- 1Department of Neuroscience and Pharmacology, University of Iowa Carver College of Medicine, Iowa City, IA, United States
- 2Department of Physiology, Medical College of Wisconsin, Milwaukee, WI, United States
- 3Department of Biomedical Engineering, Medical College of Wisconsin, Milwaukee, WI, United States
- 4Comprehensive Rodent Metabolic Phenotyping Core, Medical College of Wisconsin, Milwaukee, WI, United States
- 5Iowa Neuroscience Institute, University of Iowa Carver College of Medicine, Iowa City, IA, United States
- 6FOE Diabetes Research Center, University of Iowa Carver College of Medicine, Iowa City, IA, United States
- 7Obesity Research and Educational Initiative, University of Iowa Carver College of Medicine, Iowa City, IA, United States
Obesity is commonly associated with sympathetic overdrive, which is one of the major risk factors for the development of cardiovascular diseases, such as hypertension and heart failure. Over the past few decades, there has been a growing understanding of molecular mechanisms underlying obesity development with central origin; however, the relative contribution of these molecular changes to the regulation of cardiovascular function remains vague. A variety of G-protein coupled receptors (GPCRs) and their downstream signaling pathways activated in distinct hypothalamic neurons by different metabolic hormones, neuropeptides and monoamine neurotransmitters are crucial not only for the regulation of appetite and metabolic homeostasis but also for the sympathetic control of cardiovascular function. In this review, we will highlight the main GPCRs and associated hypothalamic nuclei that are important for both metabolic homeostasis and cardiovascular function. The potential downstream molecular mediators of these GPCRs will also be discussed.
Introduction
Obesity, a pathological state characterized by an excessive accumulation of body fat resulting from a chronic positive energy balance, continues to be a major global public health problem leading to an enormous economic burden. Obese adults are at an elevated risk of developing comorbidities including type II diabetes mellitus and hypertension (Flegal et al., 2010). Despite decades of effort dedicated to mitigating the increasing obesity prevalence worldwide, the World Health Organization (WHO) estimated that approximately 39% of the global population is now either overweight or obese (Gonzalez-Muniesa et al., 2017). In addition to diet and lifestyle modifications, the development of efficacious and safe anti-obesity pharmaceuticals remains an important weapon against the obesity pandemic. Evidence from human genome-wide association studies (GWAS) and experimental data from animal models have identified several potential candidates for anti-obesity therapeutics, including signaling molecules comprising a class of cell surface receptors known as G-Protein Coupled Receptors (GPCRs). GPCRs are important drug targets, as approximately 34% of all Food and Drug Administration (FDA) approved drugs are targeted against these receptors (Hauser et al., 2018).
G-Protein Coupled Receptors are seven-pass transmembrane domain cell surface receptors that initiate downstream signaling cascades upon binding to designated ligands, which ultimately translate into a specific adaptive cellular response. The type of response evoked by an individual ligand is dictated by the coupling of activated GPCR to distinct downstream G protein(s). G proteins are heterotrimeric guanine nucleotide-binding complexes which function as signal transducers to relay and amplify extracellular signals from ligand-activated GPCRs. These heterotrimeric complexes are composed of three subunits: Gα, Gβ, and Gγ. There are four major subtypes of Gα (Gαq/11, Gαi, Gαs, and Gα12), five different subtypes of Gβ and twelve Gγ subunits (Smrcka, 2008; Kostenis et al., 2020). GPCR activation results in the exchange of guanosine-diphosphate (GDP) to guanosine-triphosphate (GTP) on the Gα subunit, which promotes the dissociation of the Gα and Gβ γ complexes to initiate downstream signaling. The Gαq subunit signals through calcium and protein kinase C (PKC) signaling pathways by activating phosphoinositide phospholipase C beta (PLC-β), whereas Gαs stimulates and Gαi inhibits cyclic adenosine monophosphate (cAMP)-dependent signaling by modulating adenylyl cyclases activity (Neves et al., 2002). In addition to signaling by the Gα subunits, the Gβ γ subunits also appear to be capable of transducing downstream signals, further diversifying the intracellular responses initiated by a GPCR (Senarath et al., 2018; Smrcka and Fisher, 2019).
GPCR signal transduction can be regulated by Regulators of G-protein Signaling (RGS) proteins as well as β-arrestins. RGS proteins function as terminators of G protein signaling by enhancing the intrinsic GTPase activity of Gα subunits to facilitate the GTP to GDP hydrolysis, thereby promoting the reassociation between the Gα and Gβ γ subunits (Perschbacher et al., 2018). Thus, RGS proteins act to modulate the duration and subsequent termination of activated GPCR signaling. An additional layer of GPCR signaling regulation is provided by scaffold proteins such as β-arrestins, which include two subtypes, β-arrestins-1 and -2 and G protein-coupled receptor kinases (GRKs) (Lohse et al., 1990; Attramadal et al., 1992; Lymperopoulos, 2018; Chaudhary and Kim, 2021). Upon ligand induced receptor activation, GRKs are recruited to and phosphorylate activated GPCRs, subsequently allowing for β-arrestins to bind and promote the termination of further G-protein mediated signaling by inducing receptor internalization as well as potentially forming a signaling complex with the mitogen-activated protein kinases ERK1/2 to transduce ligand-specific downstream signaling (Tian et al., 2014; Eishingdrelo et al., 2015). While both β-arrestin-1 and β-arrestin-2 share a high degree of sequence and structural similarity and exhibit similar expression and localization patterns, increasing studies have shed light on functional distinction between these two β-arrestin subtypes [reviewed in Srivastava et al. (2015)]. Multiple lines of evidence from human genetic analysis as well as experimental data implicated critical roles for G proteins signaling in cardiometabolic regulation. For example, it has been reported that patients with single nucleotide polymorphisms (SNPs) in the gene encoding Gαs (GNAS) are associated with increased risk of sudden cardiac death, whereas loss-of-function mutations within GNAS are associated with early-onset obesity (Wieneke et al., 2016; Hanna et al., 2018). Furthermore, mice deficient for Gαs or Gαq/11 specifically in the dorsomedial hypothalamus (DMH) develop obesity (Chen et al., 2019; Wilson et al., 2021). Thus, functionally intact G protein signaling is essential for cardiometabolic homeostasis.
In this review, we will highlight several clinically targeted GPCRs located within the hypothalamus with well- described roles in cardiometabolic control and explore the potential G protein signaling cascade(s) these GPCRs employ to exert these cardiometabolic effects.
Hypothalamic MC4R Signaling Pathways in Cardiometabolic Regulation
The melanocortin 4 receptor (MC4R) is a highly conserved GPCR widely expressed in the central nervous system (CNS), including the cortex, hippocampus, amygdala, hypothalamus, brainstem, and spinal cord, and the highest expression of MC4R has been observed in the paraventricular nucleus of hypothalamus (PVN) among other brain nuclei (Kishi et al., 2003; Liu et al., 2003; Staubert et al., 2007). PVNMC4R neurons receive dense innervation from two distinct and counteracting populations of neurons in the arcuate nucleus of hypothalamus (ARC): catabolic proopiomelanocortin (POMC)-expressing neurons and anabolic agouti-related peptide (AgRP)-expressing neurons (Garfield et al., 2009; Gautron et al., 2015). Functionally, ARCPOMC neurons release MC4R agonist alpha-melanocyte stimulating hormone (α-MSH), leading to reduced food intake and increased energy expenditure (Kim et al., 2000; Atasoy et al., 2012; Zhan et al., 2013; Dodd et al., 2015), whereas ARCAgRP neurons release the MC4R inverse agonist AgRP, leading to the opposite physiological outcomes (Aponte et al., 2011; Atasoy et al., 2012). MC4R mutations represent the most prevalent form of monogenic obesity (Farooqi et al., 2003) and both animal models and human patients carrying loss-of-function MC4R mutations exhibit early-onset obesity caused by hyperphagia and reduced energy expenditure (Bolze and Klingenspor, 2009; Kuhnen et al., 2019; Rene et al., 2021).
Further functional dissection of MC4R signaling in different brain regions has been achieved through conditional Cre-loxP recombination techniques. For example, the necessity and sufficiency of PVNMC4R neurons in the regulation of feeding and body weight have been demonstrated through AAV-Cre-mediated postnatal deletion of MC4Rs specifically in the PVN of MC4RloxP/loxP and reactivatable MC4RloxTB/loxTB mice. AAV-Cre-mediated deletion of PVNMC4R leads to hyperphagia and obesity (Garza et al., 2008), while PVN-specific MC4R re-expression markedly suppresses hyperphagia and body weight gain in obese MC4R-null (MC4RloxTB/loxTB) mice (Shah et al., 2014). Furthermore, the Sim1-Cre-mediated selective restoration of MC4R expression in the PVN of obese MC4R-null mice normalizes food intake and reduces body weight by ∼60% (Balthasar et al., 2005; Rossi et al., 2011; Sohn et al., 2013). Addtionally, AAV-Cre injection into the DMH of MC4RloxP/loxP mice also significantly increased body weight likely due to decreased energy expenditure (Chen et al., 2017). Functional circuit mapping revealed that PVNMC4R neurons make functional synaptic connections to the lateral parabrachial nucleus (LPBN) and optogenetic stimulation of PVNMC4RLPBN terminals inhibit feeding, indicating an important role of PVNMC4R-LPBN pathway in appetite regulation (Shah et al., 2014; Garfield et al., 2015). On the other hand, MC4R regulates energy expenditure and glucose metabolism through other distinct pathways. Previous retrograde trans-synaptic neuronal tracing studies have shown that interscapular brown adipose tissue (iBAT) is innervated by sympathetic premotor neurons that express MC4R in PVN, brain stem and intermediolateral cell column (IML) (Voss-Andreae et al., 2007; Song et al., 2008; Kooijman et al., 2014). Selective restoration of MC4R expression in cholinergic neurons of obese MC4RloxTB/loxTB mice not only partially ameliorate obesity but also improve glucose homeostasis (Rossi et al., 2011; Sohn et al., 2013). We have previously shown that AAV-Cre-mediated specific re-expression of lateral hypothalamic area (LHA) MC4R signaling improves glucose tolerance in obese MC4RloxTB/loxTB without affecting body weight (Morgan et al., 2015). The improved glucose uptake in these animals was mainly driven by elevated iBAT glucose uptake, accompanied by significantly increased sympathetic traffic to iBAT (Morgan et al., 2015). These studies underscore an important role of hypothalamic MC4R signaling in the regulation of metabolic homeostasis.
Hypothalamic MC4R signaling is also involved in the control of sympathetic outflow affecting cardiovascular function. While obesity is commonly associated with sympathetic overactivity and elevated blood pressure (BP), severely obese MC4R knockout mice exhibit normal to reduced sympathetic nerve activity (SNA) when compared to their lean wild-type littermates (Tallam et al., 2005). Conversely, chronic MC4R activation causes sustained increases in BP that can be prevented by adrenergic receptor blockade (Kuo et al., 2004). The importance of MC4R in modulating SNA and BP is further supported by observations in humans that severely obese individuals due to genetic MC4R deficiency exhibit lower BP and reduced 24-h Norepinephrine (NE) excretion compared to obese individuals with normal MC4R function (Maier and Hoyer, 2010). Morgan et al. (2015) PVN is one of the integrative centers for sympathetic cardiovascular control through its descending projections to the rostral ventrolateral medulla (RVLM) and spinal cord (Stocker et al., 2013). Microinjection of a synthesized MC4R agonist, melanotan II (MTII, an α-MSH analog), directly into PVN increases SNA and BP in an SNA- and MC4R-dependent manner (Kuo et al., 2003, 2004). In addition, sympathetic response to insulin also requires PVNMC4R signaling and activation of RVLM neurons (Ward et al., 2011), and MC4R agonists depolarize RVLM-projecting pre-sympathetic PVN neurons (Ye and Li, 2011) which have been implicated in obesity-associated hypertension (Stocker et al., 2007). Moreover, pharmacological inhibition of MC4R restores normal BP in spontaneously hypertensive rats (SHR) with elevated SNA (da Silva et al., 2008). Consistent with these observations, our recent comprehensive anterograde tract-tracing revealed that PVNMC4R neurons densely innervate the ventrolateral medulla (VLM) and thoracic spinal cord (TSC) that are critical for sympathetic activation, and chemogenetic activation of PVNMC4R neurons not only suppresses feeding but also elevate BP and iBAT thermogenesis (Singh et al., 2021). These observations suggest the existence of distinct MC4R neuronal circuits for the regulation of different aspects of cardiometabolic homeostasis. Further fine-mapping of functional circuits is needed for a better understanding of hypothalamic MC4R signaling pathways and their physiological impact.
The classical signaling pathway for the MC4R is by coupling to the heterotrimeric stimulatory G protein (Gαs) which activates adenylyl cyclase to convert ATP to cAMP (Breit et al., 2011; Podyma et al., 2018). However, among all identified MC4R variants, only few have shown impaired cAMP level (Vollbach et al., 2017), which strongly suggests the existence of other signaling pathways. Indeed, more recent studies using the mouse hypothalamic cell line GT1-1 identified that MC4R can increase intracellular Ca2+ through the Gαq/phospholipase C (PLC)-dependent signaling pathway (Newman et al., 2006). In another mouse hypothalamic cell line, GT1-7, α-MSH-induced Gα protein activation (by incorporation of GTPγS35) can be partially blocked by the Gαi inhibitor pertussis toxin (PTX), while the efficacy of α-MSH to induce cAMP production increased by 53%, indicating activation of both Gαs and Gαi signaling pathways (Buch et al., 2009). Interestingly, AgRP, which promotes feeding and suppresses energy expenditure when administered centrally (Small et al., 2001, 2003), was found to selectively activate Gαi signaling in these GT1-7 cells (Buch et al., 2009). It was recently reported that mice with homozygous Gαs deficiency in MC4R-expressing cells developed obesity due to both increased food intake and decreased energy expenditure, and the ability of the MC4R agonist MTII to stimulate energy expenditure as well as to inhibit food intake was significantly impaired in these animals (Podyma et al., 2018). Another recent publication demonstrated that the inhibitory effect of PVN-targeted microinjection of MTII on food intake is lost in mice lacking Gαq/11, but not Gαs, specifically in the PVN (Li et al., 2016). Interestingly, the BP response to MTII was absent only in animals lacking Gαs specifically in the PVN (Li et al., 2016). In line with these findings, setmelanotide, a recently FDA-approved MC4R agonist, exhibits clinically significant efficacy in reducing food intake and body weight without affecting BP, likely due to biased Gαq activation (Collet et al., 2017). Using a HEK293 cell-based system that incorporated a reporter gene, it is suggested that setmelanotide was about 80 times more potent than α-MSH for Gαq/PLC activity (Clement et al., 2018). Furthermore, extracellular signal-regulated kinase 1/2 (ERK1/2) activation is also identified in cells expressing MC4R, which is thought to be involved in metabolic regulation. ERK1/2 activation through MC4R seems depending on different cellular contexts: NDP-α-MSH (an α-MSH analog) induces ERK1/2 activation via Gαi protein in HEK293 cells stably expressing MC4Rs, but through Ca2+/PKC pathway in GT1–1 cells with endogenous MC4R expression (Chai et al., 2006). Interestingly, several MC4R variants with normal cell surface expression, ligand binding and cAMP production are found to be ERK1/2 defective, which could account for the obese phenotype in these patients (He and Tao, 2014). These results are further supported by a recent publication that a gain-of-function human MC4R variant shows a signaling bias toward β-arrestin two recruitment and increased ERK1/2 activity (Lotta et al., 2019). These variants are protective against obesity and obesity-related cardiovascular diseases (Lotta et al., 2019). More studies are still needed to identify the in vivo MC4R-Gα coupling in different hypothalamic nuclei, the physiological outcome of different MC4R-Gα coupling, and the mechanisms behind biased MC4R signaling activation (Figure 1).
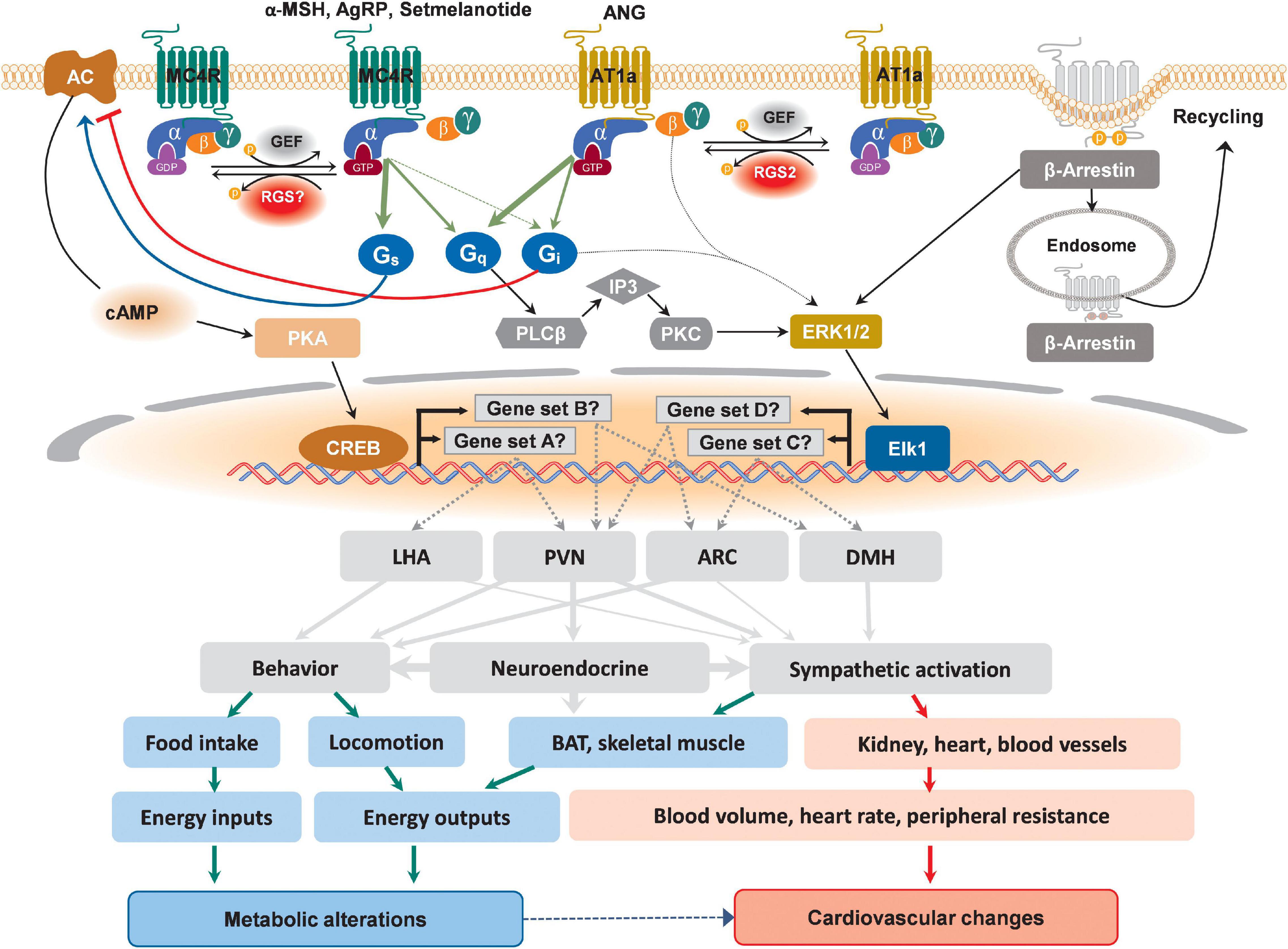
Figure 1. Schematic depicting possible divergent signaling cascades downstream of MC4R and AT1a in distinct hypothalamic nuclei that might differentially affect metabolic and cardiovascular regulations. Upon binding to its designated ligands, MC4R activates canonical Gαs-AC-cAMP-PKA-CREB pathway, leading to the transcription of genes in different hypothalamic nuclei. Additionally, the activation of non-cannonical signaling pathways involving Gαq and Gαi has also been reported for MC4R, which lead to the activation of ERK-Elk1 transcriptional axis to drive the expression of different sets of responsive genes in different hypothalamic nuclei. Differential recruitment of these divergent signaling pathways may defines ultimate physiological changes. For AT1a, although reported canonical pathway is Gαq-mediated activation of ERK, a possible non-canonical coupling to Gαi has also been supported experimentally, which, depending on the action of site, may lead to differential metabolic and cardiovascular outcomes. In addition to well-known receptor internalization for subsequent recycling or degradation, β-Arrestin-mediated activation of ERK transduction has also been reported for both MC4R and AT1a. These multilevel selectivities of GPCR signaling pathways ranging from intracellular molecular events to the brain regions of action and the effective organs could all lead to different metabolic alterations and cardiovascular changes.
The Brain Renin-Angiotensin System and the AT1A Receptor in Cardiometabolic Control
The renin-angiotensin system (RAS) is a hormone system that plays an essential role in the regulation of fluid and electrolyte balance, blood volume, systemic vascular constriction and BP, thereby establishing the RAS as a major controller of the cardiovascular system. The RAS exists as both a circulating hormone system as well as a localized, tissue-specific paracrine/autocrine/intracrine signaling system in organs such as the brain and adipose tissue. Angiotensin II (ANG), the canonical effector of the RAS, is the byproduct of angiotensinogen through a series of sequential enzymatic cleavages. ANG exerts its effect through the binding and activation of two known GPCRs – the angiotensin type I receptor (AT1, encoded by the AGTR1 gene) and the angiotensin type II receptor (AT2, encoded by the AGTR2 gene). Unlike humans, there are two AT1 isoforms in rodents - AT1a and AT1b, encoded by Agtr1a and Agtr1b, respectively (Elton et al., 1992; Iwai and Inagami, 1992; Sandberg et al., 1992). Early studies found that AT1a is critical for the BP response effects of the brain RAS, whereas the AT1b receptor is involved in the dipsogenic effects of central ANG actions (Davisson et al., 2000). Blockade of AT1a and AT1b receptors with the antagonist losartan in the paraventricular nucleus (PVN) prevents the reflex reduction in renal blood flow induced by elevated core body temperature, underscoring this receptor as a critical mediator of cardiovascular thermoregulatory responses (Chen et al., 2011).
In addition to the well-defined roles of the RAS in cardiovascular control, there is increasing evidence demonstrating a multimodal role for this hormone system in energy homeostasis, in part through affecting resting metabolic rate (RMR) (Littlejohn and Grobe, 2015). Interestingly, differential engagement of the AT1a and AT2 receptors by ANG elicits opposing actions on RMR, as brain AT1a activation increases RMR to stimulate energy expenditure, whereas adipocyte-specific activation of AT2 results in RMR suppression through inhibition of the beiging/browning process and thereby thermogenic capacity of these cells (Grobe et al., 2011; Littlejohn et al., 2016). The brain RAS is required for RMR control in response to multiple stimuli including high fat diet, leptin, and deoxycorticosterone acetate (DOCA)-salt (Grobe et al., 2011; Claflin et al., 2017). In addition to brain regions implicated in cardiovascular control such as the subfornical organ (SFO), supraoptic nucleus (SON), rostral ventrolateral medulla (RVLM) and organum vasculosum of the lamina terminalis (OLVT), AT1a expression is also observed in a subset of neurons expressing agouti-related peptide (Agrp) within the arcuate nucleus (ARC) of the hypothalamus, a region critical for the homeostatic regulation of energy balance by integrating the actions of peripheral metabolic hormones, such as leptin, insulin and ghrelin (Claflin et al., 2017; Sapouckey et al., 2017; Morselli et al., 2018; Sandgren et al., 2018; Deng and Grobe, 2019). Several studies have purported critical roles for intact AT1a signaling within the brain to mediate thermogenic SNA and RMR responses. For example, disruption of AT1a signaling by (1) central AT1a blockade by the AT1a receptor antagonist losartan, (2) whole-body Agtr1a knockout, and (3) inhibition of angiotensin-converting-enzyme (ACE, which is required to generate ANG) all resulted in abrogation of thermogenic SNA to acute leptin injections (Hilzendeger et al., 2012). Furthermore, we recently demonstrated that genetic deletion of AT1a specifically from either leptin receptor (LepR)- or agouti-related protein (AgRP)-expressing cells abolish the thermogenic SNA and RMR responses to leptin, high-fat diet (HFD), and deoxycorticosterone acetate (DOCA)-salt (Claflin et al., 2017). Given that pharmacological inhibition of the brain RAS diminishes BP responses to these stimuli, it was surprising that genetic disruption of AT1a signaling in LepR-expressing cells did not alter BP responses to DOCA-salt. In addition, AgRP neurons are undoubtedly implicated in the control of feeding behavior, yet AT1a deletion in LepR- or AgRP-expressing cells did not affect food intake. The PVN is an integrative center coordinating neural outputs for both metabolic and cardiovascular control and AT1a is enriched in PVN neurodendocrine neurons expressing corticotropin-releasing hormone (CRH) (Aguilera et al., 1995; Hurt et al., 2015). Others have demonstrated that the loss of AT1a signaling in the PVN does not alter baseline body weight and BP; however, these animals exhibit exaggerated weight gain on HFD challenge and blunted ANG- and stress-induced hypertensive responses, underscoring the importance of PVNAT1a signaling in physiological responses to metabolic and behavioral challenges (Northcott et al., 2010; Fan et al., 2012; de Kloet et al., 2013; Wang et al., 2016). Collectively, these observations support a role for hypothalamic AT1a signaling in differential regulation of BP and body weight through mechanisms that differ based upon the specific cellular localization of AT1a (Haynes et al., 1999).
AT1a activation has been reported to be coupled to multiple Gα proteins, including Gαq, Gα12/13, and Gαi, to transduce downstream second messenger signaling (Figure 1; Shirai et al., 1995; Shibata et al., 1996). This GPCR-Gα coupling appears to be both tissue- and cell-type-dependent, which determines the molecular, cellular, and physiological outputs of AT1a activation. For example, in vascular smooth muscle cells, AT1a is coupled to both Gαq/11 and Gα12/13 (Harris et al., 2007; Wirth et al., 2008). Furthermore, Sauliere and colleagues employed bioluminescence resonance energy transfer biosensors to demonstrate that ANG and the biased agonist [1Sar4Ile8Ile]-angiotensin II mediate the coupling of AT1a to Gαq/11 and Gαi in HEK293T cells expressing exogenous AT1a as well as in primary cardiac fibroblasts with endogenous AT1a receptors (Sauliere et al., 2012). Rgs2, one of RGS family proteins, is a potent negative regulator of AT1a signaling in various cell types, and roles for Rgs2 and Gαi2 in the modulation of energy homeostasis have been suggested from observations that mice lacking an RGS-sensitive Gαi2 allele displayed increased energy expenditure, whereas Rgs2-null mice are resistant to weight gain (Huang et al., 2008; Matsuzaki et al., 2011; Nunn et al., 2011). Given that Rgs2 is reported to be a regulator of AT1a signaling which couples to Gαi and that the expression of all three of these molecules is observed in a subset of AgRP-neurons, it has been hypothesized that the AT1a-Gαi2-Rgs2 signaling cascade within Agrp neurons is critical for the RMR stimulating effects but not the BP promoting effects of ANG [reviewed in Deng and Grobe (2019)]. Upon activation by its ligand, both β-arrestin 1 and β-arrestin 2 are recruited to AT1a receptors (Sanni et al., 2010). The central actions of β-arrestins in regulating energy homeostasis remain underexplored. However, a recent study by Pydi and colleagues indicated a beneficial metabolic role for central β-arrestin 1, as selective loss of β-arrestin 1 in AgRP neurons exhibited impaired glucose tolerance and insulin sensitivity when fed a high-fat diet (Pydi et al., 2020). Further, while GRKs, such as GRK2 and GRK5, have been reported to mediate ANG-induced AT1a receptor desensitization in tissue specific manner, the roles of central GRK play in maintaining energy balance through the AT1a signaling cascade are still poorly studied (Haack et al., 2012; Pollard et al., 2020).
Adverse sympathoexcitatory and hypertensive effects of MC4R agonists, such as LY2112688, have profoundly limited the therapeutic use and curtailed further development of first-generation MC4R agonists for obesity treatment (Greenfield et al., 2009; do Carmo et al., 2017). Thus, when considering central AT1a signaling as a potential target for anti-obesity therapeutics, it is important to underscore that ANG may activate divergent signaling pathways and/or engage various neural circuits to differentially control RMR and BP, as mice lacking AT1a in AgRP neurons failed to display an increase in RMR in response to HFD or DOCA-salt treatments while keeping BP response intact (Claflin et al., 2017). This biased agonism of the AT1a receptor confers potential anti-obesity treatment through stimulating RMR with minimal cardiovascular side effects. While AT1a expression has been observed in hypothalamic nuclei implicated in both cardiovascular and metabolic regulations, including the ARC, PVN, SON, and dorsal medial hypothalamus (DMH), the functional roles of these AT1a-expressing neurons and underlying neural circuits mediating cardiometabolic control remain largely underexplored and continue to be an active area of research.
Serotonin and Hypothalamic Serotonergic Receptors in Cardiometabolic Regulation
The neurotransmitter serotonin (5-hydroxytryptamine; 5-HT) has broad physiological effects including modulating moods and promoting satiety (Yabut et al., 2019). These diverse effects are mediated by a myriad of 5-HT receptors and receptor subtypes expressed in mammals. There are seven known 5-HT receptor families, 5-HT1–7, all of them are GPCR, except for the 5-HT3 receptor, which is a ligand-gated Na+ and K+ cation channel (Nichols and Nichols, 2008). Due to the inability of 5-HT to pass through the blood-brain barrier, its synthesis must occur locally within the brain, using the amino acid tryptophan as a precursor molecule (Boadle-Biber, 1993). Early evidence supporting a role for 5-HT in food intake modulation comes from observations that (1) the anti-serotonergic drug cyproheptadine stimulates appetite in human as well as in mice and (2) treatment with the 5-HT releasing agent, fenfluramine, causes significant weight loss in obese patients (Bergen, 1964; Guy-Grand, 1995). However, follow-up studies on patients who had been treated with fenfluramine revealed adverse cardiovascular effects, with an increased risk for developing primary pulmonary hypertension (PPH) (Brenot et al., 1993; Souza et al., 2008). Given the expansive physiological effects 5-HT has and the diverse set of receptors it can engage, it is not unexpected that the fenfluramine confers some adverse side effects and underscores the need to critically examine the various functions mediated by each 5-HT receptor subtype. Indeed, it has been reported that the fenfluramine metabolite, (+)-norfenfluramine, induces vasoconstriction and pressor effects through the 5-HT2A receptor, suggesting that PPH incidences observed in fenfluramine treated patients may be due to inadvertent activation of this 5-HT receptor subtype (Ni et al., 2007).
The satiety-promoting effects of 5-HT are mediated, in part, by the 5-HT1B and 5-HT2C receptors expressed in neurons of the ARC (Makarenko et al., 2002; Xu et al., 2008). Activation of 5-HT2C by selective agonist BVT.X resulted in decreased acute food intake in both genetic and diet-induced models of obesity in mice, and this appetite-suppressing effect is upstream of the melanocortin pathway, as mice deficient in the melanocortin 3 (MC3)/MC4 receptors do not exhibit food intake reduction effects induced by 5-HT2C agonism (Lam et al., 2008). On the other hand, mice with 5-HT1B receptor deletion exhibit increased weight gain and pharmacological activation of this receptor by the receptor agonist CP-94,253 inhibits food intake (Lee and Simansky, 1997; Lucas et al., 1998). Additional studies revealed that the 5-HT2C receptor is coupled to Gαq/11 whereas the 5-HT1B receptor is Gαi/o coupled [reviewed in Hoyer et al. (1994)]. Thus, engagement of different G protein coupling by these two serotonin receptors within the same hypothalamic nuclei appears to promote the satiety effects. However, additional studies are warranted to determine whether these two receptors signaling pathways occur within the same cell or different cellular populations of the ARC. The therapeutic potential of 5-HT2C signaling pathway was highlighted by the clinical use of lorcaserin, a selective 5-HT2C agonist approved by the Federal Drug Administration (FDA) in 2012 for the treatment of obesity. While initial results from Phase 2 trials hinted participants taking the drug may be at increased risk of cardiac fibrosis, subsequent analysis concluded that lorcaserin exhibited a similar cardiovascular safety profile to that of placebo (Bohula et al., 2018). However, subsequent assessment of patients taking lorcaserin revealed a higher incidence of cancer diagnosis, prompting the FDA to request the manufacturer to withdraw the drug from the United States market (Sharretts et al., 2020). In contrast to the 5-HT2C and 5-HT1B receptors, whereby the hypophagic effects of serotonin were mediated by agonism of these receptors, it is the antagonism of the 5-HT6 receptor that promotes weight loss. Indeed, mice carrying a non-functional mutant 5-HT6 receptor are partially protected from high-fat induced obesity due to decreased food intake (Frassetto et al., 2008). Furthermore, RNAi-mediated central knockdown of 5-HT6 receptor signaling similarly results in weight loss and reduced food intake as observed in 5-HT6 null mice (Woolley et al., 2001). Within the hypothalamus, the Gαs-coupled 5-HT6 receptor is expressed in cells of the PVN, VMH, and ARC (Roberts et al., 2002; Fisas et al., 2006). Consistent with previous studies demonstrating a critical role for the 5-HT6 receptor antagonism in promoting satiety, pharmacological inhibition of 5-HT6 receptor-mediated signaling with the receptor antagonist SB-399885 reduced food intake in rats, in concurrent with increased neuronal activation in cells located in the PVN and the nucleus tractus solitarius (NTS) (Garfield et al., 2014).
A recent study employing single-cell RNA-sequencing identified eleven transcriptomically distinct serotonin neurons of the dorsal and median raphe nuclei, where a subset of these serotonin neuronal clusters co-expressing thyrotropin-releasing hormone innervate the hypothalamus (Ren et al., 2019). However, the neuroanatomical basis of serotonin-sensing neurons located within hypothalamic nuclei that mediate appetite control remains largely underexplored. Given the diverse physiological responses mediated by serotonin as relayed by a vast receptor superfamily, it is essential to delineate the contribution of each serotonin receptor subtype and the relevant neurocircuitry to more effectively develop anti-obesity therapeutics while minimizing unwanted side effects.
Hypothalamic α-Adrenergic Receptors in Cardiometabolic Regulation
Norepinephrine is an important neurotransmitter that regulates a variety of CNS functions, including cardiovascular responses, neuroendocrine homeostasis, feeding and wakefulness (Shor-Posner et al., 1985; Camargo and Saad, 2001; Smythies, 2005; Mallick and Singh, 2011; Berridge et al., 2012). These actions of NE are mediated by adrenergic receptors (ARs), which can be divided into three types (α1, α2, and β) and nine subtypes (α1A–C, α2A–C, and β1–3) (Strosberg, 1993). Upon ligand binding, α1-ARs induce depolarization via Gαq-mediated increase in intracellular Ca2+ levels and closure of G protein-coupled inwardly rectifying potassium channels (GIRKs), while α2-ARs induce hyperpolarization via Gαi-mediated inhibition of voltage-gated Ca2+ channels (VGCCs) and opening of GIRKs (Hein, 2006). On the other hand, β-ARs activation is excitatory, which is mediated by Gαs-coupled signaling cascade [reviewed in Marzo et al. (2009)]. The intracellular regulators of AR signaling has been identified recently. Among these components, GRK2 was found to be significant for SNA-mediated cardiovascular homeostasis. It was previously shown that adrenal catecholamine production is tightly regulated by GRK2-controlled α2-AR signaling, providing potential mechanism of SNS hyperactivation under pathological conditions like heart failure (Lymperopoulos et al., 2007; Jafferjee et al., 2016). Whether similar mechanisms exists in hypothalamic ARs, remains to be further investigated.
In the hypothalamus, α1 and α2, especially subtype α1A and α2A are the two most abundantly expressed ARs and both are involved in the regulation of cardiometabolic control (Scheinin et al., 1994; Day et al., 1997). Early histochemical analysis revealed that the medial hypothalamus is densely innervated by ascending NE projections from the brainstem (Leibowitz and Brown, 1980). However, the distribution of AR-expressing cells in the hypothalamus remain largely unclear, mostly due to the lack of available AR subtype-specific transgenic Cre lines. Recently, a novel adrenergic NTS-ARC pathway modulating food intake was identified (Aklan et al., 2020; Chen et al., 2020). It is reported that NTS tyrosine hydroxylase (TH)-expressing neurons densely innervate the ARC, and activation of NTSTH-ARC fibers promote feeding via NE release onto ARCAgRP neurons that express α1 AR (Aklan et al., 2020). Despite the limited information of neural circuits consisting of AR-expressing neurons, the development of selective pharmacological agonists/inhibitors has greatly expanded our understanding of the physiological roles of hypothalamic α1 and α2 signaling pathways. Microinjection of α2 agonists into PVN elicits feeding, while α1 agonist injection suppresses food intake (Goldman et al., 1985; Wellman and Davies, 1991b). Consistent with the agonist studies, it is documented that PVN-specific inactivation of α1-AR signaling abolished the feeding suppression induced by systemic injection of α1 agonist (Wellman and Davies, 1991a). Moreover, systemic and PVN-specific injection of α2 antagonists suppressed food intake (Alexander et al., 1993). These findings not only demonstrate that PVN α1 and α2-ARs are involved in the regulation of feeding, but also suggest potential intracellular antagonistic mechanisms between these two receptors. In line with this working model, brain slice electrophysiological recordings of NE-responsive PVN neurons revealed that the excitatory effect of NE is mimicked by α1 agonist phenylephrine (PHE), while the inhibitory effect of NE is mimicked by α2 agonist clonidine (Wellman et al., 1993). Similar co-localization and antagonistic actions are observed in other brain regions, including NTS, DMV and VMH, suggesting that this mutual antagonistic mode of action of α1 and α2-AR is generalized across different brain regions (Young and Kuhar, 1980; Saad et al., 1984; Feldman and Felder, 1989). Antagonistic action between α1 and α2 receptors on feeding is versatile and tightly regulated. Early microdialysis studies revealed that extracellular NE within the PVN peaked at the beginning of the dark cycle, accompanied by a sharp rise in α2-AR expression (Bhakthavatsalam and Leibowitz, 1986; Jhanwar-Uniyal et al., 1986; Morien et al., 1995). In addition, this elevation of NE was significantly correlated with eating onset (Bhakthavatsalam and Leibowitz, 1986). Though it is known that α1A and α2A are the most abundant subtypes in the hypothalamus, the physiological roles of different α1 and α2 subtypes remain to be further investigated. A recent study using cell type-specific transcriptomics in ARC neurons revealed that NE activates NPY/AgRP neurons through α1A receptors and inhibits POMC neurons via α2A receptors (Paeger et al., 2017), which shed light on future subtype-specific functional and mechanistic studies. However, it is not clear whether ARs-mediated feeding control is independent of other critical metabolic regulators, such as MC4R.
Hypothalamic α1 and α2-ARs are also involved in the regulation of cardiovascular functions, mainly through the modulation of sympathetic tone that is regulated by different hypothalamic nuclei. Injection of the α1 agonist PHE into the PVN significantly increased HR and sympathetic outflow (Mendonca et al., 2018). Moreover, injection of an α1 agonist metaraminol into the median preoptic nucleus (MnPO) significantly increased BP (Llewellyn et al., 2012; Takahashi and Tanaka, 2017). On the other hand, microinjection of α2 agonist clonidine into the anterior hypothalamic nucleus (AHN) caused a rapid decrease in BP, indicating inhibited sympathetic outflows (Peng et al., 2003). Due to the lack of selective pharmacological tools to dissect the function of distinct subtypes of α1 and α2-ARs, transgenic animals that lack specific AR subtypes are generated. Mice with selective deficiency of α1A receptors are hypotensive (Rokosh and Simpson, 2002). On the other hand, α2A knockout mice are insensitive to α2 agonists-induced hypotensive effect (Altman et al., 1999), suggesting that α2A subtype mediates the most pharmacological functions of α2 agonists and that centrally abundant α2A subtype is critical for cardiovascular regulation. Future experiments should focus on conditional knockout of AR subtypes in different hypothalamic nuclei with either cell-type-specific Cre lines or viruses-mediated regional deletion to identify the relative contribution of different AR subtypes to cardiometabolic homeostasis and address how these regulatory pathways might be perturbed in the context of obesity.
Even though we are still far from fully understanding the roles of hypothalamic α1 and α2 subtypes in the modulation of cardiometabolic homeostasis, it is clear that their functions are context-dependent, and crosstalks exist between the AR signaling and other neurotransmitters. Future single-cell transcriptomic studies will help to reveal the molecular profile of hypothalamic AR-expressing cells, providing better insights into the next-generation pharmacological agents targeting AR subtypes for therapeutic use.
GLP-1, Hypothalamic GLP-1 Receptor and Cardiometabolic Regulation
Glucagon-like peptide (GLP)-1 is a small peptide hormone derived from the preproglucagon protein by enzymatic cleavage processes. Although the preproglucagon gene is observed in the L cells of the intestine, α cells of the pancreas, and some neurons residing the caudal brainstem and hypothalamus, the major source of endogenous GLP-1 is the gut (Mojsov et al., 1986). GLP-1 enhances the glucose-stimulated insulin secretion as well as inhibits glucagon secretion (Nauck et al., 1993a,b). Subsequent studies further revealed an effect of GLP-1 on food intake and body weight control (Tang-Christensen et al., 1996; Turton et al., 1996; Naslund et al., 1999). The physiological effects of GLP-1 are mediated by the GLP-1 receptor (GLP-1R) localized to both the periphery and the brain (Dunphy et al., 1998). Overexpression studies using recombinant cell lines suggest that GLP-1R can signal through multiple G proteins, including Gαs, Gαq/11, Gαi1, and Gαi2 (Wheeler et al., 1993; Montrose-Rafizadeh et al., 1999). Recruitment and subsequent signaling through the scaffolding protein, β-arrestin 1, has also been reported, suggesting that activated GLP-1R is also capable of transducing G protein independent signals (Sonoda et al., 2008). However, the G protein dependent and independent mechanisms of GLP-1R-mediated signaling transduction may not be mutually exclusive, as β-arrestin 1 knockdown impaired GLP-1 stimulated insulin secretion, cAMP production, ERK1/2 phosphorylation, as well as suppressed the anti-apoptotic effects of GLP-1 in pancreatic β cell lines obtained from β-arrestin 1 knockout mice (Sonoda et al., 2008; Quoyer et al., 2010). Interactions between β-arrestin 2, GRK2 and activated GLP-1R have been proposed by Jorgensen et al. (2011). In this study, Jorgensen and colleagues employed bioluminescence resonance energy transfer assays to assess interactions between these three molecules and observed that β-arrestin 2 competes with GRK2 for interaction with GLP-1R. However, given these assasys were performed in vitro, the physiological relevance of these interactions must be carefully evaluated in vivo in regards to the potential impact on cardiometabolic effects.
Due to its beneficial insulinotropic effect, several pharmacological GLP-1R agonists have been successfully developed and marketed for the treatment of type 2 diabetes mellitus (T2DM). These GLP-1R agonists include liraglutide and semaglutide, which are structurally similar acylated forms of GLP-1 (Knudsen and Lau, 2019). Liraglutide has been formulated as a once weekly injection treatment for T2DM, which was first approved in Europe in 2009 and then in the United States in 2010 (Peters, 2013). Similar to liraglutide, semaglutide (approved for T2DM management in 2017 in the United States and 2019 in Europe) was initially formulated as a once weekly injection GLP-1R agonist. In 2020, however, a once daily oral formulation of semaglutide was approved for T2DM patients, allowing for an easy route of administration (Granhall et al., 2019). Experimental as well as clinical observations have also shed light on additional health benefits of GLP-1R agonists, including the promotion of weight loss by reducing food intake and a decrease in major cardiovascular adverse events (MACE) (Heuvelman et al., 2020; Qiu et al., 2020). The anorexigenic effects of GLP-1R activation have been observed in both rodents and humans and this appetite-suppressing effect is likely centrally mediated (Beiroa et al., 2014; Henderson et al., 2016). Flint and colleagues observed that when GLP-1 was infused simultaneously as the start of an energy-fixed meal in a small cohort of healthy human volunteers, a significant reduction in energy intake was observed and satiety was promoted compared to control subjects who received placebo (Flint et al., 1998). An increase in energy expenditure was also observed in T2DM patients treated with liraglutide for over a year (Beiroa et al., 2014). Thus, GLP1-R agonism appears to act on both arms of the energy balance equation to promote body weight loss. Retrospective analysis of clinical trials involving liraglutide also supports the clinical benefit of this GLP-1 agonist on promoting weight loss (Mehta et al., 2017). Due to the overwhelming evidence of the beneficial effects of liraglutide in weight management, it was approved for obesity treatment in 2014. A large-scale, multi-nation clinical trial was initiated in 2018 to assess the efficacy and safety of semaglutide for weight management in obese and overweight adults. The results of this trial were recently reported and found that semaglutide demonstrated superior body weight reduction in trial participants, as compared to placebo (Davies et al., 2021). The results of this clinical trial paved the road for another GLP-1R agonist to be on the short list of potential anti-obesity therapeutics for approval for use in the general public.
The precise molecular and cellular mechanisms as well as the neurocircuitry underpinning the anorexigenic effects of GLP-1 remain to be fully elucidated, but key insights can be gleaned from several mechanistic studies. As alluded to above, the GLP-1-induced anorexigenic effect appears to be centrally mediated, as central GLP-1 administration into the ARC, the LHA or the PVN all resulted in reduced food intake and body weight in rats (Beiroa et al., 2014). c-Fos induction was observed in these hypothalamic nuclei upon GLP-1 infusion, indicating neuronal activation. In line with these observations, Secher and colleagues found that peripherally administered liraglutide can directly activate POMC neurons and indirectly inhibit AgRP neurons of the ARC (Secher et al., 2014). Central infusion of the GLP1-R antagonist Exendin (9–39) into the ARC, but not the PVN, blocked the weight loss effect induced by liraglutide, leading the authors to suggest that the ARC is a critical hypothalamic nucleus mediating GLP-1 regulation of body weight (Secher et al., 2014). In another study, Sandoval and colleagues observed that when GLP-1 was centrally infused into the ARC or the PVN, a reduction in food intake was only observed in rats with GLP-1 infused into the PVN but not the ARC (Sandoval et al., 2008). Consistent with a role for PVN neurons in GLP-1 induced food intake suppression, Liu and colleagues reported that GLP-1 expressing NTS neurons projecting to corticotropin-releasing hormone (CRH) neurons of the PVN are required for the food intake suppression by GLP-1 (Liu et al., 2017). Taken together, these observations critically support the concept that brain region-specific GLP-1R signaling cascades differentially activated by GLP-1 and liraglutide are involved in the pleiotropic effects of this ligand-receptor system.
GLP-1 and GLP-1R agonists also exert cardioprotective effects. In both rodents and humans, GLP-1R is expressed in cardiomyocytes, vascular smooth muscle cells, and endocardium (Campos et al., 1994; Wei and Mojsov, 1995). GLP-1 treatment significantly reduced infarction size in an ischemia-reperfusion model through adenylyl cyclase, phosphatidylinositol 3-kinase (PI3K), and ERK1/2 signaling cascades, as applications of inhibitors for these signaling pathways abrogate the GLP-1-mediated cardio-protection (Bose et al., 2005). More importantly, a meta-analysis of clinical trials evaluating GLP1-R agonists, such as liraglutide and semaglutide, revealed a trend toward reduction in incidences of MACE, lending credence to the potential of new cardiovascular disease treatments (Kristensen et al., 2019). The reduction in MACE by the treatment of GLP-1 and GLP-1R agonists may be attributed to the antihypertensive effect of this pleiotropic peptide hormone. Indeed, the long-term treatment of GLP-1R agonists in hypertensive T2DM patients resulted in reduced BP (Ferdinand et al., 2014; von Scholten et al., 2015). The antihypertensive effect exerted by GLP-1 and GLP-1R agonists seems dependent, in part, on dopamine beta-hydroxylase+ (DBH+) neurons located in the NTS of brainstem, as liraglutide injection into the fourth ventricle attenuated the development of hypertension in SHR rats with concomitant induction of c-FOS expression in NTS DBH+ neurons, whereas the ablation of these NTS DBH+ neurons dampened the antihypertensive effects of liraglutide injection (Katsurada et al., 2019). The precise mechanism(s) of how GLP-1 exerts its cardio-protective effects, however, remain yet to be fully clarified, as GLP-1Rs are also expressed in immune and renal cells and may additionally influence these two tissue organs to exert its cardioprotective effect (Hadjiyanni et al., 2010; Crajoinas et al., 2011). However, since these topics are beyond the scope of this review, we would like to point interested readers to recently published reviews examining the role of GLP-1/GLP-1R agonists on the immune system and the kidney (Zietek and Rath, 2016; Vitale et al., 2020).
Perspective
Despite enormous efforts in understanding the underlying pathogenesis of obesity, the development of safe and selective pharmacotherapeutics for obesity with minimal cardiovascular side effects has been unsatisfactory, largely due to the existence of divergent signaling pathways involved in both metabolic and cardiovascular regulations. The hypothalamus is crucial for orchestrating cardiometabolic homeostasis, and many GPCRs that are important for cardiometabolic regulation are expressed across different hypothalamic nuclei. As summarized in Figure 1 and Table 1, the coupling between the GPCRs and the G proteins, together with the neuroanatomical and cellular contexts, defines the physiological outcomes of activation and/or inhibition of a GPCR. Another layer of complexity in understanding the physiological roles of hypothalamic GPCRs comes from the possibly biased activation of signaling cascade in distinct types of hypothalamic neurons with either functionally segregated projection or unique molecular identity as modeled in PVN and ARC neurons using MC4R and AT1a as examples (Figure 2). Since the studies have shown that indiscriminate activation of sympathetic traffics to multiple metabolic and cardiovascular organs by various hypothalamic GPCR signaling might represent one of the common mechanisms for obesity-associated hypertension, unraveling the physiological roles of cell-type- and/or pathway-selective hypothalamic GPCR signaling is a critical step toward the future development of highly targeted therapeutics with minimal unwanted side effects, including brain circuit-specific GPCR activation and/or identification of biased agonism/antagonism for various GPCRs. Loss- and gain-of-function genetic approaches for a GPCR combined with functional circuit mapping may be necessary for a decisive evaluation of the physiological roles of a GPCR within a specific hypothalamic neurocircuitry.
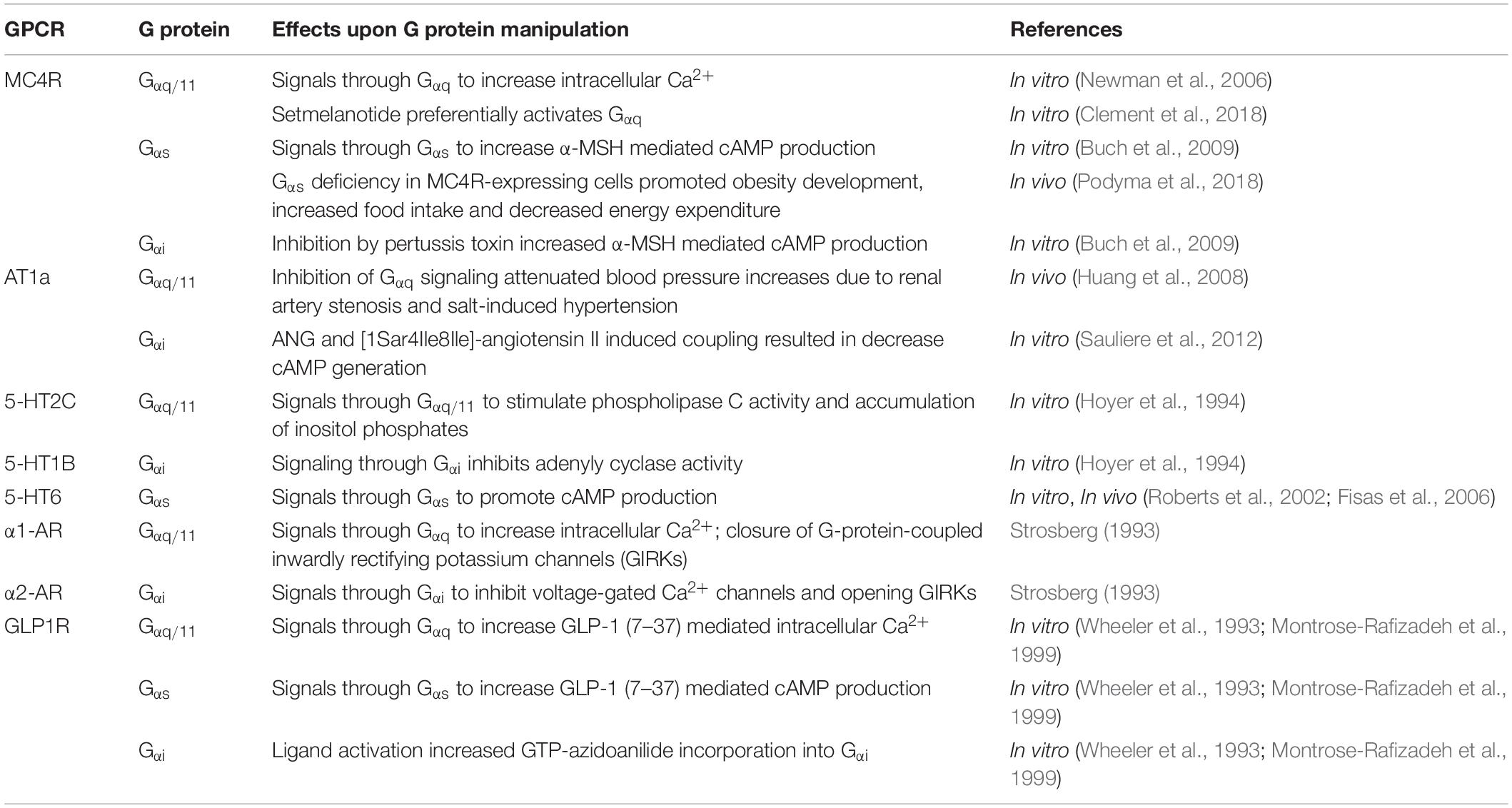
Table 1. The downstream signaling effects of hypothalamic GPCRs involved in the cardiometabolic regulation.
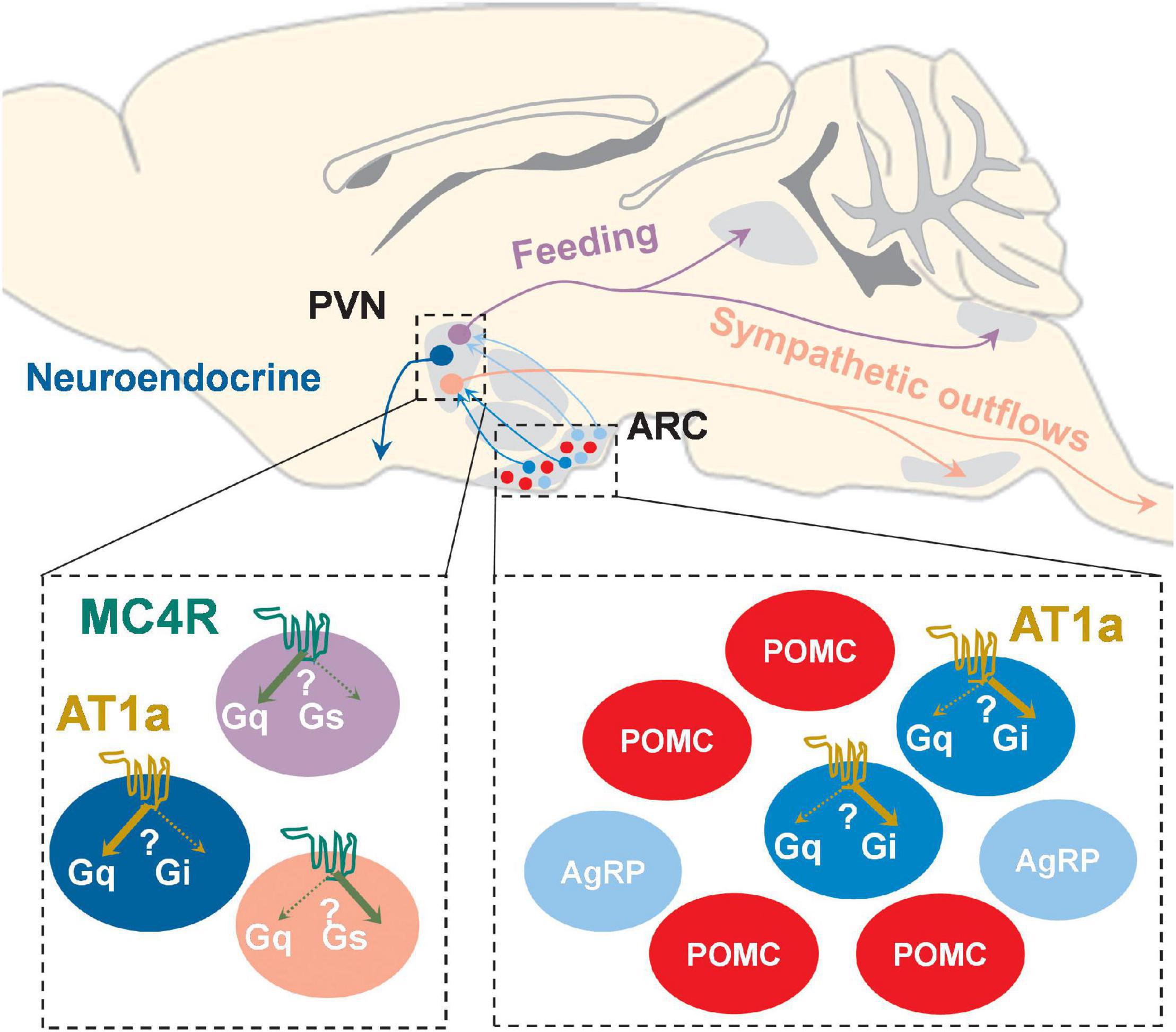
Figure 2. Schematic of possible brain circuit- and cell type-specific MC4R and AT1a signaling pathways in both PVN and ARC. PVNMC4R+ neurons project to different brain regions to differentially regulate feeding and sympathetic outflows, and it is possible that MC4Rs in these functionally and neuroanatomically distinct neurons may preferentially activate one involved downstream signaling cascade (Gαs and/or Gαq) over another. AT1a is uniquely expressed in a subset of ARC AgRP+ neurons as well as PVN neuroendocrine neurons, and it has been suggested that AT1a in these neurochemically defined neurons may preferentially coupled to either Gαq (PVN) or Gαi (ARC AgRP+).
Author Contributions
YD and GD wrote manuscript. JG provided critical inputs and revised the manuscript. HC revised and finalized the manuscript. All authors contributed to the article and approved the submitted version.
Funding
This work was supported by grants from the National Institutes of Health (HL127673, HL084207, and HL153274 to HC and HL134850 to JG), the University of Iowa Fraternal Order of Eagles Diabetes Center Pilot Grant (to HC), and the American Heart Association (19POST34380239 to GD and 18EIA33890055 to JG).
Conflict of Interest
The authors declare that the research was conducted in the absence of any commercial or financial relationships that could be construed as a potential conflict of interest.
References
Aguilera, G., Young, W. S., Kiss, A., and Bathia, A. (1995). Direct regulation of hypothalamic corticotropin-releasing-hormone neurons by angiotensin II. Neuroendocrinology 61, 437–444. doi: 10.1159/000126866
Aklan, I., Sayar Atasoy, N., Yavuz, Y., Ates, T., Coban, I., Koksalar, F., et al. (2020). NTS catecholamine neurons mediate hypoglycemic hunger via medial hypothalamic feeding pathways. Cell Metab 31, 313–326.e5.
Alexander, J. T., Cheung, W. K., Dietz, C. B., and Leibowitz, S. F. (1993). Meal patterns and macronutrient intake after peripheral and PVN injections of the alpha 2-receptor antagonist idazoxan. Physiol. Behav. 53, 623–630. doi: 10.1016/0031-9384(93)90165-c
Altman, J. D., Trendelenburg, A. U., MacMillan, L., Bernstein, D., Limbird, L., Starke, K., et al. (1999). Abnormal regulation of the sympathetic nervous system in alpha2A-adrenergic receptor knockout mice. Mol. Pharmacol. 56, 154–161. doi: 10.1124/mol.56.1.154
Aponte, Y., Atasoy, D., and Sternson, S. M. (2011). AGRP neurons are sufficient to orchestrate feeding behavior rapidly and without training. Nat. Neurosci. 14, 351–355. doi: 10.1038/nn.2739
Atasoy, D., Betley, J. N., Su, H. H., and Sternson, S. M. (2012). Deconstruction of a neural circuit for hunger. Nature 488, 172–177. doi: 10.1038/nature11270
Attramadal, H., Arriza, J. L., Aoki, C., Dawson, T. M., Codina, J., Kwatra, M. M., et al. (1992). Beta-arrestin2, a novel member of the arrestin/beta-arrestin gene family. J. Biol. Chem. 267, 17882–17890. doi: 10.1016/s0021-9258(19)37125-x
Balthasar, N., Dalgaard, L. T., Lee, C. E., Yu, J., Funahashi, H., Williams, T., et al. (2005). Divergence of melanocortin pathways in the control of food intake and energy expenditure. Cell 123, 493–505. doi: 10.1016/j.cell.2005.08.035
Beiroa, D., Imbernon, M., Gallego, R., Senra, A., Herranz, D., Villarroya, F., et al. (2014). GLP-1 agonism stimulates brown adipose tissue thermogenesis and browning through hypothalamic AMPK. Diabetes 63, 3346–3358. doi: 10.2337/db14-0302
Bergen, S. S. Jr. (1964). Appetite stimulating properties of cyproheptadine. Am. J. Dis. Child 108, 270–273. doi: 10.1001/archpedi.1964.02090010272008
Berridge, C. W., Schmeichel, B. E., and Espana, R. A. (2012). Noradrenergic modulation of wakefulness/arousal. Sleep Med. Rev. 16, 187–197. doi: 10.1016/j.smrv.2011.12.003
Bhakthavatsalam, P., and Leibowitz, S. F. (1986). Alpha 2-noradrenergic feeding rhythm in paraventricular nucleus: relation to corticosterone. Am. J. Physiol. 250, R83–R88.
Bohula, E. A., Wiviott, S. D., McGuire, D. K., Inzucchi, S. E., Kuder, J., Im, K., et al. (2018). Committee, and investigators, cardiovascular safety of lorcaserin in overweight or obese patients. N. Engl. J. Med. 379, 1107–1117.
Bolze, F., and Klingenspor, M. (2009). Mouse models for the central melanocortin system. Genes Nutr. 4, 129–134. doi: 10.1007/s12263-009-0117-6
Bose, A. K., Mocanu, M. M., Carr, R. D., Brand, C. L., and Yellon, D. M. (2005). Glucagon-like peptide 1 can directly protect the heart against ischemia/reperfusion injury. Diabetes 54, 146–151. doi: 10.2337/diabetes.54.1.146
Breit, A., Buch, T. R., Boekhoff, I., Solinski, H. J., Damm, E., and Gudermann, T. (2011). Alternative G protein coupling and biased agonism: new insights into melanocortin-4 receptor signalling. Mol. Cell. Endocrinol. 331, 232–240. doi: 10.1016/j.mce.2010.07.007
Brenot, F., Herve, P., Petitpretz, P., Parent, F., Duroux, P., and Simonneau, G. (1993). Primary pulmonary hypertension and fenfluramine use. Br. Heart J. 70, 537–541. doi: 10.1136/hrt.70.6.537
Buch, T. R., Heling, D., Damm, E., Gudermann, T., and Breit, A. (2009). Pertussis toxin-sensitive signaling of melanocortin-4 receptors in hypothalamic GT1-7 cells defines agouti-related protein as a biased agonist. J. Biol. Chem. 284, 26411–26420. doi: 10.1074/jbc.m109.039339
Camargo, L. A., and Saad, W. A. (2001). Role of the alpha(1)- and alpha(2)-adrenoceptors of the paraventricular nucleus on the water and salt intake, renal excretion, and arterial pressure induced by angiotensin II injection into the medial septal area. Brain Res. Bull. 54, 595–602. doi: 10.1016/s0361-9230(01)00469-5
Campos, R. V., Lee, Y. C., and Drucker, D. J. (1994). Divergent tissue-specific and developmental expression of receptors for glucagon and glucagon-like peptide-1 in the mouse. Endocrinology 134, 2156–2164. doi: 10.1210/endo.134.5.8156917
Chai, B., Li, J. Y., Zhang, W., Newman, E., Ammori, J., and Mulholland, M. W. (2006). Melanocortin-4 receptor-mediated inhibition of apoptosis in immortalized hypothalamic neurons via mitogen-activated protein kinase. Peptides 27, 2846–2857. doi: 10.1016/j.peptides.2006.05.005
Chaudhary, P. K., and Kim, S. (2021). The GRKs reactome: role in cell biology and pathology. Int. J. Mol. Sci. 22:3375. doi: 10.3390/ijms22073375
Chen, F., Liu, F., and Badoer, E. (2011). AT1 receptors in the paraventricular nucleus mediate the hyperthermia-induced reflex reduction of renal blood flow in rats. Am. J. Physiol. Regul. Integr. Comp. Physiol. 300, R479–R485.
Chen, J., Cheng, M., Wang, L., Zhang, L., Xu, D., Cao, P., et al. (2020). Pathway that stimulates feeding. Curr. Biol. 30, 3986–3998.e5.
Chen, M., Shrestha, Y. B., Podyma, B., Cui, Z., Naglieri, B., Sun, H., et al. (2017). Gsalpha deficiency in the dorsomedial hypothalamus underlies obesity associated with Gsalpha mutations. J. Clin. Invest. 127, 500–510. doi: 10.1172/jci88622
Chen, M., Wilson, E. A., Cui, Z., Sun, H., Shrestha, Y. B., Podyma, B., et al. (2019). Gsalpha deficiency in the dorsomedial hypothalamus leads to obesity, hyperphagia, and reduced thermogenesis associated with impaired leptin signaling. Mol. Metab. 25, 142–153. doi: 10.1016/j.molmet.2019.04.005
Claflin, K. E., Sandgren, J. A., Lambertz, A. M., Weidemann, B. J., Littlejohn, N. K., Burnett, C. M., et al. (2017). Angiotensin AT1A receptors on leptin receptor-expressing cells control resting metabolism. J. Clin. Invest. 127, 1414–1424. doi: 10.1172/jci88641
Clement, K., Biebermann, H., Farooqi, I. S., Van der Ploeg, L., Wolters, B., Poitou, C., et al. (2018). MC4R agonism promotes durable weight loss in patients with leptin receptor deficiency. Nat. Med. 24, 551–555. doi: 10.1038/s41591-018-0015-9
Collet, T. H., Dubern, B., Mokrosinski, J., Connors, H., Keogh, J. M., Mendes de Oliveira, E., et al. (2017). Evaluation of a melanocortin-4 receptor (MC4R) agonist (Setmelanotide) in MC4R deficiency. Mol. Metab. 6, 1321–1329.
Crajoinas, R. O., Oricchio, F. T., Pessoa, T. D., Pacheco, B. P., Lessa, L. M., Malnic, G., et al. (2011). Mechanisms mediating the diuretic and natriuretic actions of the incretin hormone glucagon-like peptide-1. Am. J. Physiol. Renal Physiol. 301, F355–F363.
da Silva, A. A., do Carmo, J. M., Kanyicska, B., Dubinion, J., Brandon, E., and Hall, J. E. (2008). Endogenous melanocortin system activity contributes to the elevated arterial pressure in spontaneously hypertensive rats. Hypertension 51, 884–890. doi: 10.1161/hypertensionaha.107.100636
Davies, M., Faerch, L., Jeppesen, O. K., Pakseresht, A., Pedersen, S. D., Perreault, L., et al. (2021). Semaglutide 2.4 mg once a week in adults with overweight or obesity, and type 2 diabetes (STEP 2): a randomised, double-blind, double-dummy, placebo-controlled, phase 3 trial. Lancet 397, 971–984. doi: 10.1016/s0140-6736(21)00213-0
Davisson, R. L., Oliverio, M. I., Coffman, T. M., and Sigmund, C. D. (2000). Divergent functions of angiotensin II receptor isoforms in the brain. J. Clin. Invest. 106, 103–106. doi: 10.1172/jci10022
Day, H. E., Campeau, S., Watson, S. J. Jr., and Akil, H. (1997). Distribution of alpha 1a-, alpha 1b- and alpha 1d-adrenergic receptor mRNA in the rat brain and spinal cord. J. Chem. Neuroanat. 13, 115–139.
de Kloet, A. D., Pati, D., Wang, L., Hiller, H., Sumners, C., Frazier, C. J., et al. (2013). Angiotensin type 1a receptors in the paraventricular nucleus of the hypothalamus protect against diet-induced obesity. J. Neurosci. 33, 4825–4833. doi: 10.1523/jneurosci.3806-12.2013
Deng, G., and Grobe, J. L. (2019). The renin-angiotensin system in the arcuate nucleus controls resting metabolic rate. Curr. Opin. Nephrol. Hypertens. 28, 120–127. doi: 10.1097/mnh.0000000000000477
do Carmo, J. M., da Silva, A. A., Wang, Z., Fang, T., Aberdein, N., Perez de Lara, C. E., et al. (2017). Role of the brain melanocortins in blood pressure regulation. Biochim. Biophys. Acta Mol. Basis Dis. 1863, 2508–2514. doi: 10.1016/j.bbadis.2017.03.003
Dodd, G. T., Decherf, S., Loh, K., Simonds, S. E., Wiede, F., Balland, E., et al. (2015). Leptin and insulin act on POMC neurons to promote the browning of white fat. Cell 160, 88–104. doi: 10.1016/j.cell.2014.12.022
Dunphy, J. L., Taylor, R. G., and Fuller, P. J. (1998). Tissue distribution of rat glucagon receptor and GLP-1 receptor gene expression. Mol. Cell. Endocrinol. 141, 179–186. doi: 10.1016/s0303-7207(98)00096-3
Eishingdrelo, H., Sun, W., Li, H., Wang, L., Eishingdrelo, A., Dai, S., et al. (2015). ERK and beta-arrestin interaction: a converging point of signaling pathways for multiple types of cell surface receptors. J. Biomol. Screen. 20, 341–349. doi: 10.1177/1087057114557233
Elton, T. S., Stephan, C. C., Taylor, G. R., Kimball, M. G., Martin, M. M., Durand, J. N., et al. (1992). Isolation of two distinct type I angiotensin II receptor genes. Biochem. Biophys. Res. Commun. 184, 1067–1073. doi: 10.1016/0006-291x(92)90700-u
Fan, Z. D., Zhang, L., Shi, Z., Gan, X. B., Gao, X. Y., and Zhu, G. Q. (2012). Artificial microRNA interference targeting AT(1a) receptors in paraventricular nucleus attenuates hypertension in rats. Gene Ther. 19, 810–817. doi: 10.1038/gt.2011.145
Farooqi, I. S., Keogh, J. M., Yeo, G. S., Lank, E. J., Cheetham, T., and O’Rahilly, S. (2003). Clinical spectrum of obesity and mutations in the melanocortin 4 receptor gene. N. Engl. J. Med. 348, 1085–1095. doi: 10.1056/nejmoa022050
Feldman, P. D., and Felder, R. B. (1989). Alpha-adrenergic influences on neuronal responses to visceral afferent input in the nucleus tractus solitarius. Neuropharmacology 28, 1081–1087. doi: 10.1016/0028-3908(89)90121-4
Ferdinand, K. C., White, W. B., Calhoun, D. A., Lonn, E. M., Sager, P. T., Brunelle, R., et al. (2014). Effects of the once-weekly glucagon-like peptide-1 receptor agonist dulaglutide on ambulatory blood pressure and heart rate in patients with type 2 diabetes mellitus. Hypertension 64, 731–737. doi: 10.1161/hypertensionaha.114.03062
Fisas, A., Codony, X., Romero, G., Dordal, A., Giraldo, J., Merce, R., et al. (2006). Chronic 5-HT6 receptor modulation by E-6837 induces hypophagia and sustained weight loss in diet-induced obese rats. Br. J. Pharmacol. 148, 973–983. doi: 10.1038/sj.bjp.0706807
Flegal, K. M., Carroll, M. D., Ogden, C. L., and Curtin, L. R. (2010). Prevalence and trends in obesity among US adults, 1999-2008. JAMA 303, 235–241. doi: 10.1001/jama.2009.2014
Flint, A., Raben, A., Astrup, A., and Holst, J. J. (1998). Glucagon-like peptide 1 promotes satiety and suppresses energy intake in humans. J. Clin. Invest. 101, 515–520. doi: 10.1172/jci990
Frassetto, A., Zhang, J., Lao, J. Z., White, A., Metzger, J. M., Fong, T. M., et al. (2008). Reduced sensitivity to diet-induced obesity in mice carrying a mutant 5-HT6 receptor. Brain Res. 1236, 140–144. doi: 10.1016/j.brainres.2008.08.012
Garfield, A. S., Burke, L. K., Shaw, J., Evans, M. L., and Heisler, L. K. (2014). Distribution of cells responsive to 5-HT(6) receptor antagonist-induced hypophagia. Behav. Brain Res. 266, 201–206. doi: 10.1016/j.bbr.2014.02.018
Garfield, A. S., Lam, D. D., Marston, O. J., Przydzial, M. J., and Heisler, L. K. (2009). Role of central melanocortin pathways in energy homeostasis. Trends Endocrinol. Metab. 20, 203–215. doi: 10.1016/j.tem.2009.02.002
Garfield, A. S., Li, C., Madara, J. C., Shah, B. P., Webber, E., Steger, J. S., et al. (2015). A neural basis for melanocortin-4 receptor-regulated appetite. Nat. Neurosci. 18, 863–871. doi: 10.1038/nn.4011
Garza, J. C., Kim, C. S., Liu, J., Zhang, W., and Lu, X. Y. (2008). Adeno-associated virus-mediated knockdown of melanocortin-4 receptor in the paraventricular nucleus of the hypothalamus promotes high-fat diet-induced hyperphagia and obesity. J. Endocrinol. 197, 471–482. doi: 10.1677/joe-08-0009
Gautron, L., Elmquist, J. K., and Williams, K. W. (2015). Neural control of energy balance: translating circuits to therapies. Cell 161, 133–145. doi: 10.1016/j.cell.2015.02.023
Goldman, C. K., Marino, L., and Leibowitz, S. F. (1985). Postsynaptic alpha 2-noradrenergic receptors mediate feeding induced by paraventricular nucleus injection of norepinephrine and clonidine. Eur. J. Pharmacol. 115, 11–19. doi: 10.1016/0014-2999(85)90578-3
Gonzalez-Muniesa, P., Martinez-Gonzalez, M. A., Hu, F. B., Despres, J. P., Matsuzawa, Y., Loos, R. J. F., et al. (2017). Obesity. Nat. Rev. Dis. Primers 3:17034.
Granhall, C., Donsmark, M., Blicher, T. M., Golor, G., Sondergaard, F. L., Thomsen, M., et al. (2019). Safety and pharmacokinetics of single and multiple ascending doses of the novel oral human GLP-1 analogue, oral semaglutide, in healthy subjects and subjects with Type 2 diabetes. Clin. Pharmacokinet. 58, 781–791. doi: 10.1007/s40262-018-0728-4
Greenfield, J. R., Miller, J. W., Keogh, J. M., Henning, E., Satterwhite, J. H., Cameron, G. S., et al. (2009). Modulation of blood pressure by central melanocortinergic pathways. N. Engl. J. Med. 360, 44–52. doi: 10.1056/nejmoa0803085
Grobe, J. L., Buehrer, B. A., Hilzendeger, A. M., Liu, X., Davis, D. R., Xu, D., et al. (2011). Angiotensinergic signaling in the brain mediates metabolic effects of deoxycorticosterone (DOCA)-salt in C57 mice. Hypertension 57, 600–607. doi: 10.1161/hypertensionaha.110.165829
Guy-Grand, B. (1995). Clinical studies with dexfenfluramine: from past to future. Obes. Res. 3(Suppl. 4), 491S–496S.
Haack, K. K., Engler, C. W., Papoutsi, E., Pipinos, I. I., Patel, K. P., and Zucker, I. H. (2012). Parallel changes in neuronal AT1R and GRK5 expression following exercise training in heart failure. Hypertension 60, 354–361. doi: 10.1161/hypertensionaha.112.195693
Hadjiyanni, I., Siminovitch, K. A., Danska, J. S., and Drucker, D. J. (2010). Glucagon-like peptide-1 receptor signalling selectively regulates murine lymphocyte proliferation and maintenance of peripheral regulatory T cells. Diabetologia 53, 730–740. doi: 10.1007/s00125-009-1643-x
Hanna, P., Grybek, V., Perez de Nanclares, G., Tran, L. C., de Sanctis, L., Elli, F., et al. (2018). Genetic and epigenetic defects at the GNAS locus lead to distinct patterns of skeletal growth but similar early-onset obesity. J. Bone Miner. Res. 33, 1480–1488. doi: 10.1002/jbmr.3450
Harris, D. M., Cohn, H. I., Pesant, S., Zhou, R. H., and Eckhart, A. D. (2007). Vascular smooth muscle G(q) signaling is involved in high blood pressure in both induced renal and genetic vascular smooth muscle-derived models of hypertension. Am. J. Physiol. Heart Circ. Physiol. 293, H3072–H3079.
Hauser, A. S., Chavali, S., Masuho, I., Jahn, L. J., Martemyanov, K. A., Gloriam, D. E., et al. (2018). Pharmacogenomics of GPCR drug targets. Cell 172, 41–54.e19.
Haynes, W. G., Morgan, D. A., Djalali, A., Sivitz, W. I., and Mark, A. L. (1999). Interactions between the melanocortin system and leptin in control of sympathetic nerve traffic. Hypertension 33, 542–547. doi: 10.1161/01.hyp.33.1.542
He, S., and Tao, Y. X. (2014). Defect in MAPK signaling as a cause for monogenic obesity caused by inactivating mutations in the melanocortin-4 receptor gene. Int. J. Biol. Sci. 10, 1128–1137. doi: 10.7150/ijbs.10359
Hein, L. (2006). Adrenoceptors and signal transduction in neurons. Cell Tissue Res. 326, 541–551. doi: 10.1007/s00441-006-0285-2
Henderson, S. J., Konkar, A., Hornigold, D. C., Trevaskis, J. L., Jackson, R., Fritsch Fredin, M., et al. (2016). Robust anti-obesity and metabolic effects of a dual GLP-1/glucagon receptor peptide agonist in rodents and non-human primates. Diabetes Obes. Metab. 18, 1176–1190. doi: 10.1111/dom.12735
Heuvelman, V. D., Van Raalte, D. H., and Smits, M. M. (2020). Cardiovascular effects of glucagon-like peptide 1 receptor agonists: from mechanistic studies in humans to clinical outcomes. Cardiovasc. Res. 116, 916–930. doi: 10.1093/cvr/cvz323
Hilzendeger, A. M., Morgan, D. A., Brooks, L., Dellsperger, D., Liu, X., Grobe, J. L., et al. (2012). A brain leptin-renin angiotensin system interaction in the regulation of sympathetic nerve activity. Am. J. Physiol. Heart Circ. Physiol. 303, H197–H206.
Hoyer, D., Clarke, D. E., Fozard, J. R., Hartig, P. R., Martin, G. R., Mylecharane, E. J., et al. (1994). International union of pharmacology classification of receptors for 5-hydroxytryptamine (Serotonin). Pharmacol. Rev. 46, 157–203. doi: 10.1007/978-94-009-0479-8_12
Huang, X., Charbeneau, R. A., Fu, Y., Kaur, K., Gerin, I., MacDougald, O. A., et al. (2008). Resistance to diet-induced obesity and improved insulin sensitivity in mice with a regulator of G protein signaling-insensitive G184S Gnai2 allele. Diabetes 57, 77–85. doi: 10.2337/db07-0599
Hurt, R. C., Garrett, J. C., Keifer, O. P. Jr., Linares, A., Couling, L., Speth, R. C., et al. (2015). Angiotensin type 1a receptors on corticotropin-releasing factor neurons contribute to the expression of conditioned fear. Genes Brain Behav. 14, 526–533. doi: 10.1111/gbb.12235
Iwai, N., and Inagami, T. (1992). Identification of two subtypes in the rat type I angiotensin II receptor. FEBS Lett. 298, 257–260. doi: 10.1016/0014-5793(92)80071-n
Jafferjee, M., Reyes Valero, T., Marrero, C., McCrink, K. A., Brill, A., and Lymperopoulos, A. (2016). GRK2 Up-Regulation creates a positive feedback loop for catecholamine production in chromaffin cells. Mol. Endocrinol. 30, 372–381. doi: 10.1210/me.2015-1305
Jhanwar-Uniyal, M., Roland, C. R., and Leibowitz, S. F. (1986). Diurnal rhythm of alpha 2-noradrenergic receptors in the paraventricular nucleus and other brain areas: relation to circulating corticosterone and feeding behavior. Life Sci. 38, 473–482. doi: 10.1016/0024-3205(86)90073-1
Jorgensen, R., Norklit Roed, S., Heding, A., and Elling, C. E. (2011). Beta-arrestin2 as a competitor for GRK2 interaction with the GLP-1 receptor upon receptor activation. Pharmacology 88, 174–181. doi: 10.1159/000330742
Katsurada, K., Nakata, M., Saito, T., Zhang, B., Maejima, Y., Nandi, S. S., et al. (2019). Central glucagon-like Peptide-1 receptor signaling via brainstem catecholamine neurons counteracts hypertension in spontaneously hypertensive rats. Sci. Rep. 9:12986.
Kim, M. S., Rossi, M., Abusnana, S., Sunter, D., Morgan, D. G., Small, C. J., et al. (2000). Hypothalamic localization of the feeding effect of agouti-related peptide and alpha-melanocyte-stimulating hormone. Diabetes 49, 177–182. doi: 10.2337/diabetes.49.2.177
Kishi, T., Aschkenasi, C. J., Lee, C. E., Mountjoy, K. G., Saper, C. B., and Elmquist, J. K. (2003). Expression of melanocortin 4 receptor mRNA in the central nervous system of the rat. J. Comp. Neurol. 457, 213–235. doi: 10.1002/cne.10454
Knudsen, L. B., and Lau, J. (2019). The discovery and development of liraglutide and semaglutide. Front. Endocrinol. (Lausanne) 10:155. doi: 10.3389/fendo.2019.00155
Kooijman, S., Boon, M. R., Parlevliet, E. T., Geerling, J. J., van de Pol, V., Romijn, J. A., et al. (2014). Inhibition of the central melanocortin system decreases brown adipose tissue activity. J. Lipid Res. 55, 2022–2032. doi: 10.1194/jlr.m045989
Kostenis, E., Pfeil, E. M., and Annala, S. (2020). Heterotrimeric Gq proteins as therapeutic targets? J. Biol. Chem. 295, 5206–5215. doi: 10.1074/jbc.rev119.007061
Kristensen, S. L., Rorth, R., Jhund, P. S., Docherty, K. F., Sattar, N., Preiss, D., et al. (2019). Cardiovascular, mortality, and kidney outcomes with GLP-1 receptor agonists in patients with type 2 diabetes: a systematic review and meta-analysis of cardiovascular outcome trials. Lancet Diabetes Endocrinol. 7, 776–785. doi: 10.1016/s2213-8587(19)30249-9
Kuhnen, P., Krude, H., and Biebermann, H. (2019). Melanocortin-4 receptor signalling: importance for weight regulation and obesity treatment. Trends Mol. Med. 25, 136–148. doi: 10.1016/j.molmed.2018.12.002
Kuo, J. J., da Silva, A. A., Tallam, L. S., and Hall, J. E. (2004). Role of adrenergic activity in pressor responses to chronic melanocortin receptor activation. Hypertension 43, 370–375. doi: 10.1161/01.hyp.0000111836.54204.93
Kuo, J. J., Silva, A. A., and Hall, J. E. (2003). Hypothalamic melanocortin receptors and chronic regulation of arterial pressure and renal function. Hypertension 41, 768–774. doi: 10.1161/01.hyp.0000048194.97428.1a
Lam, D. D., Przydzial, M. J., Ridley, S. H., Yeo, G. S., Rochford, J. J., O’Rahilly, S., et al. (2008). Serotonin 5-HT2C receptor agonist promotes hypophagia via downstream activation of melanocortin 4 receptors. Endocrinology 149, 1323–1328. doi: 10.1210/en.2007-1321
Lee, M. D., and Simansky, K. J. (1997). CP-94, 253: a selective serotonin1B (5-HT1B) agonist that promotes satiety. Psychopharmacology (Berl.) 131, 264–270. doi: 10.1007/s002130050292
Leibowitz, S. F., and Brown, L. L. (1980). Histochemical and pharmacological analysis of noradrenergic projections to the paraventricular hypothalamus in relation to feeding stimulation. Brain Res. 201, 289–314. doi: 10.1016/0006-8993(80)91037-9
Li, Y. Q., Shrestha, Y., Pandey, M., Chen, M., Kablan, A., Gavrilova, O., et al. (2016). G(q/11)alpha and G(s)alpha mediate distinct physiological responses to central melanocortins. J. Clin. Invest. 126, 40–49. doi: 10.1172/jci76348
Littlejohn, N. K., and Grobe, J. L. (2015). Opposing tissue-specific roles of angiotensin in the pathogenesis of obesity, and implications for obesity-related hypertension. Am. J. Physiol. Regul. Integr. Comp. Physiol. 309, R1463–R1473.
Littlejohn, N. K., Keen, H. L., Weidemann, B. J., Claflin, K. E., Tobin, K. V., Markan, K. R., et al. (2016). Suppression of resting metabolism by the angiotensin AT2 receptor. Cell Rep. 16, 1548–1560.
Liu, H., Kishi, T., Roseberry, A. G., Cai, X., Lee, C. E., Montez, J. M., et al. (2003). Transgenic mice expressing green fluorescent protein under the control of the melanocortin-4 receptor promoter. J. Neurosci. 23, 7143–7154. doi: 10.1523/jneurosci.23-18-07143.2003
Liu, J., Conde, K., Zhang, P., Lilascharoen, V., Xu, Z., Lim, B. K., et al. (2017). Enhanced AMPA receptor trafficking mediates the anorexigenic effect of endogenous glucagon-like peptide-1 in the paraventricular hypothalamus. Neuron 96, 897–909.e5.
Llewellyn, T., Zheng, H., Liu, X., Xu, B., and Patel, K. P. (2012). Median preoptic nucleus and subfornical organ drive renal sympathetic nerve activity via a glutamatergic mechanism within the paraventricular nucleus. Am. J. Physiol. Regul. Integr. Comp. Physiol. 302, R424–R432.
Lohse, M. J., Benovic, J. L., Codina, J., Caron, M. G., and Lefkowitz, R. J. (1990). beta-Arrestin: a protein that regulates beta-adrenergic receptor function. Science 248, 1547–1550. doi: 10.1126/science.2163110
Lotta, L. A., Mokrosinski, J., Mendes de Oliveira, E., Li, C., Sharp, S. J., Luan, J., et al. (2019). Human gain-of-function MC4R variants show signaling bias and protect against obesity. Cell 177, 597–607.e9.
Lucas, J. J., Yamamoto, A., Scearce-Levie, K., Saudou, F., and Hen, R. (1998). Absence of fenfluramine-induced anorexia and reduced c-Fos induction in the hypothalamus and central amygdaloid complex of serotonin 1B receptor knock-out mice. J. Neurosci. 18, 5537–5544. doi: 10.1523/jneurosci.18-14-05537.1998
Lymperopoulos, A. (2018). Arrestins in the cardiovascular system: an update. Prog. Mol. Biol. Transl. Sci. 159, 27–57. doi: 10.1016/bs.pmbts.2018.07.003
Lymperopoulos, A., Rengo, G., Funakoshi, H., Eckhart, A. D., and Koch, W. J. (2007). Adrenal GRK2 upregulation mediates sympathetic overdrive in heart failure. Nat. Med. 13, 315–323. doi: 10.1038/nm1553
Maier, T., and Hoyer, J. (2010). Modulation of blood pressure by central melanocortinergic pathways. Nephrol. Dial. Transplant. 25, 674–677. doi: 10.1093/ndt/gfp644
Makarenko, I. G., Meguid, M. M., and Ugrumov, M. V. (2002). Distribution of serotonin 5-hydroxytriptamine 1B (5-HT(1B)) receptors in the normal rat hypothalamus. Neurosci. Lett. 328, 155–159. doi: 10.1016/s0304-3940(02)00345-2
Mallick, B. N., and Singh, A. (2011). REM sleep loss increases brain excitability: role of noradrenaline and its mechanism of action. Sleep Med. Rev. 15, 165–178. doi: 10.1016/j.smrv.2010.11.001
Marzo, A., Bai, J., and Otani, S. (2009). Neuroplasticity regulation by noradrenaline in mammalian brain. Curr. Neuropharmacol. 7, 286–295. doi: 10.2174/157015909790031193
Matsuzaki, N., Nishiyama, M., Song, D., Moroi, K., and Kimura, S. (2011). Potent and selective inhibition of angiotensin AT1 receptor signaling by RGS2: roles of its N-terminal domain. Cell. Signal. 23, 1041–1049. doi: 10.1016/j.cellsig.2011.01.023
Mehta, A., Marso, S. P., and Neeland, I. J. (2017). Liraglutide for weight management: a critical review of the evidence. Obes. Sci. Pract. 3, 3–14. doi: 10.1002/osp4.84
Mendonca, M. M., Santana, J. S., da Cruz, K. R., Ianzer, D., Ghedini, P. C., Nalivaiko, E., et al. (2018). Involvement of GABAergic and adrenergic neurotransmissions on paraventricular nucleus of hypothalamus in the control of cardiac function. Front. Physiol. 9:670. doi: 10.3389/fphys.2018.00670
Mojsov, S., Heinrich, G., Wilson, I. B., Ravazzola, M., Orci, L., and Habener, J. F. (1986). Preproglucagon gene expression in pancreas and intestine diversifies at the level of post-translational processing. J. Biol. Chem. 261, 11880–11889. doi: 10.1016/s0021-9258(18)67324-7
Montrose-Rafizadeh, C., Avdonin, P., Garant, M. J., Rodgers, B. D., Kole, S., Yang, H., et al. (1999). Pancreatic glucagon-like peptide-1 receptor couples to multiple G proteins and activates mitogen-activated protein kinase pathways in Chinese hamster ovary cells. Endocrinology 140, 1132–1140. doi: 10.1210/endo.140.3.6550
Morgan, D. A., McDaniel, L. N., Yin, T., Khan, M., Jiang, J., Acevedo, M. R., et al. (2015). Regulation of glucose tolerance and sympathetic activity by MC4R signaling in the lateral hypothalamus. Diabetes 64, 1976–1987. doi: 10.2337/db14-1257
Morien, A., Wellman, P. J., and Fojt, J. (1995). Diurnal rhythms of paraventricular hypothalamic norepinephrine and food intake in rats. Pharmacol. Biochem. Behav. 52, 169–174. doi: 10.1016/0091-3057(95)00084-a
Morselli, L. L., Claflin, K. E., Cui, H., and Grobe, J. L. (2018). Control of energy expenditure by AgRP neurons of the arcuate nucleus: neurocircuitry, signaling pathways, and angiotensin. Curr. Hypertens. Rep. 20:25.
Naslund, E., Barkeling, B., King, N., Gutniak, M., Blundell, J. E., Holst, J. J., et al. (1999). Energy intake and appetite are suppressed by glucagon-like peptide-1 (GLP-1) in obese men. Int. J. Obes. Relat. Metab. Disord. 23, 304–311. doi: 10.1038/sj.ijo.0800818
Nauck, M. A., Bartels, E., Orskov, C., Ebert, R., and Creutzfeldt, W. (1993a). Additive insulinotropic effects of exogenous synthetic human gastric inhibitory polypeptide and glucagon-like peptide-1-(7-36) amide infused at near-physiological insulinotropic hormone and glucose concentrations. J. Clin. Endocrinol. Metab. 76, 912–917. doi: 10.1210/jc.76.4.912
Nauck, M. A., Kleine, N., Orskov, C., Holst, J. J., Willms, B., and Creutzfeldt, W. (1993b). Normalization of fasting hyperglycaemia by exogenous glucagon-like peptide 1 (7-36 amide) in type 2 (non-insulin-dependent) diabetic patients. Diabetologia 36, 741–744. doi: 10.1007/bf00401145
Newman, E. A., Chai, B. X., Zhang, W., Li, J. Y., Ammori, J. B., and Mulholland, M. W. (2006). Activation of the melanocortin-4 receptor mobilizes intracellular free calcium in immortalized hypothalamic neurons. J. Surg. Res. 132, 201–207. doi: 10.1016/j.jss.2006.02.003
Ni, W., Fink, G. D., and Watts, S. W. (2007). The 5-hydroxytryptamine2A receptor is involved in (+)-norfenfluramine-induced arterial contraction and blood pressure increase in deoxycorticosterone acetate-salt hypertension. J. Pharmacol. Exp. Ther. 321, 485–491. doi: 10.1124/jpet.106.114017
Northcott, C. A., Watts, S., Chen, Y., Morris, M., Chen, A., and Haywood, J. R. (2010). Adenoviral inhibition of AT1a receptors in the paraventricular nucleus inhibits acute increases in mean arterial blood pressure in the rat. Am. J. Physiol. Regul. Integr. Comp. Physiol. 299, R1202–R1211.
Nunn, C., Zhao, P., Zou, M. X., Summers, K., Guglielmo, C. G., and Chidiac, P. (2011). Resistance to age-related, normal body weight gain in RGS2 deficient mice. Cell. Signal. 23, 1375–1386. doi: 10.1016/j.cellsig.2011.03.020
Paeger, L., Karakasilioti, I., Altmuller, J., Frommolt, P., Bruning, J., and Kloppenburg, P. (2017). Antagonistic modulation of NPY/AgRP and POMC neurons in the arcuate nucleus by noradrenalin. Elife 6:e25770.
Peng, N., Chambless, B. D., Oparil, S., and Wyss, J. M. (2003). Alpha2A-adrenergic receptors mediate sympathoinhibitory responses to atrial natriuretic peptide in the mouse anterior hypothalamic nucleus. Hypertension 41, 571–575. doi: 10.1161/01.hyp.0000056998.83031.22
Perschbacher, K. J., Deng, G., Fisher, R. A., Gibson-Corley, K. N., Santillan, M. K., and Grobe, J. L. (2018). Regulators of G protein signaling in cardiovascular function during pregnancy. Physiol. Genomics 50, 590–604. doi: 10.1152/physiolgenomics.00037.2018
Peters, K. R. (2013). Liraglutide for the treatment of type 2 diabetes: a clinical update. Am. J. Ther. 20, 178–188. doi: 10.1097/mjt.0b013e3182204c16
Podyma, B., Sun, H., Wilson, E. A., Carlson, B., Pritikin, E., Gavrilova, O., et al. (2018). The stimulatory G protein Gsalpha is required in melanocortin 4 receptor-expressing cells for normal energy balance, thermogenesis, and glucose metabolism. J. Biol. Chem. 293:10993. doi: 10.1074/jbc.ra118.003450
Pollard, C. M., Ghandour, J., Cora, N., Perez, A., Parker, B. M., Desimine, V. L., et al. (2020). GRK2-mediated crosstalk between beta-adrenergic and angiotensin II receptors enhances adrenocortical aldosterone production in vitro and in vivo. Int. J. Mol. Sci. 21:574. doi: 10.3390/ijms21020574
Pydi, S. P., Cui, Z., He, Z., Barella, L. F., Pham, J., Cui, Y., et al. (2020). Beneficial metabolic role of beta-arrestin-1 expressed by AgRP neurons. Sci. Adv. 6:eaaz1341. doi: 10.1126/sciadv.aaz1341
Qiu, M., Ding, L., Wei, X., Wei, W., and Zhou, H. (2020). Effects of glucagon-like peptide 1 receptor agonists and sodium glucose cotransporter 2 inhibitors on major adverse cardiovascular events in type 2 diabetes by race, ethnicity, and region: a meta-analysis. Medicine (Baltimore) 99:e23489. doi: 10.1097/md.0000000000023489
Quoyer, J., Longuet, C., Broca, C., Linck, N., Costes, S., Varin, E., et al. (2010). GLP-1 mediates antiapoptotic effect by phosphorylating Bad through a beta-arrestin 1-mediated ERK1/2 activation in pancreatic beta-cells. J. Biol. Chem. 285, 1989–2002. doi: 10.1074/jbc.m109.067207
Ren, J., Isakova, A., Friedmann, D., Zeng, J., Grutzner, S. M., Pun, A., et al. (2019). Single-cell transcriptomes and whole-brain projections of serotonin neurons in the mouse dorsal and median raphe nuclei. Elife 8:e49424.
Rene, P., Lanfray, D., Richard, D., and Bouvier, M. (2021). Pharmacological chaperone action in humanized mouse models of MC4R-linked obesity. JCI Insight 6:e132778.
Roberts, J. C., Reavill, C., East, S. Z., Harrison, P. J., Patel, S., Routledge, C., et al. (2002). The distribution of 5-HT(6) receptors in rat brain: an autoradiographic binding study using the radiolabelled 5-HT(6) receptor antagonist [(125)I]SB-258585. Brain Res. 934, 49–57. doi: 10.1016/s0006-8993(02)02360-0
Rokosh, D. G., and Simpson, P. C. (2002). Knockout of the alpha 1A/C-adrenergic receptor subtype: the alpha 1A/C is expressed in resistance arteries and is required to maintain arterial blood pressure. Proc. Natl. Acad. Sci. U.S.A. 99, 9474–9479. doi: 10.1073/pnas.132552699
Rossi, J., Balthasar, N., Olson, D., Scott, M., Berglund, E., Lee, C. E., et al. (2011). Melanocortin-4 receptors expressed by cholinergic neurons regulate energy balance and glucose homeostasis. Cell Metab. 13, 195–204. doi: 10.1016/j.cmet.2011.01.010
Saad, W. A., Camargo, L. A., and Saad, W. A. (1984). Effects of the application of alpha 1- and alpha 2-adrenoceptor agonists and antagonists into the ventromedial hypothalamus on the sodium and potassium renal excretion. Pharmacology 28, 228–234. doi: 10.1159/000137967
Sandberg, K., Ji, H., Clark, A. J., Shapira, H., and Catt, K. J. (1992). Cloning and expression of a novel angiotensin II receptor subtype. J. Biol. Chem. 267, 9455–9458. doi: 10.1016/s0021-9258(19)50109-0
Sandgren, J. A., Linggonegoro, D. W., Zhang, S. Y., Sapouckey, S. A., Claflin, K. E., Pearson, N. A., et al. (2018). Angiotensin AT1A receptors expressed in vasopressin-producing cells of the supraoptic nucleus contribute to osmotic control of vasopressin. Am. J. Physiol. Regul. Integr. Comp. Physiol. 314, R770–R780.
Sandoval, D. A., Bagnol, D., Woods, S. C., D’Alessio, D. A., and Seeley, R. J. (2008). Arcuate glucagon-like peptide 1 receptors regulate glucose homeostasis but not food intake. Diabetes 57, 2046–2054. doi: 10.2337/db07-1824
Sanni, S. J., Hansen, J. T., Bonde, M. M., Speerschneider, T., Christensen, G. L., Munk, S., et al. (2010). beta-Arrestin 1 and 2 stabilize the angiotensin II type I receptor in distinct high-affinity conformations. Br. J. Pharmacol. 161, 150–161. doi: 10.1111/j.1476-5381.2010.00875.x
Sapouckey, S. A., Deng, G., Sigmund, C. D., and Grobe, J. L. (2017). Potential mechanisms of hypothalamic renin-angiotensin system activation by leptin and DOCA-salt for the control of resting metabolism. Physiol. Genomics 49, 722–732. doi: 10.1152/physiolgenomics.00087.2017
Sauliere, A., Bellot, M., Paris, H., Denis, C., Finana, F., Hansen, J. T., et al. (2012). Deciphering biased-agonism complexity reveals a new active AT1 receptor entity. Nat. Chem. Biol. 8, 622–630. doi: 10.1038/nchembio.961
Scheinin, M., Lomasney, J. W., Hayden-Hixson, D. M., Schambra, U. B., Caron, M. G., Lefkowitz, R. J., et al. (1994). Distribution of alpha 2-adrenergic receptor subtype gene expression in rat brain. Brain Res. Mol. Brain Res. 21, 133–149. doi: 10.1016/0169-328x(94)90386-7
Secher, A., Jelsing, J., Baquero, A. F., Hecksher-Sorensen, J., Cowley, M. A., Dalboge, L. S., et al. (2014). The arcuate nucleus mediates GLP-1 receptor agonist liraglutide-dependent weight loss. J. Clin. Invest. 124, 4473–4488. doi: 10.1172/jci75276
Senarath, K., Kankanamge, D., Samaradivakara, S., Ratnayake, K., Tennakoon, M., and Karunarathne, A. (2018). Regulation of G Protein betagamma signaling. Int. Rev. Cell Mol. Biol. 339, 133–191.
Shah, B. P., Vong, L., Olson, D. P., Koda, S., Krashes, M. J., Ye, C., et al. (2014). MC4R-expressing glutamatergic neurons in the paraventricular hypothalamus regulate feeding and are synaptically connected to the parabrachial nucleus. Proc. Natl. Acad. Sci. U.S.A. 111, 13193–13198. doi: 10.1073/pnas.1407843111
Sharretts, J., Galescu, O., Gomatam, S., Andraca-Carrera, E., Hampp, C., and Yanoff, L. (2020). Cancer risk associated with lorcaserin – The FDA’s review of the CAMELLIA-TIMI 61 trial. N. Engl. J. Med. 383, 1000–1002. doi: 10.1056/nejmp2003873
Shibata, T., Suzuki, C., Ohnishi, J., Murakami, K., and Miyazaki, H. (1996). Identification of regions in the human angiotensin II receptor type 1 responsible for Gi and Gq coupling by mutagenesis study. Biochem. Biophys. Res. Commun. 218, 383–389. doi: 10.1006/bbrc.1996.0067
Shirai, H., Takahashi, K., Katada, T., and Inagami, T. (1995). Mapping of G protein coupling sites of the angiotensin II type 1 receptor. Hypertension 25, 726–730. doi: 10.1161/01.hyp.25.4.726
Shor-Posner, G., Grinker, J. A., Marinescu, C., and Leibowitz, S. F. (1985). Role of hypothalamic norepinephrine in control of meal patterns. Physiol. Behav. 35, 209–214. doi: 10.1016/0031-9384(85)90338-5
Singh, U., Saito, K., Toth, B. A., Dickey, J. E., Rodeghiero, S. R., Deng, Y., et al. (2021). Neuroanatomical organization and functional roles of PVN MC4R pathways in physiological and behavioral regulations. bioRxiv [preprint] doi: 10.1101/2021.03.08.431341
Small, C. J., Kim, M. S., Stanley, S. A., Mitchell, J. R., Murphy, K., Morgan, D. G., et al. (2001). Effects of chronic central nervous system administration of agouti-related protein in pair-fed animals. Diabetes 50, 248–254. doi: 10.2337/diabetes.50.2.248
Small, C. J., Liu, Y. L., Stanley, S. A., Connoley, I. P., Kennedy, A., Stock, M. J., et al. (2003). Chronic CNS administration of Agouti-related protein (Agrp) reduces energy expenditure. Int. J. Obes. Relat. Metab. Disord. 27, 530–533. doi: 10.1038/sj.ijo.0802253
Smrcka, A. V. (2008). G protein betagamma subunits: central mediators of G protein-coupled receptor signaling. Cell. Mol. Life Sci. 65, 2191–2214. doi: 10.1007/s00018-008-8006-5
Smrcka, A. V., and Fisher, I. (2019). G-protein betagamma subunits as multi-functional scaffolds and transducers in G-protein-coupled receptor signaling. Cell. Mol. Life Sci. 76, 4447–4459. doi: 10.1007/s00018-019-03275-2
Smythies, J. (2005). Section III. The norepinephrine system. Int. Rev. Neurobiol. 64, 173–211. doi: 10.1016/s0074-7742(05)64003-2
Sohn, J. W., Harris, L. E., Berglund, E. D., Liu, T., Vong, L., Lowell, B. B., et al. (2013). Melanocortin 4 receptors reciprocally regulate sympathetic and parasympathetic preganglionic neurons. Cell 152, 612–619. doi: 10.1016/j.cell.2012.12.022
Song, C. K., Vaughan, C. H., Keen-Rhinehart, E., Harris, R. B., Richard, D., and Bartness, T. J. (2008). Melanocortin-4 receptor mRNA expressed in sympathetic outflow neurons to brown adipose tissue: neuroanatomical and functional evidence. Am. J. Physiol. Regul. Integr. Comp. Physiol. 295, R417–R428.
Sonoda, N., Imamura, T., Yoshizaki, T., Babendure, J. L., Lu, J. C., and Olefsky, J. M. (2008). Beta-Arrestin-1 mediates glucagon-like peptide-1 signaling to insulin secretion in cultured pancreatic beta cells. Proc. Natl. Acad. Sci. U.S.A. 105, 6614–6619. doi: 10.1073/pnas.0710402105
Souza, R., Humbert, M., Sztrymf, B., Jais, X., Yaici, A., Le Pavec, J., et al. (2008). Pulmonary arterial hypertension associated with fenfluramine exposure: report of 109 cases. Eur. Respir. J. 31, 343–348. doi: 10.1183/09031936.00104807
Srivastava, A., Gupta, B., Gupta, C., and Shukla, A. K. (2015). Emerging functional divergence of beta-arrestin isoforms in GPCR function. Trends Endocrinol. Metab. 26, 628–642. doi: 10.1016/j.tem.2015.09.001
Staubert, C., Tarnow, P., Brumm, H., Pitra, C., Gudermann, T., Gruters, A., et al. (2007). Evolutionary aspects in evaluating mutations in the melanocortin 4 receptor. Endocrinology 148, 4642–4648. doi: 10.1210/en.2007-0138
Stocker, S. D., Meador, R., and Adams, J. M. (2007). Neurons of the rostral ventrolateral medulla contribute to obesity-induced hypertension in rats. Hypertension 49, 640–646. doi: 10.1161/01.hyp.0000254828.71253.dc
Stocker, S. D., Monahan, K. D., and Browning, K. N. (2013). Neurogenic and sympathoexcitatory actions of NaCl in hypertension. Curr. Hypertens. Rep. 15, 538–546. doi: 10.1007/s11906-013-0385-9
Strosberg, A. D. (1993). Structure, function, and regulation of adrenergic receptors. Protein Sci. 2, 1198–1209. doi: 10.1002/pro.5560020802
Takahashi, M., and Tanaka, J. (2017). GABAergic modulation of noradrenaline release caused by blood pressure changes in the rat median preoptic area. Neuroreport 28, 485–491. doi: 10.1097/wnr.0000000000000780
Tallam, L. S., Stec, D. E., Willis, M. A., da Silva, A. A., and Hall, J. E. (2005). Melanocortin-4 receptor-deficient mice are not hypertensive or salt-sensitive despite obesity, hyperinsulinemia, and hyperleptinemia. Hypertension 46, 326–332. doi: 10.1161/01.hyp.0000175474.99326.bf
Tang-Christensen, M., Larsen, P. J., Goke, R., Fink-Jensen, A., Jessop, D. S., Moller, M., et al. (1996). Central administration of GLP-1-(7-36) amide inhibits food and water intake in rats. Am. J. Physiol. 271, R848–R856.
Tian, X., Kang, D. S., and Benovic, J. L. (2014). beta-arrestins and G protein-coupled receptor trafficking. Handb. Exp. Pharmacol. 219, 173–186. doi: 10.1007/978-3-642-41199-1_9
Turton, M. D., O’Shea, D., Gunn, I., Beak, S. A., Edwards, C. M., Meeran, K., et al. (1996). A role for glucagon-like peptide-1 in the central regulation of feeding. Nature 379, 69–72.
Vitale, M., Haxhi, J., Cirrito, T., and Pugliese, G. (2020). Renal protection with glucagon-like peptide-1 receptor agonists. Curr. Opin. Pharmacol. 54, 91–101.
Vollbach, H., Brandt, S., Lahr, G., Denzer, C., von Schnurbein, J., Debatin, K. M., et al. (2017). Prevalence and phenotypic characterization of MC4R variants in a large pediatric cohort. Int. J. Obes. (Lond.) 41, 13–22. doi: 10.1038/ijo.2016.161
von Scholten, B. J., Lajer, M., Goetze, J. P., Persson, F., and Rossing, P. (2015). Time course and mechanisms of the anti-hypertensive and renal effects of liraglutide treatment. Diabet. Med. 32, 343–352. doi: 10.1111/dme.12594
Voss-Andreae, A., Murphy, J. G., Ellacott, K. L., Stuart, R. C., Nillni, E. A., Cone, R. D., et al. (2007). Role of the central melanocortin circuitry in adaptive thermogenesis of brown adipose tissue. Endocrinology 148, 1550–1560. doi: 10.1210/en.2006-1389
Wang, L., Hiller, H., Smith, J. A., de Kloet, A. D., and Krause, E. G. (2016). Angiotensin type 1a receptors in the paraventricular nucleus of the hypothalamus control cardiovascular reactivity and anxiety-like behavior in male mice. Physiol. Genomics 48, 667–676. doi: 10.1152/physiolgenomics.00029.2016
Ward, K. R., Bardgett, J. F., Wolfgang, L., and Stocker, S. D. (2011). Sympathetic response to insulin is mediated by melanocortin 3/4 receptors in the hypothalamic paraventricular nucleus. Hypertension 57, 435–441. doi: 10.1161/hypertensionaha.110.160671
Wei, Y., and Mojsov, S. (1995). Tissue-specific expression of the human receptor for glucagon-like peptide-I: brain, heart and pancreatic forms have the same deduced amino acid sequences. FEBS Lett. 358, 219–224. doi: 10.1016/0014-5793(94)01430-9
Wellman, P. J., and Davies, B. T. (1991a). Reversal of phenylpropanolamine anorexia in rats by the alpha-1 receptor antagonist benoxathian. Pharmacol. Biochem. Behav. 38, 905–908. doi: 10.1016/0091-3057(91)90261-y
Wellman, P. J., and Davies, B. T. (1991b). Suppression of feeding induced by phenylephrine microinjections within the paraventricular hypothalamus in rats. Appetite 17, 121–128. doi: 10.1016/0195-6663(91)90067-3
Wellman, P. J., Davies, B. T., Morien, A., and McMahon, L. (1993). Modulation of feeding by hypothalamic paraventricular nucleus alpha 1- and alpha 2-adrenergic receptors. Life Sci. 53, 669–679. doi: 10.1016/0024-3205(93)90243-v
Wheeler, M. B., Lu, M., Dillon, J. S., Leng, X. H., Chen, C., and Boyd, A. E. III (1993). Functional expression of the rat glucagon-like peptide-I receptor, evidence for coupling to both adenylyl cyclase and phospholipase-C. Endocrinology 133, 57–62. doi: 10.1210/endo.133.1.8391428
Wieneke, H., Svendsen, J. H., Lande, J., Spencker, S., Martinez, J. G., Strohmer, B., et al. (2016). Polymorphisms in the GNAS gene as predictors of ventricular tachyarrhythmias and sudden cardiac death: results from the DISCOVERY trial and oregon sudden unexpected death study. J. Am. Heart Assoc. 5:e003905.
Wilson, E. A., Sun, H., Cui, Z., Jahnke, M. T., Pandey, M., Metzger, P., et al. (2021). Gqalpha/G11alpha deficiency in dorsomedial hypothalamus leads to obesity resulting from decreased energy expenditure and impaired sympathetic nerve activity. Am. J. Physiol. Endocrinol. Metab. 320, E270–E280.
Wirth, A., Benyo, Z., Lukasova, M., Leutgeb, B., Wettschureck, N., Gorbey, S., et al. (2008). G12-G13-LARG-mediated signaling in vascular smooth muscle is required for salt-induced hypertension. Nat. Med. 14, 64–68. doi: 10.1038/nm1666
Woolley, M. L., Bentley, J. C., Sleight, A. J., Marsden, C. A., and Fone, K. C. (2001). A role for 5-ht6 receptors in retention of spatial learning in the Morris water maze. Neuropharmacology 41, 210–219. doi: 10.1016/s0028-3908(01)00056-9
Xu, Y., Jones, J. E., Kohno, D., Williams, K. W., Lee, C. E., Choi, M. J., et al. (2008). 5-HT2CRs expressed by pro-opiomelanocortin neurons regulate energy homeostasis. Neuron 60, 582–589. doi: 10.1016/j.neuron.2008.09.033
Yabut, J. M., Crane, J. D., Green, A. E., Keating, D. J., Khan, W. I., and Steinberg, G. R. (2019). Emerging roles for serotonin in regulating metabolism: new implications for an ancient molecule. Endocr. Rev. 40, 1092–1107. doi: 10.1210/er.2018-00283
Ye, Z. Y., and Li, D. P. (2011). Activation of the melanocortin-4 receptor causes enhanced excitation in presympathetic paraventricular neurons in obese Zucker rats. Regul. Pept. 166, 112–120. doi: 10.1016/j.regpep.2010.10.001
Young, W. S. III, and Kuhar, M. J. (1980). Noradrenergic alpha 1 and alpha 2 receptors: light microscopic autoradiographic localization. Proc. Natl. Acad. Sci. U.S.A. 77, 1696–1700. doi: 10.1073/pnas.77.3.1696
Zhan, C., Zhou, J., Feng, Q., Zhang, J. E., Lin, S., Bao, J., et al. (2013). Acute and long-term suppression of feeding behavior by POMC neurons in the brainstem and hypothalamus, respectively. J. Neurosci. 33, 3624–3632. doi: 10.1523/jneurosci.2742-12.2013
Keywords: hypothalamus, signaling pathways, hypertension, obesity, G protein-coupled receptors
Citation: Deng Y, Deng G, Grobe JL and Cui H (2021) Hypothalamic GPCR Signaling Pathways in Cardiometabolic Control. Front. Physiol. 12:691226. doi: 10.3389/fphys.2021.691226
Received: 05 April 2021; Accepted: 26 May 2021;
Published: 28 June 2021.
Edited by:
Jin Kwon Jeong, George Washington University, United StatesReviewed by:
De-Pei Li, University of Missouri, United StatesAnastasios Lymperopoulos, Nova Southeastern University, United States
Copyright © 2021 Deng, Deng, Grobe and Cui. This is an open-access article distributed under the terms of the Creative Commons Attribution License (CC BY). The use, distribution or reproduction in other forums is permitted, provided the original author(s) and the copyright owner(s) are credited and that the original publication in this journal is cited, in accordance with accepted academic practice. No use, distribution or reproduction is permitted which does not comply with these terms.
*Correspondence: Huxing Cui, huxing-cui@uiowa.edu
†These authors have contributed equally to this work