- Institute of Health Studies, Arturo Prat University, Iquique, Chile
High-altitude exposure results in hypobaric hypoxia, which affects organisms by activating several mechanisms at the physiological, cellular, and molecular levels and triggering the development of several pathologies. One such pathology is high-altitude pulmonary hypertension (HAPH), which is initiated through hypoxic pulmonary vasoconstriction to distribute blood to more adequately ventilated areas of the lungs. Importantly, all layers of the pulmonary artery (adventitia, smooth muscle, and endothelium) contribute to or are involved in the development of HAPH. However, the principal action sites of HAPH are pulmonary artery smooth muscle cells (PASMCs), which interact with several extracellular and intracellular molecules and participate in mechanisms leading to proliferation, apoptosis, and fibrosis. This review summarizes the alterations in molecular pathways related to oxidative stress, inflammation, kinase activation, and other processes that occur in PASMCs during pulmonary hypertension under hypobaric hypoxia and proposes updates to pharmacological treatments to mitigate the pathological changes in PASMCs under such conditions. In general, PASMCs exposed to hypobaric hypoxia undergo oxidative stress mediated by Nox4, inflammation mediated by increases in interleukin-6 levels and inflammatory cell infiltration, and activation of the protein kinase ERK1/2, which lead to the proliferation of PASMCs and contribute to the development of hypobaric hypoxia-induced pulmonary hypertension.
Introduction
At high altitude – 3,000 m above sea level – the partial pressure of oxygen (PaO2) is decreased, creating a condition termed hypobaric hypoxia; this condition is characterized by reduced bioavailability of oxygen at the organism, tissue, and cell levels, which clearly alters organism homeostasis (Chavala, 2018; Bilo et al., 2019). Currently, more than 140 million people worldwide are working and living under the condition of hypobaric hypoxia (Moore et al., 1998; West, 2012), and the consequences associated with this exposure constitute a substantial public health burden (Herrera et al., 2015a; Brito et al., 2020).
High-altitude exposure results in different pathologies, such as acute mountain sickness, chronic mountain sickness (or Monge’s disease), acute cerebral edema (ACE), and high-altitude pulmonary edema (HAPE); however, other important pathology associated with high-altitude exposure is high-altitude pulmonary hypertension (HAPH) (León-Velarde et al., 2005; Robinson et al., 2017). HAPH corresponds to classification 3.6 for general pulmonary hypertension (Simonneau et al., 2013). The cut-off point for all types of pulmonary hypertension is a mean pulmonary arterial pressure (mPAP) ≥ 25 mm Hg (Galiè et al., 2016); however, the cut-off point for HAPH is considered to be an mPAP ≥ 30 mm Hg according to a consensus reached in 2005 (León-Velarde et al., 2005). This condition is observed in subjects who are chronically exposed to high-altitude and is due to hypoxic pulmonary vasoconstriction and subsequent pulmonary artery remodeling (Sommer et al., 2008; Neupane and Swenson, 2017).
Hypoxic pulmonary vasoconstriction is the first response to hypoxia – and certainly to hypobaric hypoxia – due to the decrease in alveolar oxygen pressure (Moudgil et al., 2005; Siques et al., 2018). This intrinsic vasoconstrictor mechanism is activated to distribute oxygen to more adequately ventilated areas of the lung to improve oxygen delivery (Von Euler and Liljestrand, 1946). However, when alveolar hypoxia is maintained, the hypoxic pulmonary vasoconstriction mechanism is exacerbated and can trigger vascular remodeling (León-Velarde et al., 2010; Tan et al., 2017) and, subsequently, pulmonary hypertension, or HAPH (Penaloza et al., 1964; Xu and Jing, 2009; León-Velarde et al., 2010). As mentioned above, pulmonary hypertension is a disease characterized by pulmonary vascular remodeling leading to a progressive increase in pulmonary vascular resistance; however, when this pathology is maintained, the afterload on the right ventricle is increased, and right ventricular hypertrophy occurs, ultimately resulting in right heart failure and death (Mikhael et al., 2019).
Importantly, all layers of the pulmonary artery (the adventitia, smooth muscle, and endothelial cell layers) and the surrounding cells (mast cells, fibroblasts, and macrophages) contribute to or are involved in the development of HAPH (Vodenicharov, 2012; Siques et al., 2018). However, some of the principal action sites of HAPH are pulmonary artery smooth muscle cells (PASMCs), which interact with several extracellular and intracellular molecules and participate in diverse mechanisms – based on the evolution and degree of hypoxia – that lead to proliferation, apoptosis, and fibrosis (Udjus et al., 2019). The aims of this review are to discuss the molecular alterations related to oxidative stress, inflammation, and protein kinase activation that occur under hypobaric hypoxia-induced pulmonary hypertension, particularly in PASMCs, and to identify current and potential molecular targeted pharmacological treatments.
PASMCs and Hypobaric Hypoxia
The structure of smooth muscle tissue is different from that of other muscle tissue subtypes, and the spindle shape of mononucleated smooth muscle cells (SMCs) permits close contact among cells in vessel walls (Vodenicharov, 2012). The thickness of the medial layer, which is composed mainly of SMCs, greatly determines pulmonary artery resistance and pressure (Siques et al., 2018). Under hypoxic conditions, the muscularization and contractibility of these vessels are enhanced (Meyrick and Reid, 1979), constituting a key feature of pulmonary vascular remodeling and subsequent hypobaric hypoxia-induced pulmonary hypertension (Pak et al., 2007). This effect has been observed both in chronic hypobaric hypoxia (Penaloza and Arias-Stella, 2007) and, albeit to a lesser extent, in intermittent hypobaric hypoxia (Brito et al., 2015). However, this effect in acute hypobaric hypoxia is controversial (Pak et al., 2007), since some studies showed PASMC proliferation under acute hypoxia, while others did not, as demonstrated in a meta-analysis (Pak et al., 2007). Therefore, more studies are necessary to clarify the effect of acute hypobaric hypoxia exposure on SMCs.
As mentioned above, vascular remodeling due to pulmonary artery vasoconstriction is a common characteristic of pulmonary hypertension under hypobaric hypoxia, during which important changes occur in the muscular vessel medial layer that result principally from accumulation of vascular SMCs. Thus, hypobaric hypoxia plays an important role in triggering the excessive proliferation of vascular SMCs and contributing to vascular remodeling (Tan et al., 2017). The processes mentioned are related to oxidative stress, kinase protein, and inflammation in SMCs under hypobaric hypoxia and are discussed below.
Oxidative Stress
Under physiological conditions, the presence of controlled concentrations of reactive oxygen species (ROS) molecules in the organism is necessary to modulate several pathways involved in maintaining homeostasis and cellular differentiation (Li et al., 2016). ROS are oxidative and unstable molecules derived from oxygen. The most well-known ROS molecules are superoxide anions (O2.–), hydroxyl radicals (.OH), peroxyl radicals (ROO.), and alkoxy radicals (RO.). Additionally, some non-radicals are either oxidizing or easily converted into oxidative species; these non-radicals include hypochlorous acid (HOCl), ozone (O3), singlet oxygen (1O2), hydrogen peroxide (H2O2), and peroxynitrite (ONOO–) (Li et al., 2016). However, when the level of ROS increases due to environmental or chemical stimuli and is not correspondingly reduced by endogenous antioxidant systems, such as those involving superoxide dismutase (SOD), catalase (CAT), glutathione peroxidase/reductase (GSH-PX/RX), and peroxiredoxin/thioredoxin (Herrera et al., 2015b; Peoples et al., 2019), oxidative stress is triggered. This oxidative stress results in damage to DNA, proteins, and lipids as well as mitochondrial permeability transition pore (MPTP) activation, mitochondrial dysfunction, and cell death (Richardson and Schadt, 2014; Farías et al., 2016, 2017).
Despite the apparent paradox – that O2 bioavailability is decreased under hypoxic conditions – there is evidence showing that increased ROS levels trigger oxidative stress in hypobaric hypoxia (Klemm et al., 2011; Lüneburg et al., 2016; Siques et al., 2018). Notably, a study on the lungs of rats exposed to hypobaric hypoxia showed that the H2O2 concentration increased and that SOD and GSH-PX activity decreased, producing oxidative stress (Xu et al., 2016). Interestingly, hypoxia and consequent pulmonary hypertension are related to oxidative stress (Bowers et al., 2004; Guzy and Schumacker, 2006; Demarco et al., 2010). This is supported by the results of a human study showing that ROS levels are two- to threefold higher in patients with pulmonary hypertension than in control subjects (Wong et al., 2013) and an animal study showing that the levels of a plasmatic oxidative biomarker (malondialdehyde) are elevated in rats with pulmonary hypertension induced by intermittent or chronic hypobaric hypoxia exposure (Lüneburg et al., 2016). Moreover, Xu et al. (2016) demonstrated that ROS and H2O2 levels are elevated in PASMCs cultured under hypoxic conditions and that the increases in the levels of these molecules are related to PASMC proliferation, contributing to the development of pulmonary hypertension.
NADPH Oxidase
Regarding the sources of ROS, studies have proposed that mitochondria and nicotinamide adenine dinucleotide phosphate (NADPH) oxidase are the major ROS producers in the cardiovascular system (Daiber et al., 2017; Fukai and Ushio-Fukai, 2020). Additionally, it has been demonstrated the involvement of mitochondria-derived ROS in the development of normobaric hypoxia-induced pulmonary hypertension (Adesina et al., 2015; Snow et al., 2020). Although, previous studies have claimed that the principal source of ROS in the cardiovascular system is the NADPH oxidase complex (Moudgil et al., 2005), and NADPH oxidase-induced oxidative stress may play an important role in pulmonary artery hypertension induced by hypoxia. In addition, studies on PASMCs under normobaric hypoxia in both humans (Ismail et al., 2009) and animals (Mittal et al., 2007) have shown that the NADPH oxidase complex (specifically the Nox4 isoform) is overexpressed, contributing to PASMC proliferation leading to pulmonary hypertension. Moreover, NADPH oxidase expression and subsequent oxidative stress are increased in the lungs of rat exposed to hypobaric hypoxia, effects that are also related to PASMC proliferation through depletion of the transcription factor CREB, triggering pulmonary hypertension (Klemm et al., 2011).
Although there have not been many studies showing the specific expression and activity of the Nox4 isoform related to PASMC proliferation and pulmonary hypertension under hypobaric hypoxia, however, it is important to mention that Nox4 is the principal isoform expressed in PASMCs of patients with pulmonary hypertension (Sturrock et al., 2006). Moreover, a recent study by He et al. (2020) showed that inhibition of oxidative stress induced by hypoxia exposure reduces PASMC proliferation by decreasing hypoxia inducible factor-1α (HIF-1α) levels and Nox4 expression. In addition, a study in mice exposed to both intermittent and chronic hypobaric hypoxia showed that Nox4 expression in the lungs increased and was higher after chronic than intermittent exposure (Lüneburg et al., 2016). Therefore, based on the above evidence, Nox4 could be related to the PASMC proliferation and pulmonary hypertension induced by hypobaric hypoxia exposure.
Regarding Nox4 pathways, studies have showed that transforming growth factor-β (TGF-β) promotes PASMC proliferation through Nox4 expression (Sturrock et al., 2006; Zhou et al., 2013), and Nox4-mediated production of H2O2 depends on TGF-β levels (Martin-Garrido et al., 2011). Notably, hypoxia also increases TGF-β levels in PASMCs, contributing to proliferation (Zhang et al., 2018). Zhang et al. (2018) also determined that rats exposed to chronic hypobaric hypoxia exhibited increased TGF-β levels and phosphorylation of smad3 (a key downstream molecule in the TGF-β signaling pathway) in pulmonary arteries, contributing to pulmonary hypertension and remodeling. Importantly, that study also showed that primary pulmonary adventitial fibroblasts and macrophages contributed to the TGF-β secretion under this hypoxic condition; these mechanisms could thus play an important role in PASMC proliferation and pulmonary artery remodeling (Zhang et al., 2018).
As mentioned before, Nox4 could play an important role in the PASMC proliferation under hypobaric hypoxia-induced pulmonary hypertension. However, it is important to highlight that Nox2 isoform mediating vascular remodeling is also demonstrated in both normobaric (Liu et al., 2005) and hypobaric (Norton et al., 2013) hypoxia-induced pulmonary hypertension. Although – to our knowledge – we did not find studies particularly in PASMCs proliferation under this latter condition, which results interesting to consider in future studies.
Another molecule that could contribute to oxidative stress is nitric oxide (NO), which is an important endogenous vasodilator that act directly in SMCs and is altered under hypobaric hypoxia. One study has revealed that NO bioavailability is decreased in the pulmonary arteries of rats with hypobaric hypoxia-induced pulmonary hypertension (Siques et al., 2014). This phenomenon could be explained by the reaction between NO and NADPH oxidase-produced O2.–, which produces ONOO–, contributing to oxidative stress (Barman et al., 2014; Siques et al., 2014; Lüneburg et al., 2016). Therefore, based on the above evidence, NADPH oxidase could play an important role in the oxidative stress that occurs in PASMCs under hypobaric hypoxia.
Pathways Related to Oxidative Stress
Reactive oxygen species are also involved in regulation of cell signaling through the mitogen-activated protein kinase (MAPK) pathway, which is associated with a variety of cellular effects, including proliferation, survival, death, and transformation (Son et al., 2013). One study on rats exposed to hypobaric hypoxia showed that the constitutive MAPK termed apoptosis signal-regulating kinase 1 (ASK1), which is known to be critical in the remodeling processes of pulmonary artery hypertension, participates in PASMC proliferation through activation of p38 MAPK and endothelin-1 (ET-1) in response to oxidative stress (Wilson et al., 2020).
ET-1
Endothelin-1 markedly affects SMCs of the vascular wall via its potent vasoconstrictor activity and is related to hypertrophic, mitogenic, and antiapoptotic effects on vascular SMCs (Wu et al., 2007). ET-1 is produced primarily by endothelial cells but can also be secreted by epithelial cells, macrophages, and fibroblasts. Importantly, studies have shown that SMCs can also produce ET-1 (Yanagisawa et al., 1988; Miller et al., 1993; Kosacka and Brzecka, 2021). The activity of ET-1 is increased in hypoxia; this increase is related to the level of HIF-1, since ET-1 contains a HIF-1 binding site at base pair 118 (Yamashita et al., 2001). Additionally, a study has demonstrated that ET-1 may play autocrine and paracrine roles (in SMCs and endothelial cells) in the remodeling of PASMCs during the development of pulmonary hypertension under hypobaric hypoxia (Nakanishi et al., 1999).
As mentioned above, ET-1 may be associated with oxidative stress, since a study in fetal PASMCs showed that increases in the levels of this protein are related to production of large amounts of ROS and that the use of antioxidants or NADPH oxidase inhibitors induces apoptosis of fetal PASMCs (Wedgwood et al., 2001). The above findings are supported by a study showing that hypoxia-induced ET-1 promotes SMC proliferation via NADPH oxidase activation, contributing to pulmonary hypertension induced by hypoxia exposure (Wu et al., 2007). Moreover, another study determined that circulating ET-1 levels were significantly higher in HAPH patients than in healthy high-altitude inhabitants (Kojonazarov et al., 2012), which is supported by the aforementioned study by Nakanishi et al. (1999).
Endothelin-1 has been found to be related to upregulation of nuclear factor of activated T cells isoform c3 (NFATc3) in the pulmonary arteries of mice under chronic hypobaric hypoxia (De Frutos et al., 2011). NFATc3 is a Ca2+-dependent transcription factor that has been implicated in the PASMC proliferative response and pulmonary hypertension under chronic hypobaric hypoxia in adult mice (De Frutos et al., 2007; Bierer et al., 2011). The role of transcription factors in this process will be discussed later.
Peroxisome Proliferator-Activated Receptor
Other molecules related to oxidative stress in PASMCs are the peroxisome proliferator-activated receptors (PPARs), which regulate several genes involved in cell metabolism, growth, and differentiation (Lefebvre et al., 2006). PPARγ is expressed in several cells, including pulmonary endothelial cells and PASMCs, where it plays an important role in normal pulmonary vascular function (Green et al., 2011, 2012). A study on human PASMCs exposed to hypoxia determined that PPARγ downregulation is related to increases in Nox4-mediated H2O2 production, ERK1/2 levels, and nuclear factor κB (NF-κB) levels (Lu et al., 2013). However, PPARγ activation attenuates signaling pathways associated with SMC proliferation and remodeling (Nisbet et al., 2010). Moreover, loss of PPARγ expression is associated with the development of pulmonary hypertension induced by hypoxia; this finding is supported by a study showing that the expression of PPARγ is decreased in the lungs of rodents with pulmonary hypertension induced by chronic hypoxia exposure (Nisbet et al., 2010). Therefore, alterations in PPARγ under hypoxia are related to kinase activation, oxidative stress, and inflammation, which could contribute to SMCs proliferation under hypobaric hypoxia.
As previously mentioned, hypobaric hypoxia-induced oxidative stress is also related to protein kinase activation and contributes to the initiation of PASMC proliferation and subsequent pulmonary hypertension. These pathways are discussed in the next section.
Protein Kinase Activity
JAK2
An important kinase protein related to pulmonary hypertension is mammalian Janus kinase (JAK). This kinase undergoes autophosphorylation at tyrosine residues, which generates binding sites for phosphorylated signal transducer and activator of transcription (STAT). The JAK family contains four evolutionarily conserved members, JAK1, JAK2, JAK3, and tyrosine kinase 2 (TYK2). It has been demonstrated that mRNA expression of JAK1, JAK2, and JAK3 is increased in PASMCs after hypoxia exposure. In addition, inhibition of JAK2 blocks STAT3 expression in PASMCs under hypoxia (Wang et al., 2005). Interestingly, the levels of tyrosine 705-phosphorylated STAT3 (PY-STAT3) are increased in the lungs of rats with pulmonary hypertension induced by hypobaric hypoxia (Huang et al., 2019).
JAK2 is involved in the regulation of various processes relevant to cell survival, proliferation, activation, and differentiation (O’Shea and Plenge, 2012; Thomas et al., 2015). A recent study demonstrated that the JAK2/STAT3 pathway is involved in the proliferation of PASMCs under hypoxic conditions; additionally, mice with SMC-specific Jak2 knockout show reduced right ventricular systolic pressure and right ventricular hypertrophy under hypoxic conditions, implying improved function of pulmonary blood vessels (Zhang et al., 2020). Moreover, the same study indicated that the JAK2/STAT3 pathway induces pulmonary artery remodeling by enhancing cyclin A2 expression in PASMCs.
ERK
Notably, some molecular channels are also related to protein kinase activation during PASMC proliferation under hypobaric hypoxia, as indicated by a study showing that transient receptor potential melastatin 7 (TRPM7), which acts as a magnesium channel in mammalian cells, is downregulated in PASMCs of patients with pulmonary hypertension and in rats with hypobaric hypoxia-induced pulmonary hypertension. In particular, inhibition of TRPM7 increased the proliferation and apoptosis resistance of PASMCs in vitro through activation of the MEK/ERK pathway (Xing et al., 2019). The role of ERK activation in PASMC proliferation could be supported by the finding that ERK activity is increased in rats with hypobaric hypoxia exposure-induced pulmonary hypertension by a metabolite derived from the lipoxygenase pathway [12(S)-hydroxyeicosatetraenoic acid (12(S)-HETE)], which is related to PASMC proliferation (Preston et al., 2006).
ERK1/2 also mediates the activation of protein kinase C (PKC) and G-protein coupled receptors, and this mechanism induces SMC proliferation under hypoxia (Dempsey et al., 1996; Lannér et al., 2005). Importantly, oxidative agents such as H2O2 and superoxide result in PKC activation independent of the classical PKC cofactor diacylglycerol; indeed, a study showed that PKC activation induced by H2O2 results in the activation of the L-type Ca2+ channel in SMCs, highlighting that inhibition of PKC activity inactivates this channel (Perez-Vizcaino et al., 2010). Therefore, we suggest that hypoxia exposure can alter oxidative status and kinase activation, leading to impairment of Ca2+ homeostasis and contractibility in PASMCs.
SphK1
Sphingosine kinase-1 (SphK1) is an important lipid kinase that is also related to PASMC proliferation. Its product sphingosine-1-phosphate (S1P) induces PASMC proliferation through activation of early growth response protein-1 (Erg-1), a product of platelet-derived growth factor (PDGF)-induced ERK activation (Sysol et al., 2016). This pathway is also activated during hypoxia-induced PASMC proliferation and pulmonary hypertension, and could be regulated by microRNA (miR-1) (Sysol et al., 2018). Interestingly, a recent study has shown that increases in S1P result in dephosphorylation of yes-associated protein (YAP), a transcriptional regulator, which induces an increase in miR-130a/b contributing to PASMC proliferation and pulmonary hypertension (Shi et al., 2021). The activity of the transcription factor YAP will be discussed later.
Inflammation
Inflammatory processes are present in various types of pulmonary hypertension in both humans and animals, contributing to pulmonary vascular remodeling (Savale et al., 2009; Tuder, 2017). It is important to mention the role of inflammatory cells, since in mice exposed to hypoxia, monocytes migrate to the lung perivascular space and differentiate into interstitial macrophages (Florentin et al., 2018). These cells contribute to the development and progression of acute or chronic inflammatory responses through the secretion of inflammatory cytokines [TNF-α, IL-1β, and interleukin-6 (IL-6)] in the pathogenesis of inflammatory lung disease (Lee et al., 2021). Interestingly, macrophages are involved in PASMC proliferation, and studies have demonstrated that the numbers of macrophages in perivascular cells increase under hypobaric hypoxia (Horita et al., 2013; Yu Y. R. et al., 2013).
MIF
Additionally, both macrophages and endothelial cells can produce a proinflammatory cytokine termed macrophage migration inhibitory factor (MIF). Under stress conditions (such as hypoxia), this molecule inhibits the random migration of macrophages and stimulates TNF-α, IL-1β, IL-6, and IL-8 production (Bloom and Bennett, 1966). MIF has been found to be related to proliferation of SMCs (Calandra et al., 1994; Chen et al., 2004; Onodera et al., 2004), and a study on rats under hypobaric hypoxia showed that an increase in MIF induces proliferation of PASMCs through activation of ERK1/2 and JNK proteins, contributing to the pulmonary hypertension (Zhang et al., 2012).
PTEN
The macrophage accumulation and IL-6 production are regulated by PTEN, since a study on rats exposed to hypobaric hypoxia has shown that selective inhibition of PTEN in PASMCs increases their proliferation and impairs pulmonary hypertension; highlighting that depletion of PTEN exacerbated macrophage accumulation and IL-6 production (Horita et al., 2013).
PTEN is a phosphatase enzyme that suppresses multiple signaling networks involved in cell proliferation, survival, and inflammation (Huang et al., 2019). It also is a specific regulator and protector against pathological vascular SMC remodeling, which is inactivated in the lungs (Huang et al., 2019) and PASMCs in human pulmonary hypertension (Horita et al., 2013). However, a study on the lungs of rats exposed to hypobaric hypoxia found that PTEN expression was decreased but that the membrane localization of its regulator caveolin-1 (cav-1) was unchanged, which could contribute to hypoxia-induced pulmonary hypertension (Huang et al., 2019). This latter finding needs further validation in PASMCs proliferation under hypobaric hypoxia.
IL-6
The level of IL-6 was demonstrated to be increased in mouse lungs and cultured PASMCs after exposure to normobaric hypoxia, elucidating that IL-6 stimulates human PASMC migration (Savale et al., 2009). In addition, mice with IL-6 deficiency exposed to hypoxia showed reductions in pulmonary hypertension, vascular remodeling, and macrophage recruitment in the lung (Savale et al., 2009). Importantly, increases in IL-6 have also been showed to occur in the lungs of mice exposed to chronic hypobaric hypoxia (Maston et al., 2018).
Interleukin-6 acts through two mechanisms in organisms to exert its biological effects. For example, activation of the membrane-bound IL-6 receptor (IL6R) is related to the classical signaling pathway that controls homeostatic processes and immunological outcomes, such as host defense against bacterial infections and activation of anti-inflammatory and regenerative epithelial pathways (Scheller et al., 2011). On the other hand, IL-6 and its soluble receptor sIL-6R form a complex of the IL-6 trans-signaling pathway that is related to pathological responses such as tissue fibrosis, inflammatory arthritis, and cardiovascular disease (Hunter and Jones, 2015). Moreover, the binding of IL-6 to either the membrane-bound IL6R or sIL-6R triggers the oligomerization of the ubiquitous transmembrane protein gp130, resulting in activation of the JAK/STAT signal transduction pathway (Campbell et al., 2014). Notably, there is evidence showing that gp130 expression is increased in the lungs of rats exposed to hypobaric hypoxia (Huang et al., 2019).
A recent study showed the importance of IL6R and its potential prosurvival effects in pulmonary hypertension under hypoxic conditions (Tamura et al., 2018). Tamura et al. (2018) exposed transgenic mice (deficient in IL6R in SMCs) to chronic normobaric hypoxia and found that they exhibited decreased activation of STAT3 and expression of antiapoptotic proteins, such as MCL-1 and BCL2, in the lungs. Moreover, the mice showed decreased collagen deposition, inflammatory cell infiltration, and pulmonary hypertension (Tamura et al., 2018).
Interestingly, inhibition of the pathway involving the IL-6/sIL-6R complex reduces the pulmonary artery remodeling and pulmonary hypertension that occur under hypobaric hypoxia, highlighting that activation of the IL-6/sIL-6R complex enhances PASMC migration (but not proliferation) and that this migration process requires STAT3 activation and increases in [Ca2+]i (Maston et al., 2018). These results indicate that IL-6, IL6R, and sIL-6R could play an important role in hypoxia-induced PASMC proliferation and pulmonary hypertension.
Caspase-1
Another interesting protein is the inflammasome effector caspase-1, which mediates inflammation through activation of the proinflammatory cytokines IL-18 and IL-1β (Udjus et al., 2019). A study showed that caspase-1 deficiency reduces pulmonary hypertension in mice under normobaric hypoxia, mainly by reducing PASMC proliferation. In addition, caspase-1 activation likely induces PASMC proliferation via the IL-18/IL-1β and IL-6/STAT3 signaling pathways. Therefore, caspase-1 could be another therapeutic target for hypoxia-induced pulmonary hypertension (Udjus et al., 2019).
MKL1
The transcriptional regulator megakaryocytic leukemia 1 (MKL1) is also an important modulator of SMCs under hypobaric hypoxia (Yuan et al., 2014). MKL1 inhibition attenuates pulmonary hypertension in rats exposed to chronic hypobaric hypoxia (Yuan et al., 2014). It also decreases the expression of inflammatory cytokines (TNF-α and IL-6) and chemokines responsible for recruitment of inflammatory cells, such as C–C motif chemokine ligand 2 (CCL2) and CCL5, resulting in decreased aggregation of cell types including macrophages, leukocytes, and T lymphocytes in the lungs (Yuan et al., 2014).
In addition, a study on calves has indicated that hypobaric hypoxia-induced pulmonary hypertension is associated with increased production of extracellular matrix proteins, specifically collagen type I, by SMCs (Crouch et al., 1989). On the other hand, a study based on this finding by Yuan et al. (2014) showed that depletion of MKL1 prevents the increased synthesis of endogenous collagen type I mRNA in vascular SMCs under hypoxic conditions (Yuan et al., 2014). Therefore, MKL1 synthesized in SMCs likely participates in pulmonary hypertension induced by hypobaric hypoxia through fibrogenesis and inflammation.
Transcription Factors
As mentioned previously, YAP is a transcriptional regulator that, together with WW domain-containing transcription regulator 1 (WWTR1 or TAZ), contributes to PASMC proliferation and subsequent pulmonary hypertension under hypoxic conditions through inhibition of cyclooxygenase 2 (COX2) activity (Dieffenbach et al., 2017). Additionally, a recent study has shown that YAP induces the activation of forkhead box M1 (FOXM1), a transcription factor that contributes to PASMC proliferation and pulmonary hypertension (Zhang et al., 2021).
FOX
The forkhead box (FOX) proteins are a superfamily of transcriptional regulators that mediate numerous functions during development and adulthood (Lam et al., 2013). FOXM1 is a transcription factor that plays a crucial role in the maintenance and differentiation of epithelial cells lining the airways, especially during the embryonic stage, during which it is essential in the formation and proliferation of pulmonary vessels (Raoof and Daoud, 2019). In mice with hypoxia-induced pulmonary artery hypertension, FOXM1 mRNA and protein levels are increased in the lungs; moreover, constitutive SMC-specific knockdown of FOXM1 inhibits hypoxia-induced pulmonary hypertension and prevents the proliferation of PASMCs, although it does not inhibit PASMC migration (Dai et al., 2018). Importantly, FOXM1 in PASMCs is regulated by HIF-1α under normoxic conditions but stimulated by HIF-2α under hypoxic conditions (Xia et al., 2009). Therefore, FOXM1 can be modulated by HIF-1α and HIF-2α, but its response to oxygen (according to its expression level) and its distribution in tissues differ (Raghavan et al., 2012). Hypoxia upregulates FOXM1 expression in SMCs by increasing the release of growth factors and inflammatory cytokines from endothelial cells, contributing to pulmonary artery hypertension (Raoof and Daoud, 2019).
Another important transcription factor that regulates the cell cycle is forkhead box protein O1 (FOXO1), which has been demonstrated to play a role opposite that of FOXM1 (Sengupta et al., 2013). FOXO1 expression is also decreased in the lungs of humans with another type of pulmonary artery hypertension. Supporting the role of FOXO1 under hypoxia, it has been demonstrated that inhibition of FOXO1 significantly increases PASMC proliferation (Savai et al., 2014).
There is a relationship among FOXM1, FOXO1, and the cell cycle regulator polo-like kinase 1 (PLK1) (Wilson et al., 2019). The latter has been shown to be an important upregulator of FOXM1 expression, contributing to PASMC proliferation in subjects with pulmonary artery hypertension (Wilson et al., 2019). Inhibiting the actions of either PLK1 or FOXM1 in human PASMCs increases the expression of the cyclin-dependent kinase inhibitor 1B (p27kip1) protein, which inhibits the cell cycle progression of PASMCs. In addition, specific inhibition of FOXO1 increases the expression of FOXM1 (Wilson et al., 2019).
More studies are necessary to assess the roles of FOX proteins in pulmonary hypertension under hypobaric hypoxia. However, a recent study has shown that the levels of forkhead box C1 (FOXC1) are increased in the pulmonary arteries of mice exposed to hypobaric hypoxia and in PASMCs cultured under hypoxic conditions (Yang et al., 2020). In addition, the viability and migration ability of PASMCs transfected with a FOXC1 overexpression plasmid have been found to be increased under hypoxic conditions. Moreover, the circular RNA mmu_circ_0000790 regulates the expression of FOXC1 by binding to miR-374c, establishing an important regulatory axis of vascular remodeling and pulmonary hypertension under hypobaric hypoxia (Yang et al., 2020). Although these new molecules and pathways were originally described in the context of other types of hypertension, they have been demonstrated to also play a role in hypoxic pulmonary hypertension.
Wnt/β-Catenin
Another pathway that is important to mention is the Wnt/β-catenin pathway. β-Catenin is a transcription factor involved in PASMC proliferation, and its expression can be inhibited by Wnt5a (in a non-canonical Wnt pathway) in hypoxia-induced pulmonary hypertension (Yu X. M. et al., 2013). Wnt5a levels are decreased under hypoxic conditions (Yu X. M. et al., 2013). Therefore, β-catenin activity is predominant under hypoxia, as supported by a recent study showing that the expression of β-catenin is increased in the lungs of rats exposed to hypobaric hypoxia (Huang et al., 2019). On the other hand, induction of Wnt5a overexpression in recombinant mice significantly inhibits β-catenin expression, human PASMC proliferation, and subsequent hypoxia-induced pulmonary hypertension (Yu X. M. et al., 2013; Jin et al., 2015). Therefore, the Wnt/β-catenin pathway could be important to consider in future studies on hypobaric hypoxia-induced PASMC proliferation and pulmonary hypertension.
Erg-1
The redox-sensitive transcription factor Erg-1 regulates cell proliferation, and the levels of this transcription factor are increased in newborn calves with pulmonary artery hypertension and remodeling induced by chronic hypobaric hypoxia. The increases in Erg-1 are related to protein kinase activation and oxidative stress, since oxidant/antioxidant imbalance due to chronic hypobaric hypoxia-induced loss of extracellular SOD activity in the pulmonary artery results in Erg-1 activation and contributes to PASMC proliferation through the ERK1/2 pathway (Hartney et al., 2011).
Proposed schematics of PASMC proliferation under hypobaric hypoxia summarizing the molecular pathways and their cellular interactions discussed in this review are depicted in Figures 1, 2, respectively.
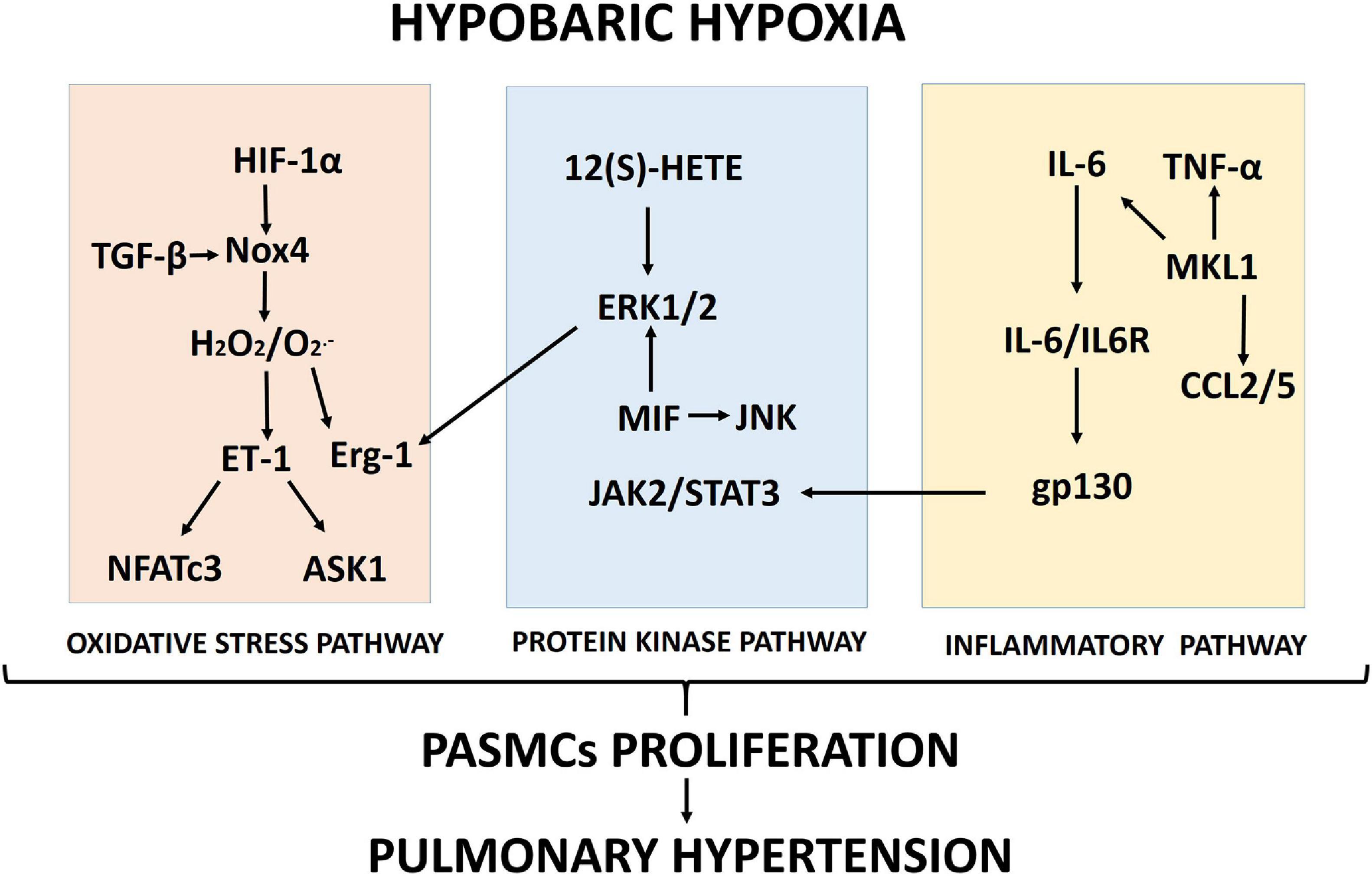
Figure 1. Proposed scheme summarizing the molecular pathways (oxidative stress, protein kinase and inflammation) involved in pulmonary artery smooth muscle cell (PASMC) proliferation induced by hypobaric hypoxia exposure.
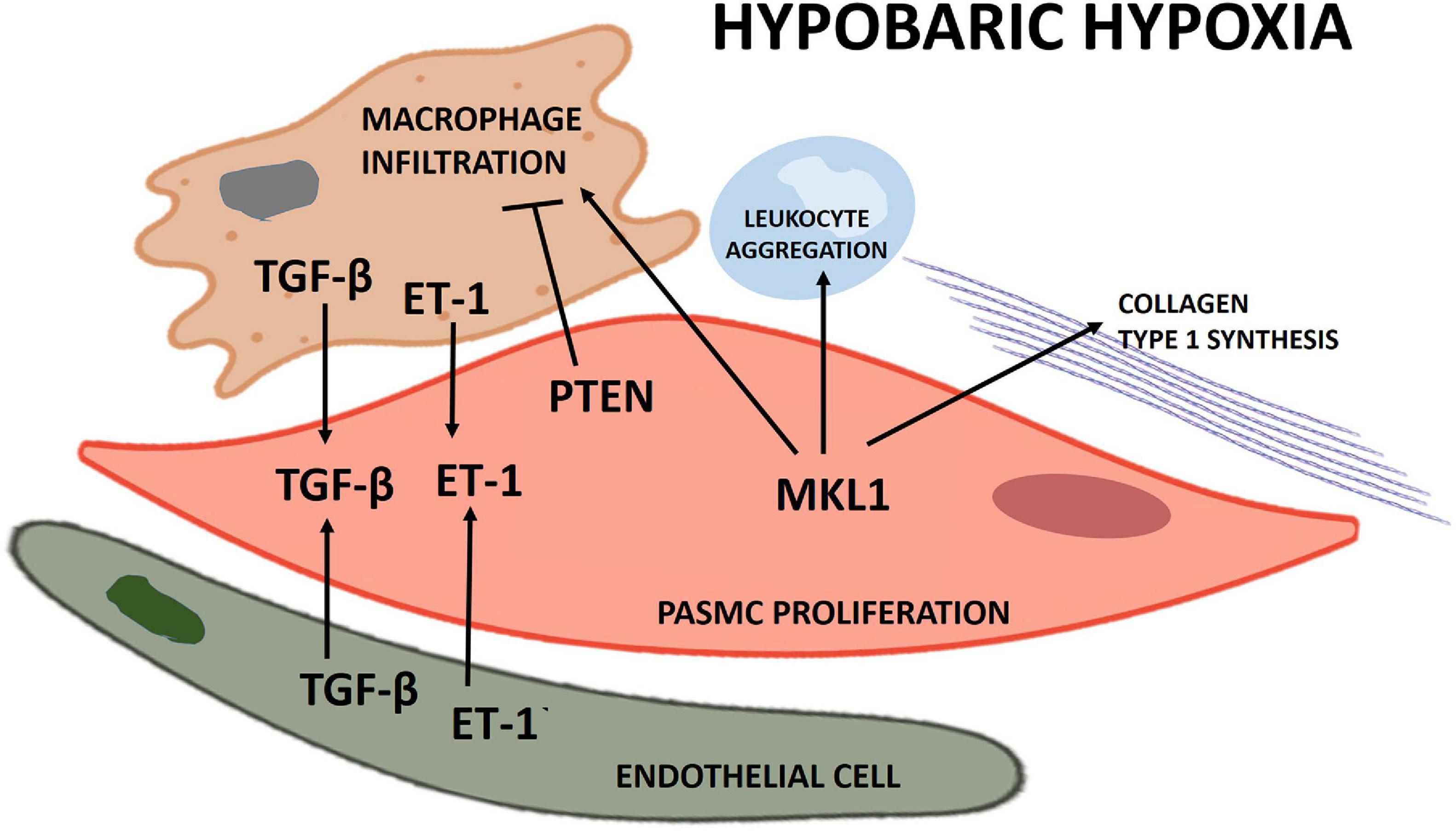
Figure 2. Scheme of the principal cellular interactions involved in pulmonary artery smooth muscle cell (PASMC) proliferation under hypobaric hypoxia.
Pharmacological Interventions to Decrease PASMCs Proliferation Under Hypoxic Conditions
Additionally, we review the current interventions for PASMCs proliferation under hypoxic conditions.
One interesting study has shown that treatment with histone deacetylase inhibitors (HDIs), such as suberoylanilide hydroxamic acid (SAHA) and butyrate (BUR), increases the expression of endothelial NO synthase (eNOS) in vascular SMCs, producing an elevation in NO that induces upregulation of p21 expression and cleavage of caspase 3 (a protein related to cell cycle arrest and apoptosis) and decreases hypobaric hypoxia-induced vascular SMC remodeling (Tan et al., 2017). However, importantly, a significant decrease in mPAP does not occur (Tan et al., 2017).
Resveratrol, a plant-derived polyphenolic compound and a phytoestrogen, has been demonstrated to alleviate hypobaric hypoxia-induced pulmonary hypertension through its anti-inflammatory, antioxidant, and antiproliferative effects (Xu et al., 2016). This compound decreased neutrophil infiltration in the lungs of rats exposed to hypobaric hypoxia and suppressed the expression of inflammatory molecules such as phosphorylated NF-kB, IL-6, IL-1β, and TNF-α. Importantly, resveratrol inhibited the production of ROS in PASMCs (probably by increasing Nrf-2/Trx-1 axis signaling) and suppressed the proliferation of PASMCs. Additionally, the decrease in the proliferation of PASMCs by resveratrol could be explained by the decrease in HIF-1α expression through MAPK/ERK1 and PI3K/AKT inhibition (Xu et al., 2016). In fact, in support of this hypothesis, a recent study by He et al. (2020) showed that resveratrol inhibited hypoxia-induced oxidative stress and the proliferation of rat PASMCs by decreasing HIF-1α levels and Nox4 expression.
Other interesting medicines derived from Tibetan plants have been used to reduce hypoxia-induced pulmonary hypertension. Echinacoside, a phenylethanoid glycoside from the Tibetan herbs Lagotis brevituba Maxim and Cistanche tubulosa, shows antiproliferative effects in rat PASMCs under normobaric hypoxia (Gai et al., 2014). In addition, echinacoside was administered to rats under hypobaric hypoxia (4,500 m), and it reduced pulmonary artery remodeling and pulmonary hypertension (Gai et al., 2020). Administration of another traditional Tibetan medicine, Tsantan Sumtang, prevented pulmonary hypertension in rats exposed to hypobaric hypoxia (4,500 m), reducing pulmonary vascular cell proliferation probably by suppressing cyclin D1 and cyclin-dependent kinase 4 (CDK4) expression through inhibition of p27kip1 degradation (He et al., 2018).
Consistent with the relation between JAK2/STAT3 pathway activation and cell proliferation, administration of tanshinone IIA (Tan), a fat-soluble pharmacologically active ingredient in Danshen, decreases hypoxia-induced PASMC proliferation via apoptosis activation through inhibition of the JAK2/STAT3 pathway both in vivo (in rats) and in vitro (Chen et al., 2014).
Aloperine, an alkaloid isolated from Sophora alopecuroides L., possesses a variety of pharmacological activities, such as anti-inflammatory and antitumor activities (Chang et al., 2019). Human PASMCs in which proliferation was induced by stimulation with platelet-derived growth factor-BB (PDGF-BB) were treated with aloperine and exhibited decreased proliferation and increased apoptosis. These effects could be explained by the decreased levels of NF-kB, TNF-α, and cyclin E1 and the increased level of p27kip1 (Chang et al., 2019).
Finally, an interesting study, which requires further validation, showed that reoxygenation results in increased apoptosis in PASMCs, thus reversing hypobaric hypoxia-induced pulmonary artery remodeling, which could be attributed to mitochondrial ROS-mediated mitochondrial dysfunction (Chen et al., 2017). A proposed scheme of the pharmacological approaches is depicted in Figure 3.
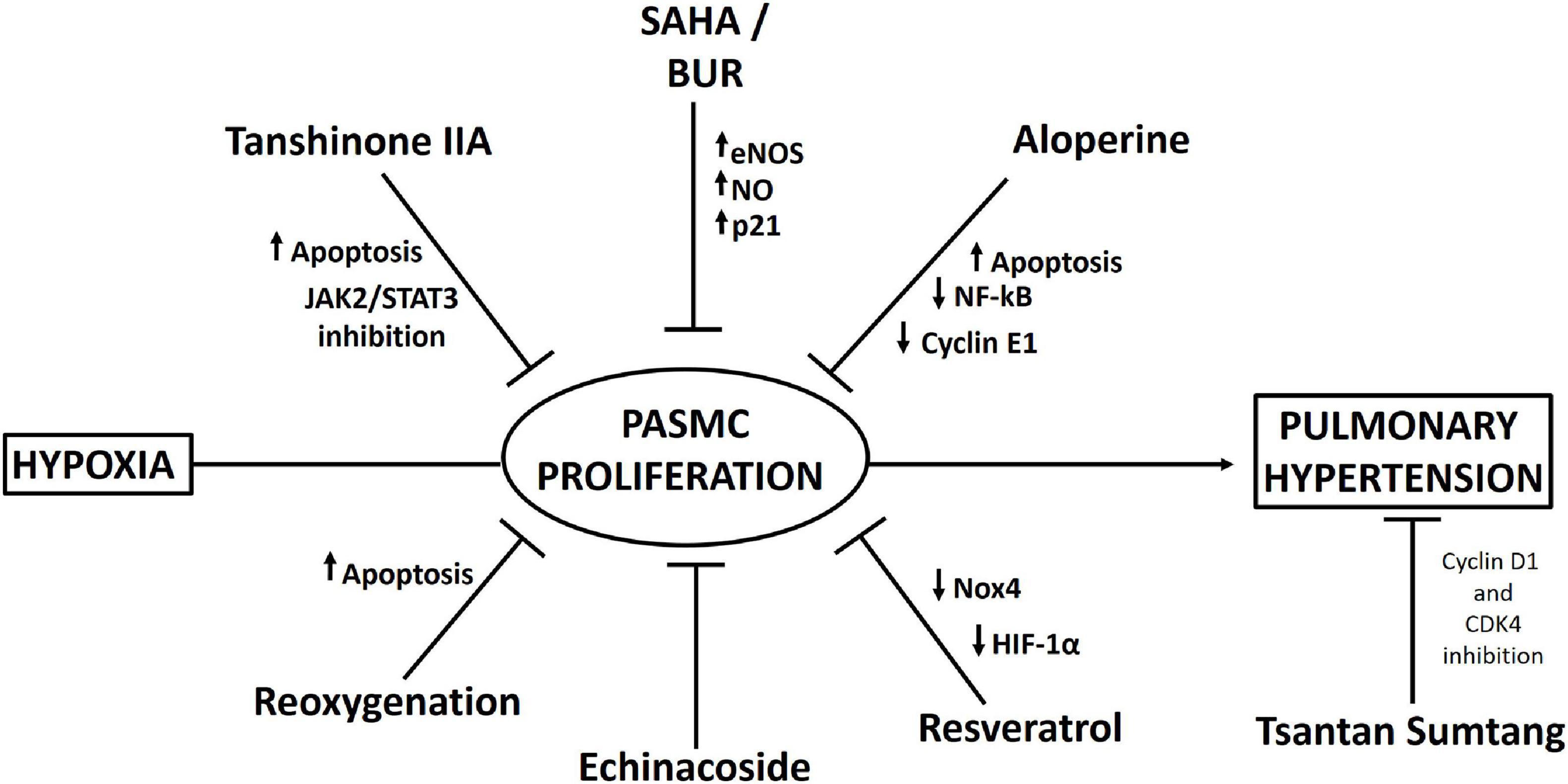
Figure 3. Scheme summarizing pharmacological interventions that have been described to decrease pulmonary artery smooth muscle cell (PASMC) proliferation and pulmonary hypertension under hypobaric hypoxia.
Conclusion
Hypoxia exposure is an important inducer of PASMC proliferation and subsequent remodeling and pulmonary hypertension. Moreover, evidence suggests that hypobaric hypoxia exposure activates several molecular pathways related to oxidative stress (the Nox4 pathway), protein kinases (ERK1/2), and inflammation (IL-6 and inflammatory cell infiltration). These pathways independently or cooperatively lead to further proliferation of PASMCs, contributing to subsequent pulmonary hypertension. In addition, based on the evidence discussed, a summarized array of some different pharmacological approaches has been developed to mitigate this proliferative effect. However, further studies are necessary to validate these treatments in people with PASMCs proliferation and subsequent HAPH induce by hypobaric hypoxia.
Author Contributions
PS, EP, JB, and SEA contributed to conceiving the review, reviewing the literature, writing and preparing the draft, and reviewing and editing the manuscript. All authors have read and agreed with the submitted version of this manuscript.
Funding
This review was funded by FIC GORE-TARAPACA and BIP 3047 7541-0.
Conflict of Interest
The authors declare that the research was conducted in the absence of any commercial or financial relationships that could be construed as a potential conflict of interest.
Publisher’s Note
All claims expressed in this article are solely those of the authors and do not necessarily represent those of their affiliated organizations, or those of the publisher, the editors and the reviewers. Any product that may be evaluated in this article, or claim that may be made by its manufacturer, is not guaranteed or endorsed by the publisher.
Abbreviations
HAPH, high-altitude pulmonary hypertension; PASMCs, pulmonary artery smooth muscle cells; PaO2, partial pressure of oxygen; ACE, acute cerebral edema; HAPE, high-altitude pulmonary edema; mPAP, mean pulmonary arterial pressure; SMCs, smooth muscle cells; ET-1, endothelin-1; NO, nitric oxide; HIF-1, hypoxia inducible factor-1; NFATc3, nuclear factor of activated T cells isoform c3; ROS, reactive oxygen species; NADPH, nicotinamide adenine dinucleotide phosphate; TGF-β, transforming growth factor-β; eNOS, endothelial nitric oxide synthase; O2.–, superoxide; .OH, hydroxyl radical; H2O2, hydrogen peroxide; CAT, catalase; GSH-PX/RX, glutathione peroxidase/reductase; HDIs, histone deacetylase inhibitors; FOX, forkhead box; FOXM1, forkhead box M1; FOXO1, forkhead box O1; PLK1, polo-like kinase 1; FOXC1, forkhead box C1; Erg-1, early growth response-1; SOD, superoxide dismutase; MPTP, mitochondrial permeability transition pore; MAPK, mitogen-activated protein kinase; ASK1, apoptosis signal-regulating kinase 1; PPARs, peroxisome proliferator-activated receptors; YAP, yes-associated protein; NF-κB, nuclear factor κB; JAK, Janus kinase; STAT, signal transducer and activator of transcription; TRPM7, transient receptor potential melastatin 7; PKC, protein kinase C; IL-6, interleukin-6; IL6R, membrane-bound interleukin-6 receptor; sIL-6R, soluble interleukin-6 receptor; MKL1, megakaryocytic leukemia 1; TNF-α, tumor necrosis factor alpha; SAHA, suberoylanilide hydroxamic acid; BUR, butyrate; JNK, c-Jun N-terminal kinase.
References
Adesina, S. E., Kang, B. Y., Bijli, K. M., Ma, J., Cheng, J., Murphy, T. C., et al. (2015). Targeting mitochondrial reactive oxygen species to modulate hypoxia-induced pulmonary hypertension. Free Radic. Biol. Med. 87, 36–47. doi: 10.1016/j.freeradbiomed.2015.05.042
Barman, S. A., Chen, F., Su, Y., Dimitropoulou, C., Wang, Y., Catravas, J. D., et al. (2014). NADPH oxidase 4 is expressed in pulmonary artery adventitia and contributes to hypertensive vascular remodeling. Arterioscler. Thromb. Vasc. Biol. 34, 1704–1715. doi: 10.1161/atvbaha.114.303848
Bierer, R., Nitta, C. H., Friedman, J., Codianni, S., de Frutos, S., Dominguez-Bautista, J. A., et al. (2011). NFATc3 is required for chronic hypoxia-induced pulmonary hypertension in adult and neonatal mice. Am. J. Physiol. Lung Cell. Mol. Physiol. 301, L872–L880. doi: 10.1152/ajplung.00405.2010
Bilo, G., Caravita, S., Torlasco, C., and Parati, G. (2019). Blood pressure at high altitude: physiology and clinical implications. Kardiol. Pol. 77, 596–603. doi: 10.33963/kp.14832
Bloom, B. R., and Bennett, B. (1966). Mechanism of a reaction in vitro associated with delayed-type hypersensitivity. Science 153, 80–82. doi: 10.1126/science.153.3731.80
Bowers, R., Cool, C., Murphy, R. C., Tuder, R. M., Hopken, M. W., Flores, S. C., et al. (2004). Oxidative stress in severe pulmonary hypertension. Am. J. Respir. Crit. Care Med. 169, 764–769. doi: 10.1164/rccm.200301-147OC
Brito, J., Siques, P., and Pena, E. (2020). Long-term chronic intermittent hypoxia: a particular form of chronic high-altitude pulmonary hypertension. Pulm. Circ. 10, 5–12. doi: 10.1177/2045894020934625
Brito, J., Siques, P., Arribas, S. M., López de Pablo, A. L., González, M. C., Naveas, N., et al. (2015). Adventitial alterations are the main features in pulmonary artery remodeling due to long-term chronic intermittent hypobaric hypoxia in rats. Biomed. Res. Int. 2015:169841. doi: 10.1155/2015/169841
Calandra, T., Bernhagen, J., Mitchell, R. A., and Bucala, R. (1994). The macrophage is an important and previously unrecognized source of macrophage migration inhibitory factor. J. Exp. Med. 179, 1895–1902. doi: 10.1084/jem.179.6.1895
Campbell, I. L., Erta, M., Lim, S. L., Frausto, R., May, U., Rose-John, S., et al. (2014). Trans-signaling is a dominant mechanism for the pathogenic actions of interleukin-6 in the brain. J. Neurosci. 34, 2503–2513. doi: 10.1523/jneurosci.2830-13.2014
Chang, Z., Zhang, P., Zhang, M., Jun, F., Hu, Z., Yang, J., et al. (2019). Aloperine suppresses human pulmonary vascular smooth muscle cell proliferation via inhibiting inflammatory response. Chin. J. Physiol. 62, 157–165. doi: 10.4103/cjp.cjp_27_19
Chavala, M. L. A. (2018). A journey between high altitude hypoxia and critical patient hypoxia: what can it teach us about compression and the management of critical disease? Med. Intensiva 42, 380–390. doi: 10.1016/j.medin.2017.08.006
Chen, J., Wang, Y. X., Dong, M. Q., Zhang, B., Luo, Y., Niu, W., et al. (2017). Reoxygenation reverses hypoxic pulmonary arterial remodeling by inducing smooth muscle cell apoptosis via reactive oxygen species-mediated mitochondrial dysfunction. J. Am. Heart Assoc. 6:e005602. doi: 10.1161/jaha.117.005602
Chen, M., Liu, Y., Yi, D., Wei, L., Li, Y., and Zhang, L. (2014). Tanshinone IIA promotes pulmonary artery smooth muscle cell apoptosis in vitro by inhibiting the JAK2/STAT3 signaling pathway. Cell. Physiol. Biochem. 33, 1130–1138. doi: 10.1159/000358682
Chen, Z., Sakuma, M., Zago, A. C., Zhang, X., Shi, C., Leng, L., et al. (2004). Evidence for a role of macrophage migration inhibitory factor in vascular disease. Arterioscler. Thromb. Vasc. Biol. 24, 709–714. doi: 10.1161/01.ATV.0000119356.35748.9e
Crouch, E. C., Parks, W. C., Rosenbaum, J. L., Chang, D., Whitehouse, L., Wu, L. J., et al. (1989). Regulation of collagen production by medial smooth muscle cells in hypoxic pulmonary hypertension. Am. Rev. Respir. Dis. 140, 1045–1051. doi: 10.1164/ajrccm/140.4.1045
Dai, J., Zhou, Q., Tang, H., Chen, T., Li, J., Raychaudhuri, P., et al. (2018). Smooth muscle cell-specific FoxM1 controls hypoxia-induced pulmonary hypertension. Cell. Signal. 51, 119–129. doi: 10.1016/j.cellsig.2018.08.003
Daiber, A., Di Lisa, F., Oelze, M., Kröller-Schön, S., Steven, S., Schulz, E., et al. (2017). Crosstalk of mitochondria with NADPH oxidase via reactive oxygen and nitrogen species signalling and its role for vascular function. Br. J. Pharmacol. 174, 1670–1689. doi: 10.1111/bph.13403
De Frutos, S., Diaz, J. M., Nitta, C. H., Sherpa, M. L., and Bosc, L. V. (2011). Endothelin-1 contributes to increased NFATc3 activation by chronic hypoxia in pulmonary arteries. Am. J. Physiol. Cell Physiol. 301, C441–C450. doi: 10.1152/ajpcell.00029.2011
De Frutos, S., Spangler, R., Alò, D., and Bosc, L. V. (2007). NFATc3 mediates chronic hypoxia-induced pulmonary arterial remodeling with alpha-actin up-regulation. J. Biol. Chem. 282, 15081–15089. doi: 10.1074/jbc.M702679200
Demarco, V. G., Whaley-Connell, A. T., Sowers, J. R., Habibi, J., and Dellsperger, K. C. (2010). Contribution of oxidative stress to pulmonary arterial hypertension. World J. Cardiol. 2, 316–324. doi: 10.4330/wjc.v2.i10.316
Dempsey, E. C., Das, M., Frid, M. G., and Stenmark, K. R. (1996). Unique growth properties of neonatal pulmonary vascular cells: importance of time- and site-specific responses, cell-cell interaction, and synergy. J. Perinatol. 16, S2–S11.
Dieffenbach, P. B., Haeger, C. M., Coronata, A. M. F., Choi, K. M., Varelas, X., Tschumperlin, D. J., et al. (2017). Arterial stiffness induces remodeling phenotypes in pulmonary artery smooth muscle cells via YAP/TAZ-mediated repression of cyclooxygenase-2. Am. J. Physiol. Lung Cell. Mol. Physiol. 313, L628–L647. doi: 10.1152/ajplung.00173.2017
Farías, J. G., Herrera, E. A., Carrasco-Pozo, C., Sotomayor-Zárate, R., Cruz, G., Morales, P., et al. (2016). Pharmacological models and approaches for pathophysiological conditions associated with hypoxia and oxidative stress. Pharmacol. Ther. 158, 1–23. doi: 10.1016/j.pharmthera.2015.11.006
Farías, J. G., Molina, V. M., Carrasco, R. A., Zepeda, A. B., Figueroa, E., Letelier, P., et al. (2017). Antioxidant therapeutic strategies for cardiovascular conditions associated with oxidative stress. Nutrients 9:966. doi: 10.3390/nu9090966
Florentin, J., Coppin, E., Vasamsetti, S. B., Zhao, J., Tai, Y. Y., Tang, Y., et al. (2018). Inflammatory macrophage expansion in pulmonary hypertension depends upon mobilization of blood-borne monocytes. J. Immunol. 200, 3612–3625. doi: 10.4049/jimmunol.1701287
Fukai, T., and Ushio-Fukai, M. (2020). Cross-talk between NADPH oxidase and mitochondria: role in ROS signaling and angiogenesis. Cells 9:1849. doi: 10.3390/cells9081849
Gai, X. Y., Tang, F., Ma, J., Zeng, K. W., Wang, S. L., Wang, Y. P., et al. (2014). Antiproliferative effect of echinacoside on rat pulmonary artery smooth muscle cells under hypoxia. J. Pharmacol. Sci. 126, 155–163. doi: 10.1254/jphs.14072fp
Gai, X., Lin, P., He, Y., Lu, D., Li, Z., Liang, Y., et al. (2020). Echinacoside prevents hypoxic pulmonary hypertension by regulating the pulmonary artery function. J. Pharmacol. Sci. 144, 237–244. doi: 10.1016/j.jphs.2020.09.002
Galiè, N., Humbert, M., Vachiery, J. L., Gibbs, S., Lang, I., Torbicki, A., et al. (2016). 2015 ESC/ERS guidelines for the diagnosis and treatment of pulmonary hypertension: the joint task force for the diagnosis and treatment of pulmonary hypertension of the European Society of Cardiology (ESC) and the European Respiratory Society (ERS): endorsed by: Association for European Paediatric and Congenital Cardiology (AEPC), International Society for Heart and Lung Transplantation (ISHLT). Eur. Heart J. 37, 67–119. doi: 10.1093/eurheartj/ehv317
Green, D. E., Murphy, T. C., Kang, B. Y., Kleinhenz, J. M., Szyndralewiez, C., Page, P., et al. (2012). The Nox4 inhibitor GKT137831 attenuates hypoxia-induced pulmonary vascular cell proliferation. Am. J. Respir. Cell Mol. Biol. 47, 718–726. doi: 10.1165/rcmb.2011-0418OC
Green, D. E., Sutliff, R. L., and Hart, C. M. (2011). Is peroxisome proliferator-activated receptor gamma (PPARγ) a therapeutic target for the treatment of pulmonary hypertension? Pulm. Circ. 1, 33–47. doi: 10.4103/2045-8932.78101
Guzy, R. D., and Schumacker, P. T. (2006). Oxygen sensing by mitochondria at complex III: the paradox of increased reactive oxygen species during hypoxia. Exp. Physiol. 91, 807–819. doi: 10.1113/expphysiol.2006.033506
Hartney, T., Birari, R., Venkataraman, S., Villegas, L., Martinez, M., Black, S. M., et al. (2011). Xanthine oxidase-derived ROS upregulate Egr-1 via ERK1/2 in PA smooth muscle cells; model to test impact of extracellular ROS in chronic hypoxia. PLoS One 6:e27531. doi: 10.1371/journal.pone.0027531
He, L. N., Lan, Y. R., He, G. M., Guo, S. J., Wen, F. Q., and Wang, T. (2020). [Resveratrol inhibits hypoxia-induced oxidative stress and proliferation in pulmonary artery smooth muscle cells through the HIF-1α/NOX4/ROS signaling pathway]. Sheng Li Xue Bao 72, 551–558.
He, Q., Nan, X., Li, S., Su, S., Ma, K., Li, Z., et al. (2018). Tsantan sumtang alleviates chronic hypoxia-induced pulmonary hypertension by inhibiting proliferation of pulmonary vascular cells. Biomed. Res. Int. 2018:9504158. doi: 10.1155/2018/9504158
Herrera, E. A., Farías, J. G., Ebensperger, G., Reyes, R. V., Llanos, A. J., and Castillo, R. L. (2015a). Pharmacological approaches in either intermittent or permanent hypoxia: a tale of two exposures. Pharmacol. Res. 101, 94–101. doi: 10.1016/j.phrs.2015.07.011
Herrera, E. A., Farías, J. G., González-Candia, A., Short, S. E., Carrasco-Pozo, C., and Castillo, R. L. (2015b). Ω3 Supplementation and intermittent hypobaric hypoxia induce cardioprotection enhancing antioxidant mechanisms in adult rats. Mar. Drugs 13, 838–860. doi: 10.3390/md13020838
Horita, H., Furgeson, S. B., Ostriker, A., Olszewski, K. A., Sullivan, T., Villegas, L. R., et al. (2013). Selective inactivation of PTEN in smooth muscle cells synergizes with hypoxia to induce severe pulmonary hypertension. J. Am. Heart Assoc. 2:e000188. doi: 10.1161/jaha.113.000188
Huang, J., Frid, M., Gewitz, M. H., Fallon, J. T., Brown, D., Krafsur, G., et al. (2019). Hypoxia-induced pulmonary hypertension and chronic lung disease: caveolin-1 dysfunction an important underlying feature. Pulm. Circ. 9:2045894019837876. doi: 10.1177/2045894019837876
Hunter, C. A., and Jones, S. A. (2015). IL-6 as a keystone cytokine in health and disease. Nat. Immunol. 16, 448–457. doi: 10.1038/ni.3153
Ismail, S., Sturrock, A., Wu, P., Cahill, B., Norman, K., Huecksteadt, T., et al. (2009). NOX4 mediates hypoxia-induced proliferation of human pulmonary artery smooth muscle cells: the role of autocrine production of transforming growth factor-{beta}1 and insulin-like growth factor binding protein-3. Am. J. Physiol. Lung Cell. Mol. Physiol. 296, L489–L499. doi: 10.1152/ajplung.90488.2008
Jin, Y., Wang, W., Chai, S., Liu, J., Yang, T., and Wang, J. (2015). Wnt5a attenuates hypoxia-induced pulmonary arteriolar remodeling and right ventricular hypertrophy in mice. Exp. Biol. Med. 240, 1742–1751. doi: 10.1177/1535370215584889
Klemm, D. J., Majka, S. M., Crossno, J. T. Jr., Psilas, J. C., Reusch, J. E., and Garat, C. V. (2011). Reduction of reactive oxygen species prevents hypoxia-induced CREB depletion in pulmonary artery smooth muscle cells. J. Cardiovasc. Pharmacol. 58, 181–191. doi: 10.1097/FJC.0b013e31821f2773
Kojonazarov, B., Isakova, J., Imanov, B., Sovkhozova, N., Sooronbaev, T., Ishizaki, T., et al. (2012). Bosentan reduces pulmonary artery pressure in high altitude residents. High Alt. Med. Biol. 13, 217–223. doi: 10.1089/ham.2011.1107
Kosacka, M., and Brzecka, A. (2021). Endothelin-1 and LOX-1 as markers of endothelial dysfunction in obstructive sleep apnea patients. Int. J. Environ. Res. Public Health 18:1319. doi: 10.3390/ijerph18031319
Lam, E. W., Brosens, J. J., Gomes, A. R., and Koo, C. Y. (2013). Forkhead box proteins: tuning forks for transcriptional harmony. Nat. Rev. Cancer 13, 482–495. doi: 10.1038/nrc3539
Lannér, M. C., Raper, M., Pratt, W. M., and Rhoades, R. A. (2005). Heterotrimeric G proteins and the platelet-derived growth factor receptor-beta contribute to hypoxic proliferation of smooth muscle cells. Am. J. Respir. Cell Mol. Biol. 33, 412–419. doi: 10.1165/rcmb.2005-0004OC
Lee, J. W., Chun, W., Lee, H. J., Min, J. H., Kim, S. M., Seo, J. Y., et al. (2021). The role of macrophages in the development of acute and chronic inflammatory lung diseases. Cells 10:897. doi: 10.3390/cells10040897
Lefebvre, P., Chinetti, G., Fruchart, J. C., and Staels, B. (2006). Sorting out the roles of PPAR alpha in energy metabolism and vascular homeostasis. J. Clin. Invest. 116, 571–580. doi: 10.1172/jci27989
León-Velarde, F., Maggiorini, M., Reeves, J. T., Aldashev, A., Asmus, I., Bernardi, L., et al. (2005). Consensus statement on chronic and subacute high altitude diseases. High Alt. Med. Biol. 6, 147–157. doi: 10.1089/ham.2005.6.147
León-Velarde, F., Villafuerte, F. C., and Richalet, J. P. (2010). Chronic mountain sickness and the heart. Prog. Cardiovasc. Dis. 52, 540–549. doi: 10.1016/j.pcad.2010.02.012
Li, R., Jia, Z., and Trush, M. A. (2016). Defining ROS in biology and medicine. React. Oxyg. Species 1, 9–21. doi: 10.20455/ros.2016.803
Liu, J. Q., Erbynn, E. M., and Folz, R. J. (2005). Chronic hypoxia-enhanced murine pulmonary vasoconstriction: role of superoxide and gp91phox. Chest 128(6 Suppl.), 594S–596S. doi: 10.1378/chest.128.6_suppl.594S
Lu, X., Bijli, K. M., Ramirez, A., Murphy, T. C., Kleinhenz, J., and Hart, C. M. (2013). Hypoxia downregulates PPARγ via an ERK1/2-NF-κB-Nox4-dependent mechanism in human pulmonary artery smooth muscle cells. Free Radic. Biol. Med. 63, 151–160. doi: 10.1016/j.freeradbiomed.2013.05.013
Lüneburg, N., Siques, P., Brito, J., Arriaza, K., Pena, E., Klose, H., et al. (2016). Long-term chronic intermittent hypobaric hypoxia in rats causes an imbalance in the asymmetric dimethylarginine/nitric oxide pathway and ROS activity: a possible synergistic mechanism for altitude pulmonary hypertension? Pulm. Med. 2016:6578578. doi: 10.1155/2016/6578578
Martin-Garrido, A., Brown, D. I., Lyle, A. N., Dikalova, A., Seidel-Rogol, B., Lassègue, B., et al. (2011). NADPH oxidase 4 mediates TGF-β-induced smooth muscle α-actin via p38MAPK and serum response factor. Free Radic. Biol. Med. 50, 354–362. doi: 10.1016/j.freeradbiomed.2010.11.007
Maston, L. D., Jones, D. T., Giermakowska, W., Resta, T. C., Ramiro-Diaz, J., Howard, T. A., et al. (2018). Interleukin-6 trans-signaling contributes to chronic hypoxia-induced pulmonary hypertension. Pulm. Circ. 8:2045894018780734. doi: 10.1177/2045894018780734
Meyrick, B., and Reid, L. (1979). Hypoxia and incorporation of 3H-thymidine by cells of the rat pulmonary arteries and alveolar wall. Am. J. Pathol. 96, 51–70.
Mikhael, M., Makar, C., Wissa, A., Le, T., Eghbali, M., and Umar, S. (2019). Oxidative stress and its implications in the right ventricular remodeling secondary to pulmonary hypertension. Front. Physiol. 10:1233. doi: 10.3389/fphys.2019.01233
Miller, R. C., Pelton, J. T., and Huggins, J. P. (1993). Endothelins–from receptors to medicine. Trends Pharmacol. Sci. 14, 54–60. doi: 10.1016/0165-6147(93)90031-e
Mittal, M., Roth, M., König, P., Hofmann, S., Dony, E., Goyal, P., et al. (2007). Hypoxia-dependent regulation of nonphagocytic NADPH oxidase subunit NOX4 in the pulmonary vasculature. Circ. Res. 101, 258–267. doi: 10.1161/circresaha.107.148015
Moore, L. G., Niermeyer, S., and Zamudio, S. (1998). Human adaptation to high altitude: regional and life-cycle perspectives. Am. J. Phys. Anthropol. 107(Suppl. 27), 25–64.
Moudgil, R., Michelakis, E. D., and Archer, S. L. (2005). Hypoxic pulmonary vasoconstriction. J. Appl. Physiol. 98, 390–403. doi: 10.1152/japplphysiol.00733.2004
Nakanishi, K., Tajima, F., Nakata, Y., Osada, H., Tachibana, S., Kawai, T., et al. (1999). Expression of endothelin-1 in rats developing hypobaric hypoxia-induced pulmonary hypertension. Lab. Invest. 79, 1347–1357.
Neupane, M., and Swenson, E. R. (2017). High-altitude pulmonary vascular diseases. Adv. Pulm. Hyperten. 15, 149–157. doi: 10.21693/1933-088X-15.3.149
Nisbet, R. E., Bland, J. M., Kleinhenz, D. J., Mitchell, P. O., Walp, E. R., Sutliff, R. L., et al. (2010). Rosiglitazone attenuates chronic hypoxia-induced pulmonary hypertension in a mouse model. Am. J. Respir. Cell Mol. Biol. 42, 482–490. doi: 10.1165/rcmb.2008-0132OC
Norton, C. E., Broughton, B. R., Jernigan, N. L., Walker, B. R., and Resta, T. C. (2013). Enhanced depolarization-induced pulmonary vasoconstriction following chronic hypoxia requires EGFR-dependent activation of NAD(P)H oxidase 2. Antioxid. Redox Signal. 18, 1777–1788. doi: 10.1089/ars.2012.4836
Onodera, S., Nishihira, J., Koyama, Y., Majima, T., Aoki, Y., Ichiyama, H., et al. (2004). Macrophage migration inhibitory factor up-regulates the expression of interleukin-8 messenger RNA in synovial fibroblasts of rheumatoid arthritis patients: common transcriptional regulatory mechanism between interleukin-8 and interleukin-1beta. Arthritis Rheum. 50, 1437–1447. doi: 10.1002/art.20190
O’Shea, J. J., and Plenge, R. (2012). JAK and STAT signaling molecules in immunoregulation and immune-mediated disease. Immunity 36, 542–550. doi: 10.1016/j.immuni.2012.03.014
Pak, O., Aldashev, A., Welsh, D., and Peacock, A. (2007). The effects of hypoxia on the cells of the pulmonary vasculature. Eur. Respir. J. 30, 364–372. doi: 10.1183/09031936.00128706
Penaloza, D., and Arias-Stella, J. (2007). The heart and pulmonary circulation at high altitudes: healthy highlanders and chronic mountain sickness. Circulation 115, 1132–1146. doi: 10.1161/circulationaha.106.624544
Penaloza, D., Arias-Stella, J., Sime, F., Recavarren, S., and Marticorena, E. (1964). The heart and pulmonary circulation in children at high altitudes: physiological, anatomical, and clinical observations. Pediatrics 34, 568–582.
Peoples, J. N., Saraf, A., Ghazal, N., Pham, T. T., and Kwong, J. Q. (2019). Mitochondrial dysfunction and oxidative stress in heart disease. Exp. Mol. Med. 51, 1–13. doi: 10.1038/s12276-019-0355-7
Perez-Vizcaino, F., Cogolludo, A., and Moreno, L. (2010). Reactive oxygen species signaling in pulmonary vascular smooth muscle. Respir. Physiol. Neurobiol. 174, 212–220. doi: 10.1016/j.resp.2010.08.009
Preston, I. R., Hill, N. S., Warburton, R. R., and Fanburg, B. L. (2006). Role of 12-lipoxygenase in hypoxia-induced rat pulmonary artery smooth muscle cell proliferation. Am. J. Physiol. Lung Cell. Mol. Physiol. 290, L367–L374. doi: 10.1152/ajplung.00114.2005
Raghavan, A., Zhou, G., Zhou, Q., Ibe, J. C., Ramchandran, R., Yang, Q., et al. (2012). Hypoxia-induced pulmonary arterial smooth muscle cell proliferation is controlled by forkhead box M1. Am. J. Respir. Cell Mol. Biol. 46, 431–436. doi: 10.1165/rcmb.2011-0128OC
Raoof, I., and Daoud, A. (2019). Hypoxia-inducible factors-α as a regulator for forkhead box protein M1 in pulmonary artery hypertension. Mustansiriya Med. J. 18, 59–62. doi: 10.4103/mj.mj_12_19
Richardson, A. G., and Schadt, E. E. (2014). The role of macromolecular damage in aging and age-related disease. J. Gerontol. A Biol. Sci. Med. Sci. 69(Suppl. 1), S28–S32. doi: 10.1093/gerona/glu056
Robinson, J. C., Abbott, C., Meadows, C. A., Roach, R. C., Honigman, B., and Bull, T. M. (2017). Long-term health outcomes in high-altitude pulmonary hypertension. High Alt. Med. Biol. 18, 61–66. doi: 10.1089/ham.2016.0098
Savai, R., Al-Tamari, H. M., Sedding, D., Kojonazarov, B., Muecke, C., Teske, R., et al. (2014). Pro-proliferative and inflammatory signaling converge on FoxO1 transcription factor in pulmonary hypertension. Nat. Med. 20, 1289–1300. doi: 10.1038/nm.3695
Savale, L., Tu, L., Rideau, D., Izziki, M., Maitre, B., Adnot, S., et al. (2009). Impact of interleukin-6 on hypoxia-induced pulmonary hypertension and lung inflammation in mice. Respir. Res. 10:6. doi: 10.1186/1465-9921-10-6
Scheller, J., Chalaris, A., Schmidt-Arras, D., and Rose-John, S. (2011). The pro- and anti-inflammatory properties of the cytokine interleukin-6. Biochim. Biophys. Acta 1813, 878–888. doi: 10.1016/j.bbamcr.2011.01.034
Sengupta, A., Kalinichenko, V. V., and Yutzey, K. E. (2013). FoxO1 and FoxM1 transcription factors have antagonistic functions in neonatal cardiomyocyte cell-cycle withdrawal and IGF1 gene regulation. Circ. Res. 112, 267–277. doi: 10.1161/circresaha.112.277442
Shi, W., Wang, Q., Wang, J., Yan, X., Feng, W., Zhang, Q., et al. (2021). Activation of yes-associated protein mediates sphingosine-1-phosphate-induced proliferation and migration of pulmonary artery smooth muscle cells and its potential mechanisms. J. Cell. Physiol. 236, 4694–4708. doi: 10.1002/jcp.30193
Simonneau, G., Gatzoulis, M. A., Adatia, I., Celermajer, D., Denton, C., Ghofrani, A., et al. (2013). Updated clinical classification of pulmonary hypertension. J. Am. Coll. Cardiol. 62(25 Suppl.), D34–D41. doi: 10.1016/j.jacc.2013.10.029
Siques, P., Brito, J., and Pena, E. (2018). Reactive oxygen species and pulmonary vasculature during hypobaric hypoxia. Front. Physiol. 9:865. doi: 10.3389/fphys.2018.00865
Siques, P., López de Pablo, A. L., Brito, J., Arribas, S. M., Flores, K., Arriaza, K., et al. (2014). Nitric oxide and superoxide anion balance in rats exposed to chronic and long term intermittent hypoxia. Biomed. Res. Int. 2014:610474. doi: 10.1155/2014/610474
Snow, J. B., Norton, C. E., Sands, M. A., Weise-Cross, L., Yan, S., Herbert, L. M., et al. (2020). Intermittent hypoxia augments pulmonary vasoconstrictor reactivity through PKCβ/mitochondrial oxidant signaling. Am. J. Respir. Cell Mol. Biol. 62, 732–746. doi: 10.1165/rcmb.2019-0351OC
Sommer, N., Dietrich, A., Schermuly, R. T., Ghofrani, H. A., Gudermann, T., Schulz, R., et al. (2008). Regulation of hypoxic pulmonary vasoconstriction: basic mechanisms. Eur. Respir. J. 32, 1639–1651. doi: 10.1183/09031936.00013908
Son, Y., Kim, S., Chung, H. T., and Pae, H. O. (2013). Reactive oxygen species in the activation of MAP kinases. Methods Enzymol. 528, 27–48. doi: 10.1016/b978-0-12-405881-1.00002-1
Sturrock, A., Cahill, B., Norman, K., Huecksteadt, T. P., Hill, K., Sanders, K., et al. (2006). Transforming growth factor-beta1 induces Nox4 NAD(P)H oxidase and reactive oxygen species-dependent proliferation in human pulmonary artery smooth muscle cells. Am. J. Physiol. Lung Cell. Mol. Physiol. 290, L661–L673. doi: 10.1152/ajplung.00269.2005
Sysol, J. R., Chen, J., Singla, S., Zhao, S., Comhair, S., Natarajan, V., et al. (2018). Micro-RNA-1 is decreased by hypoxia and contributes to the development of pulmonary vascular remodeling via regulation of sphingosine kinase 1. Am. J. Physiol. Lung Cell. Mol. Physiol. 314, L461–L472. doi: 10.1152/ajplung.00057.2017
Sysol, J. R., Natarajan, V., and Machado, R. F. (2016). PDGF induces SphK1 expression via Egr-1 to promote pulmonary artery smooth muscle cell proliferation. Am. J. Physiol. Cell. Physiol. 310, C983–C992. doi: 10.1152/ajpcell.00059.2016
Tamura, Y., Phan, C., Tu, L., Le Hiress, M., Thuillet, R., Jutant, E. M., et al. (2018). Ectopic upregulation of membrane-bound IL6R drives vascular remodeling in pulmonary arterial hypertension. J. Clin. Invest. 128, 1956–1970. doi: 10.1172/jci96462
Tan, X., Feng, L., Huang, X., Yang, Y., Yang, C., and Gao, Y. (2017). Histone deacetylase inhibitors promote eNOS expression in vascular smooth muscle cells and suppress hypoxia-induced cell growth. J. Cell. Mol. Med. 21, 2022–2035. doi: 10.1111/jcmm.13122
Thomas, S. J., Snowden, J. A., Zeidler, M. P., and Danson, S. J. (2015). The role of JAK/STAT signalling in the pathogenesis, prognosis and treatment of solid tumours. Br. J. Cancer 113, 365–371. doi: 10.1038/bjc.2015.233
Tuder, R. M. (2017). Pulmonary vascular remodeling in pulmonary hypertension. Cell Tissue Res. 367, 643–649. doi: 10.1007/s00441-016-2539-y
Udjus, C., Cero, F. T., Halvorsen, B., Behmen, D., Carlson, C. R., Bendiksen, B. A., et al. (2019). Caspase-1 induces smooth muscle cell growth in hypoxia-induced pulmonary hypertension. Am. J. Physiol. Lung Cell. Mol. Physiol. 316, L999–L1012. doi: 10.1152/ajplung.00322.2018
Vodenicharov, A. (2012). “Structure and function of smooth muscle with special reference to mast cells,” in Current Basic and Pathological Approaches to the Function of Muscle Cells and Tissues – From Molecules to Humans, ed. H. Sugi (London: InTech Open), 346–362.
Von Euler, U. S., and Liljestrand, G. (1946). Observations on the pulmonary arterial blood pressure in the cat. Acta Physiol. Scand. 12, 301–320. doi: 10.1111/j.1748-1716.1946.tb00389.x
Wang, G., Qian, G., Zhou, D., and Zhao, J. (2005). JAK-STAT signaling pathway in pulmonary arterial smooth muscle cells is activated by hypoxia. Cell Biol. Int. 29, 598–603. doi: 10.1016/j.cellbi.2005.03.014
Wedgwood, S., Dettman, R. W., and Black, S. M. (2001). ET-1 stimulates pulmonary arterial smooth muscle cell proliferation via induction of reactive oxygen species. Am. J. Physiol. Lung Cell. Mol. Physiol. 281, L1058–L1067. doi: 10.1152/ajplung.2001.281.5.L1058
West, J. B. (2012). High-altitude medicine. Am. J. Respir. Crit. Care Med. 186, 1229–1237. doi: 10.1164/rccm.201207-1323CI
Wilson, J. L., Wang, L., Zhang, Z., Hill, N. S., and Polgar, P. (2019). Participation of PLK1 and FOXM1 in the hyperplastic proliferation of pulmonary artery smooth muscle cells in pulmonary arterial hypertension. PLoS One 14:e0221728. doi: 10.1371/journal.pone.0221728
Wilson, K. S., Buist, H., Suveizdyte, K., Liles, J. T., Budas, G. R., Hughes, C., et al. (2020). Apoptosis signal-regulating kinase 1 inhibition in in vivo and in vitro models of pulmonary hypertension. Pulm. Circ. 10:2045894020922810. doi: 10.1177/2045894020922810
Wong, C. M., Bansal, G., Pavlickova, L., Marcocci, L., and Suzuki, Y. J. (2013). Reactive oxygen species and antioxidants in pulmonary hypertension. Antioxid. Redox Signal. 18, 1789–1796. doi: 10.1089/ars.2012.4568
Wu, W., Platoshyn, O., Firth, A. L., and Yuan, J. X. (2007). Hypoxia divergently regulates production of reactive oxygen species in human pulmonary and coronary artery smooth muscle cells. Am. J. Physiol. Lung Cell. Mol. Physiol. 293, L952–L959. doi: 10.1152/ajplung.00203.2007
Xia, L. M., Huang, W. J., Wang, B., Liu, M., Zhang, Q., Yan, W., et al. (2009). Transcriptional up-regulation of FoxM1 in response to hypoxia is mediated by HIF-1. J. Cell. Biochem. 106, 247–256. doi: 10.1002/jcb.21996
Xing, J., Wang, M., Hong, J., Gao, Y., Liu, Y., Gu, H., et al. (2019). TRPM7 channel inhibition exacerbates pulmonary arterial hypertension through MEK/ERK pathway. Aging 11, 4050–4065. doi: 10.18632/aging.102036
Xu, D., Li, Y., Zhang, B., Wang, Y., Liu, Y., Luo, Y., et al. (2016). Resveratrol alleviate hypoxic pulmonary hypertension via anti-inflammation and anti-oxidant pathways in rats. Int. J. Med. Sci. 13, 942–954. doi: 10.7150/ijms.16810
Xu, X. Q., and Jing, Z. C. (2009). High-altitude pulmonary hypertension. Eur. Respir. Rev. 18, 13–17. doi: 10.1183/09059180.00011104
Yamashita, K., Discher, D. J., Hu, J., Bishopric, N. H., and Webster, K. A. (2001). Molecular regulation of the endothelin-1 gene by hypoxia. Contributions of hypoxia-inducible factor-1, activator protein-1, GATA-2, AND p300/CBP. J. Biol. Chem. 276, 12645–12653. doi: 10.1074/jbc.M011344200
Yanagisawa, M., Kurihara, H., Kimura, S., Tomobe, Y., Kobayashi, M., Mitsui, Y., et al. (1988). A novel potent vasoconstrictor peptide produced by vascular endothelial cells. Nature 332, 411–415. doi: 10.1038/332411a0
Yang, L., Liang, H., Meng, X., Shen, L., Guan, Z., Hei, B., et al. (2020). mmu_circ_0000790 is involved in pulmonary vascular remodeling in mice with HPH via microRNA-374c-mediated FOXC1. Mol. Ther. Nucleic Acids 20, 292–307. doi: 10.1016/j.omtn.2019.12.027
Yu, X. M., Wang, L., Li, J. F., Liu, J., Li, J., Wang, W., et al. (2013). Wnt5a inhibits hypoxia-induced pulmonary arterial smooth muscle cell proliferation by downregulation of β-catenin. Am. J. Physiol. Lung Cell. Mol. Physiol. 304, L103–L111. doi: 10.1152/ajplung.00070.2012
Yu, Y. R., Mao, L., Piantadosi, C. A., and Gunn, M. D. (2013). CCR2 deficiency, dysregulation of Notch signaling, and spontaneous pulmonary arterial hypertension. Am. J. Respir. Cell Mol. Biol. 48, 647–654. doi: 10.1165/rcmb.2012-0182OC
Yuan, Z., Chen, J., Chen, D., Xu, G., Xia, M., Xu, Y., et al. (2014). Megakaryocytic leukemia 1 (MKL1) regulates hypoxia induced pulmonary hypertension in rats. PLoS One 9:e83895. doi: 10.1371/journal.pone.0083895
Zhang, B., Shen, M., Xu, M., Liu, L. L., Luo, Y., Xu, D. Q., et al. (2012). Role of macrophage migration inhibitory factor in the proliferation of smooth muscle cell in pulmonary hypertension. Mediators Inflamm. 2012:840737. doi: 10.1155/2012/840737
Zhang, L., Wang, Y., Wu, G., Rao, L., Wei, Y., Yue, H., et al. (2020). Blockade of JAK2 protects mice against hypoxia-induced pulmonary arterial hypertension by repressing pulmonary arterial smooth muscle cell proliferation. Cell Prolif. 53:e12742. doi: 10.1111/cpr.12742
Zhang, N., Dong, M., Luo, Y., Zhao, F., and Li, Y. (2018). Danshensu prevents hypoxic pulmonary hypertension in rats by inhibiting the proliferation of pulmonary artery smooth muscle cells via TGF-β-smad3-associated pathway. Eur. J. Pharmacol. 820, 1–7. doi: 10.1016/j.ejphar.2017.12.010
Zhang, Q., Li, W., Zhu, Y., Wang, Q., Zhai, C., Shi, W., et al. (2021). Activation of AMPK inhibits Galectin-3-induced pulmonary artery smooth muscle cells proliferation by upregulating hippo signaling effector YAP. Mol. Cell. Biochem. 476, 3037–3049. doi: 10.1007/s11010-021-04131-3
Keywords: hypobaric hypoxia, pulmonary artery smooth muscle cell, oxidative stress, kinases, inflammation, high altitude
Citation: Siques P, Pena E, Brito J and El Alam S (2021) Oxidative Stress, Kinase Activation, and Inflammatory Pathways Involved in Effects on Smooth Muscle Cells During Pulmonary Artery Hypertension Under Hypobaric Hypoxia Exposure. Front. Physiol. 12:690341. doi: 10.3389/fphys.2021.690341
Received: 02 April 2021; Accepted: 16 July 2021;
Published: 09 August 2021.
Edited by:
Cristina M. Sena, University of Coimbra, PortugalReviewed by:
Bojun Zhang, Baylor College of Medicine, United StatesJi-Wang Chen, University of Illinois at Chicago, United States
Jorge G. Farias, University of La Frontera, Chile
Copyright © 2021 Siques, Pena, Brito and El Alam. This is an open-access article distributed under the terms of the Creative Commons Attribution License (CC BY). The use, distribution or reproduction in other forums is permitted, provided the original author(s) and the copyright owner(s) are credited and that the original publication in this journal is cited, in accordance with accepted academic practice. No use, distribution or reproduction is permitted which does not comply with these terms.
*Correspondence: Eduardo Pena, RWR1YXJkby5lbnJpcXVlLmJpb0BnbWFpbC5jb20=
†These authors share first authorship