- 1INCLIVA, CIBERFES, Department of Physiology, Faculty of Medicine, University of Valencia, Valencia, Spain
- 2Department of Clinical Neurophysiology, Hospital Clínico Universitario de Valencia, Valencia, Spain
- 3Department of Neurology, Hospital Clínico Universitario de Valencia, Valencia, Spain
- 4Departament of Neuroradiology, Ascires Biomedical Group, Hospital Clinico Universitario, Valencia, Spain
- 5Department of Anatomy and Human Embryology, University of Valencia, Valencia, Spain
Oxidative stress is an early occurrence in the development of Alzheimer’s disease (AD) and one of its proposed etiologic hypotheses. There is sufficient experimental evidence supporting the theory that impaired antioxidant enzymatic activity and increased formation of reactive oxygen species (ROS) take place in this disease. However, the antioxidant treatments fail to stop its advancement. Its multifactorial condition and the diverse toxicological cascades that can be initiated by ROS could possibly explain this failure. Recently, it has been suggested that cerebral small vessel disease (CSVD) contributes to the onset of AD. Oxidative stress is a central hallmark of CSVD and is depicted as an early causative factor. Moreover, data from various epidemiological and clinicopathological studies have indicated a relationship between CSVD and AD where endothelial cells are a source of oxidative stress. These cells are also closely related to oligodendrocytes, which are, in particular, sensitive to oxidation and lead to myelination being compromised. The sleep/wake cycle is another important control in the proliferation, migration, and differentiation of oligodendrocytes, and sleep loss reduces myelin thickness. Moreover, sleep plays a crucial role in resistance against CSVD, and poor sleep quality increases the silent markers of this vascular disease. Sleep disruption is another early occurrence in AD and is related to an increase in oxidative stress. In this study, the relationship between CSVD, oligodendrocyte dysfunction, and sleep disorders is discussed while focusing on oxidative stress as a common occurrence and its possible role in the onset of AD.
Introduction
Alzheimer’s disease (AD) is a multifactorial neurodegeneration with its etiology still remaining unknown. Its complexity and multiple factors, which are constantly proven to influence its development, hinder the discovery of a curative or preventive treatment. Therefore, reviewing the events that take place, especially at the onset of the disease, and raising new hypotheses regarding their links is immensely relevant. The present review is devoted to elucidating the possibility of a connection between early events related to the onset of AD, including oxidative stress, oligodendrocyte dysfunction, cerebrovascular small disease (CVSD), and sleep disorders.
Oxidative Stress Is an Early Event in AD Physiopathology
Although free radicals are traditionally considered damaging the by-products of cellular metabolism, at present, it has become known that the production of reactive oxygen species (ROS) and reactive nitrogen species (RNS) are involved in the regulation of processes that are central to most cell signaling. During physiological and pathophysiological processes, ROS and RNS act as secondary messengers which control the expression of different genes and are involved in multiple cellular events such as Ca2+ and redox homeostasis, chemotaxis, cell growth, cell cycle, cell adhesion, and apoptosis, among others (Dröge, 2002; Poljsak and Milisav, 2012). Owing to these reasons, when the production of free radicals exceeds the antioxidant cellular capacity, oxidative stress occurs (Sies, 1986), thereby leading to the possible deregulation of several cellular mechanisms.
Alzheimer’s disease is not an exception. Free radicals are involved in many pathological cascades as both beta-amyloid peptide (Aβ) and p-tau increase ROS levels. Oxidative stress is a proven process in the early onset of AD (Nunomura et al., 2001).
However, antioxidant therapy fails to treat AD. There could be several reasons for this: on the one hand, the vulnerability of neurons to oxidative stress varies between areas, and on the other hand, ROS generation is cell-specific (Bonizzi et al., 2000) and the brain is formed by many different cell types with diverse functions. In terms of the difference in vulnerability, it has been shown that there are areas, such as hippocampal CA1 and amygdala, which are specifically affected during the early onset of AD, and these areas are vulnerable to oxidative damage. The pyramidal neurons in the CA1 region undergo massive cell death under oxidative stress conditions (Wilde et al., 1997; Vornov et al., 1998; Sarnowska, 2002; Wang et al., 2005; Bearden et al., 2009; Cruz-Sánchez et al., 2010; Chang et al., 2012; Huang et al., 2012, 2013; Uysal et al., 2012). In addition, the amygdala and prefrontal cortex are more vulnerable to oxidative effects (Masood et al., 2008; Salim et al., 2010a,b, 2011a,b; Wang and Michaelis, 2010; Patki et al., 2013a,b; Solanki et al., 2015) than other cortical areas. In particular, oxidative stress triggers amygdalar hyperactivity and dendritic shrinking (Wellman, 2001; Vyas et al., 2002; Kreibich and Blendy, 2004; Brown et al., 2005; Radley et al., 2006; Wood et al., 2010) and may further potentiate synaptic disturbances by disrupting the hippocampus–amygdala projections. Interestingly, this vulnerability of the hippocampal CA1 and amygdala to oxidative stress could offer an appealing explanation for the very early damage caused to these areas due to AD.
Oxidative Stress and CVSD in AD
Cerebrovascular small disease is a disorder that attacks the small cerebral arteries and microvessels. Its pathological presentation includes white matter hyperintensities, cerebral microbleeds, small subcortical infarct, enlarged perivascular space, brain atrophy, and lacunes visible through magnetic resonance imaging (Wardlaw et al., 2013). Vascular dysfunction is integral to the AD etiology and pathophysiology, and it includes blood–brain barrier (BBB) impairment and hemodynamic dysfunction (Klohs, 2020; Parodi-Rullán et al., 2020). CSVD also has a predictive effect on AD risk among the elderly people, and there are several epidemiological, genetic, and clinical studies related to both pathologies (Kim et al., 2020; Saridin et al., 2020). However, whether CSVD is a cause or consequence of AD pathology still remains a controversial issue. Recently, the evidence of imaging techniques suggests that intra-brain vascular dysregulation is an early pathological event during the disease development (Iturria-Medina et al., 2016). White matter hyperintensities predict an accelerated cognitive decline, increase in total cerebrospinal fluid (CSF) tau, hippocampal atrophy, and increased risk for AD (Kuller et al., 2003; Hertze et al., 2013; Bilello et al., 2015; Fiford et al., 2017; Salvadó et al., 2019). Moreover, in preclinical AD, white matter hyperintensity is observed before Aβ uptake and predicts its increase (Grimmer et al., 2012; Kandel et al., 2016). Furthermore, apolipoprotein E4 (ApoE4) carriers (that have a noticeable genetic risk factor to develop AD) have increased cerebrovascular amyloid angiopathy (Yu et al., 2015) that is caused by Aβ deposition in the vessel walls.
At present, the etiology of CVSD is unclear despite oxidative stress being the most widely supported theory which could explain the vascular dysfunction related to AD. The source of these ROS may increase the Aβ levels formed in the vessel walls or cause the dysfunction of endothelial cells (ECs). Endothelium cells contain a high quantity of mitochondria that produce high ROS levels in CVSD. These show upregulated xanthine oxidase, lipoxygenase, myeloperoxidase, and NADPH oxidases (NOX) enzymes (Doughan et al., 2008; Nazarewicz et al., 2013; Dikalov et al., 2014; Schulz et al., 2014; Sahoo et al., 2016), which also increase ROS production (Cervantes Gracia et al., 2017). The increase in ROS could culminate with an increased vessel permeability, BBB disruption (Kuriakose et al., 2019; He et al., 2020), and blood flow alteration (Freeman and Keller, 2012). Interestingly, these pathological processes have been demonstrated in AD (Klohs, 2020; Parodi-Rullán et al., 2020). This could indicate oxidative stress as the possible link between the early onset of CVSD and AD.
In recent years, it has been revealed that BBB disruption is an early marker of cognitive impairment (Montagne et al., 2015; Van De Haar et al., 2016a,b; Sweeney et al., 2018, 2019; Nation et al., 2019). The permeability of BBB increases during the very early phases of AD, and it also displays a leakage and breakdown. Besides, ApoE4 carriers show degeneration of brain pericytes, essential cells for BBB integrity (Zipser et al., 2007; Armulik et al., 2010; Bell et al., 2012; Halliday et al., 2016; Nikolakopoulou et al., 2019), and an accelerated BBB breakdown in both the hippocampus and medial temporal lobe (Montagne et al., 2020). The BBB breakdown contributes to cognitive decline in ApoE4 carriers in a manner that is independent of Aβ and tau levels. Oxidative stress could be a strong candidate for understanding early BBB disruption through cyclophilin A–matrix metalloproteinase-9 (CypA–MMP9) pathway activation (Jin et al., 2000; Gu et al., 2011). In fact, CypA-MMP9 is activated in degenerating brain capillary pericytes in ApoE4 carriers as revealed in brain tissue analysis (Halliday et al., 2016).
Oxidative Stress and Oligodendrocytes in AD
Oligodendrocytes are the myelin-forming cells in the CNS; myelin is the high lipid content sheath that surrounds and electrically isolates axons. Oligodendrocytes are derived from oligodendrocyte precursor cells (OPCs) that are produced in development and throughout adult life (Dawson et al., 2003; Richardson et al., 2011). Oligodendrocytes, especially OPCs, are highly vulnerable to oxidative damage compared with other brain cells as, after an oxidative insult, newly differentiated oligodendrocytes are damaged earlier than their counterparts (Giacci and Fitzgerald, 2018). This vulnerability could be due to a poor protective mechanism against oxidative stress in these cells. Oligodendrocytes present a lower level of antioxidant defense (Thorburne and Juurlink, 1996) and a reduced DNA repair capacity based on the non-homologous end-joining (NHEJ), which is error-prone (Spaas et al., 2021). Moreover, oxidative stress disrupts oligodendrocyte differentiation by persistent histone acetylation (French et al., 2009) and by affecting the expression of mitochondrial key genes, such as NRF2 and PPAR-γ (De Nuccio et al., 2020). Following this, under oxidative damage conditions, myelin renewal is compromised (Giacci and Fitzgerald, 2018) and may also contribute to the demyelinating phenotype observed during early AD (Sachdev et al., 2013). Demyelination due to oxidative damage has been broadly demonstrated in neurodegenerative disorders such as multiple sclerosis (Lassmann and van Horssen, 2016). However, experiments with Aβ peptide in vitro are controversial. It has been reported that Aβ causes demyelination (Horiuchi et al., 2012) in primary cultures incubated with 1 mM Aβ, and other studies report that Aβ causes remyelination with a similar concentration (Quintela-López et al., 2019). Nevertheless, in these studies, oxidative stress, which could be very important to enlighten these concepts, has not been measured.
Oligodendrocytes are also very sensitive to excitotoxicity given their high contents of AMPA and NMDA receptors which, by altering mitochondrial Ca2+ levels, makes them more vulnerable to oxidative stress than neurons (Ibarretxe et al., 2006). In the early phases of AD, both excitotoxicity and oxidative stress have been observed (Campbell and Tobler, 1984; Olney et al., 1997; Cutler et al., 2004), and their additive effect could be deleterious for oligodendrocytes.
Oxidative Stress and Sleep Disruption in AD
Right from invertebrates to superior vertebrates, all animals sleep. This indicates that it is a conserved process in evolution (Cirelli and Tononi, 2008; Joiner, 2016). Humans sleep nearly one-third of their lives and, in this process, several special circumstances occur for both vessels and oligodendrocytes as well as for the expression of antioxidant enzymes (discussed later). Proper sleep is essential for crucial brain functions such as memory consolidation and detoxification of waste products (Lloret et al., 2020). Therefore, it is not surprising that lack of sleep can have detrimental effects on brain homeostasis. One of the consequences of sleep deprivation is an increase in brain oxidative stress, either through the increase in the generation of free radicals or through the decrease in antioxidant levels (Everson et al., 2005; Villafuerte et al., 2015), especially in the hippocampus. However, some studies do not show any increase in oxidative stress following sleep deprivation in animal models; however, they all undergo acute sleep deprivation (D’Almeida et al., 1997; Cirelli et al., 1999; Gopalakrishnan et al., 2004). Nevertheless, studies in chronic sleep restriction with Drosophila mutants suggest that one function of sleep is to increase the resistance of organism to oxidative stress (Hill et al., 2018). Other studies, also on Drosophila, have revealed that an increase in oxidative damage causes breakdown of sleep, which implies that both phenomena are linked in a bidirectional manner: oxidative stress causes fragmented sleep and fragmented sleep increases oxidative stress (Koh et al., 2006).
Oxidative stress and sleep disorders have also been related in humans. Obstructive sleep apnea syndrome causes oxidative stress coinciding with each episode of apnea, measured with continuous monitoring during sleep (Lloret et al., 2007). In postmenopausal women complaining of insomnia, lipid peroxidation is increased (Hachul De Campos et al., 2006). Moreover, it has been recently reported that night workers have higher levels of oxidative stress damage and lower levels of antioxidant defenses than the day workers (Teixeira et al., 2019).
Patients with Alzheimer’s disease show a high prevalence and severity of sleep disturbances that seem to appear several years prior to the onset of cognitive decline (Lloret et al., 2020). Furthermore, ApoE4 carriers undergo a 2-fold increase in the odds of sleep-disordered breathing (Kadotani et al., 2001). These also cause poor sleep quality (Drogos et al., 2016). In contrast, better sleep consolidation seems to attenuate incidence of AD linked to APOE ε4 impact, and there is no association with age compared with APOE ε4 non-carriers (Lim et al., 2013b; Pan et al., 2021). A multicenter study showed that midlife and late-life insomnia are associated with a higher risk of late-life dementia (Sindi et al., 2018). However, the detrimental effect of sleep deprivation could also be taking place over a short term as healthy people suffering from poor sleep quality present cognitive impairment only after 1 year (Potvin et al., 2012). Moreover, high sleep fragmentation increases the risk of developing AD by 1.5 times after 6 years (Lim et al., 2013a). Following this line, a 40-year follow-up study including 1,574 men reported that sleep disturbance increases the risk of developing AD by 51% (Benedict et al., 2015). Furthermore, it has been suggested that the treatment of obstructive sleep apnea is capable of reducing the risk of dementia (Dunietz et al., 2021). This idea is supported by a recent meta-analysis reported by Bubu et al. (2017). For the explanation provided here, we can conclude that early oxidative stress in AD could provoke sleep disturbance, as well as sleep disorders, which could consequently lead to an increment in oxidative damage.
Sleep and Oligodendrocytes
Sleep is crucial in the synthesis and maintenance of the myelin due to an expression cycle of genes during the sleep/wake period (Cirelli et al., 2004; Thompson et al., 2010). Oligodendrocytes and their precursors show a different pattern of sleep-related expression of genes (Bellesi et al., 2013). Indeed, genes involved in phospholipid synthesis and myelination promoting OPC proliferation are preferentially transcribed during sleep, whereas genes implicated in apoptosis, cellular stress response (including many antioxidant enzymes), and OPC differentiation are enriched during wakefulness. Specifically, OPC proliferation doubles during sleep, correlating with the REM phase, whereas an OPC differentiation is higher during the waking state (Bellesi et al., 2013). Moreover, there is a reduction in myelin thickness following sleep loss with no changes in the internodal length (Bellesi et al., 2018). In conclusion, oligodendrocytes may express a particular vulnerability to sleep loss owing to their high susceptibility to incur oxidative stress. AD patients present high levels of sleep disruption very early, it is expected that oligodendrocytes are affected for this reason.
Oligodendrocytes and CSVD
The function of oligodendrocytes depends on proper blood perfusion, as hypoperfusion interferes with white matter repair by disrupting OPC renewal mechanisms (Miyamoto et al., 2013). Accordingly, in mice expressing human ApoE4, a decrease in the white matter levels is accompanied by microvasculature injury (Koizumi et al., 2018). ECs physiologically secrete factors that promote OPC proliferation. Furthermore, in low-oxygen environments, ECs release various factors into extracellular vesicles to increase OPC survival (Kurachi et al., 2016). Other studies have identified that this relationship is bidirectional as OPC also supports ECs, releasing signaling factors involved in BBB maintenance from OPCs to ECs (Seo et al., 2014). In order to migrate, OPCs use vessels as scaffolding (Snapyan et al., 2009), interacting specifically with pericytes (Maki et al., 2015). In this context, a decrease in the number of pericytes in the brains of patients with AD has been demonstrated (Schultz et al., 2018). Therefore, both cells, endothelial and oligodendrocytes, are very sensitive to oxidative damage and are equally related to a crossing-pathway dysfunction in AD. Hence, oxidative stress is the chief participant in the relationship between oligodendrocyte dysfunction, subsequent demyelination, and CVSD due to its early occurrence in AD.
Sleep and CVSD
Sleep and brain vascular functionality, specifically BBB function, are closely related. In fact, night sleep regulates substance transport all across and along the BBB. Sleep loss increases the BBB permeability, and sleep disruption can also lead to BBB breakdown (Hurtado-Alvarado et al., 2017).
It has been demonstrated that CSVD is associated with sleep disorders. Fifty-four percent of patients with CSVD suffer from chronic insomnia (Wang et al., 2019). Moderate-to-severe obstructive sleep apnea is also associated with CSVD (Song et al., 2017), and non-breathing-related sleep fragmentation is also common and related to the pathological markers in patients with CSVD (Wang J. et al., 2020).
One of the primary functions of sleep is to eliminate waste products from the brain. The term “glymphatic system” refers to the manner in which waste products coming from the interstitial fluid enter the brain parenchyma along with arterial perivascular spaces and exit through venous perivascular spaces (Iliff et al., 2012; Xie et al., 2013). Recent studies in mice have revealed that Aβ concentration in interstitial fluid fluctuates during the day and night (Kress et al., 2018). Its concentration peaks during the night and the loss of components of the molecular clock increases amyloid plaques formation. Hence, it is not surprising that sleep disturbance and CSVD could be important comorbidities for AD. In AD, both sleep disturbance and BBB dysfunction have been described, and both occur early on (Semyachkina-Glushkovskaya et al., 2020). Even healthy people carrying the ApoE4 allele show alterations in both processes. Therefore, sleep disruption and dysfunction with respect to the vascular functionality of the brain are two phenomena that are closely related and implicated in the onset of AD (see Figure 1 for a schematic summary).
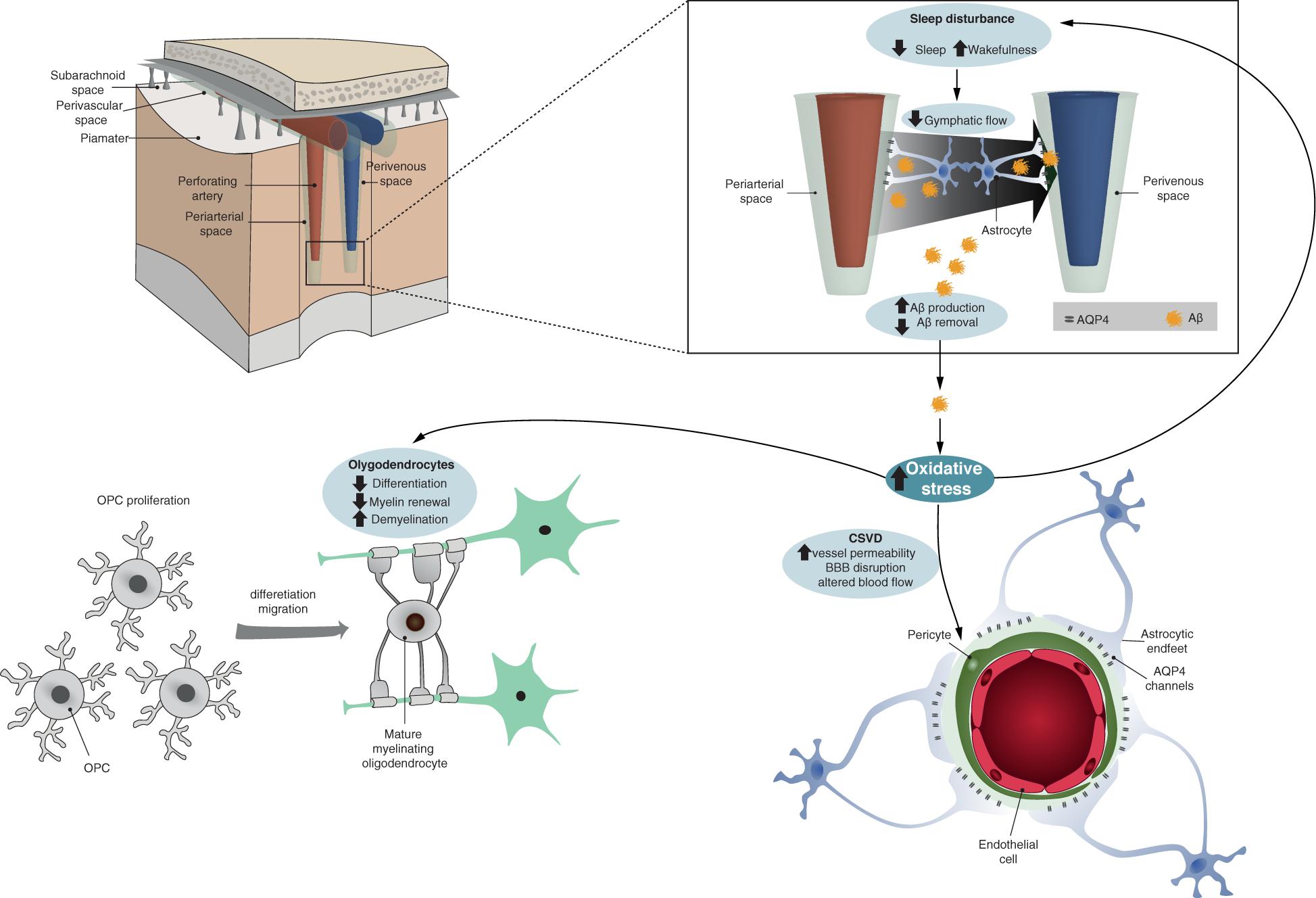
Figure 1. Schematic representation summarizing how oxidative stress could link vessel damage, sleep disruption, and oligodendrocyte dysfunction in early AD. The top panels represent brain perfusion with the glymphatic system detailed. The waste products including beta-amyloid are not properly cleared by the glymphatic system during sleep due to the disruption of latter caused by AD-related oxidative stress. Oxidative stress also increases CSVD-related alterations and oligodendrocyte/OPC unsuccessful differentiation (bottom panel).
Is Oxidative Stress the Link Between Sleep, CVSD, and Oligodendrocytes Dysfunction in AD?
In this study, we hypothesized that oxidative stress could be the molecular link between oligodendrocyte and endothelium dysfunction and sleep disruption. The primary source of ROS in cells is mitochondria, and specifically, the electronic transport chain. In AD, several evidences of mitochondrial dysfunction and oxidative stress caused by Aβ peptides have been found: energy failure prior to the development of plaque pathology, ATP formation reduction, complexes I and IV inhibition that increases ROS production, and high levels of oxidized mtDNA (Cenini et al., 2019).
Reactive oxygen species are important physiological cell-signaling molecules in the vascular endothelium. Therefore, they are normally formed in these cells. However, this fact precisely makes them more vulnerable when they are formed in excess. Cerebral ECs also have a high concentration of mitochondria, which provides an increased opportunity for generating oxidative stress. It has been shown that BBB disruption could be caused by ROS-induced metalloproteinase (MMP) activity, which stimulates the degradation of tight junctions (Dawson et al., 2003). On the contrary, it has also been mentioned that increased NOXs activity may be involved in this process. NOXs transfer electrons from NADPH to oxygen, thereby generating superoxide radical. Moreover, it is involved in BBB breakdown in CVSD and AD (Freeman and Keller, 2012).
Oligodendrocytes and endothelium closely interact with each other. This oligovascular niche is important to sustain angiogenesis and oligodendrogenesis (Arai and Lo, 2009a,b). Oligodendrocytes are highly susceptible to oxidative stress, as they are the predominant iron-containing cells of the brain (Connor and Menzies, 1995) and have reduced levels of glutathione, glutathione peroxidase, and mitochondrial manganese superoxide dismutase (Thorburne and Juurlink, 1996). In ECs, ROS-activated MMPs induce oligodendrocyte dysfunction by degrading the extracellular protein matrix, consequently impairing their differentiation (Gorter and Baron, 2020). NOX enzymes also increase in oligodendrocytes through Aβ-induced NMDA receptors/PKC pathway (Cavaliere et al., 2013; Liu et al., 2019).
Finally, as mentioned previously, ROS are generated in obstructive sleep apnea due to intermittent hypoxic episodes resulting in sleep fragmentation (Nair et al., 2011a,b). Subsequently, ROS can directly activate MMPs and also via nuclear factor kappa B (NF-κB) pathway activation (Htoo et al., 2006). In fact, plasma MMP9 levels are elevated in patients with obstructive sleep apnea (Franczak et al., 2019a; Mashaqi et al., 2021), and urinary MMP2 activity has been shown to increase in accordance with obstructive sleep apnea severity (Franczak et al., 2019b). On the contrary, it has also been described that ROS induces the synthesis and stability of hypoxia-inducible factor 1α (HIF-1α) during hypoxic periods. In turn, HIF-1α increases the activity of NOXs, which are an important prooxidant, producing superoxide and H2O2 (Takac et al., 2011; Kaur et al., 2014; Wang N. et al., 2020). These changes promote a prooxidant state, disrupt hippocampal synaptic plasticity, and impair spatial memory in patients with obstructive sleep apnea (Arias-Cavieres et al., 2020). Considering the aforementioned changes, we concluded that oxidative stress could increase MMP and NOX activities that are involved in oligodendrocyte and endothelium dysfunction and sleep disruption. Figure 2 summarizes these common molecular pathways.
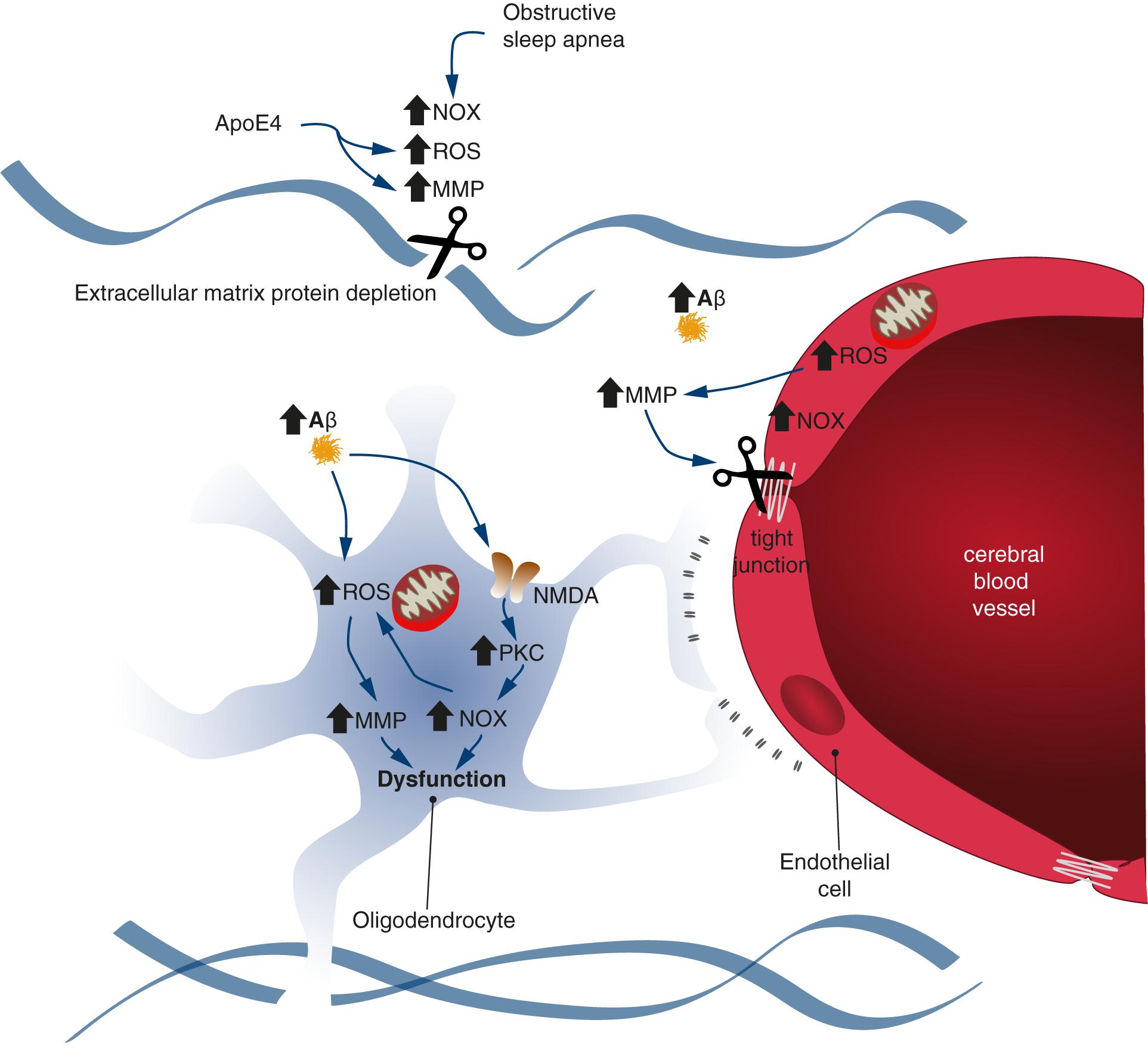
Figure 2. Schematic representation illustrating the common molecular pathways between endothelial and oligodendrocyte dysfunction and obstructive sleep apnea. Oxidative stress could increase the activity of metalloproteinases (MMPs) and NADPH oxidases (NOXs). The extracellular protein matrix and tight junctions are degraded by MMPs, which contributes to endothelial and oligodendrocyte dysfunction. The NOX activity provokes increased ROS levels in a positive feedback loop.
Concluding Remarks
Considering the arguments so far, it is apparent that oligodendrocyte dysfunction, sleep, and CSVD are closely related to each other, and all constitute oxidative stress as a very early indicator. It is noteworthy that the major lesions in AD are Aβ and tau; thus, the onset of the disease should be related to the formation of these molecules in an exacerbated form. All the present models point out that the first positive biomarker in AD is soluble Aβ that probably begins its silent cell deregulation decades before any observable cognitive impairment (Lloret et al., 2019). Here, we proposed that Aβ could generate oxidative stress early on in AD, but only selectively affecting cells, especially vulnerable ones such as oligodendrocytes and ECs. Moreover, this oxidative stress would affect myelin formation and renewal, sleep, and vessel function (including BBB function). All these processes will interact with each other and show positive feedback.
Author Contributions
AL has elaborated the items to be discussed and coordinated the work. AL and DE have written the first draft. AC-F has designed and drew the figures. JL and BL have found and selected the references. PM, AC-F, and ML have expanded and corrected the draft. All author listed have made a substantial, direct and intellectual contribution to the work, and approved it for publication.
Funding
Special Research Actions from University of Valencia, REF: UV-INV-AE-1546096.
Conflict of Interest
The authors declare that the research was conducted in the absence of any commercial or financial relationships that could be construed as a potential conflict of interest.
Publisher’s Note
All claims expressed in this article are solely those of the authors and do not necessarily represent those of their affiliated organizations, or those of the publisher, the editors and the reviewers. Any product that may be evaluated in this article, or claim that may be made by its manufacturer, is not guaranteed or endorsed by the publisher.
References
Arai, K., and Lo, E. H. (2009b). Oligovascular signaling in white matter stroke. Biol. Pharm. Bull. 32, 1639–1644. doi: 10.1248/bpb.32.1639
Arai, K., and Lo, E. H. (2009a). An oligovascular niche: cerebral endothelial cells promote the survival and proliferation of oligodendrocyte precursor cells. J. Neurosci. 29, 4351–4355. doi: 10.1523/JNEUROSCI.0035-09.2009
Arias-Cavieres, A., Khuu, M. A., Nwakudu, C. U., Barnard, J. E., Dalgin, G., and Garcia, A. J. (2020). A HIF1a-Dependent Pro-Oxidant State Disrupts Synaptic Plasticity and Impairs Spatial Memory in Response to Intermittent Hypoxia. eNeuro 7, ENEURO.0024-20.2020. doi: 10.1523/ENEURO.0024-20.2020
Armulik, A., Genové, G., Mäe, M., Nisancioglu, M. H., Wallgard, E., Niaudet, C., et al. (2010). Pericytes regulate the blood-brain barrier. Nature 468, 557–561. doi: 10.1038/nature09522
Bearden, C. E., Thompson, P. M., Avedissian, C., Klunder, A. D., Nicoletti, M., Dierschke, N., et al. (2009). Altered hippocampal morphology in unmedicated patients with major depressive illness. ASN Neuro 1, 265–273. doi: 10.1042/AN20090026
Bell, R. D., Winkler, E. A., Singh, I., Sagare, A. P., Deane, R., Wu, Z., et al. (2012). Apolipoprotein e controls cerebrovascular integrity via cyclophilin A. Nature 485, 512–516. doi: 10.1038/nature11087
Bellesi, M., Haswell, J. D., De Vivo, L., Marshall, W., Roseboom, P. H., Tononi, G., et al. (2018). Myelin modifications after chronic sleep loss in adolescent mice. Sleep 41:zsy034. doi: 10.1093/sleep/zsy034
Bellesi, M., Pfister-Genskow, M., Maret, S., Keles, S., Tononi, G., and Cirelli, C. (2013). Effects of sleep and wake on oligodendrocytes and their precursors. J. Neurosci. 33, 14288–14300. doi: 10.1523/JNEUROSCI.5102-12.2013
Benedict, C., Byberg, L., Cedernaes, J., Hogenkamp, P. S., Giedratis, V., Kilander, L., et al. (2015). Self-reported sleep disturbance is associated with Alzheimer’s disease risk in men. Alzheimers Dement. 11, 1090–1097. doi: 10.1016/j.jalz.2014.08.104
Bilello, M., Doshi, J., Nabavizadeh, S. A., Toledo, J. B., Erus, G., Xie, S. X., et al. (2015). Correlating cognitive decline with white matter lesion and brain atrophy magnetic resonance imaging measurements in Alzheimer’s disease. J. Alzheimers Dis. 48, 987–994. doi: 10.3233/JAD-150400
Bonizzi, G., Piette, J., Merville, M. P., and Bours, V. (2000). Cell type-specific role for reactive oxygen species in nuclear factor- kappaB activation by interleukin-1. Biochem. Pharmacol. 59, 7–11. doi: 10.1016/S0006-2952(99)00290-7
Brown, S. M., Henning, S., and Wellman, C. L. (2005). Mild, short-term stress alters dendritic morphology in rat medial prefrontal cortex. Cereb. Cortex 15, 1714–1722. doi: 10.1093/cercor/bhi048
Bubu, O. M., Brannick, M., Mortimer, J., Umasabor-Bubu, O., Sebastião, Y. V., Wen, Y., et al. (2017). Sleep, Cognitive impairment, and Alzheimer’s disease: a Systematic Review and Meta-Analysis. Sleep 40:zsw032. doi: 10.1093/sleep/zsw032
Campbell, S. S., and Tobler, I. (1984). Animal sleep: a review of sleep duration across phylogeny. Neurosci. Biobehav. Rev. 8, 269–300. doi: 10.1016/0149-7634(84)90054-X
Cavaliere, F., Benito-Muñoz, M., Panicker, M., and Matute, C. (2013). NMDA modulates oligodendrocyte differentiation of subventricular zone cells through PKC activation. Front. Cell Neurosci. 7:261. doi: 10.3389/fncel.2013.00261
Cenini, G., Lloret, A., and Cascella, R. (2019). Oxidative Stress in Neurodegenerative Diseases: from a Mitochondrial Point of View. Oxid. Med. Cell. Longev. 2019:2105607. doi: 10.1155/2019/2105607
Cervantes Gracia, K., Llanas-Cornejo, D., and Husi, H. (2017). CVD and Oxidative Stress. J. Clin. Med. 6:22. doi: 10.3390/jcm6020022
Chang, B. J., Jang, B. J., Son, T. G., Cho, I. H., Quan, F. S., Choe, N. H., et al. (2012). Ascorbic acid ameliorates oxidative damage induced by maternal low-level lead exposure in the hippocampus of rat pups during gestation and lactation. Food Chem. Toxicol. 50, 104–108. doi: 10.1016/j.fct.2011.09.043
Cirelli, C., Gutierrez, C. M., and Tononi, G. (2004). Extensive and Divergent Effects of Sleep and Wakefulness on Brain Gene Expression. Neuron 41, 35–43. doi: 10.1016/S0896-6273(03)00814-6
Cirelli, C., Shaw, P. J., Rechtschaffen, A., and Tononi, G. (1999). No evidence of brain cell degeneration after long-term sleep deprivation in rats. Brain Res. 840, 184–193. doi: 10.1016/S0006-8993(99)01768-0
Cirelli, C., and Tononi, G. (2008). Is sleep essential? PLoS Biol. 6:e216. doi: 10.1371/journal.pbio.0060216
Connor, J. R., and Menzies, S. L. (1995). Cellular management of iron in the brain. J. Neurol. Sci. 134, 33–44. doi: 10.1016/0022-510x(95)00206-h
Cruz-Sánchez, F. F., Gironès, X., Ortega, A., Alameda, F., and Lafuente, J. V. (2010). Oxidative stress in Alzheimer’s disease hippocampus: a topographical study. J. Neurol. Sci. 299, 163–167. doi: 10.1016/j.jns.2010.08.029
Cutler, R. G., Kelly, J., Storie, K., Pedersen, W. A., Tammara, A., and Hatanpaa, K. (2004). Invement of oxidative stress-induced abnormalities in ceramide and cholesterol metabolism in brain aging and Alzheimer’s disease. Proc. Natl. Acad. Sci. U. S. A. 101, 2070–2075. doi: 10.1073/pnas.0305799101
D’Almeida, V., Hipólide, D. C., Azzalis, L. A., Lobo, L. L., Junqueira, V. B. C., and Tufik, S. (1997). Absence of oxidative stress following paradoxical sleep deprivation in rats. Neurosci. Lett. 235, 25–28. doi: 10.1016/S0304-3940(97)00706-4
Dawson, M. R. L., Polito, A., Levine, J. M., and Reynolds, R. (2003). NG2-expressing glial progenitor cells: an abundant and widespread population of cycling cells in the adult rat CNS. Mol. Cell. Neurosci. 24, 476–488. doi: 10.1016/S1044-7431(03)00210-0
De Nuccio, C., Bernardo, A., Troiano, C., Brignone, M. S., Falchi, M., Greco, A., et al. (2020). Nrf2 and ppar-γ pathways in oligodendrocyte progenitors: focus on ros protection, mitochondrial biogenesis and promotion of cell differentiation. Int. J. Mol. Sci. 21, 1–21. doi: 10.3390/ijms21197216
Dikalov, S. I., Nazarewicz, R. R., Bikineyeva, A., Hilenski, L., Lassègue, B., Griendling, K. K., et al. (2014). Nox2-Induced production of mitochondrial Superoxide in Angiotensin ii-mediated endothelial oxidative stress and hypertension. Antioxid. Redox Signal. 20, 281–294. doi: 10.1089/ars.2012.4918
Doughan, A. K., Harrison, D. G., and Dikalov, S. I. (2008). Molecular mechanisms of angiotensin II-mediated mitochondrial dysfunction: linking mitochondrial oxidative damage and vascular endothelial dysfunction. Circ. Res. 102, 488–496. doi: 10.1161/CIRCRESAHA.107.162800
Dröge, W. (2002). Free radicals in the physiological control of cell function. Physiol. Rev. 82, 47–95. doi: 10.1152/physrev.00018.2001
Drogos, L. L., Gill, S. J., Tyndall, A. V., Raneri, J. K., Parboosingh, J. S., Naef, A., et al. (2016). Evidence of association between sleep quality and APOE ε4 in healthy older adults. Neurology 87, 1836–1842. doi: 10.1212/WNL.0000000000003255
Dunietz, G. L., Chervin, R. D., Burke, J. F., Conceicao, A. S., and Braley, T. J. (2021). Obstructive Sleep Apnea Treatment and Dementia Risk in Older Adults. Sleep zsab076. doi: 10.1093/sleep/zsab076
Everson, C. A., Laatsch, C. D., and Hogg, N. (2005). Antioxidant defense responses to sleep loss and sleep recovery. Am. J. Physiol. Regul. Integr. Comp. Physiol. 288, R374–R383. doi: 10.1152/ajpregu.00565.2004
Fiford, C. M., Manning, E. N., Bartlett, J. W., Cash, D. M., Malone, I. B., Ridgway, G. R., et al. (2017). White matter hyperintensities are associated with disproportionate progressive hippocampal atrophy. Hippocampus 27, 249–262. doi: 10.1002/hipo.22690
Franczak, A., Bil-Lula, I., Sawicki, G., Fenton, M., Ayas, N., and Skomro, R. (2019a). Matrix metalloproteinases as possible biomarkers of obstructive sleep apnea severity - A systematic review. Sleep Med. Rev. 46, 9–16. doi: 10.1016/j.smrv.2019.03.010
Franczak, A., Sawicka, J., Nocon, A., Bil-Lula, I., Fenton, M., and Hanly, P. (2019b). Urinary matrix metalloproteinase-2 (MMP-2) activity in patients with obstructive sleep apnea. Sleep Med. 64:S119. doi: 10.1016/j.sleep.2019.11.327
Freeman, L. R., and Keller, J. N. (2012). Oxidative stress and cerebral endothelial cells: regulation of the blood-brain-barrier and antioxidant based interventions. Biochim. Biophys. Acta Mol. Basis Dis. 1822, 822–829. doi: 10.1016/j.bbadis.2011.12.009
French, H. M., Reid, M., Mamontov, P., Simmons, R. A., and Grinspan, J. B. (2009). Oxidative stress disrupts oligodendrocyte maturation. J. Neurosci. Res. 87, 3076–3087. doi: 10.1002/jnr.22139
Giacci, M., and Fitzgerald, M. (2018). Oligodendroglia Are Particularly Vulnerable to Oxidative Damage After Neurotrauma In Vivo. J. Exp. Neurosci. 12:1179069518810004. doi: 10.1177/1179069518810004
Gopalakrishnan, A., Ji, L. L., and Cirelli, C. (2004). Sleep deprivation and cellular responses to oxidative stress. Sleep 27, 27–35. doi: 10.1093/sleep/27.1.27
Gorter, R. P., and Baron, W. (2020). Matrix metalloproteinases shape the oligodendrocyte (niche) during development and upon demyelination. Neurosci. Lett. 729:134980. doi: 10.1016/j.neulet.2020.134980
Grimmer, T., Faust, M., Auer, F., Alexopoulos, P., Förstl, H., Henriksen, G., et al. (2012). White matter hyperintensities predict amyloid increase in Alzheimer’s disease. Neurobiol. Aging 33, 2766–2773. doi: 10.1016/j.neurobiolaging.2012.01.016
Gu, Y., Dee, C. M., and Shen, J. (2011). Interaction of free radicals, matrix metalloproteinases and caveolin-1 impacts blood-brain barrier permeability. Front. Biosci. 3, 1216–1231. doi: 10.2741/222
Hachul De Campos, H., Brandão, L., D’Almeida, V., Grego, B., Bittencourt, L., Tufik, S., et al. (2006). Sleep disturbances, oxidative stress and cardiovascular risk parameters in postmenopausal women complaining of insomnia. Climacteric 9, 312–319. doi: 10.1080/13697130600871947
Halliday, M. R., Rege, S. V., Ma, Q., Zhao, Z., Miller, C. A., Winkler, E. A., et al. (2016). Accelerated pericyte degeneration and blood-brain barrier breakdown in apolipoprotein E4 carriers with Alzheimer’s disease. J. Cereb. Blood Flow Metab. 36, 216–227. doi: 10.1038/jcbfm.2015.44
He, P., Talukder, M. A. H., and Gao, F. (2020). Oxidative Stress and Microvessel Barrier Dysfunction. Front. Physiol. 11:472. doi: 10.3389/fphys.2020.00472
Hertze, J., Palmqvist, S., Minthon, L., and Hansson, O. (2013). Tau Pathology and Parietal White Matter Lesions Have Independent but Synergistic Effects on Early Development of Alzheimer’s Disease. Dement. Geriatr. Cogn. Dis. Extra 3, 113–122. doi: 10.1159/000348353
Hill, V. M., O’Connor, R. M., Sissoko, G. B., Irobunda, I. S., Leong, S., Canman, J. C., et al. (2018). A bidirectional relationship between sleep and oxidative stress in Drosophila. PLoS Biol. 16:e2005206. doi: 10.1371/journal.pbio.2005206
Horiuchi, M., Maezawa, I., Itoh, A., Wakayama, K., Jin, L. W., Itoh, T., et al. (2012). Amyloid β1-42 oligomer inhibits myelin sheet formation in vitro. Neurobiol. Aging 33, 499–509. doi: 10.1016/j.neurobiolaging.2010.05.007
Htoo, A. K., Greenberg, H., Tongia, S., Chen, G., Henderson, T., Wilson, D., et al. (2006). Activation of nuclear factor κB in obstructive sleep apnea: a pathway leading to systemic inflammation. Sleep Breath. 10, 43–50. doi: 10.1007/s11325-005-0046-6
Huang, T. T., Zou, Y., and Corniola, R. (2012). Oxidative stress and adult neurogenesis-Effects of radiation and superoxide dismutase deficiency. Semin. Cell Dev.Biol. 23, 738–744. doi: 10.1016/j.semcdb.2012.04.003
Huang, Y., Coupland, N. J., Lebel, R. M., Carter, R., Seres, P., Wilman, A. H., et al. (2013). Structural changes in hippocampal subfields in major depressive disorder: a high-field magnetic resonance imaging study. Biol. Psychiatry 74, 62–68. doi: 10.1016/j.biopsych.2013.01.005
Hurtado-Alvarado, G., Velázquez-Moctezuma, J., and Gómerz-González, B. (2017). Chronic sleep restriction disrupts interendothelial junctions in the hippocampus and increases blood-brain barrier permeability. J. Microsc. 268, 28–38. doi: 10.1111/jmi.12583
Ibarretxe, G., Sánchez-Gómez, M. V., Campos-Esparza, M. R., Alberdi, E., and Matute, C. (2006). Differential oxidative stress in oligodendrocytes and neurons after excitotoxic insults and protection by natural polyphenols. Glia 53, 201–211. doi: 10.1002/glia.20267
Iliff, J. J., Wang, M., Liao, Y., Plogg, B. A., Peng, W., Gundersen, G. A., et al. (2012). A paravascular pathway facilitates CSF flow through the brain parenchyma and the clearance of interstitial solutes, including amyloid β. Sci. Transl. Med. 4:147ra111. doi: 10.1126/scitranslmed.3003748
Iturria-Medina, Y., Sotero, R. C., Toussaint, P. J., Mateos-Pérez, J. M., Evans, A. C., Weiner, M. W., et al. (2016). Early role of vascular dysregulation on late-onset Alzheimer’s disease based on multifactorial data-driven analysis. Nat. Commun. 7, 1–14. doi: 10.1038/ncomms11934
Jin, Z. G., Melaragno, M. G., Liao, D. F., Yan, C., Haendeler, J., Suh, Y. A., et al. (2000). Cyclophilin A is a secreted growth factor induced by oxidative stress. Circ. Res. 27, 789–796. doi: 10.1161/01.res.87.9.789
Joiner, W. J. (2016). Unraveling the Eutionary Determinants of Sleep. Curr. Biol. 26, R1073–R1087. doi: 10.1016/j.cub.2016.08.068
Kadotani, H., Kadotani, T., Young, T., Peppard, P. E., Finn, L., Colrain, I. M., et al. (2001). Association between apolipoprotein E ε4 and sleep-disordered breathing in adults. J. Am. Med. Assoc. 285, 2888–2890. doi: 10.1001/jama.285.22.2888
Kandel, B. M., Avants, B. B., Gee, J. C., McMillan, C. T., Erus, G., Doshi, J., et al. (2016). White matter hyperintensities are more highly associated with preclinical Alzheimer’s disease than imaging and cognitive markers of neurodegeneration. Alzheimers Dement. 4, 18–27. doi: 10.1016/j.dadm.2016.03.001
Kaur, G., Sharma, A., Guruprasad, K., and Pati, P. K. (2014). Versatile roles of plant NADPH oxidases and emerging concepts. Biotechnol. Adv. 32, 551–563. doi: 10.1016/j.biotechadv.2014.02.002
Kim, H. W., Hong, J., and Jeon, J. C. (2020). Cerebral Small Vessel Disease and Alzheimer’s Disease: a Review. Front. Neurol. 11:927. doi: 10.3389/fneur.2020.00927
Klohs, J. (2020). An Integrated View on Vascular Dysfunction in Alzheimer’s Disease. Neurodegener. Dis. 19, 109–127. doi: 10.1159/000505625
Koh, K., Evans, J. M., Hendricks, J. C., and Sehgal, A. (2006). A Drosophila model for age-associated changes in sleep:wake cycles. Proc. Natl. Acad. Sci. U. S. A. 103, 13843–13847. doi: 10.1073/pnas.0605903103
Koizumi, K., Hattori, Y., Ahn, S. J., Buendia, I., Ciacciarelli, A., Uekawa, K., et al. (2018). Apoε4 disrupts neurovascular regulation and undermines white matter integrity and cognitive function. Nat. Commun. 9:3816. doi: 10.1038/s41467-018-06301-2
Kreibich, A. S., and Blendy, J. A. (2004). cAMP response element-binding protein is required for stress but not cocaine-induced reinstatement. J. Neurosci. 24, 6686–6692. doi: 10.1523/JNEUROSCI.1706-04.2004
Kress, G. J., Liao, F., Dimitry, J., Cedeno, M. R., FitzGerald, G. A., and Holtzman, D. M. (2018). Regulation of amyloid-β dynamics and pathology by the circadian clock. J. Exp. Med. 215, 1059–1068. doi: 10.1084/jem.20172347
Kuller, L. H., Lopez, O. L., Newman, A., Beauchamp, N. J., Burke, G., Dulberg, C., et al. (2003). Risk factors for dementia in the Cardiovascular Health Cognition Study. Neuroepidemiology 22, 13–22. doi: 10.1159/000067109
Kurachi, M., Mikuni, M., and Ishizaki, Y. (2016). Extracellular vesicles from vascular endothelial cells promote survival, proliferation and motility of oligodendrocyte precursor cells. PLoS One 11:e0159158. doi: 10.1371/journal.pone.0159158
Kuriakose, M., Younger, D., Ravula, A. R., Alay, E., Rama Rao, K. V., and Chandra, N. (2019). Synergistic Role of Oxidative Stress and Blood-Brain Barrier Permeability as Injury Mechanisms in the Acute Pathophysiology of Blast-induced Neurotrauma. Sci. Rep. 9:7717. doi: 10.1038/s41598-019-44147-w
Lassmann, H., and van Horssen, J. (2016). Oxidative stress and its impact on neurons and glia in multiple sclerosis lesions. Biochim. Biophys. Acta Mol. Basis Dis. 1862, 506–510. doi: 10.1016/j.bbadis.2015.09.018
Lim, A. S. P., Kowgier, M., Yu, L., Buchman, A. S., and Bennett, D. A. (2013a). Sleep fragmentation and the risk of incident Alzheimer’s disease and cognitive decline in older persons. Sleep 36, 1027–1032. doi: 10.5665/sleep.2802
Lim, A. S. P., Yu, L., Kowgier, M., Schneider, J. A., Buchman, A. S., and Bennett, D. A. (2013b). Modification of the relationship of the apolipoprotein E ε4 allele to the risk of Alzheimer disease and neurofibrillary tangle density by sleep. JAMA Neurol. 70, 1544–1551. doi: 10.1001/jamaneurol.2013.4215
Liu, J., Chang, L., Song, Y., Li, H., and Wu, Y. (2019). The Role of NMDA Receptors in Alzheimer’s Disease. Front. Neurosci. 13:43. doi: 10.3389/fnins.2019.00043
Lloret, A., Buj, J., Badia, M. C., Sastre, J., Morera, J., and Viña, J. (2007). Obstructive sleep apnea: arterial oxygen desaturation coincides with increases in systemic oxidative stress markers measured with continuous monitoring. Free Radic. Biol. Med. 42, 893–894. doi: 10.1016/j.freeradbiomed.2006.10.051
Lloret, A., Esteve, D., Lloret, M. A., Cervera-Ferri, A., Lopez, B., Nepomuceno, M., et al. (2019). When Does Alzheimer’s Disease Really Start? The Role of Biomarkers. Int. J. Mol. Sci. 20:5536. doi: 10.3390/ijms20225536
Lloret, M. A., Cervera-Ferri, A., Nepomuceno, M., Monllor, P., Esteve, D., and Lloret, A. (2020). Is sleep disruption a cause or consequence of alzheimer’s disease? Reviewing its possible role as a biomarker. Int. J. Mol. Sci. 21:1168. doi: 10.3390/ijms21031168
Maki, T., Maeda, M., Uemura, M., Lo, E. K., Terasaki, Y., Liang, A. C., et al. (2015). Potential interactions between pericytes and oligodendrocyte precursor cells in perivascular regions of cerebral white matter. Neurosci. Lett. 597, 164–169. doi: 10.1016/j.neulet.2015.04.047
Mashaqi, S., Mansour, H. M., Alameddin, H., Combs, D., Patel, S., Estep, L., et al. (2021). Matrix metalloproteinase-9 as a messenger in the cross talk between obstructive sleep apnea and comorbid systemic hypertension, cardiac remodeling, and ischemic stroke: a literature review. J. Clin. Sleep Med. 17, 567–591. doi: 10.5664/jcsm.8928
Masood, A., Nadeem, A., Mustafa, S. J., and O’Donnell, J. M. (2008). Reversal of oxidative stress-induced anxiety by inhibition of phosphodiesterase-2 in mice. J. Pharmacol. Exp. Ther. 326, 369–379. doi: 10.1124/jpet.108.137208
Miyamoto, N., Maki, T., Pham, L. D. D., Hayakawa, K., Seo, J. H., Mandeville, E. T., et al. (2013). Oxidative stress interferes with white matter renewal after prolonged cerebral hypoperfusion in mice. Stroke 44, 3516–3521. doi: 10.1161/STROKEAHA.113.002813
Montagne, A., Barnes, S. R., Sweeney, M. D., Halliday, M. R., Sagare, A. P., and Zhao, Z. (2015). Blood-Brain barrier breakdown in the aging human hippocampus. Neuron 85, 296–302. doi: 10.1016/j.neuron.2014.12.032
Montagne, A., Nation, D. A., Sagare, A. P., Barisano, G., Sweeney, M. D., Chakhoyan, A., et al. (2020). APOE4 leads to blood–brain barrier dysfunction predicting cognitive decline. Nature 581, 71–76. doi: 10.1038/s41586-020-2247-3
Nair, D., Dayyat, E. A., Zhang, S. X., Wang, Y., and Gozal, D. (2011a). Intermittent hypoxia-induced cognitive deficits are mediated by NADPH oxidase activity in a murine model of sleep apnea. PLoS One 6:e19847. doi: 10.1371/journal.pone.0019847
Nair, D., Zhang, S. X., Ramesh, V., Hakim, F., Kaushal, N., Wang, Y., et al. (2011b). Sleep fragmentation induces cognitive deficits via nicotinamide adenine dinucleotide phosphate oxidase-dependent pathways in mouse. Am. J. Respir. Crit. Care Med. 184, 1305–1312. doi: 10.1164/rccm.201107-1173OC
Nation, D. A., Sweeney, M. D., Montagne, A., Sagare, A. P., D’Orazio, L. M., Pachicano, M., et al. (2019). Blood–brain barrier breakdown is an early biomarker of human cognitive dysfunction. Nat. Med. 25, 270–276. doi: 10.1038/s41591-018-0297-y
Nazarewicz, R. R., Dikalova, A. E., Bikineyeva, A., and Dikalov, S. I. (2013). Nox2 as a potential target of mitochondrial superoxide and its role in endothelial oxidative stress. Am. J. Physiol. Heart Circ. Physiol. 305, H1131–H1140. doi: 10.1152/ajpheart.00063.2013
Nikolakopoulou, A. M., Montagne, A., Kisler, K., Dai, Z., Wang, Y., Huuskonen, M. T., et al. (2019). Pericyte loss leads to circulatory failure and pleiotrophin depletion causing neuron loss. Nat. Neurosci. 22, 1089–1098. doi: 10.1038/s41593-019-0434-z
Nunomura, A., Perry, G., Aliev, G., Hirai, K., Takeda, A., Balraj, E. K., et al. (2001). Oxidative damage is the earliest event in Alzheimer disease. J. Neuropathol. Exp. Neurol. 60, 759–767. doi: 10.1093/jnen/60.8.759
Olney, J. W., Wozniak, D. F., and Farber, N. B. (1997). Excitotoxic neurodegeneration in Alzheimer disease: new hypothesis and new therapeutic strategies. Arch. Neurol. 54, 1234–1240. doi: 10.1001/archneur.1997.00550220042012
Pan, T., Liu, S., Ke, S., Wang, E., Jiang, Y., and Wang, S. (2021). Association of obstructive sleep apnea with cognitive decline and age among non-demented older adults. Neurosci. Lett. 756:135955. doi: 10.1016/j.neulet.2021.135955
Parodi-Rullán, R., Ghiso, J., Cabrera, E., Rostagno, A., and Fossati, S. (2020). Alzheimer’s amyloid β heterogeneous species differentially affect brain endothelial cell viability, blood-brain barrier integrity, and angiogenesis. Aging Cell 19:e13258. doi: 10.1111/acel.13258
Patki, G., Allam, F. H., Atrooz, F., Dao, A. T., Solanki, N., Chugh, G., et al. (2013a). Grape Powder Intake Prevents Ovariectomy-Induced Anxiety-Like Behavior, Memory Impairment and High Blood Pressure in Female Wistar Rats. PLoS One 8:74522. doi: 10.1371/journal.pone.0074522
Patki, G., Solanki, N., Atrooz, F., Allam, F., and Salim, S. (2013b). Depression, anxiety-like behavior and memory impairment are associated with increased oxidative stress and inflammation in a rat model of social stress. Brain Res. 1539, 73–86. doi: 10.1016/j.brainres.2013.09.033
Poljsak, B., and Milisav, I. (2012). The neglected significance of “antioxidative stress.” Oxid. Med. Cell. Longev. 2012:480895. doi: 10.1155/2012/480895
Potvin, O., Lorrain, D., Forget, H., Dubé, M., Grenier, S., Préville, M., et al. (2012). Sleep quality and 1-year incident cognitive impairment in community-dwelling older adults. Sleep 35, 491–499. doi: 10.5665/sleep.1732
Quintela-López, T., Ortiz-Sanz, C., Serrano-Regal, M. P., Gaminde-Blasco, A., Valero, J., Baleriola, J., et al. (2019). Aβ oligomers promote oligodendrocyte differentiation and maturation via integrin β1 and Fyn kinase signaling. Cell Death Dis. 10:445. doi: 10.1038/s41419-019-1636-8
Radley, J. J., Rocher, A. B., Miller, M., Janssen, W. G. M., Liston, C., Hof, P. R., et al. (2006). Repeated stress induces dendritic spine loss in the rat medial prefrontal cortex. Cereb. Cortex 16, 313–320. doi: 10.1093/cercor/bhi104
Richardson, W. D., Young, K. M., Tripathi, R. B., and McKenzie, I. (2011). NG2-glia as Multipotent Neural Stem Cells: fact or Fantasy? Neuron 70, 661–673. doi: 10.1016/j.neuron.2011.05.013
Sachdev, P. S., Zhuang, L., Braidy, N., and Wen, W. (2013). Is Alzheimer’s a disease of the white matter? Curr. Opin. Psychiatry 26, 244–251. doi: 10.1097/YCO.0b013e32835ed6e8
Sahoo, S., Meijles, D. N., and Pagano, P. J. (2016). NADPH oxidases: key modulators in aging and age-related cardiovascular diseases? Clin. Sci. 130, 317–335. doi: 10.1042/CS20150087
Salim, S., Asghar, M., Chugh, G., Taneja, M., Xia, Z., and Saha, K. (2010a). Oxidative stress: a potential recipe for anxiety, hypertension and insulin resistance. Brain Res. 1359, 178–185. doi: 10.1016/j.brainres.2010.08.093
Salim, S., Asghar, M., Taneja, M., Hovatta, I., Chugh, G., Vollert, C., et al. (2011a). Potential contribution of oxidative stress and inflammation to anxiety and hypertension. Brain Res. 1404, 63–71. doi: 10.1016/j.brainres.2011.06.024
Salim, S., Asghar, M., Taneja, M., Hovatta, I., Wu, Y. L., Saha, K., et al. (2011b). Novel role of RGS2 in regulation of antioxidant homeostasis in neuronal cells. FEBS Lett. 585, 1375–1381. doi: 10.1016/j.febslet.2011.04.023
Salim, S., Sarraj, N., Taneja, M., Saha, K., Tejada-Simon, M. V., and Chugh, G. (2010b). Moderate treadmill exercise prevents oxidative stress-induced anxiety-like behavior in rats. Behav. Brain Res. 208, 545–552. doi: 10.1016/j.bbr.2009.12.039
Salvadó, G., Brugulat-Serrat, A., Sudre, C. H., Grau-Rivera, O., Suárez-Calvet, M., Falcon, C., et al. (2019). Spatial patterns of white matter hyperintensities associated with Alzheimer’s disease risk factors in a cognitively healthy middle-aged cohort. Alzheimers Res. Ther. 11:12. doi: 10.1186/s13195-018-0460-1
Saridin, F. N., Hilal, S., Villaraza, S. G., Reilhac, A., Gyanwali, B., Tanaka, T., et al. (2020). Brain amyloid β, cerebral small vessel disease, and cognition: a memory clinic study. Neurology 95, e2845–e2853. doi: 10.1212/WNL.0000000000011029
Sarnowska, A. (2002). Application of organotypic hippocampal culture for study of selective neuronal death. Folia Neuropathol. 40, 101–106. Retrieved from europepmc:article/med/12230254,
Schultz, N., Brännström, K., Byman, E., Moussaud, S., Nielsen, H. M., Olofsson, A., et al. (2018). Amyloid-beta 1-40 is associated with alterations in NG2+ pericyte population ex vivo and in vitro. Aging Cell 17:e12728. doi: 10.1111/acel.12728
Schulz, E., Wenzel, P., Münzel, T., and Daiber, A. (2014). Mitochondrial redox signaling: interaction of mitochondrial reactive oxygen species with other sources of oxidative stress. Antioxid. Redox Signal. 20, 308–324. doi: 10.1089/ars.2012.4609
Semyachkina-Glushkovskaya, O., Postnov, D., Penzel, T., and Kurths, J. (2020). Sleep as a Novel Biomarker and a Promising Therapeutic Target for Cerebral Small Vessel Disease: a Review Focusing on Alzheimer’s Disease and the Blood-Brain Barrier. Int. J. Mol. Sci. 21:6293. doi: 10.3390/ijms21176293
Seo, J. H., Maki, T., Maeda, M., Miyamoto, N., Liang, A. C., Hayakawa, K., et al. (2014). Oligodendrocyte precursor cells support blood-brain barrier integrity via TGF-β signaling. PLoS One 9:e103174. doi: 10.1371/journal.pone.0103174
Sies, H. (1986). Biochemistry of Oxidative Stress. Angew. Chem. Int. Ed. Engl. 25, 1058–1071. doi: 10.1002/anie.198610581
Sindi, S., Kåreholt, I., Johansson, L., Skoog, J., Sjöberg, L., Wang, H. X., et al. (2018). Sleep disturbances and dementia risk: a multicenter study. Alzheimers Dement. 14, 1235–1242. doi: 10.1016/j.jalz.2018.05.012
Snapyan, M., Lemasson, M., Brill, M. S., Blais, M., Massouh, M., Ninkovic, J., et al. (2009). Vasculature guides migrating neuronal precursors in the adult mammalian forebrain via brain-derived neurotrophic factor signaling. J. Neurosci. 29, 4172–4188. doi: 10.1523/JNEUROSCI.4956-08.2009
Solanki, N., Alkadhi, I., Atrooz, F., Patki, G., and Salim, S. (2015). Grape powder prevents cognitive, behavioral, and biochemical impairments in a rat model of posttraumatic stress disorder. Nutr. Res. 35, 65–75. doi: 10.1016/j.nutres.2014.11.008
Song, T. J., Park, J. H., Choi, K., Chang, Y., Moon, J., Kim, J. H., et al. (2017). Moderate-to-severe obstructive sleep apnea is associated with cerebral small vessel disease. Sleep Med. 30, 36–42. doi: 10.1016/j.sleep.2016.03.006
Spaas, J., van Veggel, L., Schepers, M., Tiane, A., van Horssen, J., Wilson, D. M., et al. (2021). Oxidative stress and impaired oligodendrocyte precursor cell differentiation in neurological disorders. Cell. Mol. Life Sci. 78, 4615–4637. doi: 10.1007/s00018-021-03802-0
Sweeney, M. D., Montagne, A., Sagare, A. P., Nation, D. A., Schneider, L. S., Chui, H. C., et al. (2019). Vascular dysfunction—The disregarded partner of Alzheimer’s disease. Alzheimers Dement. 15, 158–167. doi: 10.1016/j.jalz.2018.07.222
Sweeney, M. D., Sagare, A. P., and Zlokovic, B. V. (2018). Blood-brain barrier breakdown in Alzheimer disease and other neurodegenerative disorders. Nat. Rev. Neurol. 14, 133–150. doi: 10.1038/nrneurol.2017.188
Takac, I., Schröder, K., Zhang, L., Lardy, B., Anilkumar, N., Lambeth, J. D., et al. (2011). The E-loop is involved in hydrogen peroxide formation by the NADPH oxidase Nox4. J. Biol. Chem. 286, 13304–13313. doi: 10.1074/jbc.M110.192138
Teixeira, K. R. C., dos Santos, C. P., de Medeiros, L. A., Mendes, J. A., Cunha, T. M., De Angelis, K., et al. (2019). Night workers have lower levels of antioxidant defenses and higher levels of oxidative stress damage when compared to day workers. Sci. Rep. 9:4455. doi: 10.1038/s41598-019-40989-6
Thompson, C. L., Wisor, J. P., Lee, C. K., Pathak, S. D., Gerashchenko, D., Smith, K. A., et al. (2010). Molecular and anatomical signatures of sleep deprivation in the mouse brain. Front. Neurosci. 4:165. doi: 10.3389/fnins.2010.00165
Thorburne, S. K., and Juurlink, B. H. (1996). Low glutathione and high iron govern the susceptibility of oligodendroglial precursors to oxidative stress. J. Neurochem. 67, 1014–1022. doi: 10.1046/j.1471-4159.1996.67031014.x
Uysal, N., Tugyan, K., Aksu, I., Ozbal, S., Ozdemir, D., Dayi, A., et al. (2012). Age-related changes in apoptosis in rat hippocampus induced by oxidative stress. Biotech. Histochem. 87, 98–104. doi: 10.3109/10520295.2011.556665
Van De Haar, H. J., Burgmans, S., Jansen, J. F. A., Van Osch, M. J. P., Van Buchem, M. A., Muller, M., et al. (2016a). Blood-brain barrier leakage in patients with early Alzheimer disease. Radiology 281, 527–535. doi: 10.1148/radiol.2016152244
van de Haar, H. J., Jansen, J. F. A., van Osch, M. J. P., van Buchem, M. A., Muller, M., Wong, S. M., et al. (2016b). Neurovascular unit impairment in early Alzheimer’s disease measured with magnetic resonance imaging. Neurobiol. Aging 45, 190–196. doi: 10.1016/j.neurobiolaging.2016.06.006
Villafuerte, G., Miguel-Puga, A., Murillo Rodríguez, E., Machado, S., Manjarrez, E., and Arias-Carrión, O. (2015). Sleep deprivation and oxidative stress in animal models: a systematic review. Oxid. Med. Cell. Longev. 2015:234952. doi: 10.1155/2015/234952
Vornov, J. J., Park, J., and Thomas, A. G. (1998). Regional vulnerability to endogenous and exogenous oxidative stress in organotypic hippocampal culture. Exp. Neurol. 149, 109–122. doi: 10.1006/exnr.1997.6673
Vyas, A., Mitra, R., Shankaranarayana Rao, B. S., and Chattarji, S. (2002). Chronic stress induces contrasting patterns of dendritic remodeling in hippocampal and amygdaloid neurons. J. Neurosci. 22, 6810–6818. doi: 10.1523/jneurosci.22-15-06810.2002
Wang, J., Chen, X., Liao, J., Zhou, L., Han, H., Tao, J., et al. (2020). Non breathing-related sleep fragmentation and imaging markers in patients with atherosclerotic cerebral small vessel disease (CSVD): a cross-sectional case-control study. BMC Neurol. 20:98. doi: 10.1186/s12883-020-01647-x
Wang, J., Chen, X., Liao, J., Zhou, L., Liao, S., Shan, Y., et al. (2019). The influence of non-breathing-related sleep fragmentation on cognitive function in patients with cerebral small vessel disease. Neuropsychiatr. Dis. Treat. 15, 1009–1014. doi: 10.2147/NDT.S193869
Wang, N., Shi, X. F., Khan, S. A., Wang, B., Semenza, G. L., Prabhakar, N. R., et al. (2020). Hypoxia-inducible factor-1 mediates pancreatic β-cell dysfunction by intermittent hypoxia. Am. J. Physiol. Cell Physiol. 319, C922–C932. doi: 10.1152/ajpcell.00309.2020
Wang, X., and Michaelis, E. K. (2010). Selective neuronal vulnerability to oxidative stress in the brain. Front. Aging Neurosci. 2:12. doi: 10.3389/fnagi.2010.00012
Wang, X., Pal, R., Chen, X. W., Limpeanchob, N., Kumar, K. N., and Michaelis, E. K. (2005). High intrinsic oxidative stress may underlie selective vulnerability of the hippocampal CA1 region. Mol. Brain Res. 140, 120–126. doi: 10.1016/j.molbrainres.2005.07.018
Wardlaw, J. M., Smith, E. E., Biessels, G. J., Cordonnier, C., Fazekas, F., Frayne, R., et al. (2013). Neuroimaging standards for research into small vessel disease and its contribution to ageing and neurodegeneration. Lancet Neurol. 12, 822–838. doi: 10.1016/S1474-4422(13)70124-8
Wellman, C. L. (2001). Dendritic reorganization in pyramidal neurons in medial prefrontal cortex after chronic corticosterone administration. J. Neurobiol. 49, 245–253. doi: 10.1002/neu.1079
Wilde, G. J. C., Pringle, A. K., Wright, P., and Iannotti, F. (1997). Differential vulnerability of the CA1 and CA3 subfields of the hippocampus to superoxide and hydroxyl radicals in vitro. J. Neurochem. 69, 883–886. doi: 10.1046/j.1471-4159.1997.69020883.x
Wood, S. K., Walker, H. E., Valentino, R. J., and Bhatnagar, S. (2010). Individual differences in reactivity to social stress predict susceptibility and resilience to a depressive phenotype: role of corticotropin-releasing factor. Endocrinology 151, 1795–1805. doi: 10.1210/en.2009-1026
Xie, L., Kang, H., Xu, Q., Chen, M. J., Liao, Y., Thiyagarajan, M., et al. (2013). Sleep drives metabolite clearance from the adult brain. Science 342, 373–377. doi: 10.1126/science.1241224
Yu, L., Boyle, P. A., Nag, S., Leurgans, S., Buchman, A. S., Wilson, R. S., et al. (2015). APOE and cerebral amyloid angiopathy in community-dwelling older persons. Neurobiol. Aging 36, 2946–2953. doi: 10.1016/j.neurobiolaging.2015.08.008
Keywords: demyelination, sleep dysfunction, blood-brain barrier permeability, ApoE4 and AD risk, vessel dysfunction, oligodendrocyte precursor cell, reactive oxygen species, beta-amyloid
Citation: Lloret A, Esteve D, Lloret MA, Monllor P, López B, León JL and Cervera-Ferri A (2021) Is Oxidative Stress the Link Between Cerebral Small Vessel Disease, Sleep Disruption, and Oligodendrocyte Dysfunction in the Onset of Alzheimer’s Disease? Front. Physiol. 12:708061. doi: 10.3389/fphys.2021.708061
Received: 11 May 2021; Accepted: 28 July 2021;
Published: 25 August 2021.
Edited by:
Cristina M. Sena, University of Coimbra, PortugalReviewed by:
Magdalena Chrzanowska, Versiti Blood Research Institute, United StatesPaula I. Moreira, University of Coimbra, Portugal
Copyright © 2021 Lloret, Esteve, Lloret, Monllor, López, León and Cervera-Ferri. This is an open-access article distributed under the terms of the Creative Commons Attribution License (CC BY). The use, distribution or reproduction in other forums is permitted, provided the original author(s) and the copyright owner(s) are credited and that the original publication in this journal is cited, in accordance with accepted academic practice. No use, distribution or reproduction is permitted which does not comply with these terms.
*Correspondence: Ana Lloret, YW5hLmxsb3JldEB1di5lcw==