- 1Department of Entomology, Institute of Plant Protection, Agricultural Research Organization, Bet Dagan, Israel
- 2Laboratory of Entomology and Agricultural Zoology, Department of Agriculture Crop Production and Rural Environment, University of Thessaly, Volos, Greece
Insects, similarly to other small terrestrial invertebrates, are particularly susceptible to climatic stress. Physiological adjustments to cope with the environment (i.e., acclimation) together with genetic makeup eventually determine the tolerance of a species to climatic extremes, and constrain its distribution. Temperature and desiccation resistance in insects are both conditioned by acclimation and may be interconnected, particularly for species inhabiting xeric environments. We determined the effect of temperature acclimation on desiccation resistance of the peach fruit fly (Bactrocera zonata, Tephritidae) – an invasive, polyphagous pest, currently spreading through both xeric and mesic environments in Africa and the Eurasian continent. Following acclimation at three constant temperatures (20, 25, and 30°C), the survival of adult flies deprived of food and water was monitored in extreme dry and humid conditions (<10 and >90% relative humidity, respectively). We found that flies acclimated at higher temperatures were significantly heavier, and contained more lipids and protein. Acclimation temperature significantly and similarly affected the survival of males and females at both high and low humidity conditions. In both cases, flies maintained at 30°C survived longer compared to 20 and 25°C – habituated counterparts. Regardless of the effect of acclimation temperature on survival, overall life expectancy was significantly shortened when flies were assayed under desiccating conditions. Additionally, our experiments indicate no significant difference in survival patterns between males and females, and that acclimation temperature had similar effects after both short (5–10 days) and long (11–20 days) acclimation periods. We conclude that acclimation at 30°C prolongs the survival of B. zonata, regardless of ambient humidity levels. Temperature probably affected survival through modulating feeding and metabolism, allowing for accumulation of larger energetic reserves, which in turn, promoted a greater ability to resist starvation, and possibly desiccation as well. Our study set the grounds for understanding the phenotypic plasticity of B. zonata from the hydric perspective, and for further evaluating the invasion potential of this pest.
Introduction
Dispersion of insects to new environments and consequent geographic range expansions implies an evolutionary process in which the organism’s inherent phenotypic plasticity is selected by new environmental conditions (Beaman et al., 2016; Tay and Gordon, 2019). Adaptations to the environment usually build up gradually during natural dispersions of insect species to adjacent habitats or previously unoccupied environments (e.g., Ouisse et al., 2020). In other cases though, the process may be abrupt, such as during anthropogenic introductions of insects through long-range trading of commodities, or migration of animals carrying phoretic alien insect species (e.g., Meurisse et al., 2019). In both circumstances, the intrinsic physiological and behavioral plasticity of the species will determine its ability to respond to change in its natural habitat, or establish in novel environments (Bubliy et al., 2012; Little et al., 2020).
Acclimation (i.e., the habituation of the organisms’ physiology, metabolism, and/or behavior to the prevailing environmental conditions) is a form of plasticity that may confer resistance to environmental stress (Little et al., 2020; Terblanche and Hoffmann, 2020). Acclimation has been extensively studied in the past to examine response of insects to temperature stress (reviewed by Sgro et al., 2016; Terblanche and Hoffmann, 2020). Such studies show that thermal habituation can increase insect tolerance to acute heat or cold stress (Sgro et al., 2016). Similarly, short term acclimation to a desiccating environment can contribute to the resistance of insects to dehydration (Chown et al., 2011). Hence, understanding the effects of acclimation is a major step toward predicting the contribution of phenotypic plasticity to the potential distribution and invasion dynamics of plants and animals (Beaman et al., 2016; Bujan et al., 2021). This may be particularly relevant to organisms having negative economic or ecological impact, such as disease vectors, agricultural pests, or aggressive invaders, which disrupt the ecological stability of colonized environments (Lockwood et al., 2007).
Due to their reduced size and high surface to volume ratio insects are particularly prone to desiccation stress by transpiration of water through the cuticle and spiracle openings. Additionally, water may be lost through excretions of solid waste and fluids (reviewed by Weldon et al., 2019). To regulate their water balance, insects employ various physiological adaptations that enhance their resistance to desiccation or increase intrinsic water stores. These include secretion of hydrocarbons that reduce cuticular permeability to water or regulation of spiracle opening to decrease water loss through gas exchange (Gibbs et al., 1997; Kalra et al., 2017; Weldon et al., 2019). Such mechanisms may synergistically contribute to overall resistance to dehydration, and constitute a part of a plastic response driven by prior acclimation to a desiccating environment (Chown et al., 2011). Additionally, insects may generate water internally through catabolism of lipid and carbohydrate reserves, and thus better tolerate desiccation stress (i.e., metabolic water, as in e.g., drosophillid flies; Gibbs et al., 1997; Weldon et al., 2019 and referenced therein). Consequently, similarly to tolerance to other types of climatic stress (e.g., cold tolerance, Mohammadzadeh and Izadi, 2018; Raza et al., 2020), obtaining sufficient nutritional reserves may be imperative for the ability to resist desiccation (e.g., Gefen et al., 2006).
Nevertheless, depending on environmental conditions, higher respiration rates associated with metabolism may come at the expense of tolerance to extreme humidity (Kleynhans and Terblanche, 2011). For instance, under high temperature and relative humidity (RH) conditions, metabolism and respiration rates are not expected to drastically affect water balance in insects (Contreras and Bradley, 2011). Contrarily, when RH is low, gas exchange and metabolic regulation may come at a cost to the organism’s water balance, reducing its tolerance to extreme dry environments (Terblanche et al., 2008).
Temperature and desiccation stress often coincide for insects inhabiting xeric environments and their regulation may involve mutual adaptations. Indeed, the physiological and behavioral mechanisms activated to regulate metabolic rate and oxygen consumption in response to temperature and humidity in ectothermic organism are, in many cases, strongly related (e.g., regulation of gas exchange and heat and water balances in insects, Boardman and Terblanche, 2015; Thorat and Nath, 2018). However, little information is available regarding the effects of thermal acclimation on the ability of insects to withstand draught conditions and avoid dehydration (Kellermann et al., 2018; Thorat and Nath, 2018; Terblanche and Hoffmann, 2020), and the available data is often incomplete. For example, desiccation resistance was demonstrated to associate with temperature acclimation in Drosophilid (Kalra et al., 2017) and Tse-Tse flies (Terblanche and Chown, 2006), and to be mediated through excess secretion of cuticular lipids (Kalra et al., 2017). Similarly, when exposed to a desiccating environment, heat acclimated butterflies gained from reduced loss of body mass, possibly resulting from increased retention of water (Fischer and Kirste, 2018). However, in other cases (e.g., Drosophila: Gibbs et al., 1998, Chirodica beetles: Terblanche et al., 2005), thermal acclimation did not contribute to increased desiccation tolerance (see Chown et al., 2011 for other examples). Accordingly, thermal acclimation may affect the plasticity and tolerance of insects to extreme environmental humidity conditions, but such effects seem to be subject to variation (Chown et al., 2011; Terblanche and Hoffmann, 2020).
The peach fruit fly [Bactrocera zonata (Saunders); Diptera: Tephritidae], an invasive polyphagous pest of commercially-grown fruits, is progressively expanding its distribution in North Africa, the Middle East, and the Arabian peninsula in the past decades. Native to tropical southeastern Asia, the fly has invaded and established stable populations in Egypt during the early 1990s and has since dispersed to Libya, Sudan, and the desert of the Sinai Peninsula (Zingore et al., 2020). It has been intercepted on several occasions at the border between Israel and Egypt (EPPO Bulletin, 2005, 2010), and is currently frequently detected in the urban and suburban areas of Tel-Aviv, Israel (Gazit and Akiva, 2017). While no information currently exists on the source of the current outbreak in Tel Aviv area, it is suspected that the fly originates from the Israel-Egypt border and was unintentionally introduced to this urban area by humans.
The persistent detection of this fly in hot and dry desert regions, tropical habitats, and urban environments, where moderate temperatures and RH prevail throughout most of the year (Portnov and Safriel, 2004), point to a high phenotypic plasticity supporting its survival in both xeric and mesic environments. The current geographic distribution and the versatile climatic environments occupied by this species provide an excellent foundation to explore its plastic and adaptive responses to humidity stress.
The current paper is a first approach to characterize the ability of B. zonata to overcome hydric stress and adapt to xeric and humid environments. Here, we address the possible link between temperature acclimation and tolerance to humidity, and examine if temperature could trigger enhanced resistance to humidity stress. Accordingly, we exposed flies to different thermal regimes and subsequently assayed them for survival at humid and dry environments. As metabolism is modulated by temperature, we additionally examined the effect of acclimation temperature on weight and nutritional reserves of the fly, and tested for possible correlations with survival.
Materials and Methods
Fly origin and maintenance: B. zonata pupae were obtained from a laboratory colony established in 2012 from flies collected in the area of Tel Aviv, Israel, and routinely maintained at the quarantine facility of the Plant Protection and Inspection Services Laboratories in Bet-Dagan, Israel (see Gazit and Akiva, 2017 for details). The colony’s breeding population was last refreshed with wild flies in 2019. Following emergence, 1–2-day-old adults were separated by sex into 30 cm × 30 cm × 30 cm cubic screen/Plexiglas cages supplied with a standard diet of sugar and hydrolyzed yeast (3:1 ratio by weight; Tsitsipis, 1989 and references therein) and water. Male and female cages were maintained at three constant acclimation temperatures (20, 25, and 30°C), ambient humidity (50 ± 10% RH), and photoperiod of 16:8, hours of light:dark, respectively. Adult survival in extreme dry and humid environments (see following section) was subsequently determined for the three thermal acclimation regimes following 5–10 and 11–20 days of acclimation (short and long acclimation period, respectively). The use of constant temperature regimes to study acclimation has been previously suggested as unrealistic ecologically (Terblanche et al., 2010; Terblanche and Hoffmann, 2020). Nevertheless, herein, we decided to use such a protocol in order to maximize the expression of habituation traits in our experiment.
Bioassays
Following acclimation, randomly sampled flies were weighed to the nearest 0.1 mg, individually confined to aerated 5 ml glass vials, and introduced into two transparent humidity chambers. The humidity in each chamber was preconditioned to 5 ± 3% RH (extreme dry) or 95 ± 3% RH (extreme humid) using dehydrated silica gel or a beaker with water placed inside the chamber, respectively. Assays continued uninterruptedly for 4 days at 25°C and 16:8 light:dark cycle, during which mortality was monitored visually every 2 h (during day time) or 10–12 h (during night time), without compromising the atmosphere within the chambers. Humidity levels in each chamber were constantly recorded using HOBO U12 data loggers (Onset, MA, United States), and verified to remain within the limit of the preset values. When possible three replicates were conducted for each combination of acclimation temperature, acclimation duration, and humidity regimes (n = 19 groups). However, due to shortage in flies, some combinations included two replicates (n = 5 groups; overall n = 24 groups). For each group, a replicate consisted of 8–10 individuals of each sex (overall n = 18–30 flies in each group, total n = 646 flies).
Chemical Analyses
We evaluated the effect of acclimation temperature on the accumulation of lipid and protein reserves in males and females by using the colorimetric phospho-vanillin and Bradford assays, (respectively) as previously described (Nestel et al., 2004). Assays were performed on individual, 18 day old flies (n = 7–15 flies per treatment) sampled from each acclimation group. Briefly, for lipid quantifications, flies were homogenized in 100 μl of Na2SO4 (2%) after which lipids were extracted by adding 1,400 μl of Chloroform:Methanol (1:1 by volume, respectively) to each sample. Solvents were later evaporated from a 250 μl aliquot of the supernatant, and the remaining residues were reacted with 250 μl of H2SO4 at 90°C for 10 min. Subsequently, 30 μl duplicates of the resulting sample were mixed with 270 μl of Vanillin reagent (600 mg vanillin dissolved in 100 ml distilled water and 400 ml 85% H3PO4), and the optical density was recorded at 530 nm. Total lipid amounts were calculated using a standard curve generated with Triolein.
Protein analyses were performed by individually homogenizing each fly in 500 μl of phosphate buffered saline. Subsequently, 10 μl duplicates of the resulting supernatant were reacted with 200 μl of diluted Bradford reagent (Biorad, United States), and absorbance was recorded at 595 nm. Total Soluble protein amounts were extrapolated from standard curves generated with bovine serum albumin.
Statistical Data Analysis
Cox proportional hazards models were used to test the effects of acclimation temperature, sex, and RH on survival of the flies in each of the two acclimation periods. Additionally, the risk of death was computed for each group. These models were adjusted for the body weight of the flies at the beginning of the assay, and accordingly, the predicted median survival times were computed by using marginal estimates at the mean weight. During model construction, two-way interactions were examined and confirmed as non-significant. Accordingly, these were not included in the final analyses.
Generalized linear models were used to examine the effects of acclimation temperature and sex on the fresh weight of flies for each of the two acclimation periods. A similar model was employed to examine the effects of acclimation temperature and sex on lipid and protein contents of 18-day old flies. Within these models, means of different groups were separated by Tukey’s HSD comparisons.
Means and SEs are reported. Values of p less than 0.05 were considered statistically significant. Statistical analysis was conducted using the JMP statistical package (SAS, Cary, NC, United States).
Results
Effect of Acclimation Temperature on Weight Gain
Weight gain was significantly affected by acclimation temperature [F(2, 347) = 83.87, p < 0.001] and sex [F(1, 347) = 31.45, p < 0.001] when flies were acclimated for a short (5–10 days) period. Overall, elevated temperatures were associated with a significant increase in weight for both males and females (Tukey HSD comparisons, p < 0.05, Figure 1A). Depending on temperature, females weighed 9.5 ± 0.25, 10.77 ± 0.21, and 13.36 ± 0.21 mg (at 20, 25, and 30°C, respectively). Males were similarly affected by temperature and weighed 9.07 ± 0.28, 9.85 ± 0.20, and 11.38 ± 0.26 mg when acclimated at 20, 25, and 30°C, respectively). Sex had a significant effect on weight only for 25 and 30°C – acclimated flies, resulting in a significant interaction between sex and acclimation temperature [F(1, 347) = 5.36, p = 0.0051, Figure 1A].
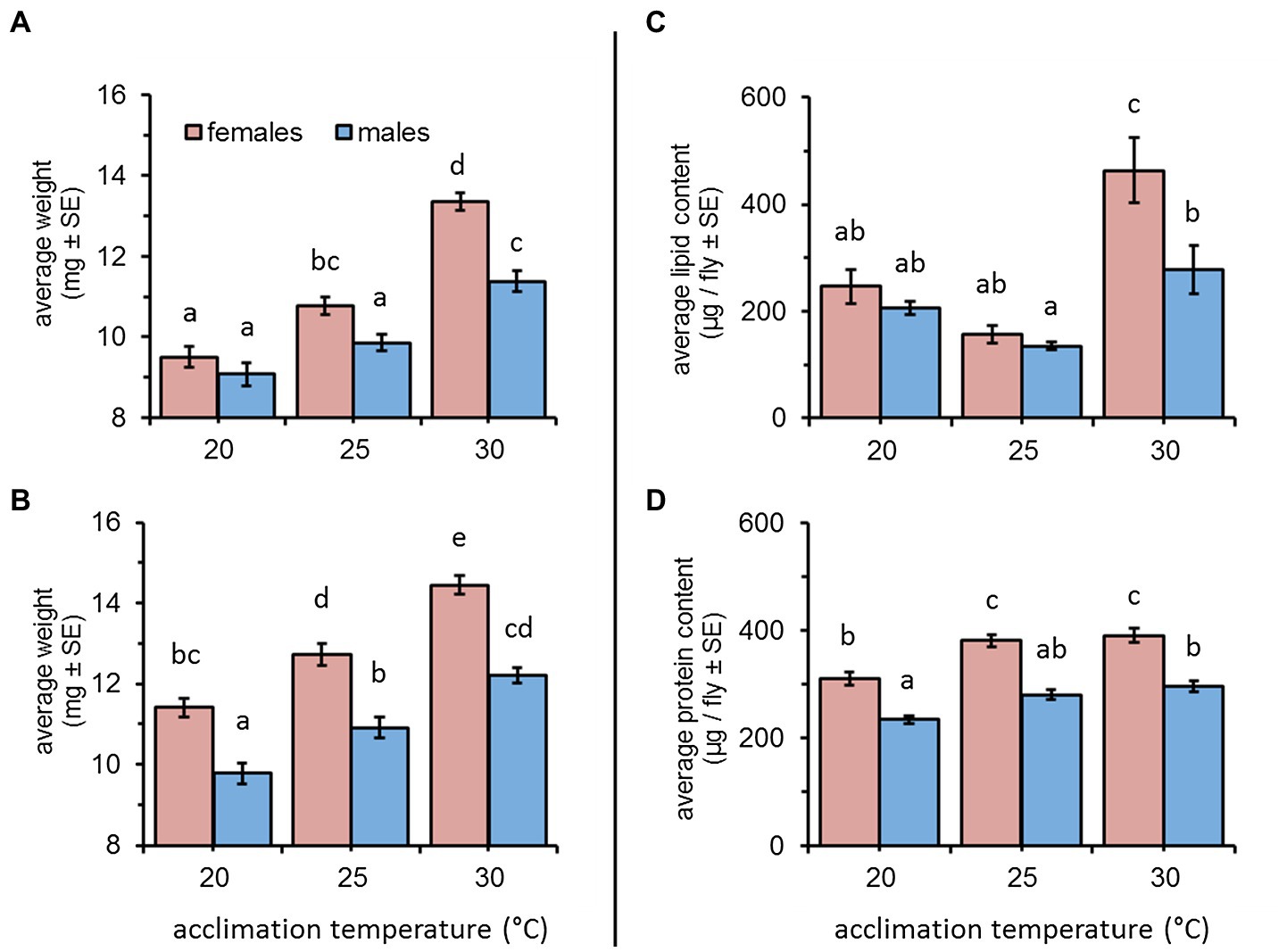
Figure 1. Average weight, lipid, and protein content of female and male Bactrocera zonata acclimated at 20, 25, and 30°C constant temperature regimes. Weight was recorded following a short (5–10 days, A) and long (11–20 days, B) acclimation period (n = 37–59 flies in each group). Lipids (C) and proteins (D) were quantified in individuals acclimated for 18 days (n = 7–15 flies in each group). Means separated by different letters are significantly different (post hoc Tukey HSD comparisons).
Similar effects were recorded for flies acclimated for a period of 11–20 days (acclimation temperature: F(2, 297) = 75.75, p < 0.001); sex: [F(1, 297) = 94.07, p < 0.001]. Here, also weight was significantly increased at elevated temperatures (Tukey HSD comparisons, p < 0.05, Figure 1B). Females weighed 11.40 ± 0.22, 12.71 ± 0.27, and 14.44 ± 0.23 mg, and males weighed 9.77 ± 0.25, 10.91 ± 0.25, and 12.19 ± 0.18 mg when acclimated at 20, 25, and 30°C (respectively). Females were consistently heavier than males, regardless of acclimation temperature (sex × acclimation temperature: F(2, 297) = 1.02, p = 0.360, Figure 1B).
Effect of Acclimation Temperature on Lipid and Protein Reserves
Lipid contents of flies acclimated for 18 days were significantly affected by acclimation temperature [F(2, 68) = 22.83, p < 0.001] and sex [F(1, 68) = 8.86, p = 0.004]. However, the effect of sex differed between the three acclimation temperatures [acclimation temperature × sex: F(2, 68) = 3.45, p = 0.037]. When acclimated at 20°C and 25°C, the lipid contents of males and females remained relatively low (males: 205.34 ± 12.31 and 134.42 ± 6.93; females: 246.20 ± 31.97 and 156.55 ± 17.11 μg lipids/fly, respectively), and was not affected by sex (Tukey HSD comparisons, Figure 1C). Flies acclimated at 30°C accumulated higher lipid levels. This effect was significant for females who nearly doubled their amount of lipid reserves compared to 20 and 25°C – acclimated counterparts (462.98 ± 60.58 μg lipids/fly, Tukey HSD comparisons p ≤ 0.0006, Figure 1C). A similar increase in lipid content was detected for males (277.84 ± 44.30 μg lipids/fly). However, this increase was significantly lower compared to females, and different only compared to 25°C-acclimated counterparts (Tukey HSD comparisons p ≥ 0.037, Figure 1C).
The effect of acclimation temperature on protein reserves was also significant [F(2, 73) = 20.62, p < 0.001] and similar in males and females [acclimation temperature × sex: F(2, 73) = 0.50, p = 0.608]. Regardless of acclimation temperature, females contained significantly more protein than males [sex: F(1, 73) = 84.99, p < 0.001, Tukey HSD comparisons, Figure 1D]. Flies acclimated at 30 and 25°C contained similar protein levels, and for females, these were significantly elevated compared to the protein content of 20°C-acclimated counterparts (390.08 ± 13.17, 380.99 ± 11.34, and 310.08 ± 11.99 μg proteins/fly, respectively, Figure 1D). Male protein content significantly differed between 30 and 20°C-acclimated counterparts (295.62 ± 10.09 and 233.29 ± 7.59 μg proteins/fly, respectively), and remained intermediate at 25°C (280.05 ± 9.22 μg proteins/fly, Tukey HSD comparisons, Figure 1D).
Acclimation Effect on Survival at Low and High Relative Humidity
Flies Assayed Following a Short (5–10 days) Acclimation Period
Survival time of males and females acclimated at 20, 25, and 30°C significantly depended on acclimation temperature [Cox proportional hazards regression analysis: χ2(2) = 42.71, p < 0.001] and RH regimes [χ2(1) = 14.77, p < 0.001; Table 1; Figures 2, 3]. Nevertheless, sex was not a significant factor in our analysis [χ2(1) = 0.11, p = 0.738]. No significant interactions were detected between acclimation temperature, RH regime, and sex, indicating that males and females responded similarly to acclimation and RH treatments. Additionally, body weight was significantly associated with survival [χ2(1) = 50.68, p < 0.001], and the two measures were positively correlated for males and females (see Supplementary Figure S1 for pooled data excluding censored flies). No significant interactions between body weight and sex, acclimation temperature, or RH were detected, indicating that increased fresh weight similarly contributed to the life expectancy of males and females in all treatment groups.
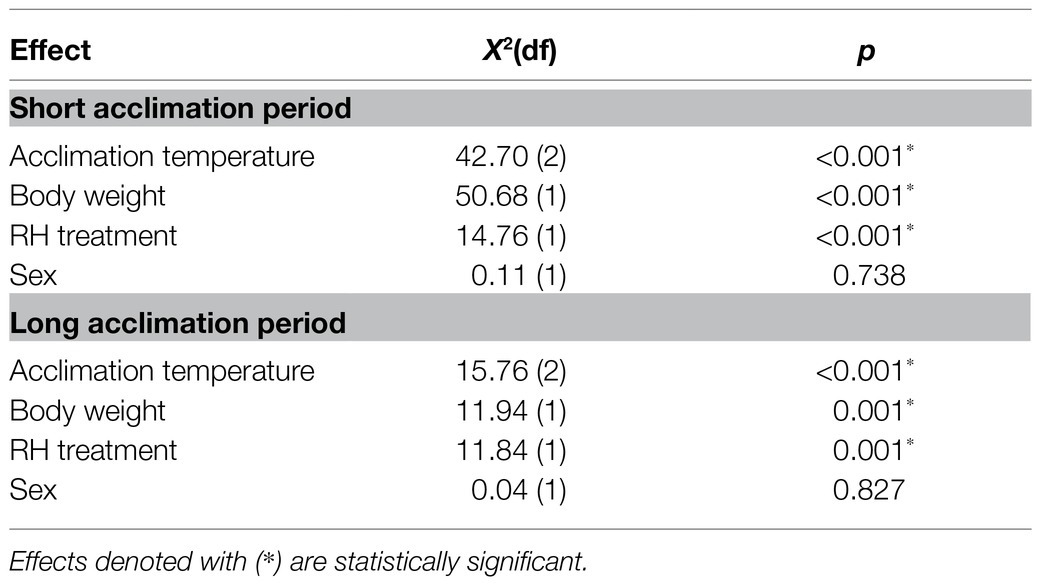
Table 1. Summary of the final Cox proportional hazards regression model describing the effects of acclimation temperature, body weight, relative humidity (RH) regime, and sex on survival of adult Bactrocera zonata exposed to extreme dry (5% RH) or extreme humid (95% RH) environments. Survival was assayed following acclimation at 20, 25, and 30°C constant temperature regimes for a short (5–10 days) or long (11–20 days) acclimation period.
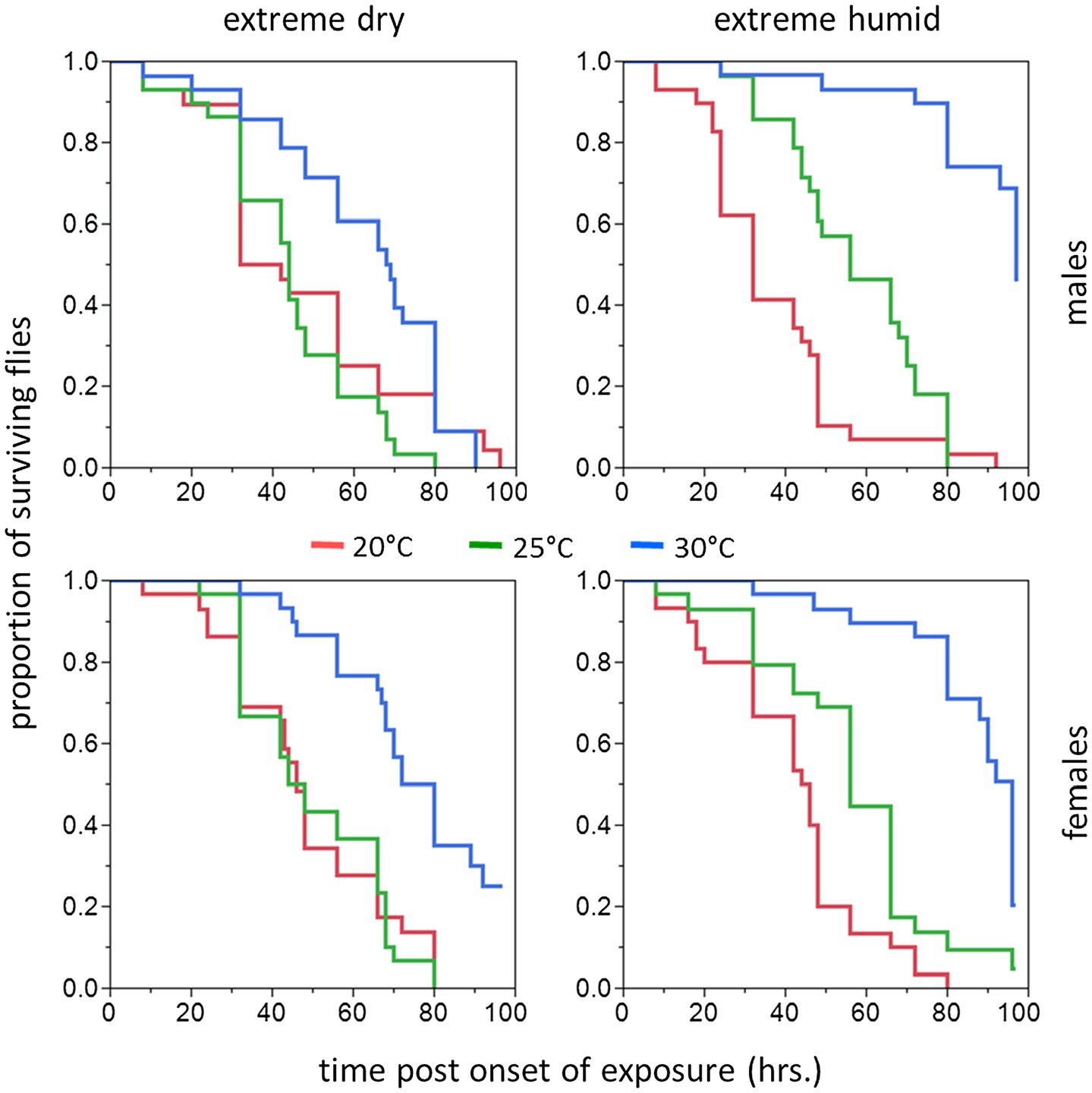
Figure 2. Cumulative survival plots of B. zonata adults exposed to extreme dry (5% RH) or extreme humid (95% RH) environments (Left and Right Panel, respectively) for 5–10 days (short acclimation period). Males (upper panels) and females (lower panels) were assayed following acclimation at 20, 25, and 30°C constant temperature regimes (n = 28–30 flies in each group).
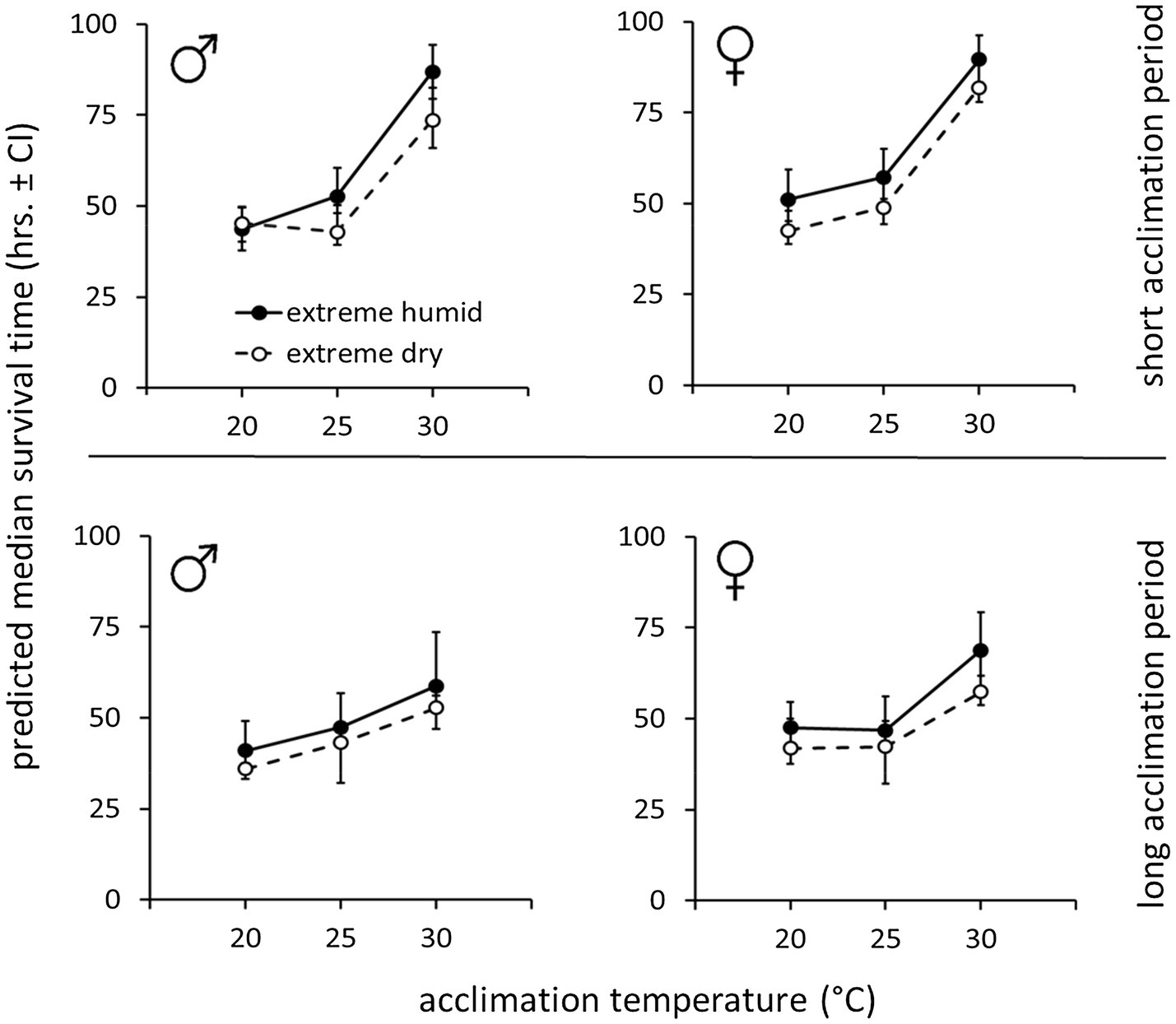
Figure 3. Weight-corrected median lifespan predictions (hours, ±95% CI) of B. zonata males and females maintained under extreme dry (5% RH) or extreme humid (95% RH) environments following acclimation at 20, 25, and 30°C for a short (5–10 days) or long (11–20 days) acclimation period (n = 18–30 flies in each group, total n = 646 flies).
Accordingly, weight-corrected predictions of survival time indicated that males and females gained enhanced endurance and survived longer, when acclimated at 30°C, compared to counterparts maintained at 20 or 25°C (Figure 3, see Table 2 for means ± SE values). Temperature similarly affected survival at the two RH treatments. Nevertheless, flies essayed under 5% RH survived for shorter periods compared to counterparts assayed under 95% RH regardless of acclimation temperature (Figure 3; Table 2).
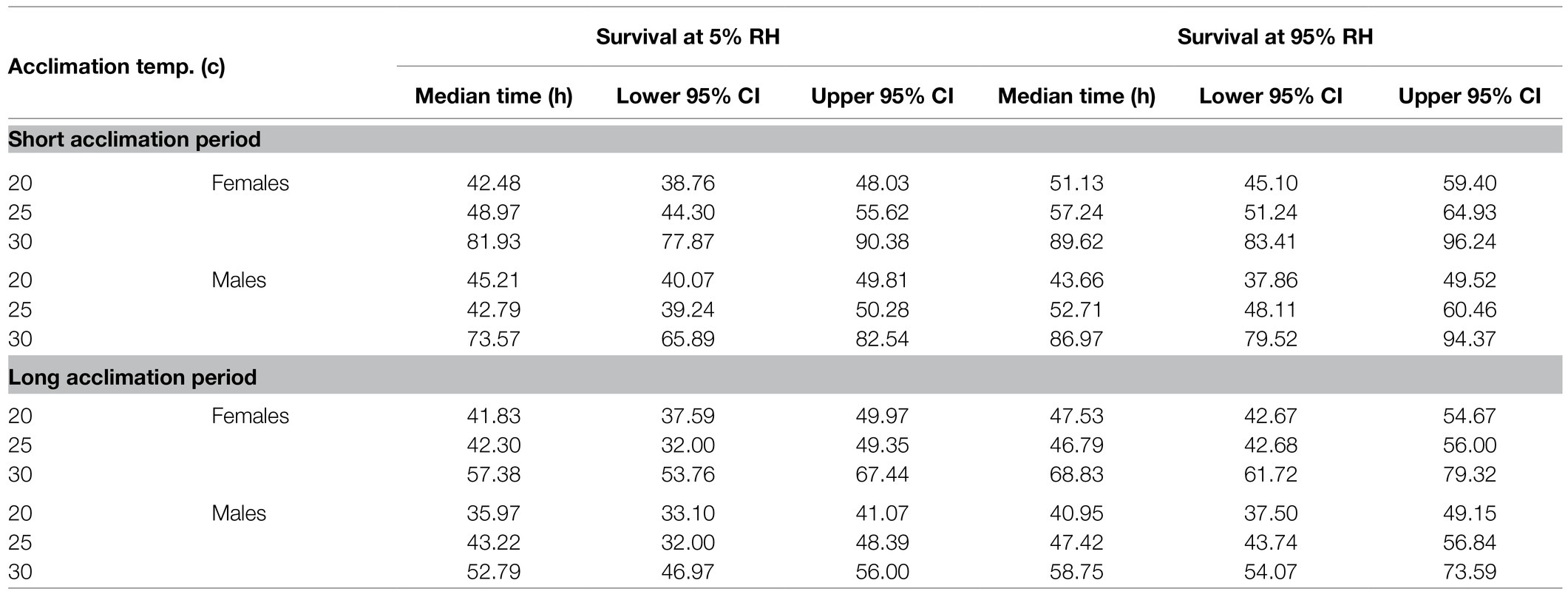
Table 2. Predicted median survival times of males and females exposed to extreme dry (5% RH) or extreme humid (95% RH) environments following acclimation at 20, 25, and 30°C constant temperature regimes for a short (5–10 days) or long (11–20 days) acclimation period. Values represent estimated marginal means (± CI) adjusted for the mean weight of the flies and are depicted in Figure 3.
Hazard ratios calculated by the model corroborated these effects and indicated that the risk of death for flies acclimated at 30°C (pooled males and females at both RH regimes) significantly decreased by 63.1 and 65.8% compared to 20°C [HR (95% CI) = 0.342 (0.242, 0.485), p < 0.001] or 25°C – acclimated counterparts [HR (95% CI) = 0.369 (0.265, 0.512), p < 0.001]. Acclimation at 25°C did not reduce the risk of death [0.927 (0.708, 1.215), p = 0.584] compared to 20°C. Additionally, the risk of death for flies assayed at 95% RH (pooled males and females from all acclimation temperatures) was significantly reduced by 37.4% compared to counterparts maintained at 5% RH [HR (95% CI) = 0.626 (0.492, 0.795), p < 0.001].
Flies Assayed Following a Long (11–20 Days) Acclimation Period
Similarly to counterparts acclimated for a short period, the life expectancy of 11–20 day acclimated males and females was significantly affected by acclimation temperature [χ2(2) = 15.76, p < 0.001] and RH regime [χ2(1) = 11.84, p = 0.001]. Here also, survival times were not affected by sex [χ2(1) = 0.04, p = 0.827, Table 1; Figures 3, 4]. Body weight significantly contributed to life expectancy [χ2(1) = 11.94, p = 0.001] and was positively correlated with male and female survival (see Supplementary Figure S1 for pooled data excluding censored flies). Additionally, no significant two or three way interactions were detected indicating that male and female survival was similarly affected by weight, temperature, and RH regime. Accordingly, weight-corrected lifespan predictions of males and females were generally elevated when flies were acclimated at 30°C, compared to counterparts maintained at 20 and 25°C (Figures 3, 4; Table 2). However, differences appeared to be smaller when compared to counterparts acclimated for a short period of 5–10 days. Additionally, survival at low RH tended to be lower for both males and females at all acclimation temperatures (Figure 3; Table 2).
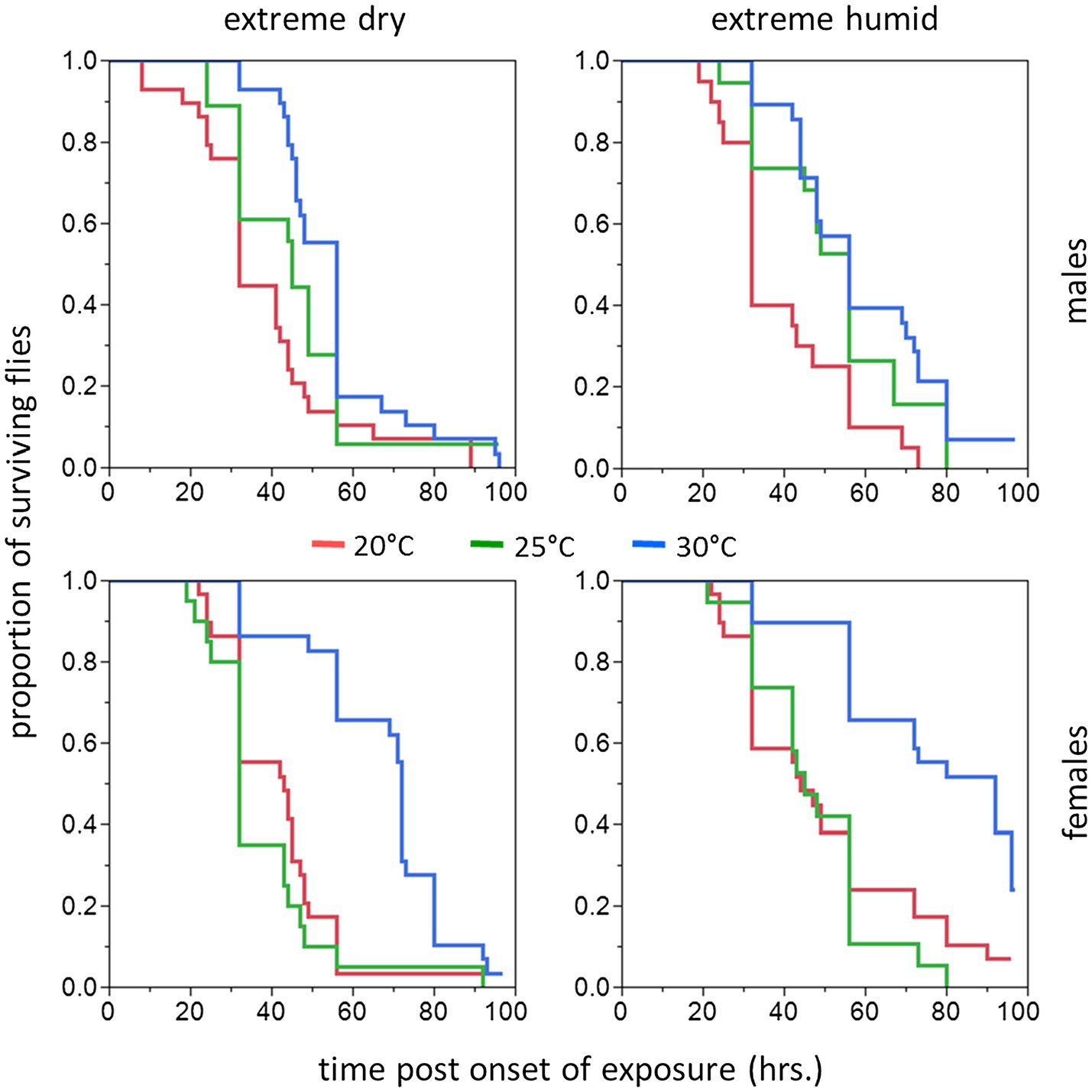
Figure 4. Cumulative survival plots of B. zonata adults exposed to extreme dry (5% RH) or extreme humid (95% RH) environments (Left and Right Panel, respectively) for 11–20 days (long acclimation period). Males (upper panels) and females (lower panels) were assayed following acclimation at 20, 25, and 30°C constant temperature regimes (n = 18–29 flies in each group).
Hazard ratios assigned significant effects to acclimation temperature and RH regime. The risk of death was 45.5–42.4% lower for flies acclimated at 30°C (pooled males and females at both RH regiems) compared to counterparts maintained at 20°C [HR (95% CI) = 0.544 (0.392, 0.754), p < 0.001], or 25°C [HR (95% CI) = 0.576 (0.417, 0.796), p < 0.001]. The risk of death for 20 and 25°C-acclimated flies was similar [HR (95% CI) = 0.943 (0.693, 1.283), p = 0.713]. Additionally, the risk of death for flies challenged with 95% RH (pooled sexes at all acclimation temperatures) was 32.8% lower compared to flies challenged with 5% RH [HR(95% CI) = 0.658 (0.518, 0.835), p < 0.001].
Overall, our results point that regardless of acclimation period, flies gain weight according to the temperature at which they are maintained. Similarly, the buildup of lipid and protein reserves is affected by temperature, and acclimation at 30°C resulted in significantly elevated values of all of these measures. After correcting for the positive effect of weight on survival, overall lifetime predictions of males and females were found to be prolonged following acclimation at 30°C. This effect was consistent regardless of acclimation period or the ambient humidity at which the flies were maintained. Finally, we found that survival time was reduced in the low humidity environment.
Discussion
Effect of Acclimation Temperature
Acclimation at constant temperature regimes had a profound effect on the survival of B. zonata in our experiments (Table 1; Figure 3). For both males and females, preconditioning at 30°C resulted in higher survival rates at both low and high humidity regimes compared to counterparts acclimated at 20 and 25°C. During these bioassays, flies were subjected to contrasting RH regimes while simultaneously deprived of food, thus experiencing stress from desiccation and starvation alike (a widely used setting for testing desiccation resistance in Drosophila, Gibbs et al., 1997). Thus, the exact mechanisms triggered by temperature and which contributed to survival in our experiments are difficult to pinpoint. Nevertheless, the difference in mortality rates in the two RH regimes suggests that under high RH, where intrinsic water is retained more readily, flies eventually died of starvation. Conversely, in the desiccating environment, acute water loss may have preceded or complemented the depletion of nutritional reserves and eventually resulted in higher mortality rates.
The contribution of acclimation at 30°C to survival at both RH regimes suggests that a similar mechanism acted in both cases. Our results point that weight, associated with acclimation temperature may be involved. Flies maintained for 5–10 days at 30°C were 33 and 19.7% heavier compared to flies acclimated at 20 and 25°C (respectively). Similarly, flies maintained at 30°C for 11–20 days were 25.7 and 12.7% heavier compared to 20 and 25°C – acclimated counterparts. This gain in weight was positively and significantly correlated with the survival of the flies (Table 1; Supplementary Figure S1). Accordingly, we suggest that the elevated survival rates of 30°C-acclimated flies resulted, at least partly, from temperature-mediated gain in weight. Although not quantified in our study, foraging activity seemed to be modulated by temperature, and was encouraged in flies maintained at 30°C (D. Nestel, personal observations). Thus, increased feeding activity and possibly accelerated metabolism probably accounted for the recorded weight gain and contributed to survival following acclimation at 30°C.
Similar effects of acclimation temperature on weight gain were reported for other insects as well (Weldon et al., 2013; Fischer and Kirste, 2018), and weight was previously associated with increased tolerance to desiccation in some tephritids (Weldon et al., 2013, 2016). However, in other cases, the correlation between body weight and survival under different temperature and RH regimes was uncertain (e.g., C. capitata, Weldon et al., 2018). Our analysis suggests that weight gain affected survival only indirectly, possibly through temperature-dependent accumulation of nutritional reserves. We base this conclusion on the following observations: first, the analysis we employed estimated weight-adjusted survival times to be higher in flies acclimated at 30°C (Figure 3), suggesting that temperature, in addition to weight-gain contributed to increased survival. Secondly, survival was not affected by sex although females were generally heavier than males. Finally, our analysis reveals similar survival patterns in flies acclimated for 5–10 days and 11–20 days, regardless of increased weight of the latter (Figures 1A,B). This suggests that body weight may be an indirect predictor of survival and highlight the possibility that accumulation of nutritional reserves mediated by temperature were responsible for prolonging survival in our study.
In this regard, lipids or other nutritional reserves (e.g., glycogen) may have been involved in mediating survival under stress. Lipids and glycogen are used as an energy source during starvation and their catabolism yields substantial amounts of water as a metabolic byproduct, thus contributing to desiccation resistance as well (Gibbs et al., 1997; Arrese and Soulages, 2010). The association between lipid utilization and enhanced survival during starvation and desiccation resistance assays applies for fruit flies as well (e.g., Tejeda et al., 2014; Weldon et al., 2016). According to our study, males and females acclimated at 30°C accumulated significantly higher lipid levels then counterparts maintained at 20 and 25°C (Figure 1C). Their resistance to starvation and desiccation was thus probably better supported by these resources. Nevertheless, females contained significantly more lipids than males, which may be related to the accumulation of lipoprotein during egg maturation (e.g., Haq et al., 2010; Yadav and Singh, 2018). Higher lipid loads in females could have been expected to increase their survival compared to males. However, this situation was not observed. Possibly, some of these reserves remain inaccessible once eggs are produced, excluding the possibility of egg-reabsorption and use of lipids for maintenance (Aluja et al., 2011). Thus, although, we do not measure lipid allocation for reproduction and maintenance, it is possible that the load of lipid reserves aimed at maintenance are similar in both sexes, resulting in similar survival patterns of males and females. These possibilities require further studies.
Similarly to lipids, protein content was also associated with acclimation temperature, but in this case, flies maintained at 25 and 30°C accumulated similar levels of protein (Figure 1D). These results, together with other studies suggest that protein reserves contribute negligibly to survival under starvation and desiccation stress (see Weldon et al., 2016, 2019). Taking it altogether, we assume that accumulation of nutritional reserves (possibly lipids) resulting from acclimation at higher temperatures, promoted the tolerance of B. zonata to starvation and desiccation in our study.
Effect of RH
Survival was significantly reduced under the low RH regime, indicating that desiccation was a significant stress factor for the flies in our experiments. Additionally, acclimation at 30°C prolonged survival under low RH conditions.
Increased resistance to desiccation can potentially be achieved through efficient water management. In this context, three mechanisms have been suggested: increased water storage (e.g., by increased body size or lipid catabolism), ability to reduce water loss (e.g., by respiratory adaptations or excretion of cuticular lipids), and increased tolerance to water loss through activation of stress-induced osmoregulatory mechanisms (reviewed by Weldon et al., 2019). One or more of these mechanisms may have acted either alone or in conjunction to support the survival of B. zonata under low RH in our experiments. However, if acclimation temperature had affected any of these mechanisms, we would expect a significant interaction between acclimation temperature and RH treatment (which was not detected). Accordingly, as it stands we cannot provide evidence as to the effect acclimation temperature through such mechanisms in our experiment. Nevertheless, the fact that acclimation at 30°C similarly affected survival in the two RH regimes suggest that it acted mainly through increasing resistance to starvation.
Effect of Sex
Contrary to acclimation temperature and humidity, sex was not a significant predictor of survival in our dataset (Table 1; Figure 3). This finding contrasts with studies on other tephritids, such as Bactrocera. tryoni (Froggatt; Weldon and Taylor, 2010; Weldon et al., 2013), Anastrepha ludens (Loew; Tejeda et al., 2014), and Ceratitis capitata (Wiedmann; Weldon et al., 2016) highlighting males as more tolerant to desiccation compared to females. Nevertheless, these sex-related differences in desiccation resistance seem to depend on temperature, age, and origin of the flies, and may be reversed in other species (see Matzkin et al., 2009; Weldon and Taylor, 2010; Weldon et al., 2013, 2016). For example, female tolerance to desiccation may be related to partitioning of water resources between egg production and soma maintenance, i.e., that water contained in eggs remain inaccessible to gravid females, thus, reducing their tolerance to low humidity (Weldon et al., 2013, 2016). If females in our experiments were not yet producing eggs (sexual maturity in our B. zonata colony takes more than 15 days), they were probably able to sequester available water and survive similarly to males. Alternatively, if eggs were being produced the partitioning of lipids between maintenance and reproduction may have played part in determining survival (as discussed above). In any case, differences in male and female survival seem to be conditional on the context of the experimental setting and involved species, and need to be interpreted accordingly.
Effect of Acclimation Period
Although we do not compare flies of different acclimation periods, our results point that survival was similarly affected by temperature and humidity regardless of the flies preconditioning duration. Thus, a short acclimation period of 5–10 days at 30°C had a similar effect as a long acclimation, and was sufficient to prolong survival in our experiments. Nevertheless, flies acclimated for a long period were generally less tolerant and survived for shorter periods compared with younger counterparts. This seems to apply particularly for males (Figure 3), and may be related to the older age of the flies. Indeed, age was found to significantly affect survival under starvation and desiccation stress in other tephritids (Weldon and Taylor, 2010), as well as in Drosophilla (Gibbs and Markow, 2001). In the latter case, age was associated with greater rates of water loss leading to mortality under desiccating conditions. A decline in desiccation resistance in older flies was previously suggested to associate with allocation of resources to support reproductive organs or sexual activity (see Weldon and Taylor, 2010). Our experiments do not allow for isolating specific factors contributing to possible age-associated differences in survival.
Thermal Acclimation and Tolerance to Humidity Stress
Regardless of the strong climatic association between temperature and humidity, the effect of thermal acclimation on tolerance to humidity stress has been poorly studied in insects (Terblanche and Hoffmann, 2020). The few studies conducted up to date have shown, even within the same species, an inconsistent and varied desiccation resistance response resulting from adaptive phenotypic plasticity to thermal acclimation (Fischer and Kirste, 2018). For example, while thermal treatment during exposure to hydric stress of a laboratory strain of C. capitata affected tolerance to humidity stress (Weldon et al., 2016), field collected populations from different mesic and xeric environments with diverse thermal regimes, showed inconsistent tolerance to hydric stress (Weldon et al., 2018). Similarly, different species of tsetse flies derived from laboratory colonies showed diverse and complex water balance responses to humidity stress when exposed to various combined temperature and humidity conditions (Kleynhans and Terblanche, 2011). In some cases, the resulting responses, both in C. capitata and tsetse flies, contrasted with the response predicted from the geographic occupancy of the species (i.e., species derived from xeric or mesic environments; Kleynhans and Terblanche, 2011; Weldon et al., 2018). The observed variability may point to different laboratory methodologies followed to explore acclimation effects on the tolerance to extreme climatic conditions, or the effect of genotypic variability within and between populations (Terblanche and Hoffmann, 2020). In case of the present study, we used a laboratory colony, which probably have a reduced genetic variability and thus express a relatively uniform physiological response to temperature acclimation and RH. We also applied a uniform experimental protocol to all the assayed individuals, minimizing random effects resulting from genetic and methodological factors. Our results clearly show that acclimation at 30°C positively affect the survival of B. zonata in extreme high and low humidity conditions. We assume that temperature acted by modulation of feeding and metabolism, resulting in accumulation of weight and nutritional reserves. Consequently, survival was affected mainly through the increase of the flies resistance to starvation.
Summary
Native to the humid tropics of South and South-East Asia (EPPO Bulletin, 2010), B. zonata has dispersed to North Africa and east Mediterranean (EPPO Bulletin, 2010) regions characterized by dry climatic conditions. Given its apparent adaptability to humidity stress in its habitat, the species has a large potential to expand its range further into Mediterranean Europe, where it is considered a type A1 pest by the European and Mediterranean Plant Protection Organization (EPPO Bulletin, 2010), and to other tropical and subtropical areas of the World (Ni et al., 2012). Herein, we demonstrate that thermal acclimation contribute to desiccation resistance of this fly. Our results suggest that acclimation temperature indirectly affect survival through modulation of feeding and accumulation of nutritional reserves. Accordingly, we highlight the conditioning of metabolism as a major link between thermal acclimation and desiccation resistance. This study, thus, set the basis to understand the current geographic range of the species from the hydric perspective. The thermal limits of its expansion still need to be clarified and studied in order to produce more accurate risk maps and prepare preventive actions to reduce risk of introduction. This evaluation is currently underway in our laboratory.
Data Availability Statement
The raw data supporting the conclusions of this article will be made available by the authors, without undue reservation.
Author Contributions
DN conceived and planned the study. YA and EN-L executed the experiments. MB-Y and EV analyzed the data. DN, MB-Y, NP, and EV contributed to writing and approval of the manuscript. All authors contributed to the article and approved the submitted version.
Funding
This study was funded by the European Union’s Horizon 2020 Research and Innovation Program FFIPM (grant agreement No 818184).
Conflict of Interest
The authors declare that the research was conducted in the absence of any commercial or financial relationships that could be construed as a potential conflict of interest.
Publisher’s Note
All claims expressed in this article are solely those of the authors and do not necessarily represent those of their affiliated organizations, or those of the publisher, the editors and the reviewers. Any product that may be evaluated in this article, or claim that may be made by its manufacturer, is not guaranteed or endorsed by the publisher.
Acknowledgments
We thank Constantin Grach for helping in the early establishment of assay systems and Yoav Gazit (The Israel Cohen Institute for Biological Control) for supplying flies for the study.
Supplementary Material
The Supplementary Material for this article can be found online at: https://www.frontiersin.org/articles/10.3389/fphys.2021.686424/full#supplementary-material
References
Aluja, M., Birke, A., Guillen, L., Diaz-Fleischer, F., and Nestel, D. (2011). Coping with an unpredictable and stressful environment: the life history and metabolic responses to variable food and host availability in a polyphagous tephritid fly. J. Insect Physiol. 57, 1592–1601. doi: 10.1016/j.jinsphys.2011.08.001
Arrese, E. L., and Soulages, J. L. (2010). Insect fat body: energy, metabolism, and regulation. Annu. Rev. Entomol. 55, 207–225. doi: 10.1146/annurev-ento-112408-085356
Beaman, J. E., White, C. R., and Seebacher, F. (2016). Evolution of plasticity: mechanistic link between development and reversible acclimation. Trends Ecol. Evol. 31, 237–249. doi: 10.1016/j.tree.2016.01.004
Boardman, L., and Terblanche, J. S. (2015). Oxygen safety margins set thermal limits in an insect models system. J. Exp. Biol. 218, 1677–1685. doi: 10.1242/jeb.120261
Bubliy, O. A., Kristensen, T. N., Kellerman, V., and Loeschcke, V. (2012). Humidity affects genetic architecture of host resistance in Drosophila melanogaster. J. Evol. Biol. 25, 1180–1188. doi: 10.1111/j.1420-9101.2012.02506.x
Bujan, J., Charavel, E., Bates, O. K., Gippet, J. M., Darras, H., Lebas, C., et al. (2021). Increased acclimation ability accompanies a thermal niche shift of a recent invasion. J. Anim. Ecol. 90, 483–491. doi: 10.1111/1365-2656.13381
Chown, S. L., Sørensen, J. G., and Terblanche, J. S. (2011). Water loss in insects: an environmental change perspective. J. Insect Physiol. 57, 1070–1084. doi: 10.1016/j.jinsphys.2011.05.004
Contreras, H. L., and Bradley, T. J. (2011). The effect of ambient humidity and metabolic rate on the gas-exchange pattern of the semi-aquatic insect Aquarius remiges. J. Exp. Biol. 214, 1086–1091. doi: 10.1242/jeb.050971
EPPO Bulletin (2005). Bactrocera zonata. EPPO Bull. 35, 371–373. doi: 10.1111/j.1365-2338.2005.00848.x
EPPO Bulletin (2010). Bactrocera zonata: procedure for official control. EPPO Bull. 40, 390–395. doi: 10.1111/j.1365-2338.2010.02421.x
Fischer, K., and Kirste, M. (2018). Temperature and humidity acclimation increase desiccation resistance in the butterfly Bicyclus anynana. Entomol. Exp. Appl. 166, 289–297. doi: 10.1111/eea.12662
Gazit, Y., and Akiva, R. (2017). Toxicity of malathion and spinosad to Bactrocera zonata and Ceratitis capitata (Diptera: Tephritidae). Fla. Entomol. 100, 385–389. doi: 10.1653/024.100.0240
Gefen, E., Marlon, A. J., and Gibbs, A. G. (2006). Selection for desiccation resistance in adult Drosophila melanogaster affects larval development and metabolite accumulation. J. Exp. Biol. 209, 3293–3300. doi: 10.1242/jeb.02397
Gibbs, A. G., Chippindale, A. K., and Rose, M. R. (1997). Physiological mechanisms of evolved desiccation resistance in Drosophila melanogaster. J. Exp. Biol. 200, 1821–1832. doi: 10.1242/jeb.200.12.1821
Gibbs, A. G., Louie, A. K., and Ayala, J. A. (1998). Effects of temperature on cuticular lipids and water balance in a desert Drosophila: is thermal acclimation beneficial? J. Exp. Biol. 201, 71–80. doi: 10.1242/jeb.201.1.71
Gibbs, A. G., and Markow, T. A. (2001). Effects of age on water balance in Drosophila species. Physiol. Biochem. Zool. 74, 520–530. doi: 10.1086/322162
Haq, I., Mayr, L., Teal, P. E. A., Hendrichs, J., Robinson, A. S., Stauffer, C., et al. (2010). Total body nitrogen and total body carbon as indicators of body protein and body lipids in the melon fly Bactrocera cucurbitae: effects of methoprene, a juvenile hormone analogue, and diet supplementation with hydrolyzed yeast. J. Insect Physiol. 56, 1807–1815. doi: 10.1016/j.jinsphys.2010.07.011
Kalra, B., Tamang, A. M., and Parkash, R. (2017). Cross-tolerance effects due to adult heat hardening, desiccation and starvation acclimation of tropical drosophilid-Zaprionus indianus. Comp. Biochem. Physiol. A Mol. Integr. Physiol. 209, 65–73. doi: 10.1016/j.cbpa.2017.04.014
Kellermann, V., Hoffmann, A. A., Overgaard, J., Loeschcke, V., and Sgro, C. M. (2018). Plasticity for desiccation tolerance across Drosophila species is affected by phylogeny and climate in complex ways. Proc. Biol. Sci. 285:20180048. doi: 10.1098/rspb.2018.0048
Kleynhans, E., and Terblanche, J. S. (2011). Complex interactions between temperature and relative humidity on water balance of adult TseTse (Glossinidae, Diptera): implications for climate change. Front. Physiol. 2:74. doi: 10.3389/fphys.2011.00074
Little, C. M., Chapman, T. W., and Hillier, N. K. (2020). Plasticity is key to success of Drosophila suzukii (Diptera: Drosophilidae) invasion. J. Insect Sci. 20:5. doi: 10.1093/jisesa/ieaa034
Lockwood, J. L., Hoopes, M. F., and Marchetti, M. P. (2007). Invasion Ecology. Malden, MA: Blackwell Publishing.
Matzkin, L. M., Watts, T. D., and Markow, T. A. (2009). Evolution of stress resistance in Drosophila: interspecific variation in tolerance to desiccation and starvation. Funct. Ecol. 23, 521–527. doi: 10.1111/j.1365-2435.2008.01533.x
Meurisse, N., Rassati, D., Hurley, B. P., Brockerhoff, E. G., and Haack, R. A. (2019). Common pathways by which non-native forest insects move internationally and domestically. J. Pest. Sci. 92, 13–27. doi: 10.1007/s10340-018-0990-0
Mohammadzadeh, M., and Izadi, H. (2018). Cooling rate and starvation affect supercooling point and cold tolerance of the Khapra beetle, Trogoderma granarium everts fourth instar larvae (Coleoptera: Dermestidae). J. Therm. Biol. 71, 24–31. doi: 10.1016/j.jtherbio.2017.10.007
Nestel, D., Nemny-Lavy, E., and Ling Chang, C. (2004). Lipid and protein loads in pupating larvae and emerging adults as affected by the composition of Mediterranean fruit fly (Ceratitis capitata) meridic larval diets. Arch. Insect Biochem. Physiol. 56, 97–109. doi: 10.1002/arch.20000
Ni, W. L., Li, Z. H., Chen, H. J., Wan, F. H., Qu, W. W., Zhang, Z., et al. (2012). Including climatic change in pest risk assessment: the peach fruit fly, Bactrocera zonata (Diptera: Tephritidae). Bull. Entomol. Res. 102, 173–183. doi: 10.1017/S0007485311000538
Ouisse, T., Day, E., Laville, L., Hendricks, F., Convey, P., and Renault, D. (2020). Effects of elevational range shift on the morphology and physiology of a carabid beetle invading the sub-Antarctic Kerguelen Islands. Sci. Rep. 10:1234. doi: 10.1038/s41598-020-57868-0
Portnov, B. A., and Safriel, U. N. (2004). “Prospective desertification trends in the Negev—implications for urban and regional development,” in Environmental Challenges in the Mediterranean 2000–2050 (NATO Science Series, Series IV: Earth and Environmental Sciences). Vol. 37. ed. A. Marquina (Dordrecht: Springer).
Raza, M. F., Wang, Y., Cai, Z., Bai, S., Yao, Z., Awan, U. A., et al. (2020). Gut microbiota promotes host resistance to low-temperature stress by stimulating its arginine and proline metabolism pathway in adult Bactrocera dorsalis. PLoS Pathog. 16:e1008441. doi: 10.1371/journal.ppat.1008441
Sgro, C. M., Terblanche, J. S., and Hoffmann, A. A. (2016). What can plasticity contribute to insect responses to climate change? Annu. Rev. Entomol. 61, 433–451. doi: 10.1146/annurev-ento-010715-023859
Tay, W. T., and Gordon, H. J. (2019). Going global—genomic insights into insect invasions. Curr. Opin. Insect Sci. 31, 123–130. doi: 10.1016/j.cois.2018.12.002
Tejeda, M. T., Arredodndo, J., Perez-Staples, D., Ramos-Morales, P., Liedo, P., and Diaz-Fleischer, F. (2014). Effects of size, sex and teneral resources on the resistance to hydric stress in the tephritid fruit fly Anastrepha ludens. Insect Physiol. 70, 73–80. doi: 10.1016/j.jinsphys.2014.08.011
Terblanche, J. S., and Chown, S. L. (2006). The relative contributions of developmental plasticity and adult acclimation to physiological variation in the tsetse fly, Glossina pallidipes (Diptera, Glossinidae). J. Exp. Biol. 209, 1064–1073. doi: 10.1242/jeb.02129
Terblanche, J. S., and Hoffmann, A. A. (2020). Validating measurements of acclimation for climate change adaptation. Curr. Opin. Insect Sci. 41, 7–16. doi: 10.1016/j.cois.2018.12.002
Terblanche, J. S., Marais, E., Hetz, S. K., and Chown, S. L. (2008). Control of discontinuous gas exchange in Samia cynthia: effects of atmospheric oxygen, carbon dioxide and moisture. J. Exp. Biol. 211, 3272–3280. doi: 10.1242/jeb.022467
Terblanche, J. S., Nyamukondiwa, C., and Kleynhans, E. (2010). Thermal variability alters climatic stress resistance and plastic responses in a globally invasive pest, the Mediterranean fruit fly (Ceratitis capitata). Entomol. Exp. Appl. 137, 304–315. doi: 10.1111/j.1570-7458.2010.01067.x
Terblanche, J. S., Sinclair, B. J., Klok, C. J., McFarlane, M. L., and Chown, S. L. (2005). The effects of acclimation on thermal tolerance, desiccation resistance and metabolic rate in Chirodica chalcoptera (Coleoptera: Chrysomelidae). J. Insect Physiol. 51, 1013–1023. doi: 10.1016/j.jinsphys.2005.04.016
Thorat, L., and Nath, B. B. (2018). Insects with survival kits for desiccation tolerance under extreme water deficits. Front. Physiol. 9:1843. doi: 10.3389/fphys.2018.01843
Tsitsipis, J. A. (1989). “Nutrition: requirements,” in World Crop Pests: Fruit Flies: Their Biology, Natural Enemies and Control. Vol. 3A. eds. A. S. Robinson and G. Hooper (Amsterdam, NL: Elsevier), 103–119.
Weldon, C. W., Boardman, L., Marlin, D., and Terblanche, J. S. (2016). Physiological mechanisms of dehydration tolerance contribute to the invasion potential of Ceratitis capitata (Wiedmann) relative to its less widely distributed congeners. Front. Zool. 13:15. doi: 10.1186/s12983-016-0147-z
Weldon, C. W., Díaz-Fleischer, F., and Pérez-Staples, D. (2019). “Desiccation resistance of tephritid flies,” in Area-Wide Management of Fruit Fly Pests. eds. D. Perez-Staples, F. Diaz-Fleischer, P. Montoya, and M. T. Vera (Boca Raton, FL: CRC Press), 27–43.
Weldon, C. W., Nyamukondiwa, C., Karsten, M., Chown, S. L., and Terblanche, J. S. (2018). Geographic variation and plasticity in climate stress resistance among southern Africa populations of Ceratitis capitata (Wiedmann; Diptera: Tephritidae). Sci. Rep. 8:9849. doi: 10.1038/s41598-018-28259-3
Weldon, C. W., and Taylor, P. W. (2010). Desiccation resistance of adult Queensland fruit flies Bactrocera tryoni decreases with age. Physiol. Entomol. 35, 385–390. doi: 10.1111/j.1365-3032.2010.00744.x
Weldon, C. W., Yap, S., and Taylor, P. W. (2013). Desiccation resistance of wild and mass-reared Bactrocera tryoni (Diptera: Tephritidae). Bull. Entomol. Res. 103, 690–699. doi: 10.1017/S0007485313000394
Yadav, J. P., and Singh, P. (2018). Effect of metabolites on stress, adaptation and longevity in laboratory populations of Drosophila flies. J. Zool. 305, 43–52. doi: 10.1111/jzo.12535
Keywords: Tephritidae, Bactrocera zonata, desiccation resistance, temperature acclimation, nutritional reserves
Citation: Ben-Yosef M, Verykouki E, Altman Y, Nemni-Lavi E, Papadopoulos NT and Nestel D (2021) Effects of Thermal Acclimation on the Tolerance of Bactrocera zonata (Diptera: Tephritidae) to Hydric Stress. Front. Physiol. 12:686424. doi: 10.3389/fphys.2021.686424
Edited by:
Bin Tang, Hangzhou Normal University, ChinaReviewed by:
Changying Niu, Huazhong Agricultural University, ChinaWeiwei Zheng, Huazhong Agricultural University, China
Hamzeh Izadi, Vali-e-Asr University of Rafsanjan, Iran
Michele Cesari, University of Modena and Reggio Emilia, Italy
Copyright © 2021 Ben-Yosef, Verykouki, Altman, Nemni-Lavi, Papadopoulos and Nestel. This is an open-access article distributed under the terms of the Creative Commons Attribution License (CC BY). The use, distribution or reproduction in other forums is permitted, provided the original author(s) and the copyright owner(s) are credited and that the original publication in this journal is cited, in accordance with accepted academic practice. No use, distribution or reproduction is permitted which does not comply with these terms.
*Correspondence: Michael Ben-Yosef, bWljaGFlbGJAdm9sY2FuaS5hZ3JpLmdvdi5pbA==