- 1School of Kinesiology, The University of Western Ontario, London, ON, Canada
- 2Faculty of Kinesiology, University of Calgary, Calgary, ON, Canada
During competitive freestyle swimming, the change of direction requires a turn followed by ∼15 m of underwater kicking at various intensities that require a ∼5 s breath-hold (BH). Upon surfacing, breathing must be regulated, as head rotation is necessary to facilitate the breath while completing the length of the pool (∼25 s). This study compared the respiratory and muscle deoxygenation responses of regulated breathing vs. free breathing, during these 25–5 s cycles. It was hypothesized that with the addition of a BH and sprint during heavy-intensity (HVY) exercise, oxygen uptake (VO2) and oxygen saturation (SatO2) would decrease, and muscle deoxygenation ([HHb]) and total hemoglobin ([Hbtot]) would increase. Ten healthy male participants (24 ± 3 years) performed 4–6 min trials of HVY cycling in the following conditions: (1) continuous free breathing (CONLD); (2) continuous with 5 s BH every 25 s (CONLD-BH); (3) Fartlek (FLK), a 5 s sprint followed by 25 s of HVY; and (4) a combined Fartlek and BH (FLK-BH). Continuous collection of VO2 and SatO2, [Hbtot], and [HHb] via breath-by-breath gas analysis and near-infrared spectroscopy (normalized to baseline) was performed. Breathing frequency and tidal volumes were matched between CONLD and CONLD-BH and between FLK and FLK-BH. As a result, VO2 was unchanged between CONLD (2.12 ± 0.35 L/min) and CONLD-BH (2.15 ± 0.42 L/min; p = 0.116) and between FLK (2.24 ± 0.40 L/min) and FLK-BH (2.20 ± 0.45 L/min; p = 0.861). SatO2 was higher in CONLD (63 ± 1.9%) than CONLD-BH (59 ± 3.3%; p < 0.001), but was unchanged between FLK (61 ± 2.2%) and FLK-BH (62 ± 3.1%; p = 0.462). Δ[Hbtot] is higher in CONLD (3.3 ± 1.6 μM) than CONLD-BH (-2.5 ± 1.2 μM; Δ177%; p < 0.001), but was unchanged between FLK (2.0 ± 1.6 μM) and FLK-BH (0.82 ± 1.4 μM; p = 0.979). Δ[HHb] was higher in CONLD (7.3 ± 1.8μM) than CONLD-BH (7.0 ± 2.0μM; Δ4%; p = 0.011) and lower in FLK (6.7 ± 1.8μM) compared to FLK-BH (8.7 ± 2.4 μM; p < 0.001). It is suggested that the unchanged VO2 between CONLD and CONLD-BH was supported by increased deoxygenation as reflected by decreased Δ[Hbtot] and blunted Δ[HHb], via apneic-driven redistribution of blood flow away from working muscles, which was reflected by the decreased SatO2. However, the preserved VO2 during FLK-BH vs. FLK has been underpinned by an increase in [HHb].
Introduction
Competitive swimming requires the performance of high-intensity work while performing regular periods of apnea. For example, the swimming “flip-turn” and push-off technique, facilitating a change in direction at the end of the pool, is a maneuver that requires high-power output (PO) of the lower extremities (kicking) combined with apnea. International swimming rules stipulate that the swimmers must surface after covering a maximum of 15 m from the underwater kicking phase. This underwater kicking phase endures for ∼5 s. While the backstroke affords swimmers to breathe freely during swimming as their face is not underwater, the breaths during the front crawl, performed in the prone position, is confined to the rhythmical rotation of the body along the sagittal plane in the position to when the face is exposed to the air once every stroke cycle. This imposes a regulated breathing paradigm dictated by the specific characteristics of this swimming stroke.
Previous work by Lim et al. (2018) simulated the cardiorespiratory response of the lower extremities to multiple laps of backstroke swimming by repeating cycles of 25 s of free-breathing, the approximate duration of swimming one length of a 50 m pool, with 5 s of breath-holding (BH) as are experienced during the aforementioned flip-turn and push-off phases. However, since these trials were performed on a cycle ergometer on land, as opposed to in water with facial submersion, their observations provided only a cursory understanding of the physiological effects of the ventilatory techniques that may be common to swimmers. Some swimmers, with better underwater hydrodynamics, may choose to perform a 5 s sprint kick, in combination with the BH, with the hope that the added swimming speed will offset any negative physiological effects of the sprint in the latter stages of the race. These apneic exercise interventions were separated into four 6 min bouts on a cycle ergometer performed at an intensity corresponding to a PO of 50% of the difference between the lactate threshold (LT) and maximal oxygen consumption (VO2max) of an individual (Δ50%): (1) constant load (CONLD), (2) CONLD with 5 s BHs every 25 s (CONLD-BH), (3) Fartlek (25 s at Δ50% and a 5 s sprint) (FLK), and (4) FLK with 5 s BHs every 25 s (FLK-BH). The addition of a BH, which reduced breathing opportunities within CONLD (CONLD-BH), elicited an increase in minute ventilation (VE) during the 25 s free-breathing periods, along with the elevated deoxyhemoglobin-to-VO2 ratio ([HHb]/VO2) during the 5 s BHs. This reflected greater local muscle deoxygenation that supported O2 utilization and mitigated the repeated hypoxia of the 5 s BH. They suggested that the stimulus for increased ventilation was underpinned by the transient increases in the end-tidal partial pressure of CO2 (PETCO2) and decreases in O2 (PETO2) during the 5 s BHs, as these factors have been indicated to modulate ventilatory responses (Whipp and Davis, 1979). Similar to previous apnea studies, the observed decrease in mean concentration of total hemoglobin ([Hbtot]) and mean concentration of deoxyhemoglobin ([HHb]) during the 5 s apnea period of CONLD-BH compared to CONLD suggests an overall reduction in blood flow and greater reliance on O2 extraction to support O2 utilization, reflecting the previously observed apnea-driven O2 conservation response at the level of the muscle (Andersson et al., 2004). The BH did not impose a great enough physiological stress to cause a reduction in PO to either the CONLD-BH or FLK-BH condition. However, the added stress of the sprint resulted in decreased VO2, despite increases in [Hbtot] and [HHb]. The redistribution of blood flow and reduced cardiac output (Andersson et al., 2004) have been replicated earlier under apneic conditions (Lindholm and Linnarsson, 2002; Hoffmann et al., 2005) and are similarly experienced during deep diving (Ferrigno et al., 1997). The supply of Hb to the blood has also been observed during these dives as a function of splenic contractions (Hurford et al., 1990), which elicits greater O2 and CO2 carrying capacities in blood. This deep diving response does not appear to extend to high-intensity knee extensions (Kennedy et al., 2008) or cycling exercise (Chacaroun et al., 2019) under hypoxic conditions.
Prone swimming strokes impose a regulated breathing paradigm that should abolish the transient increases in VE during free-breathing. However, to what extent this is true remains unknown and could be evaluated by imposing a paradigm where regulation of frequency of breathing (fB) and inspiratory tidal volume (VTI) of each individual are controlled for each minute of exercise.
Therefore, the purpose of this study was to investigate respiration and muscle deoxygenation under regulated breathing vs. free-breathing conditions between the aforementioned protocols. We hypothesized that with the reduced breathing opportunities from the regulated ventilation during the 25 s breathing periods, (1) mean VO2 would decrease, and mean VCO2 would decrease in CONLD-BH compared to CONLD and in FLK-BH compared to FLK. Moreover, (2) in comparison to the conflicting results between CONLD-BH and FLK-BH as suggested by Lim et al. (2018), we expected to observe increases in [Hbtot], [HHb], and [La–] to account for the increased physiological stress of regulated breathing during both the conditions.
Materials and Methods
Ten males (mean 23.7 ± 2.5 years) participated in this study after their written informed consent was given. Inclusion criteria were that the participants were healthy and active (i.e., exercising one to three times per week). Smokers and individuals who take medication for the cardiopulmonary system were excluded. None of the participants were involved in sports. However, they were participating in 30–60 min of moderate- to heavy-intensity (HVY) resistance training of the upper and lower extremities two to three times per week. All procedures were approved by the Western University Research Ethics Board for Health Sciences Research Involving Human Participants and were in accordance with the 1964 Helsinki Declaration and its later amendments or comparable ethical standards. Baseline characteristics for the participants are summarized in Table 1.
Test Conditions
The activity levels of participants were maintained throughout the duration of this study. Participants were asked to avoid caffeine for 6 h before each test. Five tests were performed on an electronically braked cycle ergometer, each on a separate day with a minimum interval of 48 h between the tests. Participants wore a nose clip to prevent nose breathing and a mouthpiece to facilitate gas exchange analysis and ventilatory measurements.
Ramp Incremental Test (Day 1)
Participants were instructed to complete a ramp test to exhaustion on a cycle ergometer. The PO was increased by 25 W/min, while a cadence of 70 rpm was maintained. Verbal encouragement was given to participants to maximize their performance. When participants were unable to cycle above 60 rpm for more than five consecutive seconds, the protocol was stopped. This incremental test was used to determine the maximal aerobic capacity (VO2max), peak power output (PPO), and the estimated LT. Above the LT, excess [H+] derived from an increase in lactate production is buffered by the carbonic anhydrase reaction, which yields greater CO2 output in relation to O2 utilization than that observed when exercising below the LT (Beaver et al., 1986). This increased CO2 results in an increase in VE, which is reflective of the LT. Therefore, this estimated LT was used as a proxy of the actual LT as the exercise intensity at which VCO2/VO2 began to increase disproportionately to increases in PO (also referred to as the gas exchange threshold).
Constant Load Exercise (CONLD)
A constant load step procedure (CONLD) was performed at the PO at 20% of the difference between LT and VO2max (Δ20%) of an individual. A 4 min baseline period at 20 W was followed by constant load cycling at 70 rpm for 6 min while free-breathing in order to stabilize the gas exchange response. Five fB measurements were recorded at 1, 2, 3, 4, and 5 min after the onset of the PO.
Constant Load With BHs (CONLD-BH)
Another square-wave test similar to CONLD was performed by participants at Δ20% at 70 rpm. Every 25 s from the beginning of the warm-up, participants performed a 5 s BH. A 5 s countdown was given before each BH period. During the remaining 25 s of the 30 s cycles, breathing was regulated to CONLD: participants matched their fB and VTI for each minute of exercise to that achieved during the CONLD condition, with the guidance of a metronome and feedback from the attending researcher, respectively.
Fartlek
After the initial 4 min warm-up, participants commenced HVY work at Δ20% for 6 min with a cadence of 70 rpm. Every 25 s, a 5 s interval at the PPO of an individual was performed. Subjects could breathe freely. Like in CONLD, fB measurements were recorded for each of the first 5 min after the onset of exercise.
Fartlek With BHs (FLK-BH)
Finally, a similar protocol to FLK was performed by participants. Notably, 5 s BHs were incorporated every 25 s, so the sprints were performed under apnea. fB and Vt were matched to the minute-by-minute measurements taken in FLK.
The ramp protocol was performed always on day 1. Each participant was required to complete CONLD and FLK before CONLD-BH and FLK-BH, respectively, to establish the ventilatory thresholds for the BH protocols. Otherwise, each participant was randomly prescribed one of the six possible orders to complete the submaximal exercise conditions (e.g., CONLD, FLK, FLK-BH, and CONLD-BH) by an online random sequence generator. Comparisons of the physiological outcomes were made within the performance of each participant between apneic and non-apneic conditions, such that participants acted as their own controls.
Experimental Considerations
During pilot testing, we noted that no individuals were able to complete the 6 min work at the prescribed Δ50% PO with the periodic BHs and/or sprints. The PO was reduced until all participants were able to complete these 6 min trials. This PO corresponded with a prescribed PO of Δ20%.
Measurements
Breath-by-breath gas exchanges and ventilatory rates at the mouth were assessed using a mass spectrometer (Innovision, AMIS 2000, Lindvedvej, Denmark) and are described in detail elsewhere (Babcock et al., 1994). Briefly, flow rates during inspiration and expiration were determined with a low dead space bidirectional turbine (Alpha Technologies, Laguna Beach, CA, United States, VMM 110) and pneumotach (Hans Rudolph, Shawnee, Kansas, United States, Model 4813) calibrated with a 3 L syringe. Gas samples at the mouth were analyzed for O2, CO2, and N2 concentrations. Changes in concentrations of gases were matched to the corresponding increase or decrease in volumes of the gases. There was a 20 ms interval between collection samples that were sent electronically to a computer to analyze individual breaths. Each breath started with inspiration and ended with expiration. Therefore, each 5 s BH was recorded as a single breath.
The procedures for near-infrared spectrometry (NIRS) data collection were similar to those previously described (Inglis et al., 2017). Continuous measurement of the quadriceps was performed with a NIRS device (Oxiplex TS, model 95,205, ISS, Champaign, IL, United States). Laser diodes pulsed quickly (110 MHz) at two different wavelengths near the infrared region (690 and 828 nm). These were connected to a plastic probe that was placed midway between the lateral epicondyle and the greater trochanter of the femur on the belly of the vastus lateralis muscle head. An elastic strap secured the device in place. An optically dense, black vinyl sheet was placed over the device to prevent the exposure to extraneous light. A tensor bandage was wrapped gently around the leg of the participant to secure the NIRS device to the site of interest and to further prevent the intrusion of external light into the site of NIRS measurement. Care was taken to ensure that no blood flow occlusion occurred to the leg. Deoxygenated hemoglobin concentration ([HHb]) and oxygenated hemoglobin ([HbO]) were measured, whereas [Hbtot] and tissue oxygen saturation (SatO2) were derived with this apparatus. [Hbtot] was calculated as the sum of [HHb] and [HbO], and SatO2 was estimated as the percentage of [HHb] to [Hbtot]. To account for individual differences in absolute tissue absorption, [HHb] and [Hbtot] were adjusted to baseline values (Δ[HHb] and Δ[Hbtot], respectively).
Rubbing alcohol was applied to the left index finger of each participant before each test. Blood lactate concentrations ([La–]) were taken 3 min pre- and post-exercise for each test. A lancet (ACCU-CHEK Safe-T-Pro Plus) exposed blood on the finger, which was examined by the SensLab GmbH Lactate SCOUT arterialized-capillary lactate analyzer.
Analysis
Gas exchange and NIRS data were cleaned by the removal of aberrant data points that were at least 3 SDs from the local mean. The data were interpolated linearly to convert from breath-by-breath to 1 s intervals. Then, data points were averaged into 5 s bins. This analysis technique has been described previously (Keir et al., 2014). [HHb] and [Hbtot] were adjusted to zero with baseline as described earlier. These baselines represented the average of 60 s before the square-wave change in PO. Δ[HHb]/VO2 was determined as the ratio of the normalized [HHb] to VO2. Between-group comparisons on gas exchange and NIRS variables were performed from the start of the square-wave change in PO (the exercise on-transient) to the end of the bout (0–360 s). Within-group comparisons were performed between the first 25 s and the last 5 s of each 30 s cycle for VO2 and VCO2. Lamarra et al. (1987) described this mono-exponential function that models the on-transient VO2 curve during a step transition:
where y(t) represents VO2 as a function of time during the transition to the steady state of new PO. yBSL is VO2 before the transition, Ap is the amplitude (increase above yBSL), t is the dependent time variable, TD is the time delay, and τ is the time constant (time that elapses for 63% of the response to occur). The curve was fit to the data by applying the Levenberg–Marquardt algorithm for non-linear least squares analysis (Origin 9.7; OriginLab, Northampton, MA, United States).
Statistics
Ten participants were recruited based on a sample size power calculation of the measured VO2max. It was found that 10 participants were sufficient (80% power) to calculate pre vs. post VO2max (α = 0.05) to within an SD of ± 30 mL with the consideration of a 20% dropout rate. Mean analyses from 0 s (start of square-wave change in PO) to 360 s (end of exercise) (n = 10) of VO2, VCO2, PETO2, PETCO2, VE, fB, VTI, Δ[HHb], Δ[Hbtot], SatO2, and Δ[HHb]/VO2 between conditions (CONLD, CONLD-BH, FLK, and FLK-BH) were performed by a one-way repeated measures (1-RM) analysis of variance (ANOVA). [La–] was compared between conditions and time (PRE vs. POST) by a two-way RM (2-RM) ANOVA. The first 25 s and last 5 s of each apneic cycle were analyzed for VO2 and VCO2 via a 2-RM ANOVA. The Shapiro–Wilk and Brown–Forsythe tests were performed to assess the normality and heteroscedasticity of the data, respectively. The 1-RM ANOVA comparisons for VCO2, PETO2, PETCO2, VE, fB, VTI, Δ[HHb], Δ[Hbtot], SatO2, and Δ[HHb]/VO2 were performed by a Friedman RM ANOVA on ranks due to a rejection of either or both tests, whereas mean VO2 was evaluated with a parametric 1-RM ANOVA. Data are reported as mean ± SD. The statistical significance threshold was p < 0.05.
Results
Participants successfully matched VE between CONLD and CONLD-BH (p = 0.889) and between FLK and FLK-BH (p = 0.889) with regard to the onset of the square-wave increase in PO and exercise cessation (Table 2 and Figure 1A). This was supported by the sustained mean fB between CONLD and CONLD-BH (p = 0.72) and between FLK and FLK-BH (p = 0.99) (Figure 1B) and mean VTI between CONLD and CONLD-BH (p = 0.953) (Figure 1C). VTI was statistically greater in FLK-BH compared to FLK (p < 0.001) by ∼80 mL/min.
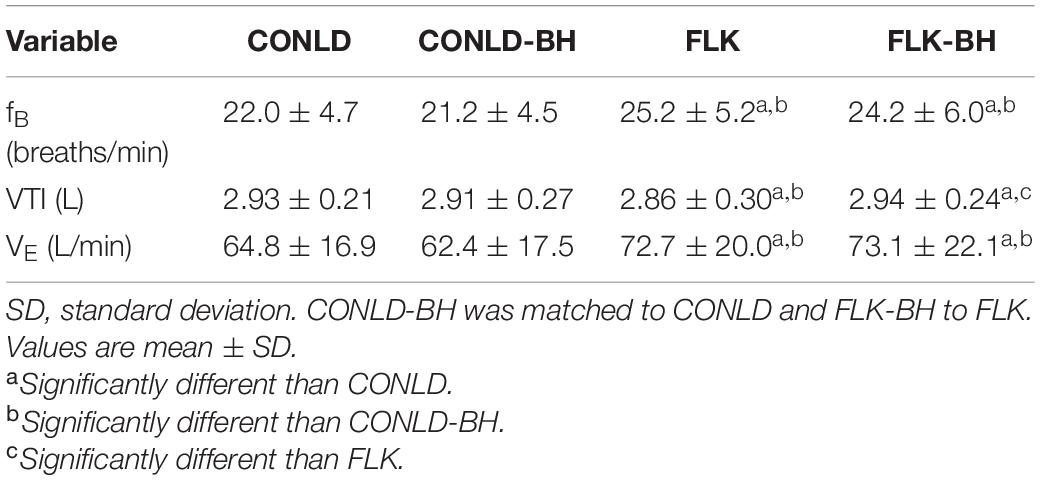
Table 2. Breathing frequency (fB), inspiratory tidal volume (VTI), and minute ventilation (VE) under each condition.
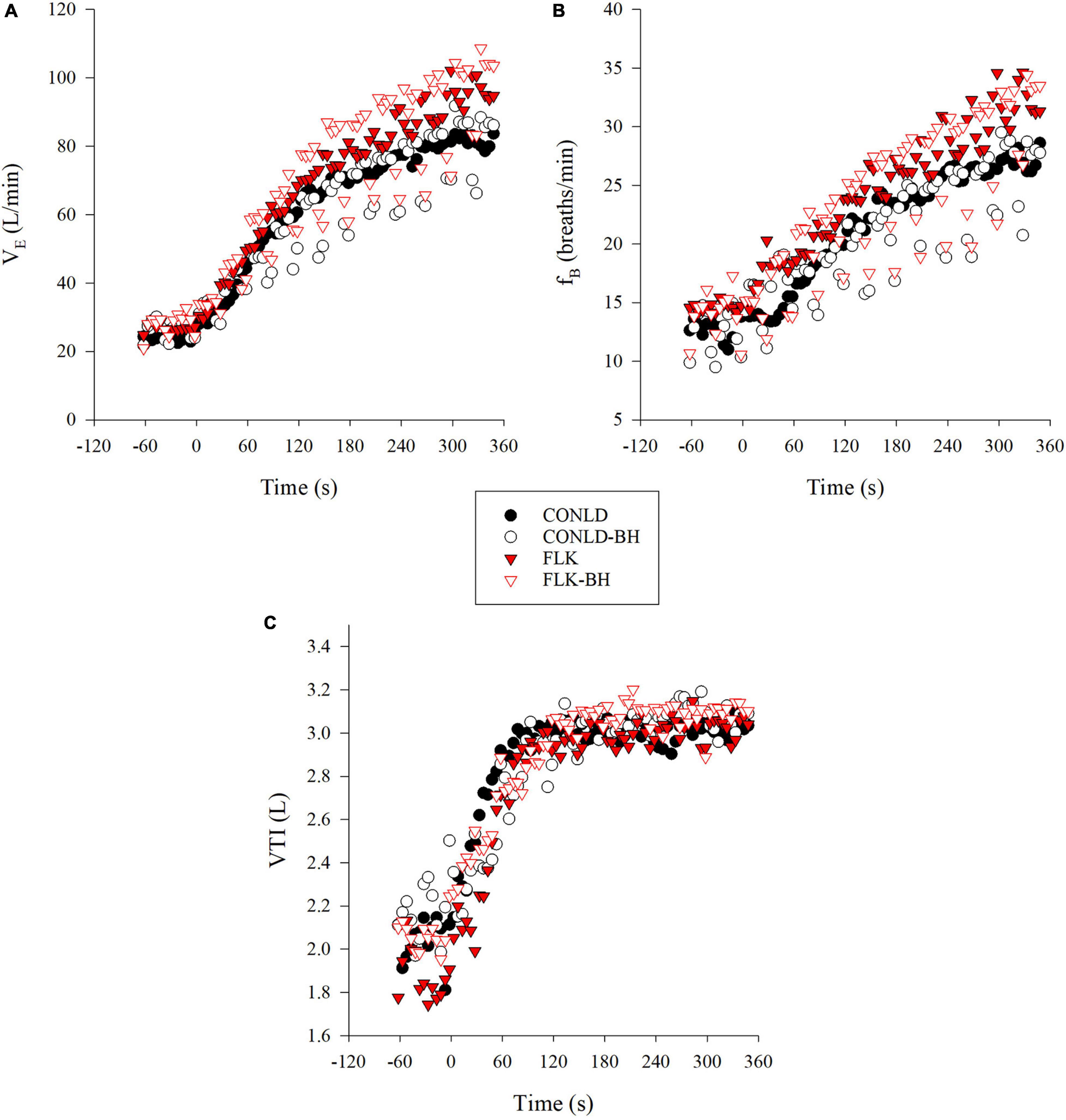
Figure 1. Breathing data during CONLD (filled circles), CONLD-BH (open circles), FLK (filled red triangles), and FLK-BH (open red triangles). (A) Minute ventilation (VE), (B) frequency of breathing (fB), and (C) inspiratory tidal volume (VTI). Time 0 marks the start of the exercise transition. Data were interpolated from breath-by-breath to second-by-second and 5 s averaged for graphical representation.
Gas Exchange Variables
Mean VO2 from time 0 to 360 s was similar between CONLD and CONLD-BH (p = 0.406) and between FLK and FLK-BH (p = 0.165) (Table 3 and Figure 2A). VO2 was greater in FLK and FLK-BH than in CONLD (p < 0.001 for both comparisons) and greater in FLK than CONLD-BH (p < 0.001) over the same time period. Mean VO2 for the last 5 s of each 30 s cycle was greater in CONLD than CONLD-BH (p = 0.001) and greater in FLK than in FLK-BH (p < 0.001) and CONLD-BH (p < 0.001) (Table 4). Mean PETO2 was greater in CONLD than CONLD-BH (p < 0.001) but was unchanged in FLK compared to FLK-BH (p = 0.682) (Table 3 and Figure 2C). PETO2 was lower in CONLD and CONLD-BH than in FLK and FLK-BH (p < 0.001 for all comparisons) (Table 3 and Figure 2C). PETCO2 was lower in CONLD than CONLD-BH (p < 0.001) but was greater in FLK than FLK-BH (p < 0.001) (Table 3 and Figure 2D). VCO2 from 0 s to the end of exercise was similar between CONLD and CONLD-BH (p = 0.914) and between FLK and FLK-BH (p = 0.641), but was greater in FLK and FLK-BH than in CONLD and CONLD-BH (p < 0.001 for all comparisons) (Table 3 and Figure 2B).
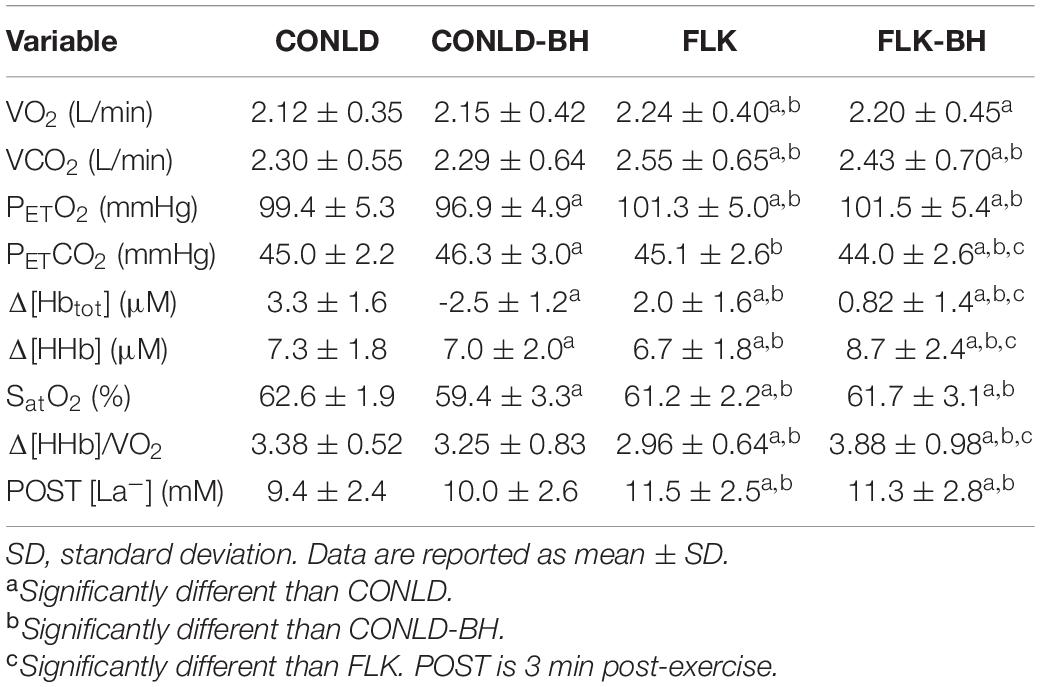
Table 3. Mean outcome measures for each of the four conditions, namely, CONLD, CONLD-BH, FLK, and FLK-BH from 0 to 360 s.
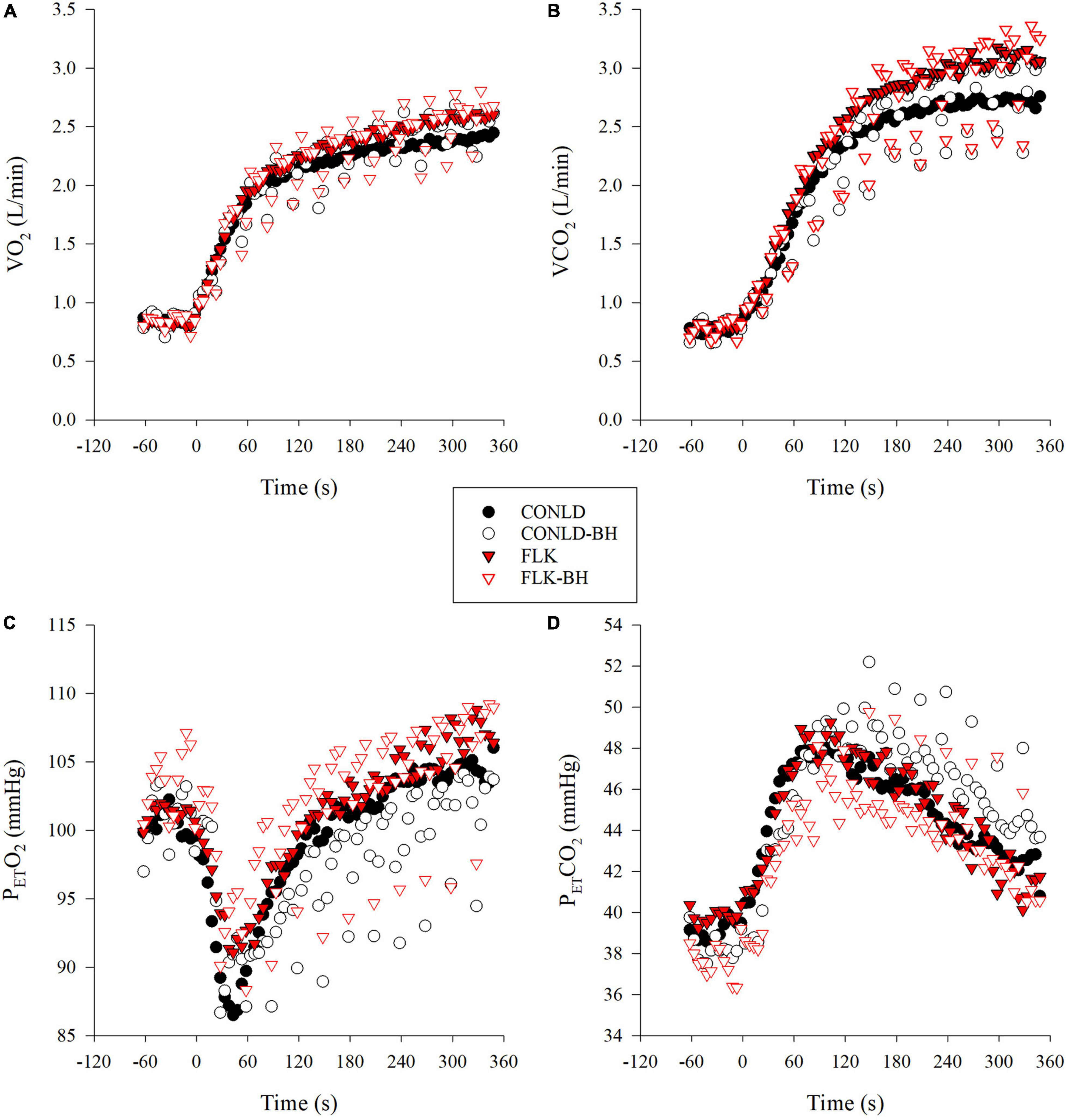
Figure 2. Mean participant respiratory variables during constant A20% work (CONLD, filled circles), constant A20% work with 5 s BHs every 30 s (CONLD-BH, open circles), ?20% work with 5 s sprints at peak power output (PPO) every 30 s (FLK, filled red triangles) and A20% work with 5 s BHs, and sprints at PPO every 30 s (FLK-BH, open red triangles) for 6 min. (A) Oxygen consumption (VO2), (B) carbon dioxide elimination (VCO2), (C) end-tidal partial pressure of oxygen (PETO2), and (D) end-tidal partial pressure of carbon dioxide (PETCO2). Time 0 marks the start of the exercise transition. Data were interpolated from breath-by-breath to second-by-second and 5 s averaged for graphical representation.
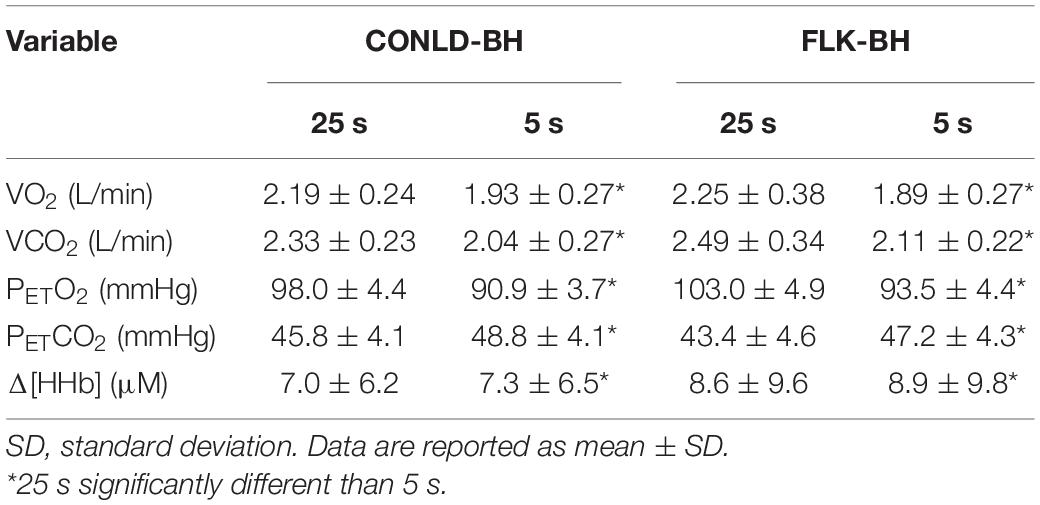
Table 4. Mean outcome measures for both BH conditions (CONLD-BH and FLK-BH) for each 30 s apneic cycle (25 s regulated breathing, 5 s apnea) from 0 to 360 s.
Δ[Hbtot], Δ[HHb], SatO2, and Δ[HHb]/VO2
NIRS-derived normalized total hemoglobin content (Δ[Hbtot]) was greater in CONLD compared to CONLD-BH (p < 0.001), FLK (p < 0.001), and FLK-BH (p < 0.001), and also in FLK compared to FLK-BH (p < 0.001) (Table 3 and Figure 3C). Deoxygenated hemoglobin normalized to baseline (Δ[HHb]) was greater in CONLD than in CONLD-BH (p = 0.011) and FLK (p < 0.001), and also in FLK-BH than in other three conditions (p < 0.001 for all comparisons) from the onset to the cessation of exercise (Table 3 and Figure 3A). SatO2 was greater in CONLD compared to CONLD-BH (p < 0.001), FLK (p < 0.001), and FLK-BH (p < 0.001), and it was not significantly different in FLK compared to FLK-BH (p = 0.434) (Table 3 and Figure 3D). The ratio of Δ[HHb]/VO2 was similar between CONLD and CONLD-BH (p = 0.086), but lower in FLK than FLK-BH (p < 0.001) (Table 3 and Figure 3B).
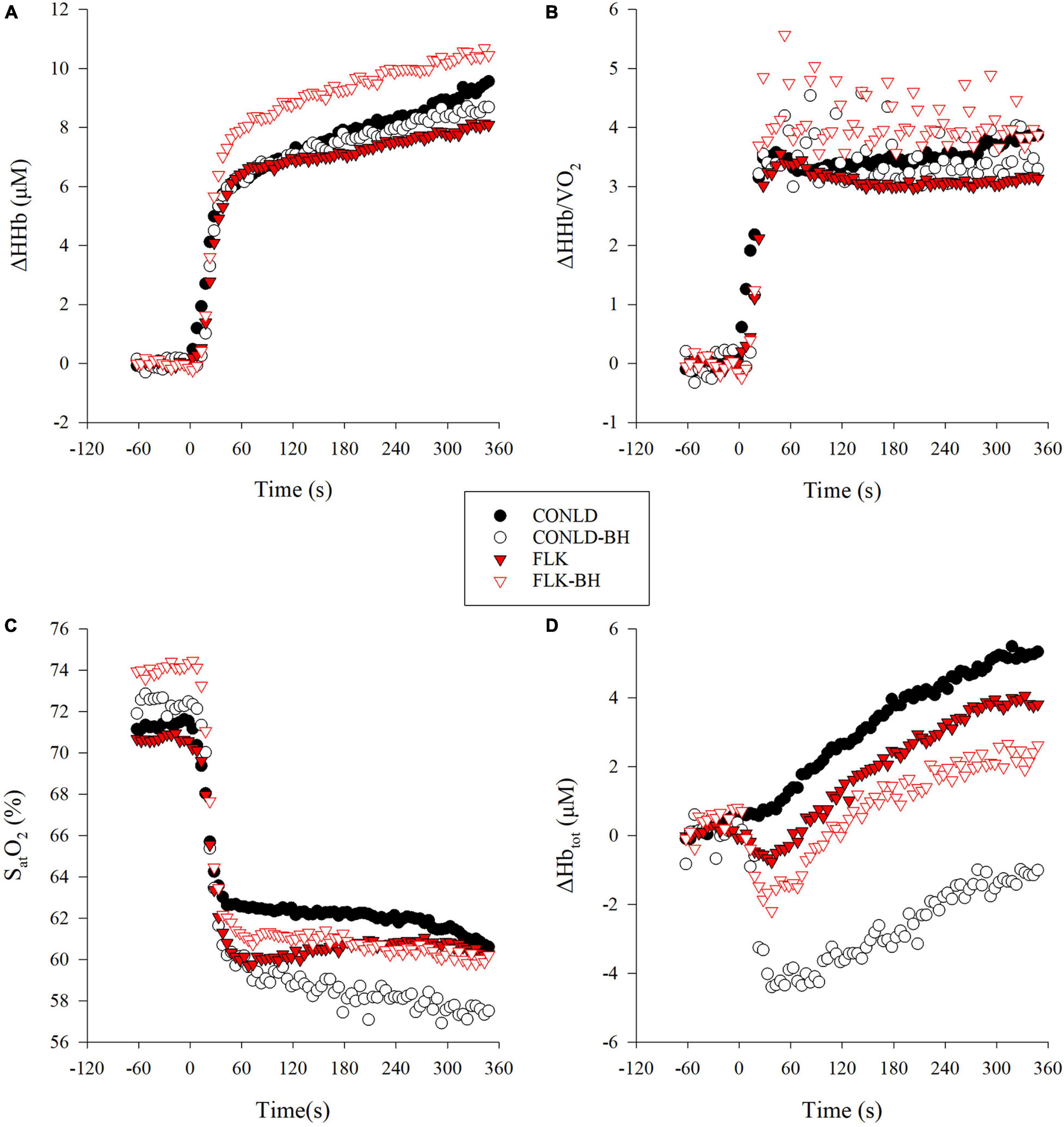
Figure 3. Local muscle deoxygenation variables from near infrared spectrometry (NIRS) data in CONLD (filled circles), CONLD-BH (open circles), FLK (filled red triangles), and FLK-BH (open red triangles). (A) Deoxyhemoglobin concentration normalized to baseline (Δ[HHb]), (B) ratio of normalized deoxyhemoglobin to VO2 (Δ[HHb]/VO2), (C) oxygen saturation of hemoglobin SatO2, and (D) total hemoglobin concentration normalized to baseline (Δ[Hbtot]). Time 0 marks the start of the exercise transition. Data were interpolated from breath-by-breath to second-by-second and 5 s averaged for graphical representation.
Lactate ([La–])
Post-exercise arterialized capillary lactate concentration ([La–]) was unchanged in CONLD compared to CONLD-BH (p = 1.000), and both were lower compared to FLK and FLK-BH (p < 0.001 and p = 0.007, respectively) (Table 3 and Figure 4). Post-exercise [La–] was similar between FLK and FLK-BH (p = 1.000) (Table 3 and Figure 4).
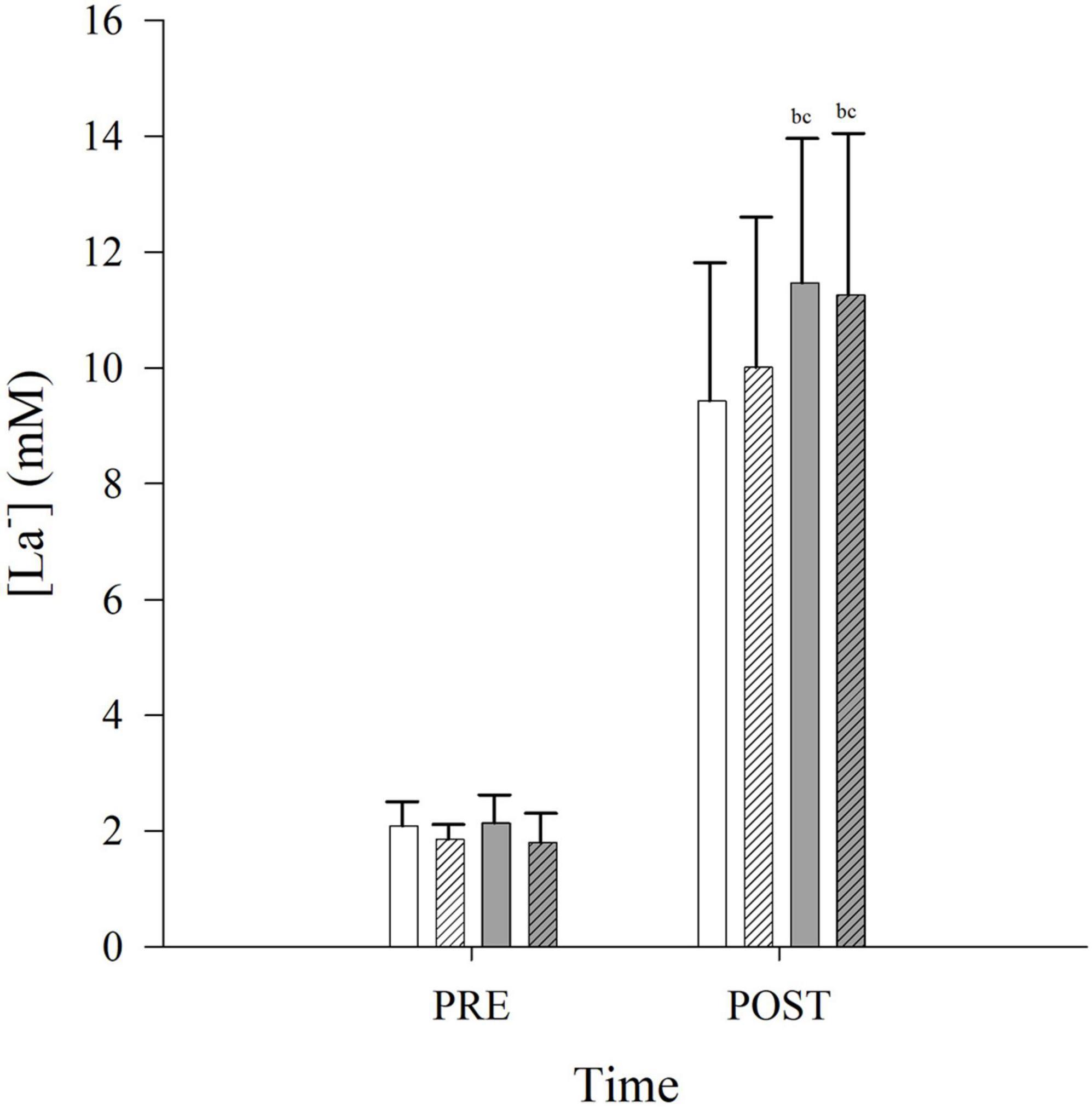
Figure 4. Arterialized capillary lactate concentrations ([La–]) PRE and POST exercise in CONLD (white), CONLD-BH (white with diagonal lines), FLK (gray) and FLK-BH (gray with diagonal lines). All four POST [La–] were greater than the PRE [La–]. aSignificantly greater than CONLD. bSignificantly greater than POST CONLD. cSignificantly greater than POST CONLD-BH.
Discussion
The goal of this study was to monitor the physiological adjustments to repeated cycles of 5 s BHs followed by 25 s of regulated breathing during CONLD and FLK exercise that lasted 6 min. Main findings included (i) unchanged VO2 between CONLD and CONLD-BH, and in FLK and FLK-BH, despite the imposed regulatory breathing paradigm; (ii) Δ[Hbtot] and SatO2 were lower, and a marginal decrease in Δ[HHb] was observed in CONLD-BH compared to CONLD, whereas Δ[HHb] and SatO2 were greater in FLK-BH compared to FLK.
CONLD and CONLD-BH
The changes in PO in CONLD and CONLD-BH, relative to the previous related research (Δ50%-Δ20%) (Lim et al., 2018), reflect the additions of the regulated breathing to this 25 s breathing/5 s apnea protocol. The purpose of this modification was to simulate the difference in breathing patterns between swimming backstroke (25 s free-breathing) and front crawl (25 s regulated breathing). By introducing these restrictions, participants were forced to perform a much lower intensity (189 W, Δ20%) compared to that suggested by Lim et al. (2018) (218 W, Δ50%, p = 0.027), despite having similar aerobic fitness (VO2max: 3.23 and 3.17 L/min, p = 0.82, respectively), LTs (VO2 at LT: 1.72 and 1.77 L/min, p = 0.57, respectively; PO at LT: 155 and 156 W, respectively), and PPO (303 and 314 W, p = 0.58, respectively) to perform the 6 min trials. Moreover, the reduced PO of this study elicited lower mean VE (68 L/min) compared to that suggested by Lim et al. (99 L/min) (Lim et al., 2018). Within the context of this PO adjustment of this study, VO2 was unchanged with the addition of the 5 s apneic periods and matching of fB and VTI during the 25 s breathing periods (Table 2) of CONLD-BH, demonstrating that any potential increase in O2 cost derived from the periodic apneas had been met. The data in this study show that the aerobic metabolic demand (i.e., VO2) was supported by the decreased SatO2 (Table 3 and Figure 3C) and increased muscle deoxygenation under the reduced perfusion conditions, as reflected by the much greater decrease in mean Δ[Hbtot] (Table 3 and Figure 3D) and modest decrease in Δ[HHb] (Table 3 and Figure 3A). A similar reduction in SatO2 and an increase in muscle deoxygenation were observed by Kume et al. (2016) at a PO corresponding to 65% of VO2max interspersed with 4 s exhalations followed by a maximal inhalation, simulating hypoventilation. Furthermore, previous work by Hoffmann et al. (2005) under apneic and rebreathing exercise conditions during higher intensity exercise, which generated similar hypoxia (decreased PETO2 in rebreathing), demonstrated greater mean arterial pressure and lower heart rate in the apneic compared to the rebreathing condition, demonstrating that only the apneic state elicited the observed O2 conservation or diving response that is associated with the decreased intramuscular blood flow. They concluded that the mechanical cessation of breathing was the key stimulus to this drop in perfusion. This has been corroborated by similar protocols comparing rebreathing with apnea (Lindholm et al., 1999). The data of this study confirm a similar response under the combination of the regulated breathing and BH paradigm. Moreover, the observed decrease in Δ[Hbtot] shown in this study was not observed during hypoxic exercise (∼12% fraction of oxygen in inspired air [FIO2]) performed at low-moderate (single-leg knee extensions) (DeLorey et al., 2004) and supra-LT intensities (Ainslie et al., 2007), suggesting again that the reduced blood flow in this study was apnea-related. Furthermore, earlier research by Lim et al. (2018), within a comparable protocol as this study, and Kume et al. (2013), performing intermittent apneas during continuous exercise at a comparable intensity, identified similar temporal reductions in Δ[Hbtot] resolved by similar increases in muscle deoxygenation. This decrease was observed despite previous suggestions that Δ[Hbtot] is increased during deep diving via splenic contractions (Hurford et al., 1990).
The drop in PETO2 (Table 4 and Figure 2C) and increased VO2 (Table 4 and Figure 2A) observed immediately post-apnea in this study reflect continued alveolar to capillary O2 diffusion facilitating the unchanged VO2. This response has also been observed under 20 s apneas (Lindholm and Linnarsson, 2002) and also reflected the actual arterial O2 (PaO2) under these transient apneic conditions (Suskind et al., 1950).
The maintenance of mean VO2 between CONLD and CONLD-BH after the exercise on-transient response was supported not only by the microvasculature hemodynamic changes outlined earlier, but also by the increased VO2 during the 25 s of regulated breathing (Table 4 and Figure 2A) and the reduced PETO2 immediately post-BH (Table 4 and Figure 2C) suggested earlier (Yamamoto et al., 1987). Specifically, Woorons et al. (2007) found that intermittent apneas performed at a high pulmonary volume (i.e., near total lung capacity) during higher intensity exercise (∼70% VO2max), similar to this study, maintained pulmonary arterial SatO2.
The unchanged mean VCO2, between CONLD and CONLD-BH, suggests that the H+ buffering, associated with the lactate production of anaerobic glycolysis, has been reflected in the observed increase in PETCO2 and that the BH did not impose a greater anaerobic glycolytic contribution. The unchanged post-exercise [La–] between CONLD and CONLD-BH trials is in contrast to other studies that have reported increased [La–] accumulation during incremental (Seo et al., 2020) (50 W + 12.5 W/min to exhaustion) and intermittent (Lim et al., 2018) exercise under hypoxic or apneic condition, respectively. However, these studies were performed at much higher supra-LT PO, which would elicit much greater blood [La–].
From a swimming front crawl perspective, at this Δ20% PO intensity, the addition of the regulated breathing paradigm and the 5 s BH, while maintaining a similar intensity of kicking, may be performed without negative physiological consequences. Notably, this is within the context of the legs only paradigm of this experiment as opposed to the simultaneous arm and leg action that is performed after the underwater portion of the swim.
FLK and FLK-BH
The FLK condition mimics the strategies of those swimmers who believe that sprint kicking during the underwater portion after the turn, along with their own superior hydrodynamics compared to their opponents, will give them a competitive advantage. In contrast to the study by Lim et al. (2018), utilizing a similar protocol, albeit at much higher PO, our results showed that implementing the intermittent 5 s periods of apnea to FLK (FLK-BH) did not affect mean VO2. Similar to CONLD-BH and CONLD, fB and VTI were also regulated in FLK-BH to FLK; however, although VTI was statistically greater in FLK-BH (Table 2), the unchanged VE suggested that this ∼80 mL/min change in VTI (Δ1.6% over the 6 min) had no physiological effects. It was notable that VTI was lower in FLK compared to CONLD (2.86 ± 0.30 L and 2.93 ± 0.21 L, respectively). However, coupled with the increased fB (25.2 ± 5.2 breaths/min and 22.0 ± 4.7 breaths/min), a higher VE, in conjunction with the higher VCO2 in FLK vs. CONLD, was observed (Table 2). The lowered PETO2 immediately post-apnea (Table 4 and Figure 2A) suggests that the observed pulmonary alveoli to capillary diffusion continued during the 5 s facilitating O2 transport. Moreover, the increased Δ[HHb] and Δ[HHb]/VO2 in FLK-BH compared to FLK, which reflected a greater reliance on muscle deoxygenation, at the active muscle was responsible for this result (duManoir et al., 2010; Murias et al., 2011).
In elite swimmers, similar maintenance of VO2 has been demonstrated during 4 min of submaximal intensity swimming with apnea induced by regulated breathing conditions of every two arm strokes up to a maximum of five-arm strokes, notwithstanding the reduced VE in the latter condition (Dicker et al., 1980). The consequences of the imposed regulated breathing and comparable intensity in the current study were apparently resolved by the greater alveolar to pulmonary capillary O2 diffusion. The muscle deoxygenation response was not recorded in the earlier work by Dicker et al. (1980). However, a similar increase in Δ[HHb] was observed by Billaut and Buchheit (2013) during 10 repeated 10 s maximal sprints followed by 30 s rest, under hypoxic conditions (13% FIO2) compared to normoxia. Conversely, others have studied the effects of repeated 3 s loadless cycling recovery periods, as opposed to the 5 s of high PO as in this study, and observed an improved microvascular O2 delivery reflected by decreased Δ[HHb]/VO2 (Belfry et al., 2012). It is suggested that the contrasting results of this study were attributed to the apneic O2 conservation effect (Kume et al., 2013), as reflected by the decreased Δ[Hbtot] in FLK-BH compared to FLK. This result is similar to the CONLD-BH compared to CONLD, of this study and others, reflecting a similar redistribution of blood flow away from working muscles during apnea (Kume et al., 2013; Lim et al., 2018). Furthermore, it is suggested that the unchanged mean VCO2 and [La–] during FLK-BH compared to FLK despite the apneic periods was a consequence of the continued buffering and pulmonary capillary to alveolar diffusion and buffering during this 5 s BH (Table 4). Conversely, Lim et al. (2018), under a similar apnea protocol (5 s), but with free-breathing (25 s), as opposed to regulated breathing of this study, showed lower mean VCO2 in FLK-BH compared to FLK and higher lactates associated with the higher mean PO (Δ50% vs. Δ20%), suggesting a relative decrease in ventilatory buffering at this much higher relative PO.
To our knowledge, this was the first study that regulated breathing during supra-threshold PO exercise on a cycle ergometer following periods of apnea, to a free-breathing protocol at the same PO similar to the lower extremity work associated with swimming in the prone position. Consequently, mean VO2 was maintained in these apneic conditions (CONLD-BH and FLK-BH) compared to their free-breathing counterparts (CONLD and FLK, respectively) by greater muscle deoxygenation, despite decreased intramuscular blood perfusion. The regulated fB and VE during the 25 s breathing periods of this study eliminated the transient increases in VE that previously corresponded with greater post-apneic VO2 and VCO2 (Lim et al., 2018). VO2 was sustained in CONLD-BH compared to CONLD, and in FLK-BH compared to FLK, as a function of the continued pulmonary diffusion during apnea, as is reflected by the immediate increases in PETO2 post-apnea (Table 4). At the level of the muscle, the preserved arterial O2 content along with the increased muscle deoxygenation facilitated the unchanged VO2. Although these protocols were designed to replicate the breathing opportunities afforded during regulated compared to free-breathing conditions, the cardiorespiratory responses to facial immersion supine exercise, and the combined arm and leg muscle action specific to swimming (Guyatt et al., 1965; Christie et al., 1990; Leahy et al., 2019) were not studied, due to the inherent difficulties of calculating gas exchange while submerged, and the unavailability of a recumbent ergometer that would interface successfully with our data collection equipment. Additional research is needed to clarify the role of these specific swimming characteristics in these physiological outcomes. Furthermore, our results may not reflect the responses of well-trained competitive swimmer, and as such, future studies should compare the physiological resolution of expert swimmers, compared to non-swimmers, to these breathing restrictions. Finally, only healthy participants were tested; therefore, no comparisons to diseased populations should be made.
Moreover, only male participants were included in this study to best match participant characteristics to the previous study (Lim et al., 2018). Therefore, these observations may not apply to females because of sex differences in body composition, fluctuations in reproductive hormones (estrogen and progesterone) (Arora et al., 1998), and lactate production during exercise (Jurkowski et al., 1981). Also, the sample size (n = 10) of this study adequately powered the statistical analyses used; a larger sample size may be required to generalize these results.
Conclusion
The initial and necessary reduction of PO (Δ50%–Δ20%) imposed by the regulated breathing condition demonstrated the severe cardiorespiratory consequences of this regulated breathing protocol compared to the free-breathing paradigm instituted previously by our lab. However, under this reduced PO, mean VO2 was maintained after the implementation of 5 s apneic periods and 25 s regulated post-BH breathing during supra-threshold exercise. The mechanism for this sustained VO2 under the apnea condition, with its reduced breathing opportunities, was expounded through an increase in muscle deoxygenation (Δ[HHb]) relative to VO2 within the constraints of the O2 conservation or deep diving response (decreased Δ[Hbtot]). This was in contrast with the systematic increases in VE and unchanged Δ[HHb]/VO2 observed during free as opposed to regulated breathing conditions, under an otherwise identical apneic protocol compared to this study. From a practical perspective, swimmers competing in the predominantly aerobic front crawl events (400, 800, and 1,500 m) would be advised to increase their minute ventilation by increasing the frequency of breathing to twice per arm cycle as often as is comfortable, increase tidal volumes, or suffer negative performance consequences.
Data Availability Statement
The raw data supporting the conclusions of this article will be made available by the authors, without undue reservation.
Ethics Statement
The studies involving human participants were reviewed and approved by the Western University Research Ethics Board for Health Sciences Research Involving Human Participants. The patients/participants provided their written informed consent to participate in this study.
Author Contributions
GB and DL conceived, designed the study, collected, and analyzed the data. GB and KG interpreted the results and drafted the manuscript. GB and JM edited the manuscript. All authors contributed to the article and approved the submitted version.
Funding
Funding for this project was provided by the Natural Sciences and Engineering Research Council (NSERC) grant.
Conflict of Interest
The authors declare that the research was conducted in the absence of any commercial or financial relationships that could be construed as a potential conflict of interest.
Publisher’s Note
All claims expressed in this article are solely those of the authors and do not necessarily represent those of their affiliated organizations, or those of the publisher, the editors and the reviewers. Any product that may be evaluated in this article, or claim that may be made by its manufacturer, is not guaranteed or endorsed by the publisher.
References
Ainslie, P. N., Barach, A., Murrell, C., Hamlin, M., Hellemans, J., and Ogoh, S. (2007). Alterations in cerebral autoregulation and cerebral blood flow velocity during acute hypoxia: rest and exercise. Am. J. Physiol. Heart Circul. Physiol. 292, H976–H983. doi: 10.1152/ajpheart.00639.2006
Andersson, J. P. A., Linér, M. H., Fredsted, A., and Schagatay, E. K. A. (2004). Cardiovascular and respiratory responses to apneas with and without face immersion in exercising humans. J. Appl. Physiol. 96, 1005–1010. doi: 10.1152/japplphysiol.01057.2002
Arora, S., Veves, A., Caballaro, A. E., Smakowski, P., and LoGerfo, F. W. (1998). Estrogen improves endothelial function. J. Vasc. Surg. 27, 1141–1147. doi: 10.1016/S0741-5214(98)70016-3
Babcock, M. A., Paterson, D. H., Cunningham, D. A., and Dickinson, J. R. (1994). Exercise on-transient gas exchange kinetics are slowed as a function of age. Med. Sci. Sports Exer. 26, 440–446.
Beaver, W. L., Wasserman, K., and Whipp, B. J. (1986). A new method for detecting anaerobic threshold by gas exchange. J. Appl. Physiol. 60, 2020–2027. doi: 10.1152/jappl.1986.60.6.2020
Belfry, G. R., Paterson, D. H., Murias, J. M., and Thomas, S. G. (2012). The effects of short recovery duration on VO2 and muscle deoxygenation during intermittent exercise. Eur. J. Appl. Physiol. 112, 1907–1915. doi: 10.1007/s00421-011-2152-4
Billaut, F., and Buchheit, M. (2013). Repeated-sprint performance and vastus lateralis oxygenation: effect of limited O2 availability. Scand. J. Med. Sci. Sports 23, e185–93. doi: 10.1111/sms.12052
Chacaroun, S., Vega-Escamilla y Gonzalez, I., Flore, P., Doutreleau, S., and Verges, S. (2019). Physiological responses to hypoxic constant-load and high-intensity interval exercise sessions in healthy subjects. Eur. J. Appl. Physiol. 119, 123–134. doi: 10.1007/s00421-018-4006-9
Christie, J. L., Sheldahl, L. M., Tristani, F. E., Wann, L. S., Sagar, K. B., Levandoski, S. G., et al. (1990). Cardiovascular regulation during head-out water immersion exercise. J. Appl. Physiol. 69, 657–664. doi: 10.1152/jappl.1990.69.2.657
DeLorey, D. S., Shaw, C. N., Shoemaker, J. K., Kowalchuk, J. M., and Paterson, D. H. (2004). The effect of hypoxia on pulmonary O2 uptake, leg blood flow and muscle deoxygenation during single-leg knee-extension exercise. Exp. Physiol. 89, 293–302. doi: 10.1113/expphysiol.2003.026864
Dicker, S. G., Lofthus, G. K., Thornton, N. W., and Brooks, G. A. (1980). Respiratory and heart rate responses to tethered controlled frequency breathing swimming. Med. Sci. Sports Exerc. 12, 20–23.
duManoir, G. R., DeLorey, D. S., Kowalchuk, J. M., and Paterson, D. H. (2010). Kinetics of VO2 limb blood flow and regional muscle deoxygenation in young adults during moderate intensity, knee-extension exercise. Eur. J. Appl. Physiol. 108, 607–617. doi: 10.1007/s00421-009-1263-7
Ferrigno, M., Ferretti, G., Ellis, A., Warkander, D., Costa, M., Cerretelli, P., et al. (1997). Cardiovascular changes during deep breath-hold dives in a pressure chamber. J. Appl. Physiol. 83, 1282–1290. doi: 10.1152/jappl.1997.83.4.1282
Guyatt, A. R., Newman, F., Cinkotai, F. F., Palmer, J. I., and Thomson, M. L. (1965). Pulmonary diffusing capacity in man during immersion in water. J. Appl. Physiol. 20, 878–881. doi: 10.1152/jappl.1965.20.5.878
Hoffmann, U., Smerecnik, M., Leyk, D., and Essfeld, D. (2005). Cardiovascular responses to apnea during dynamic exercise. Int. J. Sports Med. 26, 426–431.
Hurford, W. E., Hong, S. K., Park, Y. S., Ahn, D. W., Shiraki, K., Mohri, M., et al. (1990). Splenic contraction during breath-hold diving in the Korean ama. J. Appl. Physiol. 69, 932–936. doi: 10.1152/jappl.1990.69.3.932
Inglis, E. C., Iannetta, D., and Murias, J. M. (2017). The plateau in the NIRS-derived [HHb] signal near the end of a ramp incremental test does not indicate the upper limit of O2 extraction in the vastus lateralis. Am. J. Physiol. Regul. Integr. Compar. Physiol. 313, R723–R729. doi: 10.1152/ajpregu.00261.2017
Jurkowski, J., Jones, N. L., Toews, C. J., and Sutton, J. R. (1981). Effects of menstrual cycle on blood lactate, O2 delivery, and performance during exercise. J. Appl. Physiol. 51, 1493–1499.
Keir, D. A., Murias, J. M., Paterson, D. H., and Kowalchuk, J. M. (2014). Breath-by-breath pulmonary O2 uptake kinetics: effect of data processing on confidence in estimating model parameters. Exp. Physiol. 99, 1511–1522. doi: 10.1113/expphysiol.2014.080812
Kennedy, M. D., Warburton, D. E. R., Boliek, C. A., Esch, B. T. A., Scott, J. M., and Haykowsky, M. J. (2008). The oxygen delivery response to acute hypoxia during incremental knee extension exercise differs in active and trained males. Dyn. Med. 7:11. doi: 10.1186/1476-5918-7-11
Kume, D., Akahoshi, S., Song, J., Yamagata, T., Wakimoto, T., Nagao, M., et al. (2013). Intermittent breath holding during moderate bicycle exercise provokes consistent changes in muscle oxygenation and greater blood lactate response. J. Sports Med. Phys. Fitness 53, 327–335.
Kume, D., Akahoshi, S., Yamagata, T., Wakimoto, T., and Nagao, N. (2016). Does voluntary hypoventilation during exercise impact EMG activity? Springerplus 5:149. doi: 10.1186/s40064-016-1845-x
Lamarra, N., Whipp, B. J., Ward, S. A., and Wasserman, K. (1987). Effect of interbreath fluctuations on characterizing exercise gas exchange kinetics. J. Appl. Physiol. 62, 2003–2012. doi: 10.1152/jappl.1987.62.5.2003
Leahy, M. G., Summers, M. N., Peters, C. M., Molgat-Seon, Y., Geary, C. M., and Sheel, A. W. (2019). The mechanics of breathing during swimming. Med. Sci. Sports Exerc. 51, 1467–1476.
Lim, D. J., Kim, J. J., Marsh, G. D., and Belfry, G. R. (2018). Physiological resolution of periodic breath holding during heavy-intensity Fartlek exercise. Eur. J. Appl. Physiol. 118, 2627–2639. doi: 10.1007/s00421-018-3986-9
Lindholm, P., and Linnarsson, D. (2002). Pulmonary gas exchange during apnoea in exercising men. Eur. J. Appl. Physiol. 86, 487–491. doi: 10.1007/s00421-002-0581-9
Lindholm, P., Sundblad, P., and Linnarsson, D. (1999). Oxygen-conserving effects of apnea in exercising men. J. Appl. Physiol. 87, 2122–2127. doi: 10.1152/jappl.1999.87.6.2122
Murias, J. M., Spencer, M. D., DeLorey, D. S., Gurd, B. J., Kowalchuk, J. M., and Paterson, D. H. (2011). Speeding of V̇O-2 kinetics during moderate-intensity exercise subsequent to heavy-intensity exercise is associated with improved local O2 distribution. J. Appl. Physiol. 111, 1410–1415. doi: 10.1152/japplphysiol.00607.2011
Seo, J.-B., Kim, S.-W., Jung, W.-S., Park, H.-Y., and Lim, K. (2020). Effects of various hypobaric hypoxia on metabolic response, skeletal muscle oxygenation, and exercise performance in healthy males. J. Mens Health 16, 107–120. doi: 10.31083/jomh.v16i4.312
Suskind, M., Bruce, R. A., McDowell, M. E., Yu, P. N. G., Frank, W., and Lovejoy, J. (1950). Normal variations in end-tidal air and arterial blood carbon dioxide and oxygen tensions during moderate exercise. J. Appl. Physiol. 3, 282–290. doi: 10.1152/jappl.1950.3.5.282
Whipp, B. J., and Davis, J. A. (1979). Peripheral chemoreceptors and exercise hyperpnea. Med. Sci. Sports 11, 204–212.
Woorons, X., Mollard, P., Pichon, A., Duvallet, A., Richalet, J.-P., and Lamberto, C. (2007). Prolonged expiration down to residual volume leads to severe arterial hypoxemia in athletes during submaximal exercise. Respirat. Physiol. Neurobiol. 158, 75–82. doi: 10.1016/j.resp.2007.02.017
Keywords: apnea, regulated breathing, gas exchange, muscle deoxygenation, swimming, front crawl
Citation: Grossman KJ, Lim DJ, Murias JM and Belfry GR (2021) The Effect of Breathing Patterns Common to Competitive Swimming on Gas Exchange and Muscle Deoxygenation During Heavy-Intensity Fartlek Exercise. Front. Physiol. 12:723951. doi: 10.3389/fphys.2021.723951
Received: 11 June 2021; Accepted: 14 October 2021;
Published: 24 November 2021.
Edited by:
Fabrice Joulia, Université de Toulon, FranceReviewed by:
Carlo Capelli, University of Verona, ItalyFrederic Lemaitre, Université de Rouen, France
Copyright © 2021 Grossman, Lim, Murias and Belfry. This is an open-access article distributed under the terms of the Creative Commons Attribution License (CC BY). The use, distribution or reproduction in other forums is permitted, provided the original author(s) and the copyright owner(s) are credited and that the original publication in this journal is cited, in accordance with accepted academic practice. No use, distribution or reproduction is permitted which does not comply with these terms.
*Correspondence: Glen R. Belfry, Z2JlbGZyeUB1d28uY2E=