential for starvation resistance. J. Biol. 7:10. doi: 10.1186/jbiol72
Huang, K., Chen, W., Zhu, F., Li, P. W., Kapahi, P., and Bai, H. (2019). RiboTag translatomic profiling of Drosophila oenocytes under aging and induced oxidative stress. BMC Genomics 20:50. doi: 10.1186/s12864-018-5404-4
Igaki, T., Pagliarini, R. A., and Xu, T. (2006). Loss of cell polarity drives tumor growth and invasion through JNK activation in Drosophila. Curr. Biol. 16, 1139–1146. doi: 10.1016/j.cub.2006.04.042
Ikura, Y. (2014). Transitions of histopathologic criteria for diagnosis of nonalcoholic fatty liver disease during the last three decades. World J. Hepatol. 6, 894–900. doi: 10.4254/wjh.v6.i12.894
Ipsen, D. H., Lykkesfeldt, J., and Tveden-Nyborg, P. (2018). Molecular mechanisms of hepatic lipid accumulation in non-alcoholic fatty liver disease. Cell Mol. Life Sci. 75, 3313–3327. doi: 10.1007/s00018-018-2860-6
Iredale, J. P. (2010). Darwin, development, Drosophila, and disease. Hepatology 51, 1–3. doi: 10.1002/hep.23235
Ji, D., Zhao, Q., Qin, Y., Tong, H., Wang, Q., Yu, M., et al. (2021). Germacrone improves liver fibrosis by regulating the PI3K/AKT/mTOR signalling pathway. Cell Biol. Int. 2021:11607. doi: 10.1002/cbin.11607
Johnson, A. A., and Stolzing, A. (2019). The role of lipid metabolism in aging, lifespan regulation, and age-related disease. Aging Cell 18:e13048. doi: 10.1111/acel.13048
Kim, S. K., and Rulifson, E. J. (2004). Conserved mechanisms of glucose sensing and regulation by Drosophila corpora cardiaca cells. Nature 431, 316–320. doi: 10.1038/nature02897
Kuchay, M. S., Choudhary, N. S., Mishra, S. K., and Misra, A. (2020). Nonalcoholic fatty liver disease should be considered for treatment allocation in standard management algorithms for type 2 diabetes. Diabetes Metab. Syndr. 14, 2233–2239. doi: 10.1016/j.dsx.2020.11.015
Kunte, A. S., Matthews, K. A., and Rawson, R. B. (2006). Fatty acid auxotrophy in Drosophila larvae lacking SREBP. Cell Metab. 3, 439–448. doi: 10.1016/j.cmet.2006.04.011
Lee, J. Y., Lee, J. H., and Cheon, C. K. (2020). Functional characterization of gomisin N in high-fat-induced drosophila obesity models. Int. J. Mol. Sci. 21:21197209. doi: 10.3390/ijms21197209
Li, S., Yu, X., and Feng, Q. (2019). Fat body biology in the last decade. Annu. Rev. Entomol. 64, 315–333. doi: 10.1146/annurev-ento-011118-112007
Li, X. D., He, S. S., Wan, T. T., and Li, Y. B. (2021). Liraglutide protects palmitate-induced INS-1 cell injury by enhancing autophagy mediated via FoxO1. Mol. Med. Rep. 23:11786. doi: 10.3892/mmr.2020.11786
Liao, S., Amcoff, M., and Nassel, D. R. (2020). Impact of high-fat diet on lifespan, metabolism, fecundity and behavioral senescence in Drosophila. Insect. Biochem. Mol. Biol. 2020:103495. doi: 10.1016/j.ibmb.2020.103495
Link, N., and Bellen, H. J. (2020). Using Drosophila to drive the diagnosis and understand the mechanisms of rare human diseases. Development 147:191411. doi: 10.1242/dev.191411
Liss, K. H., and Finck, B. N. (2017). PPARs and nonalcoholic fatty liver disease. Biochimie 136, 65–74. doi: 10.1016/j.biochi.2016.11.009
Lv, X., Dong, Y., Hu, L., Lu, F., Zhou, C., and Qin, S. (2020). Glucagon-like peptide-1 receptor agonists (GLP-1 RAs) for the management of nonalcoholic fatty liver disease (NAFLD): A systematic review. Endocrinol. Diabetes Metab. 3:e00163. doi: 10.1002/edm2.163
Mariano, V., Achsel, T., Bagni, C., and Kanellopoulos, A. K. (2020). Modelling learning and memory in drosophila to understand intellectual disabilities. Neuroscience 445, 12–30. doi: 10.1016/j.neuroscience.2020.07.034
Mattila, J., Havula, E., Suominen, E., Teesalu, M., Surakka, I., Hynynen, R., et al. (2015). Mondo-mlx mediates organismal sugar sensing through the gli-similar transcription factor sugarbabe. Cell Rep. 13, 350–364.
Men, T. T., Thanh, D. N., Yamaguchi, M., Suzuki, T., Hattori, G., Arii, M., et al. (2016). A Drosophila model for screening antiobesity agents. Biomed. Res. Int. 2016:6293163. doi: 10.1155/2016/6293163
Meng, Z., Liu, X., Li, T., Fang, T., Cheng, Y., Han, L., et al. (2021). The SGLT2 inhibitor empagliflozin negatively regulates IL-17/IL-23 axis-mediated inflammatory responses in T2DM with NAFLD via the AMPK/mTOR/autophagy pathway. Int. Immunopharmacol. 94:107492. doi: 10.1016/j.intimp.2021.107492
Miguel-Aliaga, I., Jasper, H., and Lemaitre, B. (2018). Anatomy and physiology of the digestive tract of drosophila melanogaster. Genetics 210, 357–396. doi: 10.1534/genetics.118.300224
Millet-Boureima, C., Porras Marroquin, J., and Gamberi, C. (2018). Modeling Renal Disease “On the Fly”. Biomed. Res. Int. 2018:5697436. doi: 10.1155/2018/5697436
Musselman, L. P., and Kuhnlein, R. P. (2018). Drosophila as a model to study obesity and metabolic disease. J. Exp. Biol. 221:163881. doi: 10.1242/jeb.163881
Nayak, N., and Mishra, M. (2019). Simple techniques to study multifaceted diabesity in the fly model. Toxicol. Mech. Methods 29, 549–560. doi: 10.1080/15376516.2019.1634171
Oh, Y., Lai, J. S., Mills, H. J., Erdjument-Bromage, H., Giammarinaro, B., Saadipour, K., et al. (2019). A glucose-sensing neuron pair regulates insulin and glucagon in Drosophila. Nature 574, 559–564. doi: 10.1038/s41586-019-1675-4
Oldham, S., Montagne, J., Radimerski, T., Thomas, G., and Hafen, E. (2000). Genetic and biochemical characterization of dTOR, the Drosophila homolog of the target of rapamycin. Genes Dev. 14, 2689–2694. doi: 10.1101/gad.845700
Pagliarini, R. A., and Xu, T. (2003). A genetic screen in Drosophila for metastatic behavior. Science 302, 1227–1231. doi: 10.1126/science.1088474
Palm, W., Sampaio, J. L., Brankatschk, M., Carvalho, M., Mahmoud, A., Shevchenko, A., et al. (2012). Lipoproteins in Drosophila melanogaster–assembly, function, and influence on tissue lipid composition. PLoS Genet. 8:e1002828. doi: 10.1371/journal.pgen.1002828
Pandey, U. B., and Nichols, C. D. (2011). Human disease models in Drosophila melanogaster and the role of the fly in therapeutic drug discovery. Pharmacol. Rev. 63, 411–436. doi: 10.1124/pr.110.003293
Papanikolopoulou, K., Mudher, A., and Skoulakis, E. (2019). An assessment of the translational relevance of Drosophila in drug discovery. Expert Opin. Drug Discov. 14, 303–313. doi: 10.1080/17460441.2019.1569624
Parise, E. R. (2019). Nonalcoholic Fatty Liver Disease (Nafld), More Than a Liver Disease. Arq. Gastroenterol. 56, 243–245. doi: 10.1590/S0004-2803.201900000-45
Parvy, J. P., Napal, L., Rubin, T., Poidevin, M., Perrin, L., Wicker-Thomas, C., et al. (2012). Drosophila melanogaster Acetyl-CoA-carboxylase sustains a fatty acid-dependent remote signal to waterproof the respiratory system. PLoS Genet. 8:e1002925. doi: 10.1371/journal.pgen.1002925
Parvy, J. P., Wang, P., Garrido, D., Maria, A., Blais, C., Poidevin, M., et al. (2014). Forward and feedback regulation of cyclic steroid production in Drosophila melanogaster. Development 141, 3955–3965. doi: 10.1242/dev.102020
Perrimon, N., Bonini, N. M., and Dhillon, P. (2016). Fruit flies on the front line: the translational impact of Drosophila. Dis. Model Mech. 9, 229–231. doi: 10.1242/dmm.024810
Piazza, N., and Wessells, R. J. (2011). Drosophila models of cardiac disease. Prog. Mol. Biol. Transl. Sci. 100, 155–210. doi: 10.1016/B978-0-12-384878-9.00005-4
Pospisilik, J. A., Schramek, D., Schnidar, H., Cronin, S. J., Nehme, N. T., Zhang, X., et al. (2010). Drosophila genome-wide obesity screen reveals hedgehog as a determinant of brown versus white adipose cell fate. Cell 140, 148–160. doi: 10.1016/j.cell.2009.12.027
Powell, E. E., Wong, V. W., and Rinella, M. (2021). Non-alcoholic fatty liver disease. Lancet 2021:3. doi: 10.1016/S0140-6736(20)32511-3
Rajan, A., and Perrimon, N. (2012). Drosophila cytokine unpaired 2 regulates physiological homeostasis by remotely controlling insulin secretion. Cell 151, 123–137. doi: 10.1016/j.cell.2012.08.019
Ramos, L. F., Silva, C. M., Pansa, C. C., and Moraes, K. C. M. (2021). Non-alcoholic fatty liver disease: molecular and cellular interplays of the lipid metabolism in a steatotic liver. Expert Rev. Gastroenterol. Hepatol. 15, 25–40. doi: 10.1080/17474124.2020.1820321
Rani, L., and Gautam, N. K. (2018). Drosophila renal organ as a model for identification of targets and screening of potential therapeutic agents for diabetic nephropathy. Curr. Drug Targets 19, 1980–1990. doi: 10.2174/1389450119666180808114533
Richardson, H. E., and Portela, M. (2018). Modelling Cooperative Tumorigenesis in Drosophila. Biomed. Res. Int. 2018:4258387. doi: 10.1155/2018/4258387
Rosato, V., Masarone, M., Dallio, M., Federico, A., Aglitti, A., and Persico, M. (2019). NAFLD and extra-hepatic comorbidities: current evidence on a multi-organ metabolic syndrome. Int. J. Environ. Res. Public Health 16:16183415. doi: 10.3390/ijerph16183415
Rulifson, E. J., Kim, S. K., and Nusse, R. (2002). Ablation of insulin-producing neurons in flies: growth and diabetic phenotypes. Science 296, 1118–1120. doi: 10.1126/science.1070058
Salazar, J. L., Yang, S. A., and Yamamoto, S. (2020). Post-developmental roles of notch signaling in the nervous system. Biomolecules 10:10070985. doi: 10.3390/biom10070985
Samji, P., Rajendran, M. K., Warrier, V. P., Ganesh, A., and Devarajan, K. (2021). Regulation of Hippo signaling pathway in cancer: A MicroRNA perspective. Cell Signal. 78:109858. doi: 10.1016/j.cellsig.2020.109858
Samuel, V. T., and Shulman, G. I. (2018). Nonalcoholic Fatty Liver Disease as a Nexus of Metabolic and Hepatic Diseases. Cell Metab. 27, 22–41. doi: 10.1016/j.cmet.2017.08.002
Sanguesa, G., Roglans, N., Baena, M., Velazquez, A. M., Laguna, J. C., and Alegret, M. (2019). mTOR is a key protein involved in the metabolic effects of simple sugars. Int. J. Mol. Sci. 20:200511117. doi: 10.3390/ijms20051117
Sanyal, A. J. (2019). Past, present and future perspectives in nonalcoholic fatty liver disease. Nat. Rev. Gastroenterol. Hepatol. 16, 377–386. doi: 10.1038/s41575-019-0144-8
Saxton, R. A., and Sabatini, D. M. (2017). mTOR Signaling in Growth, Metabolism, and Disease. Cell 168, 960–976. doi: 10.1016/j.cell.2017.02.004
Scherer, P. E., Williams, S., Fogliano, M., Baldini, G., and Lodish, H. F. (1995). A novel serum protein similar to C1q, produced exclusively in adipocytes. J. Biol. Chem. 270, 26746–26749. doi: 10.1074/jbc.270.45.26746
Schmitt, S., Ugrankar, R., Greene, S. E., Prajapati, M., and Lehmann, M. (2015). Drosophila Lipin interacts with insulin and TOR signaling pathways in the control of growth and lipid metabolism. J. Cell Sci. 128, 4395–4406. doi: 10.1242/jcs.173740
Senturk, M., and Bellen, H. J. (2018). Genetic strategies to tackle neurological diseases in fruit flies. Curr Opin. Neurobiol. 50, 24–32. doi: 10.1016/j.conb.2017.10.017
Seo, Y. S., Kim, J. H., Jo, N. Y., Choi, K. M., Baik, S. H., Park, J. J., et al. (2008). PPAR agonists treatment is effective in a nonalcoholic fatty liver disease animal model by modulating fatty-acid metabolic enzymes. J. Gastroenterol. Hepatol. 23, 102–109. doi: 10.1111/j.1440-1746.2006.04819.x
Shabalala, S. C., Dludla, P. V., Mabasa, L., Kappo, A. P., Basson, A. K., Pheiffer, C., et al. (2020). The effect of adiponectin in the pathogenesis of non-alcoholic fatty liver disease (NAFLD) and the potential role of polyphenols in the modulation of adiponectin signaling. Biomed. Pharmacother. 131:110785. doi: 10.1016/j.biopha.2020.110785
Shen, Z. Q., Chen, Y. F., Chen, J. R., Jou, Y. S., Wu, P. C., Kao, C. H., et al. (2017). CISD2 Haploinsufficiency Disrupts Calcium Homeostasis, Causes Nonalcoholic Fatty Liver Disease, and Promotes Hepatocellular Carcinoma. Cell Rep. 21, 2198–2211. doi: 10.1016/j.celrep.2017.10.099
Storelli, G., Nam, H. J., Simcox, J., Villanueva, C. J., and Thummel, C. S. (2019). Drosophila HNF4 Directs a switch in lipid metabolism that supports the transition to adulthood. Dev. Cell 48:e206. doi: 10.1016/j.devcel.2018.11.030
Tamarai, K., Bhatti, J. S., and Reddy, P. H. (2019). Molecular and cellular bases of diabetes: Focus on type 2 diabetes mouse model-TallyHo. Biochim. Biophys. Acta Mol. Basis Dis. 1865, 2276–2284. doi: 10.1016/j.bbadis.2019.05.004
Tennessen, J. M., Barry, W. E., Cox, J., and Thummel, C. S. (2014). Methods for studying metabolism in Drosophila. Methods 68, 105–115. doi: 10.1016/j.ymeth.2014.02.034
Teshome, G., Ambachew, S., Fasil, A., and Abebe, M. (2020). Efficacy of glucagon-like peptide-1 analogs in nonalcoholic fatty liver disease: a systematic review. Hepat. Med. 12, 139–151. doi: 10.2147/HMER.S265631
Toprak, U., Hegedus, D., Dogan, C., and Guney, G. (2020). A journey into the world of insect lipid metabolism. Arch. Insect. Biochem. Physiol. 104, e21682. doi: 10.1002/arch.21682
Ugur, B., Chen, K., and Bellen, H. J. (2016). Drosophila tools and assays for the study of human diseases. Dis. Model Mech. 9, 235–244. doi: 10.1242/dmm.023762
van Erk, M., van Luijk, J., Yang, F., Leeuwenburgh, S. C. G., Sanchez-Fernandez, M. J., Hermans, E., et al. (2021). A Systematic review and meta-analyses on animal models used in bone adhesive research. J. Orthop Res. 2021:25057. doi: 10.1002/jor.25057
Van Norman, G. A. (2019). Limitations of animal studies for predicting toxicity in clinical trials: is it time to rethink our current approach? JACC Basic Transl. Sci. 4, 845–854. doi: 10.1016/j.jacbts.2019.10.008
Villanueva, J. E., Livelo, C., Trujillo, A. S., Chandran, S., Woodworth, B., Andrade, L., et al. (2019). Time-restricted feeding restores muscle function in Drosophila models of obesity and circadian-rhythm disruption. Nat. Commun. 10, 2700. doi: 10.1038/s41467-019-10563-9
WGO (2020). Global burden of liver disease: A true burden on health sciences and economies!!., W.G. Organisation. Geneva: WGO.
Yamada, T., Habara, O., Kubo, H., and Nishimura, T. (2018). Fat body glycogen serves as a metabolic safeguard for the maintenance of sugar levels in Drosophila. Development 145:158865. doi: 10.1242/dev.158865
Yamada, T., Hironaka, K. I., Habara, O., Morishita, Y., and Nishimura, T. (2020). A developmental checkpoint directs metabolic remodelling as a strategy against starvation in Drosophila. Nat. Metab. 2, 1096–1112. doi: 10.1038/s42255-020-00293-4
Yang, J., McCart, C., Woods, D. J., Terhzaz, S., Greenwood, K. G., ffrench-Constant, R. H., et al. (2007). A Drosophila systems approach to xenobiotic metabolism. Physiol. Genomics 30, 223–231. doi: 10.1152/physiolgenomics.00018.2007
Yoo, J., Jeong, I. K., Ahn, K. J., Chung, H. Y., and Hwang, Y. C. (2021). Fenofibrate, a PPARalpha agonist, reduces hepatic fat accumulation through the upregulation of TFEB-mediated lipophagy. Metabolism 120:154798. doi: 10.1016/j.metabol.2021.154798
Yoon, M. S. (2017). The Role of Mammalian Target of Rapamycin (mTOR) in Insulin Signaling. Nutrients 9:9111176. doi: 10.3390/nu9111176
Keywords: alternative animal model, Drosophila, genetics, liver diseases, system biology
Citation: Moraes KCM and Montagne J (2021) Drosophila melanogaster: A Powerful Tiny Animal Model for the Study of Metabolic Hepatic Diseases. Front. Physiol. 12:728407. doi: 10.3389/fphys.2021.728407
Received: 21 June 2021; Accepted: 27 August 2021;
Published: 16 September 2021.
Edited by:
Jorge Simon, CIC bioGUNE, SpainReviewed by:
Diego Saenz De Urturi Indart, Yale University School of Medicine, United StatesAyman El-Kadi, University of Alberta, Canada
Copyright © 2021 Moraes and Montagne. This is an open-access article distributed under the terms of the Creative Commons Attribution License (CC BY). The use, distribution or reproduction in other forums is permitted, provided the original author(s) and the copyright owner(s) are credited and that the original publication in this journal is cited, in accordance with accepted academic practice. No use, distribution or reproduction is permitted which does not comply with these terms.
*Correspondence: Karen C. M. Moraes, a2NtLm1vcmFlc0B1bmVzcC5icg==; Jacques Montagne, amFjcXVlcy5tb250YWduZUBpMmJjLnBhcmlzLXNhY2xheS5mcg==