- Department of Physiology, School of Medicine, Tulane University Health Sciences Center, New Orleans, LA, United States
The discovery of atrial, brain, and C-type natriuretic peptides (ANP, BNP, and CNP) and their cognate receptors has greatly increased our knowledge of the control of hypertension and cardiovascular homeostasis. ANP and BNP are potent endogenous hypotensive hormones that elicit natriuretic, diuretic, vasorelaxant, antihypertrophic, antiproliferative, and antiinflammatory effects, largely directed toward the reduction of blood pressure (BP) and cardiovascular diseases (CVDs). The principal receptor involved in the regulatory actions of ANP and BNP is guanylyl cyclase/natriuretic peptide receptor-A (GC-A/NPRA), which produces the intracellular second messenger cGMP. Cellular, biochemical, molecular, genetic, and clinical studies have facilitated understanding of the functional roles of natriuretic peptides (NPs), as well as the functions of their receptors, and signaling mechanisms in CVDs. Transgenic and gene-targeting (gene-knockout and gene-duplication) strategies have produced genetically altered novel mouse models and have advanced our knowledge of the importance of NPs and their receptors at physiological and pathophysiological levels in both normal and disease states. The current review describes the past and recent research on the cellular, molecular, genetic mechanisms and functional roles of the ANP-BNP/NPRA system in the physiology and pathophysiology of cardiovascular homeostasis as well as clinical and diagnostic markers of cardiac disorders and heart failure. However, the therapeutic potentials of NPs and their receptors for the diagnosis and treatment of cardiovascular diseases, including hypertension, heart failure, and stroke have just begun to be expanded. More in-depth investigations are needed in this field to extend the therapeutic use of NPs and their receptors to treat and prevent CVDs.
Introduction
The natriuretic peptides (NPs) family contains a group of hormones that are pivotal in the control of cardiovascular, endocrine, renal, and vascular homeostasis (Brenner et al., 1990; Drewett and Garbers, 1994; de Bold et al., 2001; McGrath et al., 2005; Pandey, 2005a; Rubattu et al., 2006; Kishimoto et al., 2011). Atrial and brain natriuretic peptides (ANP, BNP) are the members of the NPs hormone family. A third member of the NPs family, C-type, natriuretic peptide (CNP) was also isolated and identified but each NP was found to be derived from a separate gene (Rosenzweig and Seidman, 1991; LaPointe, 2005; Schulz, 2005). ANP and BNP exhibit diuretic, natriuretic, vasorelaxant, antiproliferative, antiinflammatory, and antihypertrophic effects that are directed toward the reducing and controlling of body fluid volume, blood pressure (BP) and cardiovascular diseases (CVDs) (McGrath et al., 2005; Ellmers et al., 2007; Wang T.J. et al., 2007; Pandey, 2011; Volpe et al., 2014; Cannone et al., 2019). Although the possibility of NPs role in metabolic regulation has been given limited consideration but NPs potently affect lipid and glucose metabolism, may also contribute to the pathophysiological link between metabolic and CVDs (Schlueter et al., 2014; Jordan et al., 2018). ANP has been shown to induce lipolysis and lipid oxidation and to ameliorate insulin resistance (Birkenfeld et al., 2008; Coue and Moro, 2016). All three NPs (ANP, BNP, and CNP) have highly homologous sequence structures, bind to cognate cell surface receptors, and elicit discrete biological and physiological functions (Brenner et al., 1990; Koller and Goddel, 1992; Anand-Srivastava and Trachte, 1993; Khurana and Pandey, 1993; Pandey, 2008). Each member of the family of endogenous NPs, including ANP, BNP, CNP, and urodilatin, has an integral role in BP, renal dysfunction, and CVDs (de Bold, 1985; Levin et al., 1998; Pandey, 2011; Rubattu and Volpe, 2014).
Importantly, NPs not only regulate BP and CVDs, but also maintain natural antagonistic actions to the renin-angiotensin-aldosterone system (RAAS), exert antimitogenic effect, and inhibit myocardial hypertrophy and fibrosis. Moreover, NPs play roles in endothelial cell function, cartilage growth, immunity, and mitochondrial biogenesis (Pandey, 2005a; Vollmer, 2005; Garbers et al., 2006; Ellmers et al., 2007). The demonstration of structurally related NPs indicated that the physiological control of BP and cardiovascular homeostasis is both complex and multifactorial. Studies of a combination of cellular, biochemical, molecular, genetic, and pharmacological properties of NPs and their prototype receptors have suggested the hallmark functions with physiological and pathophysiological responsiveness, including cardiovascular, endocrine, renal, neuronal, skeletal, and immunological importance in health and disease (Kuhn, 2005; Vollmer, 2005; Kishimoto et al., 2011; Misono et al., 2011; Jordan et al., 2018; Pandey, 2018).
Different NPs receptors have been classified namely; NP receptor-A (NPRA), NP receptor-B (NPRB), and NP receptor-C (NPRC). NPRA and NPRB exhibit an intrinsic intracellular guanylyl cyclase (GC) catalytic domain and are designated as GC receptor-A (GC-A/NPRA) and GC receptor-B (GC-B/NPRB) (Drewett and Garbers, 1994; Levin et al., 1998; Pandey, 2005a). Both ANP and BNP bind and activate NPRA, which produces intracellular second messenger cGMP in response to hormone binding. CNP activates NPRB and also produces cGMP. All three NPs (ANP, BNP, CNP) bind to NPRC, which lacks a GC catalytic domain and does not increase levels of intracellular cGMP (Fuller et al., 1988; Garbers, 1992; Koller and Goddel, 1992; Khurana and Pandey, 1993; Matsukawa et al., 1999). NPRA is a principal loci involved in the regulatory action of ANP and BNP (Lucas et al., 2000; Tremblay et al., 2002; Pandey, 2005a). Determining the insight into the intricacies of ANP-BNP/NPRA/cGMP signaling is of primary importance if we are to understand both the receptor biology and CVDs arising from cell- and tissue-specific abnormal hormone-receptor interplay. The binding of ANP and BNP to the extracellular domain of NPRA seem to exerts a conformational shift or change, whereby the signal is transmitted to the intracellular GC catalytic region of NPRA, which then activates generation of the second messenger cGMP in target cells and tissues (Pandey and Singh, 1990; Garbers, 1992; Koller and Goddel, 1992).
Although, great importance has been placed on the functional roles of NPs and their receptors in renal, cardiovascular, endocrine, neuronal, skeletal, and adipose homeostasis, in-depth research is still attempting to fully understand their potential molecular and therapeutic targets in the diseases states. We expect that future research on NPs and their receptors will yield new therapeutic targets and novel loci for the prevention and treatment of hypertension, stroke, and cardiovascular events. Earlier studies focused on elucidating the biochemical, cellular, molecular, and genetic aspects of the mode of functioning of NPRA, which still are not fully understood (Kishimoto et al., 2011; Misono et al., 2011; Pandey, 2011). Studies of cultured cells in vitro and transgenic and gene-targeted (gene-knockout and gene-duplication) mouse models in vivo have greatly advanced our understanding of NPs and their receptors by delineating the normal and abnormal control of physiological and pathophysiological functions in CVDs (Pandey, 2005a, 2019; Ellmers et al., 2007; Volpe et al., 2019a).
Natriuretic Peptide Hormone Family
Almost four decades ago, it was firmly established that atrial homogenate contains diuretic and natriuretic activity and simultaneously identified and characterized the hormone atrial natriuretic factor (ANF) now designated as ANP in cardiac myocytes (de Bold et al., 1981; de Bold, 1985). ANP, the initial member of the NP hormone family, is predominantly synthesized and secreted from the cardiac atrium, which led to classification of the heart as an endocrine organ. The primary structure deduced from the cDNA sequence, demonstrated that ANP is synthesized as a 152-amino acid pre-pro-ANP that contains the sequences of biologically active hormone in the carboxyl-terminal region and has the major form of circulating ANP as a 28-amino acid peptide molecule (Maki et al., 1984). It was found that a 17-amino acid disulfide-bonded ring structure of circulating ANP is essential for its physiological functions. Indeed, disruption of the ring structure of ANP abolished its functional activity (Misono et al., 1984; de Bold, 1985; Brenner et al., 1990). BNP is synthesized as a 134-amino acid pre-pro-BNP that produces a 108-amino acid prohormone. Processing of pro-BNP yields a 75-residue amino-terminal-BNP (NT-pro-BNP) and a biologically active 32-amino acid circulating BNP molecule (Sudoh et al., 1988; Seilhamer et al., 1989). CNP is synthesized as a 103-amino acid pre-pro-CNP cleaved to a 53-amino acid peptide by the protease furin. It is subsequently processed to yield a 22-amino-acid biologically active CNP (Wu et al., 2003).
Cellular, biochemical, molecular, and immunohistological studies have suggested that three specific NPs (ANP, BNP, CNP) and their three distinct receptor subtypes (NPRA, NPRB, NPRC) have widespread cell and tissue distributions, indicating the pleotropic actions at the systemic and local levels (Pandey et al., 1986, 1988; Leitman et al., 1988; Brenner et al., 1990; Levin et al., 1998). ANP and BNP exhibit the most variability in primary sequence structure across species, while CNP is highly conserved among species. Subsequently, a 32-amino acid peptide, urodilatin (URO) was identified in urine. However, URO is not detected in the circulation. It appears to be a unique intrarenal NP with largely unexplored physiological functions (Schulz-Knappe et al., 1988; Saxenhofer et al., 1990; Feller et al., 1990; Goetz, 1991). Immunohistological staining has indicated that URO is mainly synthesized in renal cortical tubules around the collecting ducts; however, its exact role has yet to be determined (Sugimoto et al., 1993; Forssmann et al., 1994; Meyer et al., 1996). Additionally, another member of the NPs hormone family, the D-type natriuretic peptide (DNP), was initially isolated from the venom of green mamba (Dendroaspis angusticeps) as a 38-amino acid biologically active peptide molecule (Schweitz et al., 1992; Lisy et al., 1999). ANP shows a rapid clearance rate in the circulation; its half-life ranges from 0.5 to 3.5 min in experimental animals (Nakao et al., 1986; Yandle et al., 1986; Ruskoaho, 1992). In human, the half-life ranges between 2 and 2.5 min (Nakao et al., 1986). The clearance of BNP in humans occurs with both a short half-life of 3–4 min and a long half-life of 20–23 min (Mukoyama et al., 1991; Holmes et al., 1993). CNP has a half-life of 2−3 min in humans and approximately 1.5−2.0 min in experimental animals (Hunt et al., 1994; Charles et al., 1995).
The design of chimeric NPs has led to synthesis of the biologically active novel NPs of clinical importance, which represent single-chemical molecule with combined structural and functional properties (Lisy et al., 2008; Ichiki et al., 2019). Chimeric NPs exert the actions of more than one NP molecule, often with reduced undesirable or adverse hypotensive effects. Previously, a chimeric NP, namely CD-NP containing 22 residues of CNP and 15 residues at the carboxyl-terminus of DNP was synthesized, which showed vasorelaxant properties and cardiac and renal protective effects (Lisy et al., 2008; Lee et al., 2009; Ichiki et al., 2019). The synthetic CD-NP showed high resistance to NP degrading enzyme, neutral endopeptidases, making it clinically useful than endogenous naturally occurring NPs (Ichiki et al., 2019). Interestingly, it is expected that endogenous GC-A/NPRA activators and designer NPs will be powerful tools for preserving renal and cardiovascular functions and decreasing mortality among patients with cardiac events (Chen et al., 2019).
Natriuretic Peptides Levels in Humans
The cardiac hormones, ANP and BNP are released from the heart in response to atrial stretch and distension. The ANP concentration ranges at the level of 50−100-fold greater than BNP. The primary sites of synthesis and secretion of ANP and BNP is cardiac atrium. Both ANP and BNP are also synthesized in the ventricle; however, 100−1,000-fold lower than does the atrium. In cardiac disease states, the ventricle becomes the primary site of synthesis and release of BNP. A recent study showed that super-enhancer cluster controls the Nppa and Nppb promoters in a competitive mode instead in a cooperative manner (Man et al., 2021). These authors suggested that the super-enhancer cluster selectively regulates the expression of NPPA and NPPB during CVDs, resulting in an increased expression of NPPA after the NPPB region is deleted, which augments the stress-induced expression of NPRA and prevention of premature cardiac hypertrophy in human. Circulating levels of ANP and BNP are greatly elevated in the early stages of cardiac infarction (Tomoda, 1988; Phillips et al., 1989; Morita et al., 1993). The expression of ANP and BNP also increases in the atrium and ventricle during the initiation of cardiac hypertrophy and congestive heart failure (CHF) (Mukoyama et al., 1991; Volpe, 2014; Nakagawa et al., 2019). In patients with severe CHF, the levels of ANP and BNP increase more than do control levels but the BNP concentration increases to a level 10−25-fold greater than fold increased in ANP levels (Mukoyama et al., 1991). A recent study found differential regulation of ANP and BNP in patients with acute decompensated heart failure (ADHF) (Reginauld et al., 2019). These authors suggested that a deficiency of ANP might exhibit a unique characteristics of CHF. However, the exact mechanism of this type of ANF deficiency with elevated BNP levels is not yet know. Those findings suggested that the heart plays a potential compromised compensatory endocrine role with differential regulation of ANP and BNP in ANP-deficient ADHF subpopulation (Reginauld et al., 2019). Previously, it had been suggested that obese hypertensive men, despite having high BP, have lower than anticipated ANP level in the plasma (Asferg et al., 2013). Similarly, a previous study indicated that a lack of compensatory ANP elevation occurs in the advanced phase of hypertension (Macheret et al., 2012). This study also showed the prevalence of impaired synthesis and secretion of BNP, pro-BNP, and NT-pro-BNP in hypertensive patents.
ANP and BNP show similar hemodynamic and physiological responses. But because of longer half-life of BNP (12−20 min) than ANP (0.5−4), BNP exhibits extended action and leads to enhanced natriuretic and diuretic actions as compared with ANP (Yoshimura et al., 1991; Omland et al., 1996). The cardiac atrium expresses almost 50–100- fold higher ANP mRNA levels than do extra cardiac tissues (Gardner et al., 1986). Although, in normal subjects; circulating BNP levels are far less than ANP levels, the increases in BNP concentrations in plasma can be 5−10-fold higher than the fold increases in the levels of ANP in CHF patients (Mukoyama et al., 1991; Hanford et al., 1994; Hanford and Glembotski, 1996). The half-life of NT-proBNP is much longer (90−120 min) than the half-life of ANP and BNP (Weber and Hamm, 2006).
Because of the longer half-life and stability, the measurement of NT-proBNP as a diagnostic marker is preferred over measurements of BNP and ANP. Moreover, the NT-proBNP molecule is considered to be a predictive marker after cardiac transplantation (Gardner et al., 2006). In contrast, CNP does not seem to behave as a cardiac hormone and its levels in the circulation are very low (Igaki et al., 1996). CNP is largely present in the vascular endothelial cells and central nervous system (Ogawa et al., 1992; Suga et al., 1992, 1993; Tamura et al., 1996; Chen and Burnett, 1998).
Structural Domains and Signaling Mechanisms of NPs Receptors
Initially, using photoaffinity labeling and GC activity assay, three distinct types of NP receptors were identified and classified in different cell types (Pandey et al., 1988). Using molecular cloning, GC-A/NPRA was sequenced from rat brain, human placenta, and murine testis (Chinkers et al., 1989; Lowe et al., 1989; Pandey and Singh, 1990). Three distinct sub-types of NPs receptors (NPRA, NPRB, and NPRC) constitute the NPs receptor family; however, these receptors are variable in their ligand-binding specificity, GC activity, and signal transduction mechanisms (Chinkers et al., 1989; Garbers, 1992; Koller and Goddel, 1992; Khurana and Pandey, 1993). NPRC lacks the intracellular GC catalytic domain and by default, has been designated as NP clearance receptor (Maack et al., 1987; Fuller et al., 1988). Both ANP and BNP selectively activate NPRA, whereas CNP primarily binds to NPRB; all three NPs show binding affinity to NPRC (Koller et al., 1991; Suga et al., 1992; Khurana and Pandey, 1993). ANP binding to its receptor in vivo probably exerts a chloride-dependent feedback-control on receptor function (Misono, 2000; Misono et al., 2005). The general structural topology of NPRA and NPRB is consistent with the GC-receptor family, containing four distinct regions: an extracellular ligand-binding domain, a single transmembrane spanning region, an intracellular protein kinase-like homology domain (protein-KHD), and an intracellular carboxyl-terminal GC catalytic domain. The biologically dominant form of the NP receptors is NPRA, which is distributed in several peripheral and visceral cells and tissues and mediates most of the known physiological and pathophysiological actions of ANP and BNP.
Solubilization of the extracellular domain of NPRA provided a starting point for the studies of its ligand-binding domain (Pandey and Kanungo, 1993; Huo et al., 1999). NPRA was crystalized as a dimer of two receptor molecules with a tendency to dimerize spontaneously (van den Akker et al., 2000). Based on the crystal structure of NPRC, the hormone-induced structural changes in NPRA differ from those in NPRC. Ligand-binding to NPRC causes the dimer to bend between the two subdomains of the receptor creating a clamping motion to capture the ligand (He et al., 2001). Conformational flexibility at the intra-molecular hinge region in NPRC seems to be a critical factor for the broad-specificity of this receptor protein in binding all three NPs, including ANP, BNP, and CNP (He et al., 2001). Based on crystallographic modeling, two polypeptide chains of NPRA are required to activate the functional receptor molecule (van den Akker et al., 2000). It is predicted that the transmembrane GC receptors function as homodimers and that the dimerization region of NPRA is located between the protein-KHD and GC catalytic domains, forming an amphipathic alpha helix structure (de Lean et al., 2003; Misono et al., 2005). NPRB, which is mainly localized in the brain and vascular tissues, is thought to mediate CNP-dependent actions in the endothelial cells of the vasculature and central nervous system (Schulz, 2005). NPRC consists of a large extracellular domain of 496-amino acids, a single transmembrane domain, and a very short 37-amino-acid cytoplasmic tail that contains no homology to any other known receptor domain (Fuller et al., 1988; Matsukawa et al., 1999).
After ligand binding, both NPRA and NPRC are internalized and redistributed into subcellular compartments (Rathinavelu and Isom, 1991; Koh et al., 1992; Pandey, 1992, 2005b; Mani et al., 2015; Mani and Pandey, 2019). The endocytosis and intracellular sequestration of NPRA involves a series of sequential sorting steps through which ligand-receptor complexes are eventually internalized, sequestered, redistributed, and degraded in the lysosomes. However, a population of ligand-receptor complexes recycles back to the plasma membrane, and intact ligand is released outside the cell, while the receptor is anchored on the cell surface (Pandey, 1993; Pandey et al., 2002, 2005; Mani et al., 2015; Mani and Pandey, 2019). Trafficking of NPRB occurs in a ligand-dependent manner in response to stimulation with CNP. NPRB is internalized and recycled back to the plasma membrane (Brackmann et al., 2005). Degradation of the majority of internalized ligand-receptor complexes of NPRA, NPRB, and NPRC occurs into the lysosomes. Two specific sequence motif in the carboxyl terminal domain of NPRA, namely GDAY (Gly920 –Asp921-Ala922-Tyr923) and FQQI (Phe790-Gln791-Gln792-Ile793) have been identified, which serve as consensus internalization signal motifs for endocytosis and intracellular trafficking of this receptor protein (Pandey et al., 2005; Mani et al., 2016; Mani and Pandey, 2019). However, a specific sequence motif has not yet been identified for the internalization of either NPRB or NPRC. The internalization of NPRA also involves micro-RNA and clathrin-dependent pathways (Somanna et al., 2013, 2018).
Ligand-binding seems to allosterically regulate increased specific activity of the GC catalytic domains of NPRA and NPRB, which catalyzes cGMP production (Drewett and Garbers, 1994; Pandey et al., 2000; Pandey, 2005a; Burczynska et al., 2007). ANP markedly and dose-dependent increases second messenger cGMP in target cells and tissues (Waldman et al., 1984; Pandey et al., 1985, 1988, 2002, 2005). Confocal immunofluorescence analyses have demonstrated that cGMP is continuously produced in parallel during the internalization of ligand-bound NPRA in the subcellular compartments (Mani et al., 2015, 2016; Mani and Pandey, 2019). The generation of intracellular cGMP by NPRA stimulates at least three known cGMP effector protein molecules: cGMP-dependent protein kinases (PKGs), cGMP-dependent phosphodiesterases (PDEs), and cGMP-dependent ion channels (Pfeifer et al., 1998; Kaupp and Seifert, 2002; Maurice et al., 2003; Rybalkin et al., 2003; Schlossmann et al., 2005; Reinhart et al., 2006; Pandey, 2008). The activation of cGMP-dependent effector molecules leads to a wide range of signaling mechanisms leading to physiological functions, including excretion of salt and water, vasorelaxation, antiinflammation, antifibrosis, antihypertrophic actions, and immune suppressive responses (Figure 1). Overall, the signaling mechanisms of NPs and their receptors, lead to diverse physiological and pathophysiological actions, which together provide the protection to CVDs.
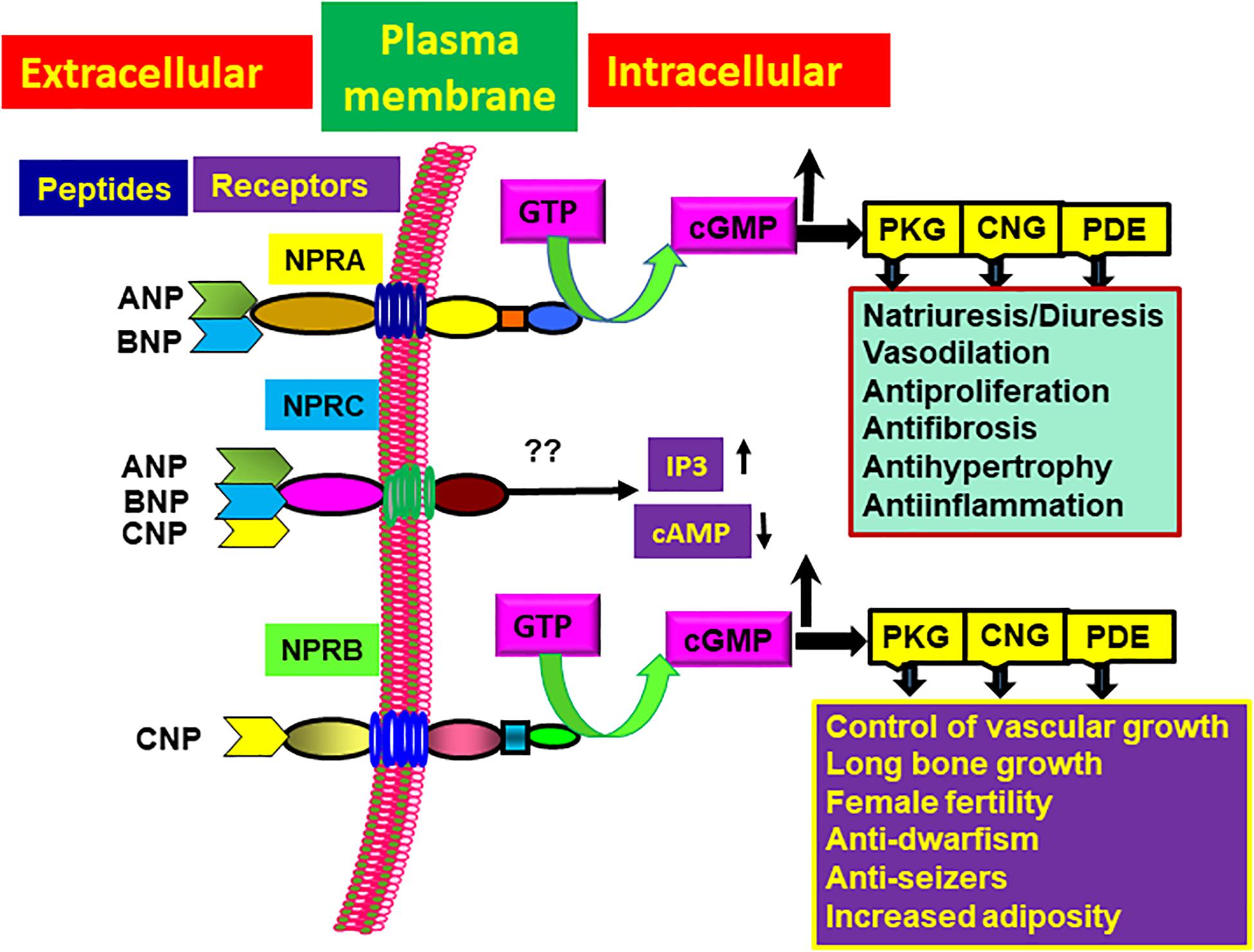
Figure 1. Ligand-binding specificity of different NPs (ANP, BNP, and CNP) with their cognate receptors (NPRA, NPRB, NPRC) and intracellular signaling mechanisms leading to the physiological responses. The diagram depicts that specific NP ligands activate their cognate receptor(s) and generate intracellular signals. The extracellular ligand binding domain (LBD) and intracellular protein kinase-like homology domain (protein-KHD) and guanylyl cyclase (GC) catalytic domain of GC-A/NPRA and GC-B/NPRB are shown. Both NPRA and NPRB produce intracellular second messenger cGMP, which activates effector molecules, including cGMP-dependent protein kinase (PKG), gated ion channels (CNG), and phosphodiesterase (PDE) to produce specific biological and physiological responsiveness.
NPs and Their Receptors in Cardiovascular Disorders
Several lines of evidence suggest that from the perspective of pathophysiology, a characteristic hallmark of CVDs is the elevation of ANP and BNP concentrations in patients with chest pain and cardiovascular dysfunction (Burnett et al., 1986; Maisel et al., 2002; Richards and Troughton, 2004; Reginauld et al., 2019). The elevation of ANP and BNP concentrations in CHF seem to be serve as a beneficial compensatory responsiveness that offset cardiac events (Stevens et al., 1995; Wang et al., 2014). However, the emerging paradigm in hypertension and CVDs is the existence of ANP and BNP deficiency with high BP and CVDs. From the point of view of the roles of ANP, BNP, and their cognate receptor NPRA in hypertension and CVDs, this section has been classified in the follow categories:
(a) Hypertension and Cardiovascular Diseases
(b) Renovascular Dysfunction
(c) Cardiac remodeling and dysfunction
(d) Metabolic Syndrome and Diabetic Conditions
(e) Immunogenic Responses and Cardiovascular Disorders
(a) Hypertension and Cardiovascular Diseases
The activation of ANP-BNP/NPRA signaling lowers fluid volume and BP by causing excretion of salt and water from the kidneys and inhibition of the vasoconstriction of vascular smooth muscle cells. The mutations in genes encoding ANP, BNP, and NPRA seems to exert deleterious effects and trigger high BP and CVDs (Ellmers et al., 2007; Pandey, 2008, 2018; Kishimoto et al., 2011). Several studies have demonstrated a link of ANP (NPPA), BNP (NPPB), and NPRA (NPR1) polymorphisms with hypertension and CVDs in humans (Rubattu et al., 2006; Webber and Marder, 2008; Xue et al., 2008; Newton-Cheh et al., 2009; Vandenwijngaert et al., 2019). In mice, the gene-knockout phenotypes of Nppa, Nppb, Nppc, Npr1, Npr2, and Npr3 are provided in Table 1.
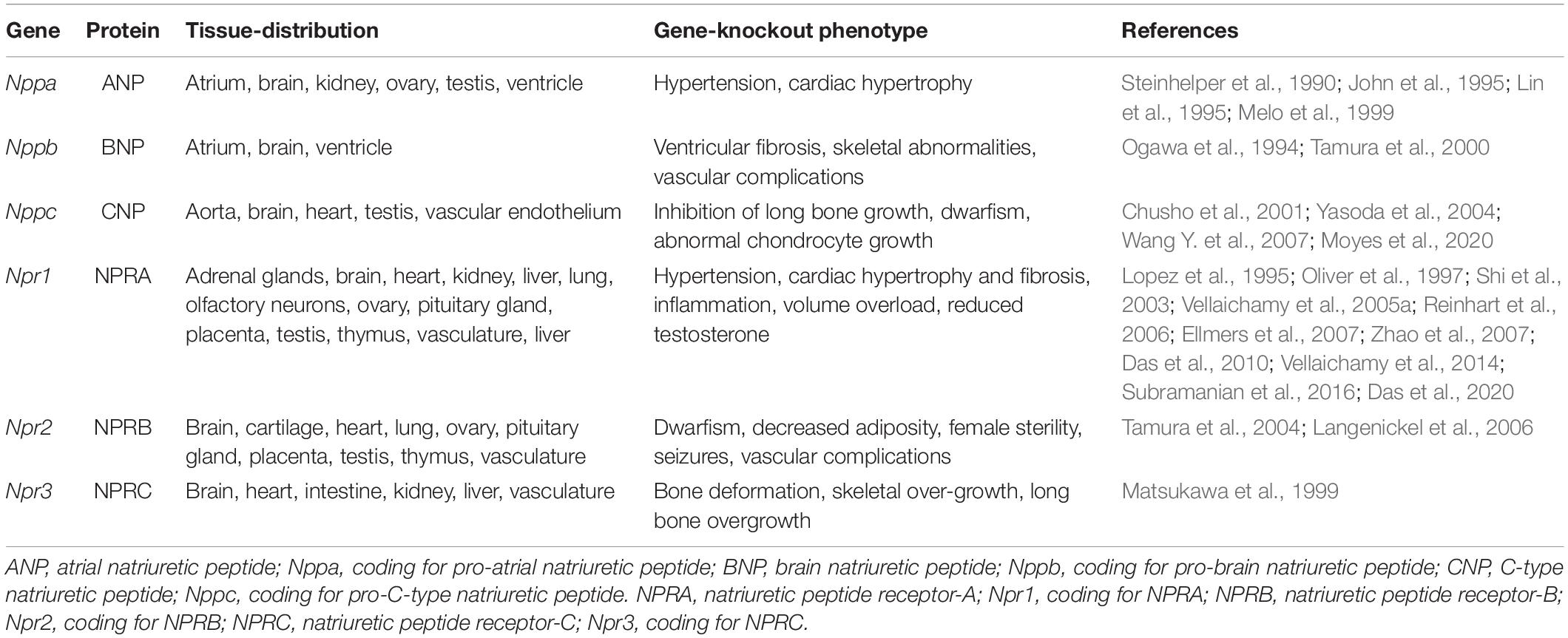
Table 1. Tissue distribution of natriuretic peptides (ANP, BNP, and CNP) along with their cognate receptors (NPRA, NPRB, and NPRC) and gene-knockout phenotypes in mice.
Genetically modified mouse models have helped to delineate the functions of ANP, BNP, and NPRA in the physiological and pathophysiological conditions of high BP and CVDs (John et al., 1995; Oliver et al., 1997; Shi et al., 2003; Vellaichamy et al., 2005a; Ellmers et al., 2007; Pandey, 2019). Transgenic mice overexpressing ANP developed sustained hypotension; also their mean arterial pressure (MAP) was decreased by 25−30 mmHg compared with the non-transgenic control groups (Steinhelper et al., 1990; Melo et al., 1999). Somatic delivery of the proANP cDNA to a spontaneously hypertensive rat (SHR) model induced a sustained reduction in systolic BP (Lin et al., 1995). Similarly, overexpression of proANP in hypertensive mice lowered systolic BP, suggesting that the proANP is an ideal gene-therapy candidate for the treatment of human hypertension and CVDs (Schillinger et al., 2005).
Several cardiovascular pathways, including NPs, RAAS, and the adrenergic systems, are considered to regulate BP and cardiovascular events. However the genetic determinants contributing to inter-individual differences in BP regulation have been linked with only the NPs and their receptors (Newton-Cheh et al., 2009). Previously, it was indicated that the polymorphism in the NPPA caused left ventricular cardiac hypertrophy in the Italian patients suffering from high BP and these patients also showed significantly reduced levels of proANP (Rubattu et al., 2006). The relationship between BP and cardiovascular disorders indicated that in the absence of ANP-BNP/NPRA signaling, even small increases in BP had excessive and detrimental effects. Mutation of a single allele in the human NPR1 was found to decrease levels of NPRA protein by 70% and showed susceptibility to BP, kidney dysfunction, and LVH (Nakayama et al., 2000). The single allele mutation in NPR1 in human could reflect parallel characteristics in the haplotype genotype of Npr1/ in mice (Shi et al., 2003; Vellaichamy et al., 2005a; Kumar et al., 2017). It has been reported that a positive association exists between the polymorphisms of NPPA, NPPB, and NPR1 causing essential hypertension and LHV (Newton-Cheh et al., 2009; Vandenwijngaert et al., 2019). Those previous studies indicated that genetic polymorphisms in NPPA, NPPB, and NPR1 seem to be linked with a family history of high BP, LVH, cardiac mass index, and paraventricular septal wall thickness. More studies will be required to characterize the functionally significant markers of NPPA, NPPB, and NPR1 polymorphism variants in a larger human population.
Global ablation of Nppa in mice can increase BP and cause hypertension (John et al., 1995). The genetic mouse model with ablation of Nppa, has suggested that ANP has a central role in hypertension. In Nppa null mutant (Nppa/) mice fed standard or intermediate-salt diets, BP was elevated by 8−10 mmHg. Haplotype (Nppa/) mice on a standard-salt diet contained a normal amount of plasma ANP and had normal BP, while on a high-salt diet these animals were hypertensive, and their BP was elevated by 25−27 mmHg. Global deletion of the Nppa allele can lead to salt-sensitive hypertension even when plasma ANP level are not significantly decreased. Global ablation of Npr1 increases BP by 36−40 mmHg in null mutant (Npr1/; 0-copy) mice as compared with wild-type (Npr1/; 2-copy) mice (Oliver et al., 1997; Shi et al., 2001, 2003; Das et al., 2010, 2020; Vellaichamy et al., 2014). In contrast, increased expression of global Npr1 in gene-duplicated (Npr1/; 3-copy and Npr1/; 4-copy) mice significantly reduced BP and enhanced kidney and heart function (Oliver et al., 1998; Shi et al., 2003; Zhao et al., 2013; Das et al., 2020).
Earlier, we examined the mechanisms that may mediate the function of increasing numbers of Npr1 gene copies, determining the excretion of urine and sodium, renal blood flow (RBF), glomerular filtration rate (GFR), and BP after blood volume expansion in Npr1 0-copy, 2-copy, and 4-copy mice in a gene-dose-dependent manner (Shi et al., 2003). The volume expansion with whole blood infusion, increased MAP in Npr1 gene-knockout (0-copy), wild-type (2-copy), and gene-duplicated (4-copy) mice. In addition, Npr1 null mutant (0-copy) mice retained significantly higher levels of Na+ and water; however, gene-duplicated (4-copy) mice as compared to wild-type (2-copy) mice had greatly reduced levels of Na+ and water. Our findings demonstrated that the ANP/NPRA axis is predominantly responsible for regulating the renal hemodynamics and Na+ excretory responses to intravascular blood volume expansion.
(b) Renovascular Dysfunction
ANP-BNP/NPRA system primarily affects glomerulus, tubular, and vascular functions in the kidneys (Nonguchi et al., 1987; Kremer et al., 1988; Light et al., 1989; Appel, 1990; Cermak et al., 1996; Kumar et al., 1997b, 2014; Pandey et al., 2000; Tripathi and Pandey, 2012; Das et al., 2020). ANP prevents contraction of mesangial cell and vascular smooth muscle cells in response to vasoconstrictors, including Ang II, endothelin, vasopressin, and agonists of the adrenergic systems (Appel, 1990; Kumar et al., 1997a; Levin et al., 1998; Pandey, 2018). NPRA antagonists, A71915 and HS-121-1 abolished the renal effect of infused ANP, including a decrease in urinary cGMP (von Geldern et al., 1990; Sano et al., 1992). In the kidneys, a combination of hemodynamic and tubular transport effects seem to be responsible for ANP-BNP/NPRA-induced natriuresis and diuresis, exerting both direct and indirect effects on tubules, including the proximal tubules, as well as cortical and innermedullary collecting ducts (Sonnenberg et al., 1986; Brenner et al., 1990; Zhao et al., 2010; Prieto et al., 2012). ANP actions facilitate the excretion of salt and water with an increase in GFR and RBF in the kidneys (Burnett et al., 1984; Camarago et al., 1984; Freeman et al., 1985; Meyer and Forsmann, 1997; Villrreal and Freeman, 1997; Melo et al., 2000; Shi et al., 2003; Pandey, 2008; Zhao et al., 2010). ANP- and BNP-induced natriuresis and diuresis causes a direct inhibition of tubular transport processes. This occurs without dependence on alterations in GFR and RBF, which favor both hemodynamic and tubular effects (Sonnenberg et al., 1986; Meyer et al., 1997). The natriuretic and diuretic actions of ANP and BNP lead to the direct inhibition of tubular transport mechanisms. It has been proposed that ANP-BNP/NPRA system also interacts with another local natriuretic system, by enhancing the action of renal dopaminergic system (Choi et al., 2014). These authors suggested that part of the inhibitory effects of ANP on sodium and water reabsorption depends on dopaminergic system. Those previous studies also indicated that ANP/NPRA signaling stimulates dopamine uptake in tubular cells and also reduces the dopamine catabolism. Similarly, urodialatin also seem to exert similar effect on renal dopaminergic system (Citarella et al., 2009; Choi et al., 2013). Confocal immunofluorescence microscopic studies have indicated that the epithelial sodium channel (ENaC) is regulated by ANP/NPRA-dependent second messenger cGMP in Xenopus 2F3 cells (Guo et al., 2013). The authors of those previous studies suggested that NPRA, but not NPRB or NPRC, is involved in the regulation of ENaC activity. Further, with the increasing concentrations of intracellular cGMP, ENaC activity was inhibited in epithelial cells from patients with cystic fibrosis (Poschet et al., 2007).
The ANP-BNP/NPRA signaling cascade plays critical roles to counteract both systemic and local RAAS (Meyer and Forsmann, 1997; Shi et al., 2001; Pandey, 2005a, 2011; Vellaichamy et al., 2007). ANP/NPRA markedly lowers renin secretion from the kidneys and also reduces plasma renin concentrations (Burnett et al., 1984; Obana et al., 1985; Paul et al., 1988; Meyer and Forsmann, 1997; Shi et al., 2003). Further, using genetically modified mouse models, ANP/NPRA signaling has been shown to suppress renin activity and other RAAS components as well as to decrease BP (Obana et al., 1985; Brenner et al., 1990; Levin et al., 1998; Shi et al., 2001; Pandey, 2005a; Vellaichamy et al., 2007; Periyasamy et al., 2019).
Studies from our laboratory using Npr1–/– null mutant mice have demonstrated that at the birth, the absence of NPRA allows increased renin mRNA expression and greater renin and Ang II levels than occur in Npr1–/– wild-type mice (Shi et al., 2001). On the contrary, in adult Npr1–/– mice, both circulating and intrarenal concentrations of renin and Ang II levels were significantly lower than those in wild-type control animals. The decrease in renin concentrations in adult Npr1–/– mice was found to be due to progressive increases in BP, which lead to inhibition of renin synthesis and secretion from juxtaglomerular cells in the kidney. It has been suggested that an increased levels of ANP released into the circulation in response to blood volume expansion, was mainly responsible for the natriuretic and diuretic actions (Paul et al., 1988; Antunes-Rodrigues et al., 1992). We have examined the quantitative contributions and possible mechanisms mediating renin synthesis and release using the varying Npr1 gene copy of Npr1–/– (0-copy), Npr1+/+ (2-copy), and Npr1++/++ (4-copy) mice in a gene-dose-dependent manner (Shi et al., 2003). Our studies have demonstrated that NPRA ablation increases the expression of AT1R and angiotensin-converting enzyme 1 (ACE 1) in the kidneys of Npr1–/– null mutant mice (Periyasamy et al., 2019).
ANP-BNP/NPRA signaling inhibits the synthesis and secretion of aldosterone in adrenal glomerulosa cells suggesting that ANP is important for natriuretic and diuretic responses (Atarashi et al., 1984; Brenner et al., 1990; Anand-Srivastava and Trachte, 1993; Pandey, 2005a). The NPRA antagonist HS-142-1 was found to eliminated the suppressive effects of ANP on aldosterone synthesis and secretion in adrenal glomerulosa cells (Oda et al., 1992). The ANP/NPRA system increases cGMP concentrations, resulting in an activation of cAMP-dependent phosphodiesterases, which decreases both cAMP and aldosterone levels in adrenal glomerulosa cells (MacFarland et al., 1991; Levin et al., 1998; Pandey, 2005a).
Interestingly, adrenal renin content and renin mRNA along with Ang II and aldosterone levels were found to be increased in the adult Npr1–/– mice than with Npr1+/+ control animals (Shi et al., 2001; Zhao et al., 2007). ANP-BNP/NPRA signaling opposes all the actions of Ang II in the phathophysioogical states (Pandey, 2005a, 2010). Our studies demonstrated that ablation of Npr1 exhibits in chronic elevation of BP in mice fed a high-salt diet (Oliver et al., 1998; Zhao et al., 2007, 2013). On the other hand, adrenal Ang II and aldosterone concentrations were decreased in Npr1 gene-duplicated animals kept on the high-salt diet; however, a low-salt diet increased the adrenal Ang II and aldosterone concentrations in Npr1 mice in a gene-dose-dependent manner (Zhao et al., 2007, 2013). Previously, it was indicated that BP of Npr1–/– mice remained at higher levels and unchanged in response to high-salt diets (Lopez et al., 1995). Our findings indicated that NPRA signaling is protective against the effects of a high-salt diet in the kidneys and heart of Npr1 gene-duplicated mice (Zhao et al., 2007, 2013).
(c) Cardiac Remodeling and Dysfunction
Plasma ANP and BNP levels are markedly elevated in the pathophysiological and clinical conditions of cardiac dysfunction and remodeling, including fibrosis, diastolic dysfunction, cardiac hypertrophy, pulmonary embolism, and CHF leading to severe conditions of CVDs (Vellaichamy et al., 2005a, 2007; Felker et al., 2006; Jaffe et al., 2006; Reinhart et al., 2006; See and de Lemos, 2006; Ellmers et al., 2007; Zhao et al., 2013; Rubattu and Volpe, 2014; Pandey, 2019). ANP and BNP exert their cardioprotective functions not only as the circulating peptide hormones but also as local autocrine and paracrine factors (Chen et al., 2019; Pandey, 2019). ANP-BNP/NPRA signaling serves cardiac protective role by various mechanisms (Table 2). The concentrations of ANP and BNP are increased proportion to the severity of cardiac dysfunction and remolding in humans and experimental animal models (Nakao et al., 1996; Chen and Burnett, 1999; Reinhart et al., 2006; Ellmers et al., 2007; Pandey, 2008; Rubattu et al., 2019). The concentrations of ANP and BNP are also markedly elevated in the cardiac tissues of patients with CHF. In humans, both of these hormones appear to reduce the preload and afterload of the heart. The high plasma levels of ANP and BNP tend to predict cardiac events and CHF but BNP levels rise 10−25-fold higher than the fold increases in ANP concentrations (Mukoyama et al., 1991; Tsutamoto et al., 1993; Chen and Burnett, 1999; Felker et al., 2006; Reinhart et al., 2006; Pandey, 2019; Volpe et al., 2019a). NPPA, NPPB, and NPR1 genes are overexpressed in hypertrophied failing heart, suggesting that the autocrine and/or paracrine effects of NPs predominate and acts endogenously to protect against maladaptive pathology of CVDs (Knowles et al., 2001; Vellaichamy et al., 2005a, 2007, 2014; Felker et al., 2006; Ellmers et al., 2007; Xue et al., 2008; Subramanian et al., 2016; Pandey, 2019; Volpe et al., 2019a, b).
The longer half-life of BNP has favored its diagnostic use over that of ANP to evaluate NPs as important indicator of CHF in patents with chest pain in emergency conditions (Reinhart et al., 2006; Rubattu and Volpe, 2014). The ventricular expression of Nppa, Nppb, and Npr1 is more closely associated with local cardiac hypertrophic and fibrotic events than are plasma ANP levels and systemic BP (Vellaichamy et al., 2005a, 2007). Nevertheless, NT-pro-BNP seems to be a stronger predictor of cardiovascular risk (Doust et al., 2005; Khan et al., 2006; Girsen et al., 2007; Kocylowski et al., 2009). In hypertrophied and failing hearts, the expression of Nppa and Nppb, which serve as endogenous protective mechanisms against maladaptive cardiac events and dysfunction is markedly increased (Knowles et al., 2001; Zahabi et al., 2003; Vellaichamy et al., 2005a; Pandey, 2019; Rubattu et al., 2019). The alterations in Nppa promoter seem to be linked with cardiac hypertrophy and dysfunction (Deschepper et al., 2001). Mice lacking NPRA develop cardiac hypertrophy and fibrosis, independent of BP (Oliver et al., 1997; Nakanishi et al., 2005; Vellaichamy et al., 2005a, 2007, 2014; Ellmers et al., 2007; Scott et al., 2009; Zhao et al., 2013). Our studies have demonstrated that the global ablation of Npr1 in mice triggers the expression of hypertrophic and fibrotic markers and matrix metalloproteinases (MMP-2, MMP-9) in the cardiac tissues (Vellaichamy et al., 2005a, 2007, 2014; Pandey, 2008, 2011; Subramanian et al., 2016). It has been indicated that ANP/NPRA signaling antagonizes Ang II-induced collagen systhesis via suppression and activation of MMP-2, MMP-9 (Parthasarathy et al., 2013).
The expression of Ca2+-ATPase-2a (SERCA-2a) is progressively decreased and the level of cytosolic Ca2+ is increased in the hypertrophied heart of Npr1–/– mice (Vellaichamy et al., 2005a; Pandey and Vellaichamy, 2010). ANP-BNP/NPRA signaling mechanism is known to decrease the cytosolic levels of Ca2+ and inosital trisphosphate in different cells and tissues (Pandey, 2014). Further, both ACE 1 and AT1R were found to be significantly increased leading to cardiac hypertrophy and fibrosis in Npr1–/– mice, but not Npr1+/+ control mice (Vellaichamy et al., 2007). In failing hypertrophied hearts, ANP-BNP/NPRA signaling antagonizes Ang II- and AT1R receptor-mediated cardiac dysfuction and remodeling (Li et al., 2002; Kilic et al., 2007; Vellaichamy et al., 2007; Zhao et al., 2013). The endothelial cell-specific arteries from Npr1 gene-disrupted mice showed significant elevaion in systolic BP (Sabrane et al., 2005). The conditional inactivation of Npr1 in cardiac myocytes, exhibited mild cardiac hypertrophy; however, ANP levels were markedly increased (Holtwick et al., 2003). Both ANP and BNP levels were elevated in the global Npr1–/– and haplotype Npr1+/– mice with myocardial infarction. These mice showed a higher incidence of CHF and significantly greater mortality rates than did wild-type mice (Nakanishi et al., 2005; Vellaichamy et al., 2005a, 2007). ANP-BNP/NPRA signaling provides cardiac protective mechanism against maladaptive cardiac disorders and remodeling of CVDs (Figure 2).
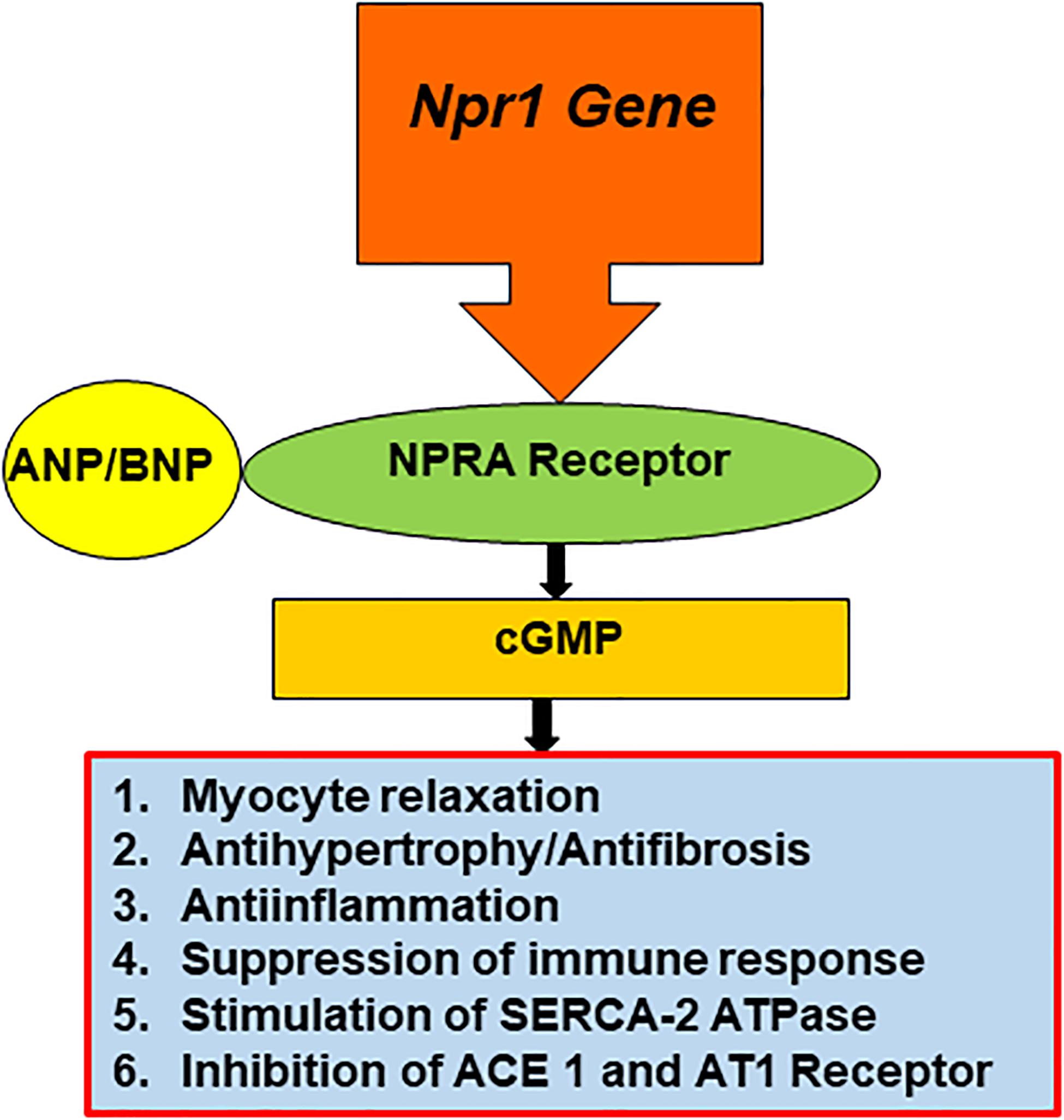
Figure 2. The schematic representation of the NP-dependent activation and signaling of ANP/NPRA in the heart. To maintain the cardiac homeostasis, Npr1 gene is transcribed and translated to produce mature NPRA protein. Specifically, NPRA is activated by ANP and BNP; in turn produces intracellular second messenger cGMP that activates various physiological responsiveness in the heart tissues and cells.
(d) Metabolic Syndrome and Diabetic Conditions
The growing evidence suggest that ANP-BNP/NPRA signaling regulates whole body metabolism and controls diabetic conditions (Wang et al., 2004; Wang T.J. et al., 2007; Moro, 2013; Coue et al., 2015). The previous studies have indicated that a decreased plasma levels of ANP might be associated with the obesity, metabolic syndrome, insulin resistance, energy balance, and glucose homeostasis in humans (Wang et al., 2004; Wang T.J. et al., 2007; Birkenfeld et al., 2008; Coue and Moro, 2016; Cannone et al., 2019). It has also been shown that NPs signaling activates the peroxisome proliferator-activated receptor-γ (PPAR-γ) and enhances the fat oxidation and mitochondrial biogenesis in the muscle tissues (Mitsuishi et al., 2008; Miyashita et al., 2009). The ANP/NPRA signaling also enhances the PPAR-γ coactivator-1α (PGC-1α), which stimulates oxidative phosphorylation (OXPHOS) in skeletal muscle tissues (Engeli et al., 2012). The previous studies have demonstrated that ANP/NPRA system accelerates lipid mobilization, mitochondrial oxidative metabolism, and fat oxidation (Birkenfeld et al., 2008, 2012; Tsukamoto et al., 2009). In fact, ANP/NPRA action modulates energy expenditure and supply of fatty acids for cardiac and skeletal muscle metabolic utilization (Engeli et al., 2012; Schlueter et al., 2014).
Furthermore, ANP/NPRA signaling seems to regulate obesity, type 2 diabetes, and resistance to insulin (Moro, 2013; Coue et al., 2015). On the other hand, previous studies have indicated that NPRC exhibits lipolytic effect of NPs in mouse adipose tissues (Sengenes et al., 2002; Bordicchia et al., 2012). Mice challenged to the low temperature environment showed stimulated release of ANP, but exhibited a reduced level of NPRC in both brown and white adipocytes (Bordicchia et al., 2012). Interestingly, ANP has been shown to enhance the browning of human white adipose tissues (Miyashita et al., 2009; Enerback, 2010). It has been suggested that insulin enhances the expression of NPRC in adipocytes in a glucose-dependent manner (Bordicchia et al., 2016). It is believed that a defective ANP-BNP/NPRA signaling might promote a maladaptive metabolic disorders, which could trigger lipid accumulation, decreased mitochondrial function, hyperglycemia, and insulin resistance leading to hypertension and CVDs in humans. It is believed that an increased circulating concentrations of ANP might serve as protective mechanisms, nevertheless, the human subjects having low circulating levels of ANP might have a greater risk of cardiometabolic syndrome (Pereira et al., 2015).
(e) Immunogenic Responses and Cardiovascular Disorders
Proinflammatory cytokines play a central role in the development of hypertension, cardiac hypertrophy, and CHF in experimental animal models and in humans (Hirota et al., 1995; Testa et al., 1996; Vanderheyden et al., 2005; Vellaichamy et al., 2005a, 2014; Das et al., 2010, 2020; Subramanian et al., 2016; Gogulamudi et al., 2019). Elevated circulating and myocardial levels of proinflammatory cytokines, including tumor necrosis factor (TNF-α), interleukin-1β (IL-1β), and interleukin-6 (IL-6) have been reported in the plasma of cardiomyopathic patients, correlating with the severity of their disease (Testa et al., 1996; Vanderheyden et al., 2005). Myocardial cells are capable of producing substantial amounts of proinflammatory cytokines in response to ischemia and experimental load-induced stress (Baumgarten et al., 2002; Palmieri et al., 2002). Inappropriate activation of TNF-α, IL-1β, and IL-6 has been shown to induce phenotypic changes in CVDs, encompassing the myocyte hypertrophy, myocardial apoptosis, extracellular matrix deposition, and contractile dysfunction (Thaik et al., 1995; Sekiguchi et al., 2004; Vellaichamy et al., 2005a, 2014; Subramanian et al., 2016).
Investigations in our laboratory have indicated that the ANP-BNP/NPRA system acts as a negative regulator of inflammation and hypertrophic growth in the kidneys and heart (Vellaichamy et al., 2005a, b, 2007, 2014; Das et al., 2010, 2020; Subramanian et al., 2016; Kumar et al., 2017). ANP inhibited TNF-α production in interferon-gamma (IFN-γ)-activated macrophages and blocked TNF-α-induced adhesion molecule expression in endothelial cells (Tsukagoshi et al., 2001; Vollmer, 2005). We have used Npr1 gene-knockout and gene-duplicated mouse models in efforts to determine whether genetically determined differences in Npr1 expression changes the levels of cardiac proinflammatory cytokines (Zhao et al., 2013; Vellaichamy et al., 2014; Subramanian et al., 2016). Our findings suggested that ablation of Npr1 triggered a sustained activation of proinflammatory cytokines gene expression and protein levels associated with exaggerated ventricular remodeling and cardiac hypertrophy leading to CVDs. However, gene-duplication of Npr1 in mice attenuated cardiac proinflammatory cytokines expression and protected against cardiac remodeling (Vellaichamy et al., 2005a, 2007, 2014; Subramanian et al., 2016).
Increased expression of TNF-α, IL-6, TGF-β, LT-β, and IFN-γ mRNA and protein levels were found in the hearts of adult Npr1–/– mice as compared with the hearts of adult Npr1+/+ mice (Vellaichamy et al., 2014). In parallel, cytokine receptor protein levels were also increased in the heart of null mutant mice indicating increased expression of gb-130, TNF-α receptor 1 (TNF-αR1), and TNF-β receptor 1 (TGF-βR1). In contrast, significant decreases in IL-6, TNF-α, and TGF-β1 cytokine protein levels were found in the gene-duplicated hearts of Npr1++/++ mice as compared with the hearts of wild-type mice.
The activated ANP-BNP/NPRA system may serve a protective function by inhibiting the ventricular expression of proinflammatory cytokines in CVDs (Vellaichamy et al., 2005a, b, 2014). Some previous observations advanced the notion that disruption of the ANP/NPRA/cGMP signaling pathway can augment activation of proinflammatory cytokines leading to extracellular matrix remodeling, pathological hypertrophy, and CHF. Expression of myocardial proinflammatory cytokine genes, including TNF-α, IL-1β, and IL-6 was found to be significantly higher in patients with compensated CHF condition, along with increased cytokine gene expression, which could play an adaptive role in left ventricular remodeling and other CVDs (Oral et al., 2003; Vanderheyden et al., 2005). It has been shown that cardiac-specific over expression of proinflammatory cytokines in mouse hearts leads to cardiac hypertrophy and ventricular disorders similar to those in human heart disease, indicating that proinflammatory cytokines are critically involved in the cardiac remodeling process in CVDs (Hirota et al., 1995; Krown et al., 1996; Oral et al., 2003; Vellaichamy et al., 2005a, 2014; Subramanian et al., 2016). Blockade of the action of proinflammatory cytokines has been shown to prevent hypertension, cardiac hypertrophy, and diastolic dysfunction in experimental animal models (Villarreal and Dillmann, 1992; Krown et al., 1996; Isono et al., 1998; Kurrelmeyer et al., 2000; Li et al., 2000; Vellaichamy et al., 2005a, 2007).
Evidence suggests that the ANP-BNP/NPRA system has antiinflammatory activity, and could inhibit the bacterial toxin (LPS)- and IFN- gamma- induced expression of proinflammatory cytokines and nuclear factor-kappa B (NF-κB) in macrophages (Tsukagoshi et al., 2001; Vollmer, 2005). Ablation of Npr1 also enhances the expression and activation of transcription factors, NF-κB and activating protein-1 (AP-1), which seem to be associated with cardiac hypertrophy, fibrosis, and extracellular matrix remodeling (Vellaichamy et al., 2005a, 2007, 2014). Npr1 gene-disruption activates NF-κB and leading to cardiac remodeling and hypertrophic conditions (Figure 3). A significant increases in mRNA expression and protein levels of NF-κB and IKK-β isoforms were observed in the hearts and kidneys of Npr1–/– and Npr1+/– mice as compared with Npr1+/+mice, suggesting that the NF-κB signaling pathway is activated in the hearts and kidneys of mutant mice (Vellaichamy et al., 2005a, 2014; Subramanian et al., 2016). The increased NF-κB binding activity was positively correlated with increased expression of proinflammatory cytokine genes in Npr1 null mutant mice with initiation and development of CVDs (Vellaichamy et al., 2005a, 2014; Subramanian et al., 2016).
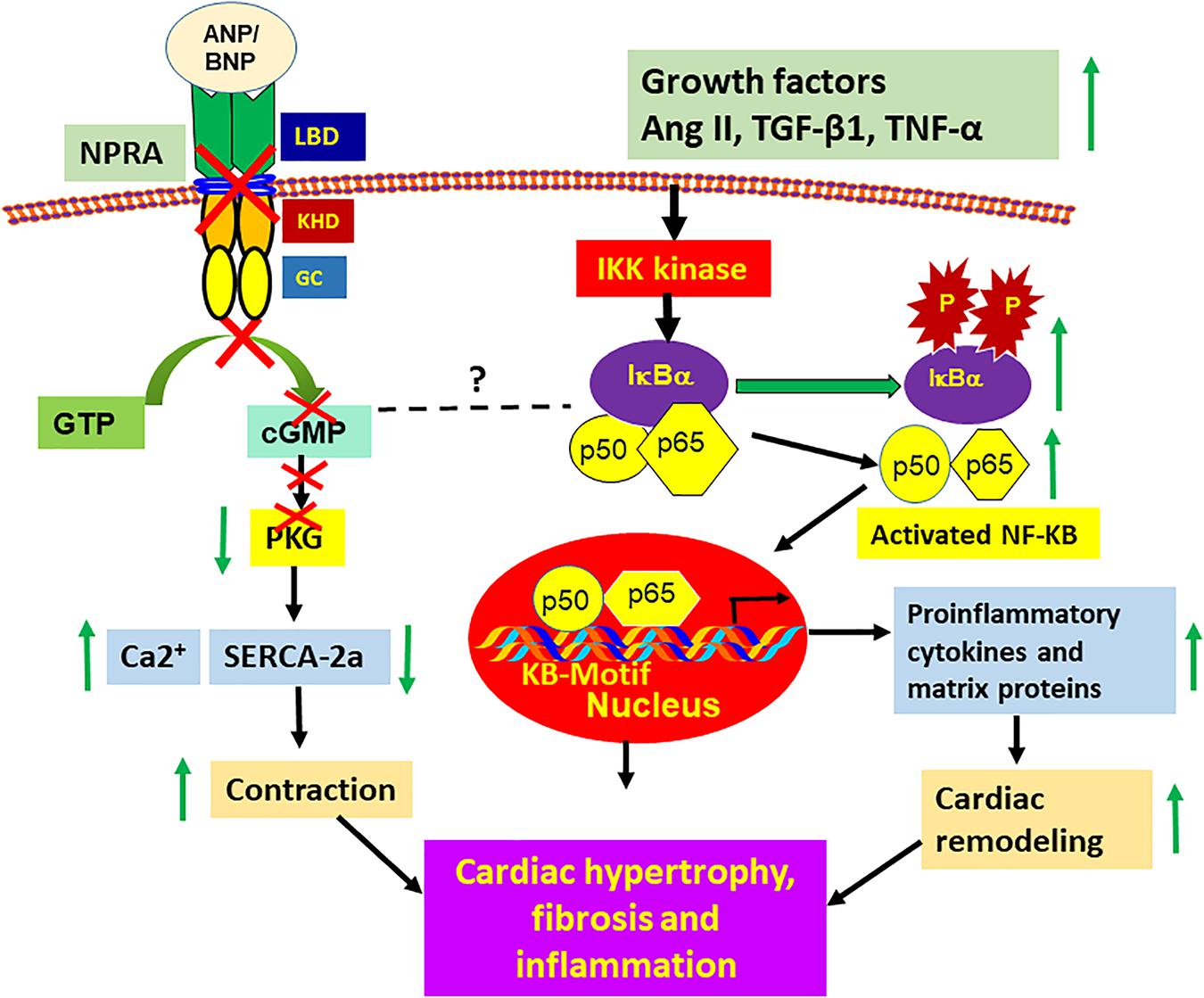
Figure 3. Ablation of Npr1 triggers the activation of proinflammatory pathways that promotes cardiac hypertrophy, fibrosis, and remodeling. Disruption of ANP/NPRA signaling causes unbalanced activation of master transcription factor NF-κB that initiates the expression of proinflammatory cytokines and matrix proteins; thereby promotes specific cardiac structural and molecular changes in Npr1–/– mice heart. The activated NF-κB translocates into the nucleus and promotes the genes transcription and expression of various proinflammatory cytokines and extracellular matrix proteins, including MMP-2 and MMP-9. Increased activation of NF-κB, thereby promotes medial thickening and perivascular fibrosis, in turn leads to the cardiac hypertrophy, fibrosis, remodeling and heart failure. On the other hand, the absence of ANP/NPRA signaling also simultaneously inhibits SERCA-2a that increases the cytosolic Ca2+ levels, thereby results in the increased contractile activity of the heart, which also promotes cardiac hypertrophy and remodeling. Ang II, angiotensin II; TGF-β1, transforming growth factor-β1; TNF-α, tumor necrosis factor-α; IKK, inhibitory kappa kinase; IKB, inhibitory kappa B, NF-κB, nuclear factor-kappa B; PKG, cGMP-dependent protein kinase; SERCA-2a, sarcolemmal endoplasmic reticulum Ca2+-ATPase-2a; MMP-2 and -9, matrix metalloproteinase-2 and −9.
Previous studies indicated that the activation of NF-κB and proinflammatory cytokines serve as a causal event in the development of cardiac hypertrophy and fibrosis (Frantz et al., 2003; Purcell and Molkentin, 2003; Li et al., 2004; Vellaichamy et al., 2005a; Subramanian et al., 2016). The sustained activation of proinflammatory cytokines contributes to the pathological forms of ventricular remodeling and exaggerating the CVDs in experimental animals (Leitman et al., 1988; Hirota et al., 1995; Baumgarten et al., 2002; Palmieri et al., 2002; Vellaichamy et al., 2005a, 2014; Zhao et al., 2013). Our findings suggested that enhanced ANP-BNP/NPRA signaling can protect the heart by inhibiting ventricular expression of NF-κB, a master regulator of proinflammatory cytokines in relation to increasing Npr1 gene copies (Vellaichamy et al., 2014; Subramanian et al., 2016).
Perspectives
In the past four decades, a large body of research has provided a unique perspective on the biochemical, cellular, molecular, genetic, and clinical aspects of NPs and their receptors in relation to CVDs. The physiological and pathophysiological roles of ANP-BNP/NPRA signaling are implicated with protective mechanisms in various organ systems, including the heart, kidneys, lungs, central nervous system, gonads, adrenal glands, and vasculature. Cardiac hormones, ANP and BNP are considered to be diagnostic markers of CHF, but we need to determine their therapeutic potentials for the treatment and prevention of CVDs such as hypertension, renal insufficiency, cardiac hypertrophy, CHF, and stroke. Intraperitoneal delivery of recombinant ANP (Carperitide) facilitated the recovery of increased blood flow in ischemic conditions and exerted antihypertrophic and antifibrotic effects (Suwa et al., 2005; Park et al., 2008; Nishikimi et al., 2013). Recombinant BNP (Nesiritide) exhibited natriuretic and vasodilatory action in CHF patients (Colucci et al., 2000). In Npr1–/– gene ablated mice, impaired recovery of blood flow after hand-limb ischemia was significantly inhibited by both ANP and BNP (Tokudome et al., 2009). Genetic molecular approaches have delineated of the functions of NPRA by decreasing and/or increasing Npr1 gene-copies (gene-knockout and/or gene-duplication) in mice by genetically altering protein product levels. It has been found that common genetic variants of NPPA, NPPB, and NPR1 are associated with circulating ANP and BNP levels and BP, contributing individual variations in the regulation of BP and CVD risk factors (Wang, 2018; Reginauld et al., 2019).
The strategy of enhancing endogenous NPs and their protective roles in CHF is achieved with the drug sacubitril/valsartan, which augments circulating ANP and BNP by inhibiting of neprilysin that degrades NPs (McMurray et al., 2014). This drug combines dual properties; inhibiting both neprilysin and Ang II type 1 receptor (AT1R) blocker, which is also referred to as Ang II receptor-neprilysin inhibitor (ARNi). Enhancing endogenous ANP-BNP/NPRA signaling has proven to be critical in the first line of therapeutic targets for hypertension, cardiac dysfunction, and CHF in decades (McMurray et al., 2014).
The apparent action of ANP-BNP/NPRA system in antagonizing the RAAS, there seem to be immense potential in using the NPs as a novel therapeutic axis in treating hypertension, renal inefficiencies, and CVDs. The clinical trials have suggested both the benefits and risks of using the synthetic ANP (anaritide and carperitide) and BNP (nesiritide) for the treatment of hypertension, renal diseases and CVDs. Unfortunately, synthetic NPs as a drug have not yet been recommended for the therapeutic treatments in the United States (Kuhn et al., 2009; Saito, 2010; Hayek and Nemer, 2011; Dohi and Ito, 2014; McMurray et al., 2014). However, the success of the drug sacubitril/valsartan in treating the CHF has heightened the enthusiasm and interest in NPs as a therapeutic target for hypertension, renal diseases, and CVDs. This drug combines an inhibitor of the NPs degrading enzyme neprilysin (sacubitril) with an antagonist of Ang II receptor 1 (AT1R) (Valsartan), which lower BP and pulse pressure (Vellaichamy et al., 2005a; Ruilope et al., 2010; Williams et al., 2017). Interestingly, the new chimeric natriuretic peptide-based therapies have a great potential in treating hypertension and CVDs. Furthermore, molecular mimicking of the action of BP-lowering Npr1 expression and receptor signaling could be effective in identifying the novel mechanisms for the new therapeutic treatment strategies.
Future studies are expected to lead a better understanding of the genetic and molecular basis of the ANP-BNP/NPRA system in regulating CVDs, including high BP, stroke, CHF, and neurological disorders. The results of future investigations of NPs and their receptors should certainly help resolve the complexities of CVDs. These future studies need to be designed to elucidate the genetic and molecular basis of Npr1 function in both normal and disease states. Both ongoing and future clinical studies will be needed to identify more functionally significant markers of NPPA, NPPB, and NPR1 variants in a larger human population. The chimeric designer peptides of ANP, BNP, and DNP have opened new avenues for studies of CHF, cardiac disorders, and remodeling therapy. The future progress in this area of research should significantly strengthen and advance our understanding of the genetic and molecular approaches used to evaluate diverse pathophysiological conditions in CVDs. We need to expand the potential clinical implications of ongoing investigations on the next generation personalized medicine and pharmacogenomics of NPs and their receptors that are currently in progress. The resulting knowledge will yield novel therapeutic targets and new treatment strategies for CVDs.
Author Contributions
KP wrote the manuscript and complied information and references.
Funding
The research works in the author’s laboratory were supported by the National Institutes of Health grant (HL-062147), Tulane Carol Levin Bernick Research award, and Tulane ByWater Fellowship grant award.
Conflict of Interest
The author declares that the research was conducted in the absence of any commercial or financial relationships that could be construed as a potential conflict of interest.
Publisher’s Note
All claims expressed in this article are solely those of the authors and do not necessarily represent those of their affiliated organizations, or those of the publisher, the editors and the reviewers. Any product that may be evaluated in this article, or claim that may be made by its manufacturer, is not guaranteed or endorsed by the publisher.
Acknowledgments
The author sincerely acknowledges past and present members of his laboratory who participated in research related to this review. The author also thanks Mrs. Kamala Pandey for her assistance in the preparation of this manuscript.
References
Abell, T. J., Richards, A. M., Ikram, H., Espiner, E. A., and Yandle, T. (1989). Atrial natriuretic factor inhibits proliferation of vascular smooth muscle cells stimulated by platelet-derived growth factor. Biochem. Biophys. Res. Commun. 160, 1392–1396. doi: 10.1016/s0006-291x(89)80158-5
Airhart, N., Yang, Y. F., Roberts, C. T. Jr., and Silberbach, M. (2003). Atrial natriuretic peptide induces natriuretic peptide receptor-cGMP-dependent protein kinase interaction. J. Biol. Chem 278, 38693–38698. doi: 10.1074/jbc.m304098200
Anand-Srivastava, M. B., and Trachte, G. J. (1993). Atrial natriuretic factor receptors and signal transduction mechanisms. Pharmacol Rev 45, 455–497.
Antunes-Rodrigues, J., Machado, B. H., Andrade, H. A., Mauad, H., Ramalho, M. J., Reis, L. C., et al. (1992). Carotid aortic and renal baroreceptors mediate the atrial natriuretic peptide release induced by blood volume expansion. Proc. Natl. Acad. Sci. U.S.A. 89, 6829–6831.
Appel, R. G. (1990). Mechanism of atrial natriuretic factor-induced inhibition of rat mesangial cell mitogenesis. Am. J. Physiol. 259, E312–E318.
Appel, R. G. (1992). Growth-regulatory properties of atrial natriuretic factor. Am. J. Physiol. 262, F911–F918.
Arise, K. K., Kumar, P., Garg, R., Samivel, R., Zhao, H., Pandya, K., et al. (2020). Angiotensin II represses Npr1 expression and receptor function by recruitment of transcription factors CREB and HSF-4a and activation of HDACs. Sci. Rep. 10:4337.
Asferg, C. L., Nielsen, S. J., Andersen, U. B., Linneberg, A., Moller, D. V., Hedley, P. L., et al. (2013). Relative atrial natriuretic peptide deficiency and inadequate renin and angiotensin II suppression in obese hypertensive men. Hypertension 62, 147–153. doi: 10.1161/hypertensionaha.111.00791
Atarashi, K., Mulrow, P. J., Franco-Saenz, R., Snajdar, R., and Rapp, J. (1984). Inhibition of aldosterone production by an atrial extract. Science 224, 992–994. doi: 10.1126/science.6326267
Baumgarten, G., Knuefermann, P., Kalra, D., Gao, F., Taffet, G. E., Michael, L., et al. (2002). Load-dependent and -independent regulation of proinflammatory cytokine and cytokine receptor gene expression in the adult mammalian heart. Circulation 105, 2192–2197. doi: 10.1161/01.cir.0000015608.37608.18
Birkenfeld, A. L., Boschmann, M., Engeli, S., Moro, C., Arafat, A. M., Luft, F. C., et al. (2012). Atrial natriuretic peptide and adiponectin interactions in man. PLoS One 7:e43238. doi: 10.1371/journal.pone.0043238
Birkenfeld, A. L., Budziarek, P., Boschmann, M., Moro, C., Adams, F., Franke, G., et al. (2008). Atrial natriuretic peptide induces postprandial lipid oxidation in humans. Diabetes 57, 3199–3204. doi: 10.2337/db08-0649
Bordicchia, M., Ceresiani, M., Pavani, M., Minardi, D., Polito, M., Wabitsch, M., et al. (2016). Insulin/glucose induces natriuretic peptide clearance receptor in human adipocytes: a metabolic link with the cardiac natriuretic pathway. Am. J. Physiol. Regul. Integr. Comp. Physiol. 311, R104–R114.
Bordicchia, M., Liu, D., Amri, E. Z., Ailhaud, G., Dessi-Fulgheri, P., Zhang, C., et al. (2012). Cardiac natriuretic peptides act via p38 MAPK to induce the brown fat thermogenic program in mouse and human adipocytes. J. Clin. Invest. 122, 1022–1036. doi: 10.1172/jci59701
Brackmann, M., Schuchmann, S., Anand, R., and Braunewell, K. H. (2005). Neuronal Ca2+ sensor protein VILIP-1 affects cGMP signalling of guanylyl cyclase B by regulating clathrin-dependent receptor recycling in hippocampal neurons. J. Cell Sci. 118, 2495–2505. doi: 10.1242/jcs.02376
Brenner, B. M., Ballermann, B. J., Gunning, M. E., and Zeidel, M. L. (1990). Diverse biological actions of atrial natriuretic peptide. Physiol. Rev. 70, 665–699. doi: 10.1152/physrev.1990.70.3.665
Burczynska, B., Duda, T., and Sharma, R. K. (2007). ATP signaling site in the ARM domain of atrial natriuretic factor receptor guanylate cyclase. Mol. Cell Biochem. 301, 93–107. doi: 10.1007/s11010-006-9400-7
Burnett, J. C. Jr., Granger, J. P., and Opgenorth, T. J. (1984). Effects of synthetic atrial natriuretic factor on renal function and renin release. Am. J. Physiol. 247, F863–F866.
Burnett, J. C. Jr., Kao, P. C., Hu, D. C., Heser, D. W., Heublein, D., Granger, J. P., et al. (1986). Atrial natriuretic peptide elevation in congestive heart failure in the human. Science 231, 1145–1147. doi: 10.1126/science.2935937
Camarago, M. J., Kleinert, H. D., Atlas, S. A., Sealey, J. E., Laragh, J. H., and Maack, T. (1984). Ca2+ -dependent hemodynamic and natriuretic effects of atrial extract in isolated rat kidney. Am. J. Physiol. 246, F447–F456.
Cannone, V., Cabassi, A., Volpi, R., and Burnett, J. C. Jr. (2019). Atrial natriuretic peptide: a molecular target of novel therapeutic approaches to cardio-metabolic disease. Int. J. Mol. Sci. 20:3265. doi: 10.3390/ijms20133265
Cermak, R., Kleta, R., Forssmann, W. G., and Schlatter, E. (1996). Natriuretic peptides increase a K+ conductance in rat mesangial cells. Pflugers Arch. 431, 571–577. doi: 10.1007/bf02191905
Charles, C. J., Espiner, E. A., Richards, A. M., Nicholls, M. G., and Yandle, T. G. (1995). Biological actions and pharmacokinetics of C-type natriuretic peptide in conscious sheep. Am. J. Physiol. 268, R201–R207.
Chen, H. H., and Burnett, J. C. Jr. (1998). C-type natriuretic peptide: the endothelial component of the natriuretic peptide system. J. Cardiovasc. Pharmacol. 32(Suppl. 3), S22–S28.
Chen, H. H., and Burnett, J. C. Jr. (1999). The natriuretic peptides in heart failure: diagnostic and therapeutic potentials. Proc. Assoc. Am. Physicians 111, 406–416. doi: 10.1111/paa.1999.111.5.406
Chen, Y., Harty, G. J., Zheng, Y., Iyer, S. R., Sugihara, S., Sangaralingham, S. J., et al. (2019). CRRL269: a novel particulate guanylyl cyclase a receptor peptide activator for acute kidney injury. Circ. Res. 124, 1462–1472. doi: 10.1161/circresaha.118.314164
Chinkers, M., Garbers, D. L., Chang, M. S., Lowe, D. G., Chin, H. M., Goeddel, D. V., et al. (1989). A membrane form of guanylate cyclase is an atrial natriuretic peptide receptor. Nature 338, 78–83. doi: 10.1038/338078a0
Choi, M. R., Citarella, M. R., Lee, B. M., Kouyoumdzian, N. M., Rukavina Mikusic, N. L., and Fernandez, B. E. (2013). Urodilatin regulates renal dopamine metabolism. J. Nephrol. 26, 1042–1048. doi: 10.5301/jn.5000279
Choi, M. R., Rukavina Mikusic, N. L., Kouyoumdzian, N. M., Kravetz, M. C., and Fernandez, B. E. (2014). Atrial natriuretic peptide and renal dopaminergic system: a positive friendly relationship? Biomed. Res. Int. 2014:710781.
Chusho, H., Tamura, N., Ogawa, Y., Yasoda, A., Suda, M., Miyazawa, T., et al. (2001). Dwarfism and early death in mice lacking C-type natriuretic peptide. Proc. Natl. Acad. Sci. U.S.A. 98, 4016–4021. doi: 10.1073/pnas.071389098
Citarella, M. R., Choi, M. R., Gironacci, M. M., Medici, C., Correa, A. H., and Fernandez, B. E. (2009). Urodilatin and dopamine: a new interaction in the kidney. Regul Pept 153, 19–24. doi: 10.1016/j.regpep.2008.11.009
Colucci, W. S., Elkayam, U., Horton, D. P., Abraham, W. T., Bourge, R. C., Johnson, A. D., et al. (2000). Intravenous nesiritide, a natriuretic peptide, in the treatment of decompensated congestive heart failure. nesiritide study group. N. Engl. J. Med. 343, 246–253.
Coue, M., Badin, P. M., Vila, I. K., Laurens, C., Louche, K., Marques, M. A., et al. (2015). Defective natriuretic peptide receptor signaling in skeletal muscle links obesity to type 2 diabetes. Diabetes 64, 4033–4045. doi: 10.2337/db15-0305
Coue, M., and Moro, C. (2016). Natriuretic peptide control of energy balance and glucose homeostasis. Biochimie 124, 84–91. doi: 10.1016/j.biochi.2015.05.017
Das, S., Au, E., Krazit, S. T., and Pandey, K. N. (2010). Targeted disruption of guanylyl cyclase-A/natriuretic peptide receptor-A gene provokes renal fibrosis and remodeling in null mutant mice: role of proinflammatory cytokines. Endocrinology 151, 5841–5850. doi: 10.1210/en.2010-0655
Das, S., Neelamegam, K., Peters, W. N., Periyasamy, R., and Pandey, K. N. (2020). Depletion of cyclic-GMP levels and inhibition of cGMP-dependent protein kinase activate p21(Cip1) /p27(Kip1) pathways and lead to renal fibrosis and dysfunction. FASEB J. 34, 11925–11943. doi: 10.1096/fj.202000754r
de Bold, A. J. (1985). Atrial natriuretic factor: a hormone produced by the heart. Science 230, 767–770. doi: 10.1126/science.2932797
de Bold, A. J., Borenstein, H. B., Veress, A. T., and Sonnenberg, H. (1981). A rapid and potent natriuretic response to intravenous injection of atrial myocardial extract in rats. Life Sci. 28, 89–94. doi: 10.1016/0024-3205(81)90370-2
de Bold, A. J., Ma, K. K., Zhang, Y., De Bold, M. L., Bensimon, M., and Khoshbaten, A. (2001). The physiological and pathophysiological modulation of the endocrine function of the heart. Can. J. Physiol. Pharmacol. 79, 705–714. doi: 10.1139/y01-038
de Lean, A., Mcnicoll, N., and Labrecque, J. (2003). Natriuretic peptide receptor A activation stabilizes a membrane-distal dimer interface. J. Biol. Chem. 278, 11159–11166. doi: 10.1074/jbc.m212862200
Deschepper, C. F., Masciotra, S., Zahabi, A., Boutin-Ganache, I., Picard, S., and Reudelhuber, T. L. (2001). Function alterations of the Nppa promoter are linked to cardiac ventricular hypertrophy in WKY/WKHA rat crosses. Circ. Res. 88, 223–228. doi: 10.1161/01.res.88.2.223
Dohi, K., and Ito, M. (2014). Novel diuretic strategies for the treatment of heart failure in Japan. Circ. J. 78, 1816–1823. doi: 10.1253/circj.cj-14-0592
Doust, J. A., Pietrzak, E., Dobson, A., and Glasziou, P. (2005). How well does B-type natriuretic peptide predict death and cardiac events in patients with heart failure: systematic review. BWJ 330:625. doi: 10.1136/bmj.330.7492.625
Drewett, J. G., and Garbers, D. L. (1994). The family of guanylyl cyclase receptors and their ligands. Endocr Rev 15, 135–162. doi: 10.1210/edrv-15-2-135
Ellmers, L. J., Knowles, J. W., Kim, H. S., Smithies, O., Maeda, N., and Cameron, V. A. (2002). Ventricular expression of natriuretic peptides in Npr1(-/-) mice with cardiac hypertrophy and fibrosis. Am. J. Physiol. Heart Circ. Physiol. 283, H707–H714.
Ellmers, L. J., Scott, N. J., Piuhola, J., Maeda, N., Smithies, O., Frampton, C. M., et al. (2007). Npr1-regulated gene pathways contributing to cardiac hypertrophy and fibrosis. J. Mol. Endocrinol. 38, 245–257. doi: 10.1677/jme.1.02138
Engeli, S., Birkenfeld, A. L., Badin, P. M., Bourlier, V., Louche, K., Viguerie, N., et al. (2012). Natriuretic peptides enhance the oxidative capacity of human skeletal muscle. J. Clin. Invest. 122, 4675–4679. doi: 10.1172/jci64526
Felker, G. M., Petersen, J. W., and Mark, D. B. (2006). Natriuretic peptides in the diagnosis and management of heart failure. CMAJ 175, 611–617.
Feller, S. M., Mägert, H. J., Schulz-Knappe, P., and Forssmann, W. G. (1990). “Urodilatin (hANF 95-126) -characteristics of a new atrial natriuretic factor peptide,” in Atrial Natriuretic Factor, ed. A. D. Struthers (Oxford: Blackwell), 209–226.
Forssmann, W. G., Meyer, M., and Schulz-Knappe, P. (1994). Urodilatin: from cardiac hormones to clinical trials. Exp. Nephrol. 2, 318–323.
Frantz, S., Fraccarollo, D., Wagner, H., Behr, T. M., Jung, P., Angermann, C. E., et al. (2003). Sustained activation of nuclear factor kappa B and activator protein 1 in chronic heart failure. Cardiovasc. Res. 57, 749–756. doi: 10.1016/s0008-6363(02)00723-x
Freeman, R. H., Davis, J. O., and Vari, R. C. (1985). Renal response to atrial natriuretic factor in conscious dogs with caval constriction. Am. J. Physiol. 248, R495–R500.
Fuller, F., Porter, J. G., Arfsten, A. E., Miller, J., Schilling, J. W., Scarborough, R. M., et al. (1988). Atrial natriuretic peptide clearance receptor. Complete sequence and functional expression of cDNA clones. J. Biol. Chem. 263, 9395–9401. doi: 10.1016/s0021-9258(19)76554-5
Garbers, D. L. (1992). Guanylyl cyclase receptors and their endocrine, paracrine, and autocrine ligands. Cell 71, 1–4.
Garbers, D. L., Chrisman, T. D., Wiegn, P., Katafuchi, T., Albanesi, J. P., Bielinski, V., et al. (2006). Membrane guanylyl cyclase receptors: an update. Trends Endocrinol. Metab 17, 251–258.
Garcia, R., Thibault, G., Cantin, M., and Genest, J. (1984). Effect of a purified atrial natriuretic factor on rat and rabbit vascular strips and vascular beds. Am. J. Physiol. 247, R34–R39.
Gardner, D. G., Deschepper, C. F., Ganong, W. F., Hane, S., Fiddes, J., Baxter, J. D., et al. (1986). Extra-atrial expression of the gene for atrial natriuretic factor. Proc. Natl. Acad. Sci. U.S.A. 83, 6697–6701. doi: 10.1073/pnas.83.18.6697
Gardner, R. S., Chong, K. S., Murday, A. J., Morton, J. J., and Mcdonagh, T. A. (2006). N-terminal brain natriuretic peptide is predictive of death after cardiac transplantation. Heart 92, 121–123. doi: 10.1136/hrt.2004.057778
Girsen, A., Ala-Kopsala, M., Makikallio, K., Vuolteenaho, O., and Rasanen, J. (2007). Cardiovascular hemodynamics and umbilical artery N-terminal peptide of proB-type natriuretic peptide in human fetuses with growth restriction. Ultrasound Obstet. Gynecol. 29, 296–303. doi: 10.1002/uog.3934
Goetz, K. L. (1991). Renal natriuretic peptide (urodilatin?) and atriopeptin: evolving concepts. Am. J. Physiol. 261, F921–F932.
Gogulamudi, V. R., Mani, I., Subramanian, U., and Pandey, K. N. (2019). Genetic disruption of Npr1 depletes regulatory T cells and provokes high levels of proinflammatory cytokines and fibrosis in the kidneys of female mutant mice. Am. J. Physiol. Renal. Physiol. 316, F1254–F1272.
Guo, L. J., Alli, A. A., Eaton, D. C., and Bao, H. F. (2013). ENaC is regulated by natriuretic peptide receptor-dependent cGMP signaling. Am. J. Physiol. Renal. Physiol. 304, F930–F937.
Hanford, D. S., and Glembotski, C. C. (1996). Stabilization of the B-type natriuretic peptide mRNA in cardiac myocytes by alpha-adrenergic receptor activation: potential roles for protein kinase C and mitogen-activated protein kinase. Mol. Endocrinol. 10, 1719–1727. doi: 10.1210/mend.10.12.8961280
Hanford, D. S., Thuerauf, D. J., Murray, S. F., and Glembotski, C. C. (1994). Brain natriuretic peptide is induced by alpha 1-adrenergic agonists as a primary response gene in cultured rat cardiac myocytes. J. Biol. Chem. 269, 26227–26233. doi: 10.1016/s0021-9258(18)47183-9
Hayek, S., and Nemer, M. (2011). Cardiac natriuretic peptides: from basic discovery to clinical practice. Cardiovasc. Ther. 29, 362–376. doi: 10.1111/j.1755-5922.2010.00152.x
He, X., Chow, D., Martick, M. M., and Garcia, K. C. (2001). Allosteric activation of a spring-loaded natriuretic peptide receptor dimer by hormone. Science 293, 1657–1662. doi: 10.1126/science.1062246
Hirota, H., Yoshida, K., Kishimoto, T., and Taga, T. (1995). Continuous activation of gp130, a signal-transducing receptor component for interleukin 6-related cytokines, causes myocardial hypertrophy in mice. Proc. Natl. Acad. Sci. U.S.A. 92, 4862–4866. doi: 10.1073/pnas.92.11.4862
Holmes, S. J., Espiner, E. A., Richards, A. M., Yandle, T. G., and Frampton, C. (1993). Renal, endocrine, and hemodynamic effects of human brain natriuretic peptide in normal man. J. Clin. Endocrinol. Metab. 76, 91–96. doi: 10.1210/jc.76.1.91
Holtwick, R., Van Eickels, M., Skryabin, B. V., Baba, H. A., Bubikat, A., Begrow, F., et al. (2003). Pressure-independent cardiac hypertrophy in mice with cardiomyocyte-restricted inactivation of the atrial natriuretic peptide receptor guanylyl cyclase-A. J. Clin. Invest. 111, 1399–1407. doi: 10.1172/jci17061
Hunt, P. J., Richards, A. M., Espiner, E. A., Nicholls, M. G., and Yandle, T. G. (1994). Bioactivity and metabolism of C-type natriuretic peptide in normal man. J. Clin. Endocrinol. Metab. 78, 1428–1435. doi: 10.1210/jc.78.6.1428
Huo, X., Abe, T., and Misono, K. S. (1999). Ligand binding-dependent limited proteolysis of the atrial natriuretic peptide receptor: juxtamembrane hinge structure essential for transmembrane signal transduction. Biochemistry 38, 16941–16951. doi: 10.1021/bi9919448
Ichiki, T., Dzhoyashvili, N., and Burnett, J. C. Jr. (2019). Natriuretic peptide based therapeutics for heart failure: cenderitide: a novel first-in-class designer natriuretic peptide. Int J Cardiol 281, 166–171. doi: 10.1016/j.ijcard.2018.06.002
Igaki, T., Itoh, H., Suga, S., Hama, N., Ogawa, Y., Komatsu, Y., et al. (1996). C-type natriuretic peptide in chronic renal failure and its action in humans. Kidney Int. Suppl. 55, S144–S147.
Isono, M., Haneda, M., Maeda, S., Omatsu-Kanbe, M., and Kikkawa, R. (1998). Atrial natriuretic peptide inhibits endothelin-1-induced activation of JNK in glomerular mesangial cells. Kidney Int. 53, 1133–1142. doi: 10.1046/j.1523-1755.1998.00869.x
Itoh, H., Pratt, R. E., and Dzau, V. (1990). Atrial natriuretic polypeptide inhibits hypertrophy of vascular smooth muscle cells. J. Clin. Invest. 86, 1690–1697. doi: 10.1172/jci114893
Jaffe, A. S., Babuin, L., and Apple, F. S. (2006). Biomarkers in acute cardiac disease: the present and the future. J. Am. Coll. Cardiol. 48, 1–11.
John, S. W., Krege, J. H., Oliver, P. M., Hagaman, J. R., Hodgin, J. B., Pang, S. C., et al. (1995). Genetic decreases in atrial natriuretic peptide and salt-sensitive hypertension. Science 267, 679–681. doi: 10.1126/science.7839143
Jordan, J., Birkenfeld, A. L., Melander, O., and Moro, C. (2018). natriuretic peptides in cardiovascular and metabolic crosstalk: implications for hypertension management. Hypertension 72, 270–276. doi: 10.1161/hypertensionaha.118.11081
Kaupp, U. B., and Seifert, R. (2002). Cyclic nucleotide-gated ion channels. Physiol. Rev. 82, 769–824.
Khan, I. A., Fink, J., Nass, C., Chen, H., Christenson, R., and Defilippi, C. R. (2006). N-terminal pro-B-type natriuretic peptide and B-type natriuretic peptide for identifying coronary artery disease and left ventricular hypertrophy in ambulatory chronic kidney disease patients. Am. J. Cardiol. 97, 1530–1534. doi: 10.1016/j.amjcard.2005.11.090
Khurana, M. L., and Pandey, K. N. (1993). Receptor-mediated stimulatory effect of atrial natriuretic factor, brain natriuretic peptide, and C-type natriuretic peptide on testosterone production in purified mouse Leydig cells: activation of cholesterol side-chain cleavage enzyme. Endocrinology 133, 2141–2149. doi: 10.1210/endo.133.5.8404664
Kilic, A., Bubikat, A., Gassner, B., Baba, H. A., and Kuhn, M. (2007). Local actions of atrial natriuretic peptide counteract angiotensin II stimulated cardiac remodeling. Endocrinology 148, 4162–4169. doi: 10.1210/en.2007-0182
Kishimoto, I., Tokudome, T., Nakao, K., and Kangawa, K. (2011). Natriuretic peptide system: an overview of studies using genetically engineered animal models. FEBS J 278, 1830–1841. doi: 10.1111/j.1742-4658.2011.08116.x
Knowles, J. W., Esposito, G., Mao, L., Hagaman, J. R., Fox, J. E., Smithies, O., et al. (2001). Pressure-independent enhancement of cardiac hypertrophy in natriuretic peptide receptor A-deficient mice. J. Clin. Invest. 107, 975–984. doi: 10.1172/jci11273
Kocylowski, R. D., Dubiel, M., Gudmundsson, S., Sieg, I., Fritzer, E., Alkasi, O., et al. (2009). Biochemical tissue-specific injury markers of the heart and brain in postpartum cord blood. Am. J. Obstet. Gynecol. 200, 273.e1–273.e25.
Koh, G. Y., Nussenzveig, D. R., Okolicany, J., Price, D. A., and Maack, T. (1992). Dynamics of atrial natriuretic factor-guanylate cyclase receptors and receptor-ligand complexes in cultured glomerular mesangial and renomedullary interstitial cells. J. Biol. Chem. 267, 11987–11994. doi: 10.1016/s0021-9258(19)49795-0
Koller, K. J., and Goddel, D. V. (1992). Molecular biology of the natriuretic peptides and their receptors. Circulation 86, 1081–1088. doi: 10.1161/01.cir.86.4.1081
Koller, K. J., Lowe, D. G., Bennett, G. L., Minamino, N., Kangawa, K., Matsuo, H., et al. (1991). Selective activation of the B natriuretic peptide receptor by C-type natriuretic peptide (CNP). Science 252, 120–123. doi: 10.1126/science.1672777
Kremer, S., Troyer, D., Kreisberg, J., and Skorecki, K. (1988). Interaction of atrial natriuretic peptide-stimulated guanylyl cyclase and vasopressin-stimulated calcium signaling pathways in the glomerular mesangial cells. Arch. Biochem. Biophys. 260, 763–767. doi: 10.1016/0003-9861(88)90506-1
Krown, K. A., Page, M. T., Nguyen, C., Zechner, D., Gutierrez, V., Comstock, K. L., et al. (1996). Tumor necrosis factor alpha-induced apoptosis in cardiac myocytes. Involvement of the sphingolipid signaling cascade in cardiac cell death. J. Clin. Invest. 98, 2854–2865. doi: 10.1172/jci119114
Kuhn, M. (2005). Cardiac and intestinal natriuretic peptides: insights from genetically modified mice. Peptides 26, 1078–1085. doi: 10.1016/j.peptides.2004.08.031
Kuhn, M., Volker, K., Schwarz, K., Carbajo-Lozoya, J., Flogel, U., Jacoby, C., et al. (2009). The natriuretic peptide/guanylyl cyclase–a system functions as a stress-responsive regulator of angiogenesis in mice. J. Clin. Invest. 119, 2019–2030. doi: 10.1172/jci37430
Kumar, P., Gogulamudi, V. R., Periasamy, R., Raghavaraju, G., Subramanian, U., and Pandey, K. N. (2017). Inhibition of HDAC enhances STAT acetylation, blocks NF-kappaB, and suppresses the renal inflammation and fibrosis in Npr1 haplotype male mice. Am. J. Physiol. Renal. Physiol. 313, F781–F795.
Kumar, P., Tripathi, S., and Pandey, K. N. (2014). Histone deacetylase inhibitors modulate the transcriptional regulation of guanylyl cyclase/natriuretic peptide receptor-a gene: interactive roles of modified histones, histone acetyltransferase, p300. AND Sp1. J. Biol. Chem. 289, 6991–7002. doi: 10.1074/jbc.m113.511444
Kumar, R., Cartledge, W. A., Lincoln, T. M., and Pandey, K. N. (1997a). Expression of guanylyl cyclase-A/atrial natriuretic peptide receptor blocks the activation of protein kinase C in vascular smooth muscle cells. Role of cGMP and cGMP-dependent protein kinase. Hypertension 29, 414–421. doi: 10.1161/01.hyp.29.1.414
Kumar, R., Von Geldern, T. W., Calle, R. A., and Pandey, K. N. (1997b). Stimulation of atrial natriuretic peptide receptor/guanylyl cyclase- A signaling pathway antagonizes the activation of protein kinase C-alpha in murine Leydig cells. Biochim. Biophys. Acta 1356, 221–228. doi: 10.1016/s0167-4889(96)00168-1
Kurrelmeyer, K. M., Michael, L. H., Baumgarten, G., Taffet, G. E., Peschon, J. J., Sivasubramanian, N., et al. (2000). Endogenous tumor necrosis factor protects the adult cardiac myocyte against ischemic-induced apoptosis in a murine model of acute myocardial infarction. Proc. Natl. Acad. Sci. U.S.A. 97, 5456–5461. doi: 10.1073/pnas.070036297
Langenickel, T. H., Buttgereit, J., Pagel-Langenickel, I., Lindner, M., Monti, J., Beuerlein, K., et al. (2006). Cardiac hypertrophy in transgenic rats expressing a dominant-negative mutant of the natriuretic peptide receptor B. Proc. Natl. Acad. Sci. U.S.A. 103, 4735–4740. doi: 10.1073/pnas.0510019103
LaPointe, M. C. (2005). Molecular regulation of the brain natriuretic peptide gene. Peptides 26, 944–956. doi: 10.1016/j.peptides.2004.08.028
Lee, C. Y., Lieu, H., and Burnett, J. C. Jr. (2009). Designer natriuretic peptides. J. Investig. Med. 57, 18–21.
Leitman, D. C., Andresen, J. W., Catalano, R. M., Waldman, S. A., Tuan, J. J., and Murad, F. (1988). Atrial natriuretic peptide binding, cross-linking, and stimulation of cyclic GMP accumulation and particulate guanylate cyclase activity in cultured cells. J. Biol. Chem. 263, 3720–3728. doi: 10.1016/s0021-9258(18)68984-7
Levin, E. R., Gardner, D. G., and Samson, W. K. (1998). Natriuretic peptides. N. Engl. J. Med. 339, 321–328.
Li, Y., Ha, T., Gao, X., Kelley, J., Williams, D. L., Browder, I. W., et al. (2004). NF-kappaB activation is required for the development of cardiac hypertrophy in vivo. Am. J. Physiol. Heart Circ. Physiol. 287, H1712–H1720.
Li, Y., Kishimoto, I., Saito, Y., Harada, M., Kuwahara, K., Izumi, T., et al. (2002). Guanylyl cyclase-A inhibits angiotensin II type 1A receptor-mediated cardiac remodeling, an endogenous protective mechanism in the heart. Circulation 106, 1722–1728. doi: 10.1161/01.cir.0000029923.57048.61
Li, Y. Y., Feng, Y. Q., Kadokami, T., Mctiernan, C. F., Draviam, R., Watkins, S. C., et al. (2000). Myocardial extracellular matrix remodeling in transgenic mice overexpressing tumor necrosis factor alpha can be modulated by anti-tumor necrosis factor alpha therapy. Proc. Natl. Acad. Sci. U.S.A. 97, 12746–12751. doi: 10.1073/pnas.97.23.12746
Light, D. B., Schwiebert, E. M., Karlson, K. H., and Stanton, B. A. (1989). Atrial natriuretic peptide inhibits a cation channel in renal inner medullary collecting duct cells. Science 243, 383–385. doi: 10.1126/science.2463673
Lin, K. F., Chao, J., and Chao, L. (1995). Human atrial natriuretic peptide gene delivery reduces blood pressure in hypertensive rats. Hypertension 26, 847–853. doi: 10.1161/01.hyp.26.6.847
Lisy, O., Huntley, B. K., Mccormick, D. J., Kurlansky, P. A., and Burnett, J. C. Jr. (2008). Design, synthesis, and actions of a novel chimeric natriuretic peptide: CD-NP. J. Am. Coll. Cardiol. 52, 60–68. doi: 10.1016/j.jacc.2008.02.077
Lisy, O., Jougasaki, M., Heublein, D. M., Schirger, J. A., Chen, H. H., Wennberg, P. W., et al. (1999). Renal actions of synthetic dendroaspis natriuretic peptide. Kidney Int. 56, 502–508. doi: 10.1046/j.1523-1755.1999.00573.x
Lopez, M. J., Wong, S. K., Kishimoto, I., Dubois, S., Mach, V., Friesen, J., et al. (1995). Salt-resistant hypertension in mice lacking the guanylyl cyclase-A receptor for atrial natriuretic peptide. Nature 378, 65–68. doi: 10.1038/378065a0
Lowe, D. G., Chang, M. S., Hellmiss, R., Chen, E., Singh, S., Garbers, D. L., et al. (1989). Human atrial natriuretic peptide receptor defines a new paradigm for second messenger signal transduction. EMBO J. 8, 1377–1384. doi: 10.1002/j.1460-2075.1989.tb03518.x
Lucas, K. A., Pitari, G. M., Kazerounian, S., Ruiz-Stewart, I., Park, J., Schulz, S., et al. (2000). Guanylyl cyclases and signaling by cyclic GMP. Pharmacol. Rev. 52, 375–414.
Maack, T., Suzuki, M., Almeida, F. A., Nussenzveig, D., Scarborough, R. M., Mcenroe, G. A., et al. (1987). Physiological role of silent receptors of atrial natriuretic factor. Science 238, 675–678. doi: 10.1126/science.2823385
MacFarland, R. T., Zelus, B. D., and Beavo, J. A. (1991). High concentrations of a cGMP-stimulated phosphodiesterase mediate ANP-induced decreases in cAMP and steroidogenesis in adrenal glomerulosa cells. J. Biol. Chem. 266, 136–142. doi: 10.1016/s0021-9258(18)52413-3
Macheret, F., Heublein, D., Costello-Boerrigter, L. C., Boerrigter, G., Mckie, P., Bellavia, D., et al. (2012). Human hypertension is characterized by a lack of activation of the antihypertensive cardiac hormones ANP and BNP. J. Am. Coll. Cardiol. 60, 1558–1565. doi: 10.1016/j.jacc.2012.05.049
Maisel, A. S., Krishnaswamy, P., Nowak, R. M., Mccord, J., Hollander, J. E., Duc, P., et al. (2002). Rapid measurement of B-type natriuretic peptide in the emergency diagnosis of heart failure. N. Engl. J. Med. 347, 161–167.
Maki, M., Takayanagi, R., Misono, K., Pandey, K. N., Tibbetts, C., and Inagami, T. (1984). Structure of rat atrial natriuretic factor precursor deduced from cDNA sequence. Nature 309, 722–724. doi: 10.1038/309722a0
Man, J. C. K., Van Duijvenboden, K., Krijger, P. H. L., Hooijkaas, I. B., Van Der Made, I., De Gier-De Vries, C., et al. (2021). Genetic dissection of a super enhancer controlling the nppa-nppb cluster in the heart. Circ. Res. 128, 115–129. doi: 10.1161/circresaha.120.317045
Mani, I., Garg, R., and Pandey, K. N. (2016). Role of FQQI motif in the internalization, trafficking, and signaling of guanylyl-cyclase/natriuretic peptide receptor-A in cultured murine mesangial cells. Am. J. Physiol. Renal. Physiol. 310, F68–F84.
Mani, I., Garg, R., Tripathi, S., and Pandey, K. N. (2015). Subcellular trafficking of guanylyl cyclase/natriuretic peptide receptor-A with concurrent generation of intracellular cGMP. Biosci. Rep. 35:e00260.
Mani, I., and Pandey, K. N. (2019). Emerging concepts of receptor endocytosis and concurrent intracellular signaling: mechanisms of guanylyl cyclase/natriuretic peptide receptor-A activation and trafficking. Cell Signal. 60, 17–30. doi: 10.1016/j.cellsig.2019.03.022
Matsukawa, N., Grzesik, W. J., Takahashi, N., Pandey, K. N., Pang, S., Yamauchi, M., et al. (1999). The natriuretic peptide clearance receptor locally modulates the physiological effects of the natriuretic peptide system. Proc. Natl. Acad. Sci. U.S.A. 96, 7403–7408. doi: 10.1073/pnas.96.13.7403
Maurice, D. H., Palmer, D., Tilley, D. G., Dunkerley, H. A., Netherton, S. J., Raymond, D. R., et al. (2003). Cyclic nucleotide phosphodiesterase activity, expression, and targeting in cells of the cardiovascular system. Mol. Pharmacol. 64, 533–546. doi: 10.1124/mol.64.3.533
McGrath, M. F., De Bold, M. L., and De Bold, A. J. (2005). The endocrine function of the heart. Trends Endocrinol. Metab. 16, 469–477.
McMurray, J. J., Packer, M., Desai, A. S., Gong, J., Lefkowitz, M. P., Rizkala, A. R., et al. (2014). Angiotensin-neprilysin inhibition versus enalapril in heart failure. N. Engl. J. Med. 371, 993–1004.
Melo, L. G., Steinhelper, M. E., Pang, S. C., Tse, Y., and Ackermann, U. (2000). ANP in regulation of arterial pressure and fluid-electrolyte balance: lessons from genetic mouse models. Physiol. Genomics 3, 45–58. doi: 10.1152/physiolgenomics.2000.3.1.45
Melo, L. G., Veress, A. T., Ackermann, U., Steinhelper, M. E., Pang, S. C., Tse, Y., et al. (1999). Chronic regulation of arterial blood pressure in ANP transgenic and knockout mice: role of cardiovascular sympathetic tone. Cardiovasc. Res. 43, 437–444. doi: 10.1016/s0008-6363(99)00104-2
Meyer, M., and Forsmann, W. G. (1997). “Renal actions of atrial natriuretic peptide,” in Contemporary Endocrinology: Natriuretic Peptides in Health and Disease, eds W. K. Samson and E. R. Levin (Totawa, NJ: Humana Press), 147–170. doi: 10.1007/978-1-4612-3960-4_9
Meyer, M., Richter, R., Brunkhorst, R., Wrenger, E., Schulz-Knappe, P., Kist, A., et al. (1996). Urodilatin is involved in sodium homeostasis and exerts sodium-state-dependent natriuretic and diuretic effects. Am. J. Physiol. 271, F489–F497.
Meyer, M., Wiebe, K., Wahlers, T., Zenker, D., Schulze, F. P., Michels, P., et al. (1997). Urodilatin (INN:ularitide) as a new drug for the therapy of acute renal failure following cardiac surgery. Clin. Exp. Pharmacol. Physiol. 24, 374–376. doi: 10.1111/j.1440-1681.1997.tb01205.x
Misono, K. S. (2000). Atrial natriuretic factor binding to its receptor is dependent on chloride concentration: a possible feedback-control mechanism in renal salt regulation. Circ. Res. 86, 1135–1139. doi: 10.1161/01.res.86.11.1135
Misono, K. S., Fukumi, H., Grammer, R. T., and Inagami, T. (1984). Rat atrial natriuretic factor: complete amino acid sequence and disulfide linkage essential for biological activity. Biochem. Biophys. Res. Commun. 119, 524–529. doi: 10.1016/s0006-291x(84)80279-x
Misono, K. S., Ogawa, H., Qiu, Y., and Ogata, C. M. (2005). Structural studies of the natriuretic peptide receptor: a novel hormone-induced rotation mechanism for transmembrane signal transduction. Peptides 26, 957–968. doi: 10.1016/j.peptides.2004.12.021
Misono, K. S., Philo, J. S., Arakawa, T., Ogata, C. M., Qiu, Y., Ogawa, H., et al. (2011). Structure, signaling mechanism and regulation of the natriuretic peptide receptor guanylate cyclase. FEBS J. 278, 1818–1829. doi: 10.1111/j.1742-4658.2011.08083.x
Mitsuishi, M., Miyashita, K., and Itoh, H. (2008). cGMP rescues mitochondrial dysfunction induced by glucose and insulin in myocytes. Biochem. Biophys. Res. Commun. 367, 840–845. doi: 10.1016/j.bbrc.2008.01.017
Miyashita, K., Itoh, H., Tsujimoto, H., Tamura, N., Fukunaga, Y., Sone, M., et al. (2009). Natriuretic peptides/cGMP/cGMP-dependent protein kinase cascades promote muscle mitochondrial biogenesis and prevent obesity. Diabetes 58, 2880–2892. doi: 10.2337/db09-0393
Morita, E., Yasue, H., Yoshimura, M., Ogawa, H., Jougasaki, M., Matsumura, T., et al. (1993). Increased plasma levels of brain natriuretic peptide in patients with acute myocardial infarction. Circulation 88, 82–91. doi: 10.1161/01.cir.88.1.82
Moro, C. (2013). Natriuretic peptides and fat metabolism. Curr. Opin. Clin. Nutr. Metab. Care 16, 645–649. doi: 10.1097/mco.0b013e32836510ed
Moyes, A. J., Chu, S. M., Aubdool, A. A., Dukinfield, M. S., Margulies, K. B., Bedi, K. C., et al. (2020). C-type natriuretic peptide co-ordinates cardiac structure and function. Eur. Heart J. 41, 1006–1020. doi: 10.1093/eurheartj/ehz093
Mukoyama, M., Nakao, K., Hosoda, K., Suga, S., Saito, Y., Ogawa, Y., et al. (1991). Brain natriuretic peptide as a novel cardiac hormone in humans. Evidence for an exquisite dual natriuretic peptide system, atrial natriuretic peptide and brain natriuretic peptide. J. Clin. Invest. 87, 1402–1412. doi: 10.1172/jci115146
Nakagawa, Y., Nishikimi, T., and Kuwahara, K. (2019). Atrial and brain natriuretic peptides: hormones secreted from the heart. Peptides 111, 18–25. doi: 10.1016/j.peptides.2018.05.012
Nakanishi, M., Saito, Y., Kishimoto, I., Harada, M., Kuwahara, K., Takahashi, N., et al. (2005). Role of natriuretic peptide receptor guanylyl cyclase-A in myocardial infarction evaluated using genetically engineered mice. Hypertension 46, 441–447. doi: 10.1161/01.hyp.0000173420.31354.ef
Nakao, K., Itoh, H., Saito, Y., Mukoyama, M., and Ogawa, Y. (1996). The natriuretic peptide family. Curr. Opin. Nephrol. Hypertens 5, 4–11.
Nakao, K., Sugawara, A., Morii, N., Sakamoto, M., Yamada, T., Itoh, H., et al. (1986). The pharmacokinetics of alpha-human atrial natriuretic polypeptide in healthy subjects. Eur. J. Clin. Pharmacol. 31, 101–103. doi: 10.1007/bf00870995
Nakayama, T., Soma, M., Takahashi, Y., Rehemudula, D., Kanmatsuse, K., and Furuya, K. (2000). Functional deletion mutation of the 5’-flanking region of type A human natriuretic peptide receptor gene and its association with essential hypertension and left ventricular hypertrophy in the Japanese. Circ. Res. 86, 841–845. doi: 10.1161/01.res.86.8.841
Newton-Cheh, C., Larson, M. G., Vasan, R. S., Levy, D., Bloch, K. D., Surti, A., et al. (2009). Association of common variants in NPPA and NPPB with circulating natriuretic peptides and blood pressure. Nat. Genet. 41, 348–353. doi: 10.1038/ng.328
Nishikimi, T., Kuwahara, K., Nakagawa, Y., Kangawa, K., Minamino, N., and Nakao, K. (2013). Complexity of molecular forms of B-type natriuretic peptide in heart failure. Heart 99, 677–679. doi: 10.1136/heartjnl-2012-302929
Nonguchi, H., Knepper, M. A., and Mangiello, V. C. (1987). Effects of atrial natriuretic factor on cyclic guanosine monophosphate and cyclic adenosine monophosphate accumulation in microdissected nephron segments from rats. J. Clin. Invest. 79, 500–507. doi: 10.1172/jci112840
Obana, K., Naruse, N., Naruse, K., Sakurai, H., Demura, H., Inagami, T., et al. (1985). Synthetic rat atrial natriuretic factor inhibits in vitro and in vivo rennin secretion in rats. Endocrinology 117, 1282–1284. doi: 10.1210/endo-117-3-1282
Oda, S., Sano, T., Morishita, Y., and Matsuda, Y. (1992). Pharmacological profile of HS-142-1, a novel nonpeptide atrial natriuretic peptide (ANP) antagonist of microbial origin II. Restoration by HS-142-1 of ANP-induced inhibition of aldosterone production in adrenal glomerulosa cells. J. Pharmacol. Exp. Thr. 263, 241–245.
Ogawa, Y., Itoh, H., Tamura, N., Suga, S., Yoshimasa, T., Uehira, M., et al. (1994). Molecular cloning of the complementary DNA and gene that encode mouse brain natriuretic peptide and generation of transgenic mice that overexpress the brain natriuretic peptide gene. J. Clin. Invest. 93, 1911–1921. doi: 10.1172/jci117182
Ogawa, Y., Nakao, K., Nakagawa, O., Komatsu, Y., Hosoda, K., Suga, S., et al. (1992). Human C-type natriuretic peptide. Characterization of the gene and peptide. Hypertension 19, 809–813.
Oliver, P. M., Fox, J. E., Kim, R., Rockman, H. A., Kim, H. S., Reddick, R. L., et al. (1997). Hypertension, cardiac hypertrophy, and sudden death in mice lacking natriuretic peptide receptor A. Proc. Natl. Acad. Sci. U.S.A. 94, 14730–14735. doi: 10.1073/pnas.94.26.14730
Oliver, P. M., John, S. W., Purdy, K. E., Kim, R., Maeda, N., Goy, M. F., et al. (1998). Natriuretic peptide receptor 1 expression influences blood pressures of mice in a dose-dependent manner. Proc. Natl. Acad. Sci. U.S.A. 95, 2547–2551. doi: 10.1073/pnas.95.5.2547
Omland, T., Aakvaag, A., Bonarjee, V. V., Caidahl, K., Lie, R. T., Nilsen, D. W., et al. (1996). Plasma brain natriuretic peptide as an indicator of left ventricular systolic function and long-term survival after acute myocardial infarction. Comparison with plasma atrial natriuretic peptide and N-terminal proatrial natriuretic peptide. Circulation 93, 1963–1969. doi: 10.1161/01.cir.93.11.1963
Oral, H., Sivasubramanian, N., Dyke, D. B., Mehta, R. H., Grossman, P. M., Briesmiester, K., et al. (2003). Myocardial proinflammatory cytokine expression and left ventricular remodeling in patients with chronic mitral regurgitation. Circulation 107, 831–837. doi: 10.1161/01.cir.0000049745.38594.6d
Palmieri, E. A., Benincasa, G., Di Rella, F., Casaburi, C., Monti, M. G., De Simone, G., et al. (2002). Differential expression of TNF-alpha, IL-6, and IGF-1 by graded mechanical stress in normal rat myocardium. Am. J. Physiol. Heart Circ. Physiol. 282, H926–H934.
Pandey, K. N. (1992). Kinetic analysis of internalization, recycling and redistribution of atrial natriuretic factor-receptor complex in cultured vascular smooth-muscle cells. Ligand-dependent receptor down-regulation. Biochem. J. 288(Pt 1), 55–61. doi: 10.1042/bj2880055
Pandey, K. N. (1993). Stoichiometric analysis of internalization, recycling, and redistribution of photoaffinity-labeled guanylate cyclase/atrial natriuretic factor receptors in cultured murine Leydig tumor cells. J. Biol. Chem. 268, 4382–4390. doi: 10.1016/s0021-9258(18)53621-8
Pandey, K. N. (2005a). Biology of natriuretic peptides and their receptors. Peptides 26, 901–932. doi: 10.1016/j.peptides.2004.09.024
Pandey, K. N. (2005b). Internalization and trafficking of guanylyl cyclase/natriuretic peptide receptor-A. Peptides 26, 985–1000. doi: 10.1016/j.peptides.2004.12.020
Pandey, K. N. (2008). Emerging roles of natriuretic peptides and their receptors in pathophysiology of hypertension and cardiovascular regulation. J. Am. Soc. Hypertens 2, 210–226. doi: 10.1016/j.jash.2008.02.001
Pandey, K. N. (2010). Ligand-mediated endocytosis and intracellular sequestration of guanylyl cyclase/natriuretic peptide receptors: role of GDAY motif. Mol. Cell Biochem. 334, 81–98. doi: 10.1007/s11010-009-0332-x
Pandey, K. N. (2011). The functional genomics of guanylyl cyclase/natriuretic peptide receptor-A: perspectives and paradigms. FEBS J. 278, 1792–1807. doi: 10.1111/j.1742-4658.2011.08081.x
Pandey, K. N. (2014). Guanylyl cyclase/natriuretic peptide receptor-A signaling antagonizes phosphoinositide hydrolysis, Ca(2+) release, and activation of protein kinase C. Front. Mol. Neurosci. 7:75.
Pandey, K. N. (2018). Molecular and genetic aspects of guanylyl cyclase natriuretic peptide receptor-A in regulation of blood pressure and renal function. Physiol Genomics 50, 913–928. doi: 10.1152/physiolgenomics.00083.2018
Pandey, K. N. (2019). Genetic ablation and guanylyl cyclase/natriuretic peptide receptor-a: impact on the pathophysiology of cardiovascular dysfunction. Int. J. Mol. Sci. 20:3946. doi: 10.3390/ijms20163946
Pandey, K. N., Inagami, T., and Misono, K. S. (1986). Atrial natriuretic factor receptor on cultured Leydig tumor cells: ligand binding and photoaffinity labeling. Biochemistry 25, 8467–8472. doi: 10.1021/bi00374a022
Pandey, K. N., and Kanungo, J. (1993). Expression of extracellular ligand-binding domain of murine guanylate cyclase/atrial natriuretic factor receptor cDNA in Escherichia coli. Biochem. Biophys. Res. Commun. 190, 724–731. doi: 10.1006/bbrc.1993.1109
Pandey, K. N., Kovacs, W. J., and Inagami, T. (1985). The inhibition of progesterone secretion and the regulation of cyclic nucleotides by atrial natriuretic factor in gonadotropin responsive murine Leydig tumor cells. Biochem. Biophys. Res. Commun. 133, 800–806. doi: 10.1016/0006-291x(85)90975-1
Pandey, K. N., Kumar, R., Li, M., and Nguyen, H. (2000). Functional domains and expression of truncated atrial natriuretic peptide receptor-A: the carboxyl-terminal regions direct the receptor internalization and sequestration in COS-7 cells. Mol. Pharmacol. 57, 259–267.
Pandey, K. N., Nguyen, H. T., Garg, R., Khurana, M. L., and Fink, J. (2005). Internalization and trafficking of guanylyl (guanylate) cyclase/natriuretic peptide receptor A is regulated by an acidic tyrosine-based cytoplasmic motif GDAY. Biochem. J. 388, 103–113. doi: 10.1042/bj20041250
Pandey, K. N., Nguyen, H. T., Sharma, G. D., Shi, S. J., and Kriegel, A. M. (2002). Ligand-regulated internalization, trafficking, and down-regulation of guanylyl cyclase/atrial natriuretic peptide receptor-A in human embryonic kidney 293 cells. J. Biol. Chem. 277, 4618–4627. doi: 10.1074/jbc.m106436200
Pandey, K. N., Pavlou, S. N., and Inagami, T. (1988). Identification and characterization of three distinct atrial natriuretic factor receptors. Evidence for tissue-specific heterogeneity of receptor subtypes in vascular smooth muscle, kidney tubular epithelium, and Leydig tumor cells by ligand binding, photoaffinity labeling, and tryptic proteolysis. J Biol Chem 263, 13406–13413. doi: 10.1016/s0021-9258(18)37719-6
Pandey, K. N., and Singh, S. (1990). Molecular cloning and expression of murine guanylate cyclase/atrial natriuretic factor receptor cDNA. J. Biol. Chem. 265, 12342–12348. doi: 10.1016/s0021-9258(19)38352-8
Pandey, K. N., and Vellaichamy, E. (2010). Regulation of cardiac angiotensin-converting enzyme and angiotensin AT1 receptor gene expression in Npr1 gene-disrupted mice. Clin. Exp. Pharmacol. Physiol. 37, e70–e77.
Park, K., Itoh, H., Yamahara, K., Sone, M., Miyashita, K., Oyamada, N., et al. (2008). Therapeutic potential of atrial natriuretic peptide administration on peripheral arterial diseases. Endocrinology 149, 483–491. doi: 10.1210/en.2007-1094
Parthasarathy, A., Gopi, V., Umadevi, S., Simna, A., Sheik, M. J., Divya, H., et al. (2013). Suppression of atrial natriuretic peptide/natriuretic peptide receptor-A-mediated signaling upregulates angiotensin-II-induced collagen synthesis in adult cardiac fibroblasts. Mol. Cell Biochem. 378, 217–228. doi: 10.1007/s11010-013-1612-z
Paul, R. V., Ferguson, T., and Navar, L. G. (1988). ANF secretion and renal responses to volume expansion with equilibrated blood. Am. J. Physiol. 255, F936–F943.
Pereira, N. L., Tosakulwong, N., Scott, C. G., Jenkins, G. D., Prodduturi, N., Chai, Y., et al. (2015). Circulating atrial natriuretic peptide genetic association study identifies a novel gene cluster associated with stroke in whites. Circ. Cardiovasc. Genet. 8, 141–149. doi: 10.1161/circgenetics.114.000624
Periyasamy, R., Das, S., and Pandey, K. N. (2019). Genetic disruption of guanylyl cyclase/natriuretic peptide receptor-A upregulates renal (pro) renin receptor expression in Npr1 null mutant mice. Peptides 114, 17–28. doi: 10.1016/j.peptides.2019.03.001
Pfeifer, A., Klatt, P., Massberg, S., Ny, L., Sausbier, M., Hirneiss, C., et al. (1998). Defective smooth muscle regulation in cGMP kinase I-deficient mice. EMBO J. 17, 3045–3051. doi: 10.1093/emboj/17.11.3045
Phillips, P. A., Sasadeus, J., Hodsman, G. P., Horowitz, J., Saltups, A., and Johnston, C. I. (1989). Plasma atrial natriuretic peptide in patients with acute myocardial infarction: effects of streptokinase. Br. Heart J. 61, 139–143. doi: 10.1136/hrt.61.2.139
Poschet, J. F., Timmins, G. S., Taylor-Cousar, J. L., Ornatowski, W., Fazio, J., Perkett, E., et al. (2007). Pharmacological modulation of cGMP levels by phosphodiesterase 5 inhibitors as a therapeutic strategy for treatment of respiratory pathology in cystic fibrosis. Am. J. Physiol. Lung Cell Mol. Physiol. 293, L712–L719.
Prieto, M. C., Das, S., Somanna, N. K., Harrison-Bernard, L. M., Navar, L. G., and Pandey, K. N. (2012). Disruption of Npr1 gene differentially regulates the juxtaglomerular and distal tubular renin levels in null mutant mice. Int. J. Physiol. Pathophysiol Pharmacol 4, 128–139.
Purcell, N. H., and Molkentin, J. D. (2003). Is nuclear factor kappaB an attractive therapeutic target for treating cardiac hypertrophy? Circulation 108, 638–640. doi: 10.1161/01.cir.0000085362.40608.dd
Rathinavelu, A., and Isom, G. E. (1991). Differential internalization and processing of atrial-natriuretic-factor B and C receptor in PC12 cells. Biochem J 276(Pt 2), 493–497. doi: 10.1042/bj2760493
Reginauld, S. H., Cannone, V., Iyer, S., Scott, C., Bailey, K., Schaefer, J., et al. (2019). Differential regulation of ANP and BNP in acute decompensated heart failure: deficiency of ANP. JACC Heart Fail 7, 891–898. doi: 10.1016/j.jchf.2019.05.012
Reinhart, K., Meisner, M., and Brunkhorst, F. M. (2006). Markers for sepsis diagnosis: what is useful? Crit. Care Clin. 22, 503–519. doi: 10.1016/j.ccc.2006.03.003
Richards, M., and Troughton, R. W. (2004). NT-proBNP in heart failure: therapy decisions and monitoring. Eur. J. Heart Fail 6, 351–354. doi: 10.1016/j.ejheart.2004.01.003
Rosenzweig, A., and Seidman, C. E. (1991). Atrial natriuretic factor and related peptide hormones. Annu. Rev. Biochem. 60, 229–255. doi: 10.1146/annurev.bi.60.070191.001305
Rubattu, S., Bigatti, G., Evangelista, A., Lanzani, C., Stanzione, R., Zagato, L., et al. (2006). Association of atrial natriuretic peptide and type a natriuretic peptide receptor gene polymorphisms with left ventricular mass in human essential hypertension. J. Am. Coll. Cardiol. 48, 499–505. doi: 10.1016/j.jacc.2005.12.081
Rubattu, S., Forte, M., Marchitti, S., and Volpe, M. (2019). Molecular implications of natriuretic peptides in the protection from hypertension and target organ damage development. Int. J. Mol. Sci. 20:798. doi: 10.3390/ijms20040798
Rubattu, S., and Volpe, M. (2014). High natriuretic peptide levels and low DBP: companion markers of cardiovascular risk? J. Hypertens 32, 2142–2143. doi: 10.1097/hjh.0000000000000365
Ruilope, L. M., Dukat, A., Bohm, M., Lacourciere, Y., Gong, J., and Lefkowitz, M. P. (2010). Blood-pressure reduction with LCZ696, a novel dual-acting inhibitor of the angiotensin II receptor and neprilysin: a randomised, double-blind, placebo-controlled, active comparator study. Lancet 375, 1255–1266. doi: 10.1016/s0140-6736(09)61966-8
Ruskoaho, H. (1992). Atrial natriuretic peptide: synthesis, release, and metabolism. Pharmacol. Rev. 44, 479–602.
Rybalkin, S. D., Yan, C., Bornfeldt, K. E., and Beavo, J. A. (2003). Cyclic GMP phosphodiesterases and regulation of smooth muscle function. Circ. Res. 93, 280–291. doi: 10.1161/01.res.0000087541.15600.2b
Sabrane, K., Kruse, M. N., Fabritz, L., Zetsche, B., Mitko, D., Skryabin, B. V., et al. (2005). Vascular endothelium is critically involved in the hypotensive and hypovolemic actions of atrial natriuretic peptide. J. Clin. Invest. 115, 1666–1674. doi: 10.1172/jci23360
Saito, Y. (2010). Roles of atrial natriuretic peptide and its therapeutic use. J. Cardiol. 56, 262–270. doi: 10.1016/j.jjcc.2010.08.001
Sano, T., Morishita, Y., Matsuda, Y., and Yamada, K. (1992). Pharmacological profile of HS-142-1, a novel nonpeptide atrial natriuretic peptide antagonist of microbial origin I. Selective inhibition of the actions of natriuretic peptides in anesthetized rats. J. Pharmacol. Exp. Thr. 260, 825–831.
Saxenhofer, H., Raselli, A., Weidmann, P., Forssmann, W. G., Bub, A., Ferrari, P., et al. (1990). Urodilatin, a natriuretic factor from kidneys, can modify renal and cardiovascular function in men. Am. J. Physiol. 259, F832–F838.
Schillinger, K. J., Tsai, S. Y., Taffet, G. E., Reddy, A. K., Marian, A. J., Entman, M. L., et al. (2005). Regulatable atrial natriuretic peptide gene therapy for hypertension. Proc. Natl. Acad. Sci. U.S.A. 102, 13789–13794. doi: 10.1073/pnas.0506807102
Schlossmann, J., Feil, R., and Hofmann, F. (2005). Insights into cGMP signalling derived from cGMP kinase knockout mice. Front. Biosci. 10:1279–1289. doi: 10.2741/1618
Schlueter, N., De Sterke, A., Willmes, D. M., Spranger, J., Jordan, J., and Birkenfeld, A. L. (2014). Metabolic actions of natriuretic peptides and therapeutic potential in the metabolic syndrome. Pharmacol. Ther. 144, 12–27. doi: 10.1016/j.pharmthera.2014.04.007
Schulz-Knappe, P., Forssmann, K., Herbst, F., Hock, D., Pipkorn, R., and Forssmann, W. G. (1988). Isolation and structural analysis of “urodilatin”, a new peptide of the cardiodilatin-(ANP)-family, extracted from human urine. Klin. Wochenschr. 66, 752–759. doi: 10.1007/bf01726570
Schulz, S. (2005). C-type natriuretic peptide and guanylyl cyclase B receptor. Peptides 26, 1024–1034. doi: 10.1016/j.peptides.2004.08.027
Schweitz, H., Vigne, P., Moinier, D., Frelin, C. H., and Lazdunski, M. (1992). A new member of the natriuretic peptide family is present in the venom of the green mamba (Dendroaspis angusticeps). J. Biol. Chem. 267, 13928–13932. doi: 10.1016/s0021-9258(19)49658-0
Scott, N. J., Ellmers, L. J., Lainchbury, J. G., Maeda, N., Smithies, O., Richards, A. M., et al. (2009). Influence of natriuretic peptide receptor-1 on survival and cardiac hypertrophy during development. Biochim. Biophys. Acta 1792, 1175–1184. doi: 10.1016/j.bbadis.2009.09.009
See, R., and de Lemos, J. A. (2006). Current status of risk stratification methods in acute coronary syndromes. Curr. Cardiol. Rep. 8, 282–288. doi: 10.1007/s11886-006-0060-8
Seilhamer, J. J., Arfsten, A., Miller, J. A., Lundquist, P., Scarborough, R. M., Lewicki, J. A., et al. (1989). Human and canine gene homologs of porcine brain natriuretic peptide. Biochem. Biophys. Res. Commun. 165, 650–658. doi: 10.1016/s0006-291x(89)80015-4
Sekiguchi, K., Li, X., Coker, M., Flesch, M., Barger, P. M., Sivasubramanian, N., et al. (2004). Cross-regulation between the renin-angiotensin system and inflammatory mediators in cardiac hypertrophy and failure. Cardiovasc. Res. 63, 433–442. doi: 10.1016/j.cardiores.2004.02.005
Sen, A., Kumar, P., Garg, R., Lindsey, S. H., Katakam, P. V., Bloodworth, M., et al. (2016). Transforming growth factor beta1 antagonizes the transcription, expression and vascular signaling of guanylyl cyclase/natriuretic peptide receptor A - role of deltaEF1. FEBS J. 283, 1767–1781. doi: 10.1111/febs.13701
Sengenes, C., Zakaroff-Girard, A., Moulin, A., Berlan, M., Bouloumie, A., Lafontan, M., et al. (2002). Natriuretic peptide-dependent lipolysis in fat cells is a primate specificity. Am. J. Physiol. Regul. Integr. Comp. Physiol. 283, R257–R265.
Sharma, G. D., Nguyen, H. T., Antonov, A. S., Gerrity, R. G., Von Geldern, T., and Pandey, K. N. (2002). Expression of atrial natriuretic peptide receptor-A antagonizes the mitogen-activated protein kinases (Erk2 and P38MAPK) in cultured human vascular smooth muscle cells. Mol. Cell Biochem. 233, 165–173.
Shi, S. J., Vellaichamy, E., Chin, S. Y., Smithies, O., Navar, L. G., and Pandey, K. N. (2003). Natriuretic peptide receptor A mediates renal sodium excretory responses to blood volume expansion. Am. J. Physiol. Renal. Physiol. 285, F694–F702.
Shi, S. J., Nguyen, H. T., Sharma, G. D., Navar, L. G., and Pandey, K. N. (2001). Genetic disruption of atrial natriuretic peptide receptor-A alters renin and angiotensin II levels. Am. J. Physiol. 281, F665–F673.
Somanna, N. K., Mani, I., Tripathi, S., and Pandey, K. N. (2018). Clathrin-dependent internalization, signaling, and metabolic processing of guanylyl cyclase/natriuretic peptide receptor-A. Mol. Cell Biochem. 441, 135–150. doi: 10.1007/s11010-017-3180-0
Somanna, N. K., Pandey, A. C., Arise, K. K., Nguyen, V., and Pandey, K. N. (2013). Functional silencing of guanylyl cyclase/natriuretic peptide receptor-A by microRNA interference: analysis of receptor endocytosis. Int. J. Biochem. Mol. Biol. 4, 41–53.
Sonnenberg, H., Honrath, U., Chong, C. K., and Wilson, D. R. (1986). Atrial natriuretic factor inhibits sodium transport in medullary collecting duct. Am. J. Physiol. 250, F963–F966.
Steinhelper, M. E., Cochrane, K. L., and Field, L. J. (1990). Hypotension in transgenic mice expressing atrial natriuretic factor fusion genes. Hypertension 16, 301–307. doi: 10.1161/01.hyp.16.3.301
Stevens, T. L., Burnett, J. C. Jr., Kinoshita, M., Matsuda, Y., and Redfield, M. M. (1995). A functional role for endogenous atrial natriuretic peptide in a canine model of early left ventricular dysfunction. J. Clin. Invest. 95, 1101–1108. doi: 10.1172/jci117757
Subramanian, U., Kumar, P., Mani, I., Chen, D., Kessler, I., Periyasamy, R., et al. (2016). Retinoic acid and sodium butyrate suppress the cardiac expression of hypertrophic markers and proinflammatory mediators in Npr1 gene-disrupted haplotype mice. Physiol. Genomics 48, 477–490. doi: 10.1152/physiolgenomics.00073.2015
Sudoh, T., Minamino, N., Kangawa, K., and Matsuo, H. (1988). Brain natriuretic peptide-32: N-terminal six amino acid extended form of brain natriuretic peptide identified in porcine brain. Biochem. Biophys. Res. Commun. 155, 726–732. doi: 10.1016/s0006-291x(88)80555-2
Suga, S., Itoh, H., Komatsu, Y., Ogawa, Y., Hama, N., Yoshimasa, T., et al. (1993). Cytokine-induced C-type natriuretic peptide (CNP) secretion from vascular endothelial cells–evidence for CNP as a novel autocrine/paracrine regulator from endothelial cells. Endocrinology 133, 3038–3041. doi: 10.1210/endo.133.6.8243333
Suga, S., Nakao, K., Itoh, H., Komatsu, Y., Ogawa, Y., Hama, N., et al. (1992). Endothelial production of C-type natriuretic peptide and its marked augmentation by transforming growth factor-beta. Possible existence of “vascular natriuretic peptide system”. J. Clin. Invest. 90, 1145–1149. doi: 10.1172/jci115933
Sugimoto, T., Kikkawa, R., Haneda, M., and Shigeta, Y. (1993). Atrial natriuretic peptide inhibits endothelin-1-induced activation of mitogen-activated protein kinase in cultured rat mesangial cells. Biochem. Biophys. Res. Commun. 195, 72–78. doi: 10.1006/bbrc.1993.2011
Suwa, M., Seino, Y., Nomachi, Y., Matsuki, S., and Funahashi, K. (2005). Multicenter prospective investigation on efficacy and safety of carperitide for acute heart failure in the ‘real world’ of therapy. Circ. J. 69, 283–290. doi: 10.1253/circj.69.283
Tamura, N., Doolittle, L. K., Hammer, R. E., Shelton, J. M., Richardson, J. A., and Garbers, D. L. (2004). Critical roles of the guanylyl cyclase B receptor in endochondral ossification and development of female reproductive organs. Proc. Natl. Acad. Sci. U.S.A. 101, 17300–17305. doi: 10.1073/pnas.0407894101
Tamura, N., Ogawa, Y., Chusho, H., Nakamura, K., Nakao, K., Suda, M., et al. (2000). Cardiac fibrosis in mice lacking brain natriuretic peptide. Proc. Natl. Acad. Sci. U.S.A. 97, 4239–4244. doi: 10.1073/pnas.070371497
Tamura, N., Ogawa, Y., Yasoda, A., Itoh, H., Saito, Y., and Nakao, K. (1996). Two cardiac natriuretic peptide genes (atrial natriuretic peptide and brain natriuretic peptide) are organized in tandem in the mouse and human genomes. J. Mol. Cell Cardiol. 28, 1811–1815. doi: 10.1006/jmcc.1996.0170
Testa, M., Yeh, M., Lee, P., Fanelli, R., Loperfido, F., Berman, J. W., et al. (1996). Circulating levels of cytokines and their endogenous modulators in patients with mild to severe congestive heart failure due to coronary artery disease or hypertension. J. Am. Coll. Cardiol. 28, 964–971. doi: 10.1016/s0735-1097(96)00268-9
Thaik, C. M., Calderone, A., Takahashi, N., and Colucci, W. S. (1995). Interleukin-1 beta modulates the growth and phenotype of neonatal rat cardiac myocytes. J. Clin. Invest. 96, 1093–1099. doi: 10.1172/jci118095
Tokudome, T., Kishimoto, I., Yamahara, K., Osaki, T., Minamino, N., Horio, T., et al. (2009). Impaired recovery of blood flow after hind-limb ischemia in mice lacking guanylyl cyclase-A, a receptor for atrial and brain natriuretic peptides. Arterioscler. Thromb. Vasc. Biol. 29, 1516–1521. doi: 10.1161/atvbaha.109.187526
Tomoda, H. (1988). Atrial natriuretic peptide in acute myocardial infarction. Am. J. Cardiol. 62, 1122–1123. doi: 10.1016/0002-9149(88)90561-9
Tremblay, J., Desjardins, R., Hum, D., Gutkowska, J., and Hamet, P. (2002). Biochemistry and physiology of the natriuretic peptide receptor guanylyl cyclases. Mol. Cell Biochem. 230, 31–47. doi: 10.1007/978-1-4615-0927-1_2
Tripathi, S., and Pandey, K. N. (2012). Guanylyl cyclase/natriuretic peptide receptor-A signaling antagonizes the vascular endothelial growth factor-stimulated MAPKs and downstream effectors AP-1 and CREB in mouse mesangial cells. Mol. Cell Biochem. 368, 47–59. doi: 10.1007/s11010-012-1341-8
Tsukagoshi, H., Shimizu, Y., Kawata, T., Hisada, T., Shimizu, Y., Iwamae, S., et al. (2001). Atrial natriuretic peptide inhibits tumor necrosis factor-alpha production by interferon-gamma-activated macrophages via suppression of p38 mitogen-activated protein kinase and nuclear factor-kappa B activation. Regul. Pept. 99, 21–29. doi: 10.1016/s0167-0115(01)00218-x
Tsukamoto, O., Fujita, M., Kato, M., Yamazaki, S., Asano, Y., Ogai, A., et al. (2009). Natriuretic peptides enhance the production of adiponectin in human adipocytes and in patients with chronic heart failure. J. Am. Coll. Cardiol. 53, 2070–2077. doi: 10.1016/j.jacc.2009.02.038
Tsutamoto, T., Kanamori, T., Morigami, N., Sugimoto, Y., Yamaoka, O., and Kinoshita, M. (1993). Possibility of downregulation of atrial natriuretic peptide receptor coupled to guanylate cyclase in peripheral vascular beds of patients with chronic severe heart failure. Circulation 87, 70–75. doi: 10.1161/01.cir.87.1.70
van den Akker, F., Zhang, X., Miyagi, M., Huo, X., Misono, K. S., and Yee, V. C. (2000). Structure of the dimerized hormone-binding domain of a guanylyl-cyclase-coupled receptor. Nature 406, 101–104. doi: 10.1038/35017602
Vandenwijngaert, S., Ledsky, C. D., Lahrouchi, N., Khan, M. A. F., Wunderer, F., Ames, L., et al. (2019). Blood pressure-associated genetic variants in the natriuretic peptide receptor 1 gene modulate guanylate cyclase activity. Circ. Genom. Precis. Med. 12, e002472.
Vanderheyden, M., Paulus, W. J., Voss, M., Knuefermann, P., Sivasubramanian, N., Mann, D., et al. (2005). Myocardial cytokine gene expression is higher in aortic stenosis than in idiopathic dilated cardiomyopathy. Heart 91, 926–931. doi: 10.1136/hrt.2004.035733
Vellaichamy, E., Das, S., Subramanian, U., Maeda, N., and Pandey, K. N. (2014). Genetically altered mutant mouse models of guanylyl cyclase/natriuretic peptide receptor-A exhibit the cardiac expression of proinflammatory mediators in a gene-dose-dependent manner. Endocrinology 155, 1045–1056. doi: 10.1210/en.2013-1416
Vellaichamy, E., Khurana, M. L., Fink, J., and Pandey, K. N. (2005a). Involvement of the NF-kappa B/matrix metalloproteinase pathway in cardiac fibrosis of mice lacking guanylyl cyclase/natriuretic peptide receptor A. J. Biol. Chem. 280, 19230–19242. doi: 10.1074/jbc.m411373200
Vellaichamy, E., Sommana, N. K., and Pandey, K. N. (2005b). Reduced cGMP signaling activates NF-kappaB in hypertrophied hearts of mice lacking natriuretic peptide receptor-A. Biochem. Biophys. Res. Commun. 327, 106–111. doi: 10.1016/j.bbrc.2004.11.153
Vellaichamy, E., Zhao, D., Somanna, N., and Pandey, K. N. (2007). Genetic disruption of guanylyl cyclase/natriuretic peptide receptor-A upregulates ACE and AT1 receptor gene expression and signaling: role in cardiac hypertrophy. Physiol Genomics 31, 193–202. doi: 10.1152/physiolgenomics.00079.2007
Villarreal, F. J., and Dillmann, W. H. (1992). Cardiac hypertrophy-induced changes in mRNA levels for TGF-beta 1, fibronectin, and collagen. Am. J. Physiol. 262, H1861–H1866.
Villrreal, D., and Freeman, R. H. (1997). “Natriuretic peptides and salt sensitivity,” in Contemporary Endocrinology: Natriuretic Peptides in Health and Disease, eds W. K. Samson and E. R. Levin (Totowa, NJ: Humana Press Inc), 239–258. doi: 10.1007/978-1-4612-3960-4_14
Vollmer, A. M. (2005). The role of atrial natriuretic peptide in the immune system. Peptides 26, 1087–1094.
Volpe, M. (2014). Natriuretic peptides and cardio-renal disease. Int. J. Cardiol. 176, 630–639. doi: 10.1016/j.ijcard.2014.08.032
Volpe, M., Battistoni, A., and Rubattu, S. (2019a). Natriuretic peptides in heart failure: current achievements and future perspectives. Int. J. Cardiol. 281, 186–189. doi: 10.1016/j.ijcard.2018.04.045
Volpe, M., Rubattu, S., and Battistoni, A. (2019b). ARNi: a Novel Approach to counteract cardiovascular diseases. Int. J. Mol. Sci. 20:2092. doi: 10.3390/ijms20092092
Volpe, M., Rubattu, S., and Burnett, J. Jr. (2014). Natriuretic peptides in cardiovascular diseases: current use and perspectives. Eur. Heart J. 35, 419–425. doi: 10.1093/eurheartj/eht466
von Geldern, T. W., Budzik, G. P., Dillon, T. P., Holleman, W. H., Holst, M. A., Ksio, Y., et al. (1990). Atrial natriuretic peptide antagonists Biological evaluation and structural correlations. Mol. Pharmacol. 38, 771–778.
Waldman, S. A., Rapoport, R. M., and Murad, F. (1984). Atrial natriuretic factor selectively activates particulate guanylate cyclase and elevates cyclic GMP in rat tissues. J. Biol. Chem. 259, 14332–14334. doi: 10.1016/s0021-9258(17)42597-x
Wang, D., Gladysheva, I. P., Fan, T. H., Sullivan, R., Houng, A. K., and Reed, G. L. (2014). Atrial natriuretic peptide affects cardiac remodeling, function, heart failure, and survival in a mouse model of dilated cardiomyopathy. Hypertension 63, 514–519. doi: 10.1161/hypertensionaha.113.02164
Wang, T. J. (2018). Natriuretic peptide deficiency-when there is too little of a good thing. JAMA Cardiol. 3, 7–9. doi: 10.1001/jamacardio.2017.4208
Wang, T. J., Larson, M. G., Keyes, M. J., Levy, D., Benjamin, E. J., and Vasan, R. S. (2007). Association of plasma natriuretic peptide levels with metabolic risk factors in ambulatory individuals. Circulation 115, 1345–1353. doi: 10.1161/circulationaha.106.655142
Wang, T. J., Larson, M. G., Levy, D., Benjamin, E. J., Leip, E. P., Wilson, P. W., et al. (2004). Impact of obesity on plasma natriuretic peptide levels. Circulation 109, 594–600. doi: 10.1161/01.cir.0000112582.16683.ea
Wang, Y., De Waard, M. C., Sterner-Kock, A., Stepan, H., Schultheiss, H. P., Duncker, D. J., et al. (2007). Cardiomyocyte-restricted over-expression of C-type natriuretic peptide prevents cardiac hypertrophy induced by myocardial infarction in mice. Eur. J. Heart Fail 9, 548–557. doi: 10.1016/j.ejheart.2007.02.006
Webber, M. A., and Marder, S. R. (2008). Better pharmacotherapy for schizophrenia: what does the future hold? Curr Psychiatry Rep 10, 352–358. doi: 10.1007/s11920-008-0056-8
Weber, M., and Hamm, C. (2006). Role of B-type natriuretic peptide (BNP) and NT-proBNP in clinical routine. Heart 92, 843–849. doi: 10.1136/hrt.2005.071233
Williams, B., Cockcroft, J. R., Kario, K., Zappe, D. H., Brunel, P. C., Wang, Q., et al. (2017). Effects of sacubitril/valsartan versus olmesartan on central hemodynamics in the elderly with systolic hypertension: the PARAMETER study. Hypertension 69, 411–420. doi: 10.1161/hypertensionaha.116.08556
Wu, C., Wu, F., Pan, J., Morser, J., and Wu, Q. (2003). Furin-mediated processing of Pro-C-type natriuretic peptide. J. Biol. Chem. 278, 25847–25852. doi: 10.1074/jbc.m301223200
Xue, H., Wang, S., Wang, H., Sun, K., Song, X., Zhang, W., et al. (2008). Atrial natriuretic peptide gene promoter polymorphism is associated with left ventricular hypertrophy in hypertension. Clin. Sci. (Lond) 114, 131–137. doi: 10.1042/cs20070109
Yandle, T. G., Richards, A. M., Nicholls, M. G., Cuneo, R., Espiner, E. A., and Livesey, J. H. (1986). Metabolic clearance rate and plasma half life of alpha-human atrial natriuretic peptide in man. Life Sci. 38, 1827–1833. doi: 10.1016/0024-3205(86)90137-2
Yasoda, A., Komatsu, Y., Chusho, H., Miyazawa, T., Ozasa, A., Miura, M., et al. (2004). Overexpression of CNP in chondrocytes rescues achondroplasia through a MAPK-dependent pathway. Nat. Med. 10, 80–86. doi: 10.1038/nm971
Yoshimura, M., Yasue, H., Morita, E., Sakaino, N., Jougasaki, M., Kurose, M., et al. (1991). Hemodynamic, renal, and hormonal responses to brain natriuretic peptide infusion in patients with congestive heart failure. Circulation 84, 1581–1588. doi: 10.1161/01.cir.84.4.1581
Zahabi, A., Picard, S., Fortin, N., Reudelhuber, T. L., and Deschepper, C. F. (2003). Expression of constitutively active guanylate cyclase in cardiomyocytes inhibits the hypertrophic effects of isoproterenol and aortic constriction on mouse hearts. J. Biol. Chem. 278, 47694–47699. doi: 10.1074/jbc.m309661200
Zhao, D., Das, S., and Pandey, K. N. (2013). Interactive roles of NPR1 gene-dosage and salt diets on cardiac angiotensin II, aldosterone and pro-inflammatory cytokines levels in mutant mice. J. Hypertens 31, 134–144. doi: 10.1097/hjh.0b013e32835ac15f
Zhao, D., Pandey, K. N., and Navar, L. G. (2010). ANP-mediated inhibition of distal nephron fractional sodium reabsorption in wild-type and mice overexpressing natriuretic peptide receptor. Am. J. Physiol. Renal. Physiol. 298, F103–F108.
Keywords: natriuretic peptides, natriuretic peptide receptors, gene-targeting, cardiovascular disorders, genetic mouse models
Citation: Pandey KN (2021) Molecular Signaling Mechanisms and Function of Natriuretic Peptide Receptor-A in the Pathophysiology of Cardiovascular Homeostasis. Front. Physiol. 12:693099. doi: 10.3389/fphys.2021.693099
Received: 09 April 2021; Accepted: 26 July 2021;
Published: 19 August 2021.
Edited by:
Amie Moyes, Queen Mary University of London, United KingdomReviewed by:
Marcelo Roberto Choi, Consejo Nacional de Investigaciones Científicas y Técnicas (CONICET), ArgentinaAlessandro Cataliotti, University of Oslo, Norway
Copyright © 2021 Pandey. This is an open-access article distributed under the terms of the Creative Commons Attribution License (CC BY). The use, distribution or reproduction in other forums is permitted, provided the original author(s) and the copyright owner(s) are credited and that the original publication in this journal is cited, in accordance with accepted academic practice. No use, distribution or reproduction is permitted which does not comply with these terms.
*Correspondence: Kailash N. Pandey, a3BhbmRleUB0dWxhbmUuZWR1