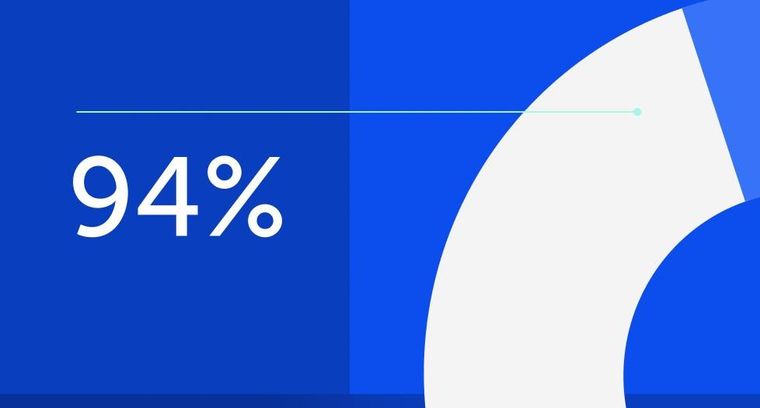
94% of researchers rate our articles as excellent or good
Learn more about the work of our research integrity team to safeguard the quality of each article we publish.
Find out more
MINI REVIEW article
Front. Physiol., 15 October 2021
Sec. Striated Muscle Physiology
Volume 12 - 2021 | https://doi.org/10.3389/fphys.2021.724010
Intermediate filaments (IFs) are a primary structural component of the cytoskeleton extending throughout the muscle cell (myofiber). Mechanotransduction, the process by which mechanical force is translated into a biochemical signal to activate downstream cellular responses, is crucial to myofiber function. Mechanical forces also act on the nuclear cytoskeleton, which is integrated with the myofiber cytoskeleton by the linker of the nucleoskeleton and cytoskeleton (LINC) complexes. Thus, the nucleus serves as the endpoint for the transmission of force through the cell. The nuclear lamina, a dense meshwork of lamin IFs between the nuclear envelope and underlying chromatin, plays a crucial role in responding to mechanical input; myofibers constantly respond to mechanical perturbation via signaling pathways by activation of specific genes. The nucleus is the largest organelle in cells and a master regulator of cell homeostasis, thus an understanding of how it responds to its mechanical environment is of great interest. The importance of the cell nucleus is magnified in skeletal muscle cells due to their syncytial nature and the extreme mechanical environment that muscle contraction creates. In this review, we summarize the bidirectional link between the organization of the nucleoskeleton and the contractile features of skeletal muscle as they relate to muscle function.
Intermediate filaments (IFs) contribute to force transmission, cellular integrity, and signaling in skeletal muscle. IFs are flexible, rod-shaped fibers averaging 10 nm in diameter, a size that is “intermediate” between microfilaments (7–8 nm) and microtubules (25 nm). IFs are classified into major families, with types I–IV localized in the cytoplasm, type V comprised of the lamins inside the nucleus, and type VI comprised of neural and lens proteins. In mature skeletal muscle, the IF cytoskeleton is composed predominantly of type III IF proteins, with the muscle-specific protein desmin being the most abundant. Desmin is an intracellular protein linking individual myofibrils laterally to each other and is important for the transmission of active and passive forces within the cytoskeleton (Shah et al., 2004). Other IFs in skeletal muscle include type IV IF proteins, such as synemin, paranemin, syncoilin and nestin, and IF type I and II keratins, all of which are important in cell integrity and cytoskeletal transmission of force (McCullagh et al., 2007; Lovering et al., 2011; Garcia-Pelagio et al., 2015).
In addition to the cytoplasmic IF network, the nuclei contain a network of lamin IF proteins. Like their cytoplasmic counterparts, the nuclear lamins are an important determinant of nuclear stiffness and are crucial in maintaining the integrity of the nuclei in the mechanically intense environment of a contracting muscle (Swift et al., 2013; Stephens et al., 2017). Many studies have investigated how forces initiated at the sarcolemma are transmitted throughout the cytoskeleton to activate downstream cellular responses (i.e., mechanotransduction). The signaling cascades that respond to changes in extracellular and intracellular mechanics have garnered the most focus (Tidball, 1991). However, recent studies show that forces acting on the cell, and changes in stiffness within the cell, can be transmitted directly to the nucleus and change the structure of the nuclear lamina and the associated chromatin (Stephens et al., 2017, 2019). Thus, the nucleus can act as an internal mechano-sensor in muscle cells (myofibers) (Navarro et al., 2016).
Although most eukaryotic cells have a single nucleus, myofibers are multinucleated. To support the large cytoplasm in the skeletal myofiber, the nuclei are evenly positioned along the cell periphery to maximize the distance between nuclei (Bruusgaard et al., 2006). This even spatial distribution of nuclei minimizes transport distances, such that each nucleus regulates the gene products in a fixed volume of a muscle fiber, a concept known as the myonuclear domain (Hall and Ralston, 1989). There is some flexibility in the size of myonuclear domains, but whether nuclear accretion is necessary for, versus the result of, increased muscle fiber size is less clear (Murach et al., 2018). Each nucleus contributes to the organization of the myofiber cytoskeleton, but importantly the nuclei throughout a single fiber also act as cellular mechano-sensors (Cho et al., 2017). Nuclear spatial distribution distributes the “centers” that organize the cytoskeleton and mechanical hubs that are critical mechanical sensors and responders. These hubs are then integrated with the force-producing myofibrils through the cytoplasmic IF network (Roman and Gomes, 2018). Thus, understanding the individual contributions of distinct IF networks and how these distinct pathways interact is critical to understanding muscle weakness with aging and disease.
The nucleus serves as the instruction manual for cells, containing the vast majority of the genome and the site of gene regulation. Thus, how it interprets stress to alter gene expression and its position to deliver cellular materials clearly contributes to cell behavior. Like most cell organelles, the nucleus is surrounded by a membrane. However, the nuclear envelope (NE) is composed of a double bilayer lipid membrane that forms a barrier between the nuclear interior and the cytoplasm (Figure 1). This NE consists of an outer nuclear membrane (ONM) and the inner nuclear membrane (INM) that are separated by the small (∼40 nm wide) perinuclear space. The two membranes are fused at the nuclear pore complexes (NPCs), which form channels responsible for nucleocytoplasmic trafficking.
Figure 1. Schematic representation of the linker of nucleoskeleton and cytoskeleton (LINC) complex. (A) The nuclear lamina is a meshwork of intermediate filaments (lamins), localized on the inner aspect of the inner nuclear membrane and helps to stabilize the nuclear envelope. The lamina is in direct contact with the chromatin, which is denser at the nuclear periphery. SUN proteins connect the inside of the nucleus to nesprins, which serve as a link between the nucleus and the myofiber cytoskeleton (including but not limited to microtubules, the actin, and desmin IFs), directly or indirectly via plectin, a cytoskeletal link in the cytoplasm. INM, inner nuclear membrane; ONM, outer nuclear membrane; NPC, nuclear pore complex. (B) Schematic showing only a few of the hundreds of myofibrils within a single muscle fiber (aka myofiber). Nuclei are located along the periphery of myofibers. Note that the LINC complex is located circumferentially around the nuclear membrane (aka nuclear envelope). Dotted white lines indicate the cytoskeletal IFs that connect the myofibrils to each other, to the sarcolemma, and to the nuclei.
The linker of nucleoskeleton and cytoskeleton (LINC) complex is a series of proteins that physically links the nucleoskeleton to the cytoskeleton and allows for transmission of force from the cytoplasm to the nucleus for many functions including nuclear spacing and gene regulation (Luxton and Starr, 2014). In mammals, nesprins (nuclear envelope spectrin repeat proteins), which contain a KASH domain, span the ONM and interact with microtubules, actin, and IFs on the cytoplasmic side of the nuclear envelope. SUN proteins (Sad1 and UNC-84 domain containing proteins) span the INM and interact directly with both the nuclear lamina and chromatin on the nucleoplasmic side of the nuclear envelope. Nesprins, specifically their KASH domains, interact with the SUN domain proteins within the perinuclear space (space between the INM and ONM). Thus, these proteins directly link protein networks that are critical to mechanotransduction. Furthermore, these proteins interact and are responsive to the mechanical environment (Jahed et al., 2021), adding a layer of complexity and tuning to this integrated network. This complexity is particularly important in muscle where LINC complex interactions are critical to maintain spacing between the two nuclear membranes (Cain et al., 2014).
Within the nucleus, the lamina is composed of ∼3.5 nm tetrameric lamin filaments and is approximately ∼14 nm thick. The lamina lines the INM, connects chromatin to the nuclear periphery, and directly interacts with transcription factors to regulate gene expression (Turgay et al., 2017). In addition, the NPCs, which allow for selective transport between the nucleus and the cytoplasm, interact with the LINC complex, the lamina, the cytoskeleton, and the chromatin to regulate gene expression (D’Angelo, 2018). Thus, the complex of proteins that regulate the movement of proteins in and out of the nucleus contacts the regulators of gene expression and the primary mechanotransduction networks. Furthermore, the NPC itself is mechanically responsive demonstrating the ability to expand in response to physical cues (Fichtman and Harel, 2014).
The lamin family consists of the A-type lamins and the B-type lamins, and are expressed as products of three differentially regulated genes. Lamin A, C, and less abundant, AΔ10 and C2, are all splice-isoforms of the LMNA gene. Lamin B1 is encoded by LMNB1 and Lamin B2 and B3 are encoded by LMNB2 gene. Different lamin isoforms interact to form the lamina and have many binding partners, including chromatin, LINC complex proteins and NPCs. Similar to other IF family members, lamins are composed of a α-helical rod domain with a short N-terminal head and a long tail domain containing an Ig-fold. The rod and head domains are essential for lamin assembly, but other regions are also important for proper protein function. The tail domain with the globular Ig-fold contains most of the interaction sites for lamin-binding partners. Lamins govern numerous biological functions, both biophysical and biochemical (Turgay et al., 2017). This includes determination of nuclear size, shape, stiffness (Dahl et al., 2004), regulation of transcription factors and chromatin, and control of cell polarization and migration (Davidson and Lammerding, 2014). However, the relative importance of individual lamin networks remains an open question highlighted by the segregation of lamin A and lamin B proteins within an integrated meshwork (Guilluy et al., 2014).
Inside the nucleus are threads of chromatin composed of DNA and associated proteins characterized as either open, transcriptionally active euchromatin or condensed, inactive heterochromatin. Chromatin associated with the NE has been described as silent chromatin, which interacts with the nuclear lamins, while active chromatin interacts with the nuclear pore complex proteins (Kalverda et al., 2008). INM proteins interact with the lamina and/or chromatin in a tissue-specific manner. Although the interactions between lamins and chromatin are critical to the organization of each, the network of chromatin within the nucleus provides a functionally separate mechanotransduction mechanism (Turgay et al., 2017; Dos Santos and Toseland, 2021).
Mechanical forces are conducted through myofibers and into nuclei to regulate muscle development, hypertrophy, and homeostasis. One mechanism that allows myofibers to respond to the constant mechanical force they are subjected to is the regulation of protein levels. The stability, assembly and transcription of the nuclear lamins, LINC complex, and the NPCs are required for proper mechano-signaling, as all of these components respond to mechanical stress (Dahl et al., 2008). Although much remains to be learned, there is reason to expect that the changes in protein levels are a direct response to cellular mechanics. Extracellular and cytoplasmic forces are transmitted across the nuclear envelope to the nuclear interior, where they can cause deformation of chromatin and nuclear bodies (Guilluy et al., 2014). Furthermore, the proportion of phosphorylated lamin A, a proxy for the percentage of lamin A that is incorporated into the network, is dependent on cellular mechanics (Swift and Discher, 2014). Because gene expression is dependent on chromatin organization (Nava et al., 2020), which can be affected by interaction with the lamina or directly by the application of force, it is likely that muscle development and homeostasis are closely tied to the mechanical state of the cell (Bronshtein et al., 2015). The nuclear lamina is crucial to transducing mechanical signals to the nucleus and plays an important role in DNA organization, DNA repair, and transcriptional regulation [reviewed in Gruenbaum and Foisner (2015)].
Beyond its gene regulation, the nuclear lamina provides critical structural support that protects the nucleus against nuclear rupture and damage (Cho et al., 2019). Additionally, the nuclear lamina serves to receive mechanical signals and thus has been called a molecular “shock absorber” of the nucleus (Osmanagic-Myers et al., 2015). Mechanical stiffness and viscosity of nuclei are dependent on A-type lamins, while deformability and elasticity of nuclei are dependent on B-type lamins. Protein expression of lamin A scales with increasing tissue stiffness (Swift et al., 2013). In addition, increased mechanical load of the nucleus suppresses lamin A/C phosphorylation, with degradation of lamin A/C, downstream to phosphorylation (Buxboim et al., 2014).
Changes to the nuclear envelope and its associated proteins can result in unstable regulation of the mechanotransduction pathway in response to mechanical stress, which drives phenotypic changes in muscle, such as weakness seen with sarcopenia and muscular dystrophies (Lacavalla et al., 2021). Mutations in the LMNA gene are associated with diseases having a wide range of phenotypes, including striated muscle dysfunction, and are collectively referred to as “laminopathies,” this includes effects on aging, for example Hutchinson-Gilford progeria (Table 1). Mutations in the genes encoding LINC complex proteins are also associated with a wide range of skeletal muscle dysfunctions, many of which have phenotypes that overlap with those of laminopathies (Bonne and Quijano-Roy, 2013). Interestingly, we recently reported reduced expression of lamin-B1 in aged skeletal muscle (Iyer et al., 2021). Synaptic nuclei (nuclei anchored underneath the neuromuscular junction) in aged skeletal muscle have reduced expression of LMNA gene (Gao et al., 2020). We have also observed changes in gene expression of LINC complex proteins in murine models of Duchenne muscular dystrophy (Iyer et al., 2016). However, the mechanisms in this reduction and its effect on aging and diseased skeletal muscle remain to be elucidated.
Table 1. Diseases associated with mutations in genes for lamin proteins and proteins associated with the LINC complex.
Muscles with mutations in the LMNA gene or absence of LMNA gene also display aberrant nuclear morphology, stability, mechanics, and DNA damage (Earle et al., 2020). Similarly, muscles with mutations in genes for LINC complex proteins such as emerin and nesprin-1 also display aberrant nuclear morphology, mechanics, and DNA damage (Fidzianska et al., 1998; Zhang et al., 2010). Mutations in the LINC complex proteins, such as SUN and nesprin, are disease modifiers that worsen the phenotype of laminopathies by further disrupting mechanotransduction pathways due to insufficient linkage to the cytoskeleton (Zhang et al., 2007; Meinke et al., 2014). Nuclear positioning is also impacted in muscles with mutations in lamin and nuclear envelope genes, with the appearance of central and clustered nuclei, as well as a reduced number of synaptic nuclei. The influence of the lamina and LINC appears to be bidirectional. That is, alterations in the lamina result in aberrant expression and organization of the LINC complex and the cytoskeleton. Conversely, mutations/deficiencies in the LINC complex or cell cytoskeleton can result in altered expression and organization of the nuclear lamina (Meinke et al., 2014). Thus, mutations of genes related to the nucleocytoskeleton pathway can severely impact mechanotransduction, and ultimately muscle function.
Proteins such as YAP and MLK1 are indicators reflecting nuclear mechanotransduction, with increased signaling in the nucleus in response to mechanical load. Interestingly, when lamin A is depleted, nuclear levels of both YAP and MLK1 are increased (Wiggan et al., 2020). Nuclear YAP is also increased in muscle stem cells with mutations in the LMNA and SYNE-1 (gene for nesprin-1 protein) genes (Owens et al., 2020a). Conversely, overexpression of LMNA results in a decrease in nuclear YAP accumulation (Swift et al., 2013). While cyclic strain typically increases the nuclear accumulation of YAP (due to increased mechanical load), with mutations in LMNA gene, cyclic strain decreases the nuclear accumulation of YAP (Bertrand et al., 2014). Such studies support the notion of a link between lamin IFs and nucleo-cytoskeletal signaling. Recently, we and others observed increased YAP nuclear accumulation and signaling in aging skeletal muscles (Yoshida et al., 2019; Iyer et al., 2021), which also had a reduction in lamin-B1, but whether such findings are linked is still unknown.
Similar to YAP, ERK 1/2 signaling typically increases in skeletal muscle with mechanical loading, such as after exercise or repeated contractions (Kramer and Goodyear, 2007). Compared to healthy controls, ERK 1/2 signaling is increased in skeletal muscle with LMNA mutations (Muchir et al., 2007), and cortical neurons depleted of lamin B1 (Giacomini et al., 2016). Such results point to the nuclear lamina playing a pivotal role in nuclear mechanotransduction. Interestingly, inhibition of ERK 1/2 in skeletal muscle with LMNA mutations improves function (Muchir et al., 2013). Future studies will hopefully determine whether pharmacological interventions to nuclear mechanotransduction pathways can help ameliorate skeletal muscle weakness in laminopathies and sarcopenia.
Skeletal muscle has the ability to adapt to loading exercise, with consequent increases in muscle mass (hypertrophy) and strength. However, in muscles with mutations in the LMNA gene there is a reduction in the amount of hypertrophy, presumably due to abnormal mechanical signaling (Owens et al., 2020b). Mutations in the LMNA gene also result in cardiac dysfunction following exercise (Cattin et al., 2016). Patients with mutations in LMNA gene who are active have more cardiac dysfunction compared to patients with mutations in the LMNA gene who are sedentary. This could be due to mechanically weakened nuclei, which respond poorly to load, further exacerbating the dysfunction (Earle et al., 2020). Thus, further studies are needed to determine optimal exercise regimens for patients to maintain health and to avoid adverse outcomes.
Increasing stiffness of the extracellular matrix results in decreased phosphorylation of lamin A and consequently decreased degradation of lamin A (Cho et al., 2019). Skeletal muscle stiffness increases following loading exercise and injury (Green et al., 2012; Silver et al., 2021), however, the direct linkage between changes in lamin and exercise/injury in skeletal muscle remains unclear. Expression of nesprin-1, but not SUN-1, increases following endurance exercise in skeletal muscle (Bostani et al., 2020). This increase in nesprin-1 expression could be the result of myogenesis, which often occurs with endurance exercise (Abreu and Kowaltowski, 2020). However, in mature muscle fibers, nesprin-1 can be replaced in part by nesprin-2, while the SUN proteins remained unchanged during fiber maturation (Randles et al., 2010). Research into the role of the nuclear envelope in skeletal muscle exercise is still in its infancy and much more work is needed to further elucidate the relationship between nuclear mechanics and muscle performance.
How mutations in genes for lamin IFs and NE proteins affect skeletal muscle function is complicated and studies are still being performed to understand the underlying mechanisms. Defects in the nuclear lamina, the LINC complex, and the NPCs can impair the ability of nuclei, and therefore the muscle cell, to respond appropriately to mechanical forces. Understanding the mechanisms of impaired mechanotransduction in aging and diseased muscle is likely to shed light on strategies designed to prevent and treat skeletal muscle dysfunction with disease and aging.
All authors listed have made a substantial, direct and intellectual contribution to the work, and approved it for publication.
This work was supported by grants to RL from the University of Maryland Claude D. Pepper Older Americans Independence Center (UM-OAIC), to RL and EF from the National Institutes of Health (R56AR073193), and to SI from the National Institutes of Health (K01AR074048) and the Muscular Dystrophy Association development grant (MDA 577897).
The authors declare that the research was conducted in the absence of any commercial or financial relationships that could be construed as a potential conflict of interest.
All claims expressed in this article are solely those of the authors and do not necessarily represent those of their affiliated organizations, or those of the publisher, the editors and the reviewers. Any product that may be evaluated in this article, or claim that may be made by its manufacturer, is not guaranteed or endorsed by the publisher.
Abreu, P., and Kowaltowski, A. J. (2020). Satellite cell self-renewal in endurance exercise is mediated by inhibition of mitochondrial oxygen consumption. J. Cachexia Sarcopenia Muscle 11, 1661–1676. doi: 10.1002/jcsm.12601
Bertrand, A. T., Ziaei, S., Ehret, C., Duchemin, H., Mamchaoui, K., Bigot, A., et al. (2014). Cellular microenvironments reveal defective mechanosensing responses and elevated YAP signaling in LMNA-mutated muscle precursors. J. Cell Sci. 127, 2873–2884.
Bonne, G., and Quijano-Roy, S. (2013). Emery-Dreifuss muscular dystrophy, laminopathies, and other nuclear envelopathies. Handb. Clin. Neurol. 113, 1367–1376. doi: 10.1016/B978-0-444-59565-2.00007-1
Bostani, M., Rahmati, M., and Mard, S. A. (2020). The effect of endurance training on levels of LINC complex proteins in skeletal muscle fibers of STZ-induced diabetic rats. Sci. Rep. 10:8738. doi: 10.1038/s41598-020-65793-5
Bronshtein, I., Kepten, E., Kanter, I., Berezin, S., Lindner, M., Redwood, A. B., et al. (2015). Loss of lamin A function increases chromatin dynamics in the nuclear interior. Nat. Commun. 6:8044. doi: 10.1038/ncomms9044
Bruusgaard, J. C., Liestol, K., and Gundersen, K. (2006). Distribution of myonuclei and microtubules in live muscle fibers of young, middle-aged, and old mice. J. Appl. Physiol. 100, 2024–2030.
Buxboim, A., Swift, J., Irianto, J., Spinler, K. R., Dingal, P. C., Athirasala, A., et al. (2014). Matrix elasticity regulates lamin-A,C phosphorylation and turnover with feedback to actomyosin. Curr. Biol. 24, 1909–1917. doi: 10.1016/j.cub.2014.07.001
Cain, N. E., Tapley, E. C., McDonald, K. L., Cain, B. M., and Starr, D. A. (2014). The SUN protein UNC-84 is required only in force-bearing cells to maintain nuclear envelope architecture. J. Cell Biol. 206, 163–172. doi: 10.1083/jcb.201405081
Cattin, M. E., Ferry, A., Vignaud, A., Mougenot, N., Jacquet, A., Wahbi, K., et al. (2016). Mutation in lamin A/C sensitizes the myocardium to exercise-induced mechanical stress but has no effect on skeletal muscles in mouse. Neuromuscul. Disord. 26, 490–499. doi: 10.1016/j.nmd.2016.05.010
Cho, S., Irianto, J., and Discher, D. E. (2017). Mechanosensing by the nucleus: From pathways to scaling relationships. J. Cell Biol. 216, 305–315.
Cho, S., Vashisth, M., Abbas, A., Majkut, S., Vogel, K., Xia, Y., et al. (2019). Mechanosensing by the Lamina Protects against Nuclear Rupture, DNA Damage, and Cell-Cycle Arrest. Dev. Cell. 49, 920–935 e925. doi: 10.1016/j.devcel.2019.04.020
Dahl, K. N., Kahn, S. M., Wilson, K. L., and Discher, D. E. (2004). The nuclear envelope lamina network has elasticity and a compressibility limit suggestive of a molecular shock absorber. J. Cell Sci. 117, 4779–4786. doi: 10.1242/jcs.01357
Dahl, K. N., Ribeiro, A. J., and Lammerding, J. (2008). Nuclear shape, mechanics, and mechanotransduction. Circ. Res. 102, 1307–1318.
D’Angelo, M. A. (2018). Nuclear pore complexes as hubs for gene regulation. Nucleus 9, 142–148. doi: 10.1080/19491034.2017.1395542
Davidson, P. M., and Lammerding, J. (2014). Broken nuclei–lamins, nuclear mechanics, and disease. Trends Cell Biol. 24, 247–256.
Dos Santos, A., and Toseland, C. P. (2021). Regulation of Nuclear Mechanics and the Impact on DNA Damage. Int. J. Mol. Sci. 22:3178. doi: 10.3390/ijms22063178
Earle, A. J., Kirby, T. J., Fedorchak, G. R., Isermann, P., Patel, J., Iruvanti, S., et al. (2020). Mutant lamins cause nuclear envelope rupture and DNA damage in skeletal muscle cells. Nat. Mater. 19, 464–473. doi: 10.1038/s41563-019-0563-5
Fichtman, B., and Harel, A. (2014). Stress and aging at the nuclear gateway. Mech. Ageing Dev. 135, 24–32. doi: 10.1016/j.mad.2014.01.003
Fidzianska, A., Toniolo, D., and Hausmanowa-Petrusewicz, I. (1998). Ultrastructural abnormality of sarcolemmal nuclei in Emery-Dreifuss muscular dystrophy (EDMD). J. Neurol. Sci. 159, 88–93. doi: 10.1016/s0022-510x(98)00130-0
Gao, N., Zhao, K., Cao, Y., Ren, X., Jing, H., Xing, G., et al. (2020). A Role of Lamin A/C in Preventing Neuromuscular Junction Decline in Mice. J. Neurosci. 40, 7203–7215. doi: 10.1523/JNEUROSCI.0443-20.2020
Garcia-Pelagio, K. P., Muriel, J., Neill, O., Desmond, P. F., Lovering, R. M., Lund, L., et al. (2015). Myopathic Changes in Murine Skeletal Muscle Lacking Synemin. Am. J. Physiol. Cell Physiol. 308, C448–C462. doi: 10.1152/ajpcell.00331.2014
Giacomini, C., Mahajani, S., Ruffilli, R., Marotta, R., and Gasparini, L. (2016). Lamin B1 protein is required for dendrite development in primary mouse cortical neurons. Mol. Biol. Cell. 27, 35–47. doi: 10.1091/mbc.E15-05-0307
Green, M. A., Sinkus, R., Gandevia, S. C., Herbert, R. D., and Bilston, L. E. (2012). Measuring changes in muscle stiffness after eccentric exercise using elastography. NMR Biomed. 25, 852–858. doi: 10.1002/nbm.1801
Gruenbaum, Y., and Foisner, R. (2015). Lamins: nuclear intermediate filament proteins with fundamental functions in nuclear mechanics and genome regulation. Annu. Rev. Biochem. 84, 131–164.
Guilluy, C., Osborne, L. D., Van Ll, Sharek, L., Superfine, R., Garcia-Mata, R., et al. (2014). Isolated nuclei adapt to force and reveal a mechanotransduction pathway in the nucleus. Nat. Cell Biol. 16, 376–381.
Iyer, S. R., Hsia, R. C., Folker, E. S., and Lovering, R. M. (2021). Age-dependent changes in nuclear-cytoplasmic signaling in skeletal muscle. Exp. Gerontol. 150:111338. doi: 10.1016/j.exger.2021.111338
Iyer, S. R., Shah, S. B., Valencia, A. P., Schneider, M. F., Hernandez-Ochoa, E. O., Stains, J. P., et al. (2016). Altered nuclear dynamics in MDX myofibers. J. Appl. Physiol. 122, 470–481. doi: 10.1152/japplphysiol.00857.2016
Jahed, Z., Domkam, N., Ornowski, J., Yerima, G., and Mofrad, M. R. K. (2021). Molecular models of LINC complex assembly at the nuclear envelope. J. Cell Sci. 134:jcs258194. doi: 10.1242/jcs.258194
Kalverda, B., Roling, M. D., and Fornerod, M. (2008). Chromatin organization in relation to the nuclear periphery. FEBS Lett. 582, 2017–2022. doi: 10.1016/j.febslet.2008.04.015
Kramer, H. F., and Goodyear, L. J. (2007). Exercise, MAPK, and NF-kappaB signaling in skeletal muscle. J. Appl. Physiol. 103, 388–395. doi: 10.1152/japplphysiol.00085.2007
Lacavalla, M. A., Cisterna, B., Zancanaro, C., and Malatesta, M. (2021). Ultrastructural immunocytochemistry shows impairment of RNA pathways in skeletal muscle nuclei of old mice: A link to sarcopenia? Eur. J. Histochem. 65:3229. doi: 10.4081/ejh.2021.3229
Lovering, R. M., O’Neill, A., Muriel, J. M., Prosser, B. L., Strong, J., and Bloch, R. J. (2011). Physiology, Structure, and Susceptibility to Injury of Skeletal Muscle in Mice Lacking Keratin 19-Based and Desmin-Based Intermediate Filaments 1. Am. J. Physiol. Cell Physiol. 300, C803–13.
Luxton, G. W., and Starr, D. A. (2014). KASHing up with the nucleus: novel functional roles of KASH proteins at the cytoplasmic surface of the nucleus. Curr. Opin. Cell Biol. 28, 69–75. doi: 10.1016/j.ceb.2014.03.002
McCullagh, K. J., Edwards, B., Poon, E., Lovering, R. M., Paulin, D., and Davies, K. E. (2007). Intermediate filament-like protein syncoilin in normal and myopathic striated muscle. Neuromuscul. Disord. 17, 970–979.
Meinke, P., Mattioli, E., Haque, F., Antoku, S., Columbaro, M., Straatman, K. R., et al. (2014). Muscular dystrophy-associated SUN1 and SUN2 variants disrupt nuclear-cytoskeletal connections and myonuclear organization. PLoS Genet. 10:e1004605. doi: 10.1371/journal.pgen.1004605
Muchir, A., Kim, Y. J., Reilly, S. A., Wu, W., Choi, J. C., and Worman, H. J. (2013). Inhibition of extracellular signal-regulated kinase 1/2 signaling has beneficial effects on skeletal muscle in a mouse model of Emery-Dreifuss muscular dystrophy caused by lamin A/C gene mutation. Skelet Muscle 3:17. doi: 10.1186/2044-5040-3-17
Muchir, A., Pavlidis, P., Decostre, V., Herron, A. J., Arimura, T., Bonne, G., et al. (2007). Activation of MAPK pathways links LMNA mutations to cardiomyopathy in Emery-Dreifuss muscular dystrophy. J. Clin. Invest. 117, 1282–1293. doi: 10.1172/JCI29042
Murach, K. A., Englund, D. A., Dupont-Versteegden, E. E., McCarthy, J. J., and Peterson, C. A. (2018). Myonuclear Domain Flexibility Challenges Rigid Assumptions on Satellite Cell Contribution to Skeletal Muscle Fiber Hypertrophy. Front. Physiol. 9:635. doi: 10.3389/fphys.2018.00635
Nava, M. M., Miroshnikova, Y. A., Biggs, L. C., Whitefield, D. B., Metge, F., Boucas, J., et al. (2020). Heterochromatin-Driven Nuclear Softening Protects the Genome against Mechanical Stress-Induced Damage. Cell 181, 800–817 e822. doi: 10.1016/j.cell.2020.03.052
Navarro, A. P., Collins, M. A., and Folker, E. S. (2016). The nucleus is a conserved mechanosensation and mechanoresponse organelle. Cytoskeleton 73, 59–67.
Osmanagic-Myers, S., Dechat, T., and Foisner, R. (2015). Lamins at the crossroads of mechanosignaling. Genes Dev. 29, 225–237. doi: 10.1101/gad.255968.114
Owens, D. J., Fischer, M., Jabre, S., Moog, S., Mamchaoui, K., Butler-Browne, G., et al. (2020a). Lamin Mutations Cause Increased YAP Nuclear Entry in Muscle Stem Cells. Cells 9:816. doi: 10.3390/cells9040816
Owens, D. J., Messeant, J., Moog, S., Viggars, M., Ferry, A., Mamchaoui, K., et al. (2020b). Lamin-Related Congenital Muscular Dystrophy Alters Mechanical Signaling and Skeletal Muscle Growth. Int. J. Mol. Sci. 22:306. doi: 10.3390/ijms22010306
Randles, K. N., Lam le, T., Sewry, C. A., Puckelwartz, M., Furling, D., Wehnert, M., et al. (2010). Nesprins, but not sun proteins, switch isoforms at the nuclear envelope during muscle development. Dev. Dyn. 239, 998–1009. doi: 10.1002/dvdy.22229
Roman, W., and Gomes, E. R. (2018). Nuclear positioning in skeletal muscle. Semin. Cell Dev. Biol. 82, 51–56. doi: 10.1016/j.semcdb.2017.11.005
Shah, S. B., Davis, J., Weisleder, N., Kostavassili, I., McCulloch, A. D., Ralston, E., et al. (2004). Structural and functional roles of desmin in mouse skeletal muscle during passive deformation. Biophys. J. 86, 2993–3008.
Silver, J. S., Gunay, K. A., Cutler, A. A., Vogler, T. O., Brown, T. E., Pawlikowski, B. T., et al. (2021). Injury-mediated stiffening persistently activates muscle stem cells through YAP and TAZ mechanotransduction. Sci. Adv. 7:501. doi: 10.1126/sciadv.abe4501
Stephens, A. D., Banigan, E. J., Adam, S. A., Goldman, R. D., and Marko, J. F. (2017). Chromatin and lamin A determine two different mechanical response regimes of the cell nucleus. Mol. Biol. Cell. 28, 1984–1996. doi: 10.1091/mbc.E16-09-0653
Stephens, A. D., Liu, P. Z., Kandula, V., Chen, H., Almassalha, L. M., Herman, C., et al. (2019). Physicochemical mechanotransduction alters nuclear shape and mechanics via heterochromatin formation. Mol. Biol. Cell. 30, 2320–2330. doi: 10.1091/mbc.E19-05-0286
Swift, J., and Discher, D. E. (2014). The nuclear lamina is mechano-responsive to ECM elasticity in mature tissue. J. Cell Sci. 127, 3005–3015. doi: 10.1242/jcs.149203
Swift, J., Ivanovska, I. L., Buxboim, A., Harada, T., Dingal, P. C., Pinter, J., et al. (2013). Nuclear lamin-A scales with tissue stiffness and enhances matrix-directed differentiation. Science 341:1240104.
Turgay, Y., Eibauer, M., Goldman, A. E., Shimi, T., Khayat, M., Ben-Harush, K., et al. (2017). The molecular architecture of lamins in somatic cells. Nature 543, 261–264. doi: 10.1038/nature21382
Wiggan, O., DeLuca, J. G., Stasevich, T. J., and Bamburg, J. R. (2020). Lamin A/C deficiency enables increased myosin-II bipolar filament ensembles that promote divergent actomyosin network anomalies through self-organization. Mol. Biol. Cell. 31, 2363–2378. doi: 10.1091/mbc.E20-01-0017-T
Yoshida, N., Endo, J., Kinouchi, K., Kitakata, H., Moriyama, H., Kataoka, M., et al. (2019). (Pro)renin receptor accelerates development of sarcopenia via activation of Wnt/YAP signaling axis. Aging Cell. 18:e12991. doi: 10.1111/acel.12991
Zhang, J., Felder, A., Liu, Y., Guo, L. T., Lange, S., Dalton, N. D., et al. (2010). Nesprin 1 is critical for nuclear positioning and anchorage. Hum. Mol. Genet 19, 329–341.
Keywords: nucleus, intermediate filaments, lamins, mechanotransduction, aging, muscle disease
Citation: Iyer SR, Folker ES and Lovering RM (2021) The Nucleoskeleton: Crossroad of Mechanotransduction in Skeletal Muscle. Front. Physiol. 12:724010. doi: 10.3389/fphys.2021.724010
Received: 11 June 2021; Accepted: 24 September 2021;
Published: 15 October 2021.
Edited by:
Francesca Soglia, University of Bologna, ItalyReviewed by:
Carol Witczak, Indiana University School of Medicine, United StatesCopyright © 2021 Iyer, Folker and Lovering. This is an open-access article distributed under the terms of the Creative Commons Attribution License (CC BY). The use, distribution or reproduction in other forums is permitted, provided the original author(s) and the copyright owner(s) are credited and that the original publication in this journal is cited, in accordance with accepted academic practice. No use, distribution or reproduction is permitted which does not comply with these terms.
*Correspondence: Richard M. Lovering, cmxvdmVyaW5nQHNvbS51bWFyeWxhbmQuZWR1
Disclaimer: All claims expressed in this article are solely those of the authors and do not necessarily represent those of their affiliated organizations, or those of the publisher, the editors and the reviewers. Any product that may be evaluated in this article or claim that may be made by its manufacturer is not guaranteed or endorsed by the publisher.
Research integrity at Frontiers
Learn more about the work of our research integrity team to safeguard the quality of each article we publish.