- 1Experimental Surgery and Regenerative Medicine, Department of General, Trauma and Reconstructive Surgery, Munich University Hospital, Munich, Germany
- 2Faculty of Sport and Health Sciences, Technical University of Munich, Munich, Germany
In skeletal muscle tissue, oxygen (O2) plays a pivotal role in both metabolism and the regulation of several intercellular pathways, which can modify proliferation, differentiation and survival of cells within the myogenic lineage. The concentration of oxygen in muscle tissue is reduced during embryogenesis and pathological conditions. Myogenic progenitor cells, namely satellite cells, are necessary for muscular regeneration in adults and are localized in a hypoxic microenvironment under the basal lamina, suggesting that the O2 level could affect their function. This review presents the effects of reduced oxygen levels (hypoxia) on satellite cell survival, myoblast regeneration and differentiation in vertebrates. Further investigations and understanding of the pathways involved in adult muscle regeneration during hypoxic conditions are maybe clinically relevant to seek for novel drug treatments for patients with severe muscle damage. We especially outlined the effect of hypoxia-inducible factor 1-alpha (HIF1A), the most studied transcriptional regulator of cellular and developmental response to hypoxia, whose investigation has recently been awarded with the Nobel price.
Introduction
Since the great oxygenation event around 2.4 billion years ago, living organisms evolved in an oxygen-containing environment and many of today's species cannot survive without it. Oxygen (O2) plays a pivotal role in metabolic signaling, energy production and cellular homeostasis of aerobic organisms. At sea level, the atmospheric oxygen is ~21% in air generating a partial O2 pressure of about 160 mmHg. However, the physiological O2 levels in different tissues are significantly lower. For example, within skeletal muscle, the oxygen fraction generally varies between 2 and 10% (15–76 mmHg), depending on their location (Greenbaum et al., 1997).
A reduced level, tension or the deprivation of oxygen is termed hypoxia. For skeletal muscle tissues, the criteria for hypoxia are met if oxygen is below ≈2% (Yun et al., 2005). Hypoxia can be physiological as e.g., during embryogenesis (Dunwoodie, 2009), as a result of stimuli, such as high altitude and exercise (Sliwicka et al., 2017), or it can be caused by pathological defects, such as torn muscle fibers (Itoigawa et al., 2010) or peripheral arterial diseases (Norgren et al., 2007).
Skeletal muscles originate from somites along the rostro-caudal axis by fusion of myoblasts to mature myofibers. After embryogenesis, some myogenic progenitor cells (MPCs) remain quiescent and localize between the sarcolemma and the basal membrane. These cells are termed satellite cells and are the resident stem cells of skeletal muscle. Satellite cells are positive for paired-box transcriptional factor 7 (PAX7) but do not express myogenic differentiation 1 (MYOD1) (Zammit et al., 2004). Once activated by extrinsic factors, they express MYOD1 and become satellite cells-derived myoblasts, which regenerate muscle and contribute to muscle hypertrophy. Studies that stain satellite cell-related cells for markers such as PAX7 and MYOD1 have revealed the following cell types: PAX7+/MYOD1− (quiescent and self-renewing satellite cells), PAX7+/MYOD1+ (activated, proliferating satellite cell-derived myoblasts) and PAX7−/MYOD1+/Myogenin+ (myoblasts that differentiate into multinuclear myotubes) (Liu et al., 2012).
During skeletal muscle development and regeneration, satellite cells are frequently exposed to hypoxia in their microenvironments. These so-called hypoxic niches have been reported to be critical for the regulation of satellite cell activation, self-renewal, proliferation and differentiation at the site of injured skeletal muscle (Parmar et al., 2007). This affects the activity of oxygen-sensing pathways, including the NOTCH-(Gustafsson et al., 2005), PI3K-AKT-mTOR- (Ren et al., 2010), MAPK14- (Ren et al., 2010) and HIF1α-depended pathways (Majmundar et al., 2015) and in turn these pathways then regulate hypoxia-induced responses. Amongst these pathways, the best investigated oxygen responsive factor is the transcription factor hypoxia-inducible factor 1α (HIF1A).
In this systematic review, we will discuss the molecular adaption of MPCs and satellite cells to hypoxia, focusing on RNA and protein level, as an understanding of the role of hypoxia in the myogenic lineage could be critical to develop hypoxia-related therapies for muscular conditions. To create the review, we searched for all data based on skeletal muscle tissue and hypoxia, excluding clinical trials (Supplementary Figure 1). The review was structured by the myogenic timeline of muscle cell regeneration, starting with self-renewal, followed by proliferation and finishing with differentiation.
Methods
Search Strategy
We searched PubMed and Web of Science for the keyword combinations shown in Table 1.
Duplicates and articles in languages other than English were excluded from the obtained results. The remaining articles received with these keyword combinations were screened by their title and abstract, by excluding all clinical studies, studies including adipocytes, cardiac or smooth muscle cells. Residual manuscripts were screened by their full text, reviews, studies including immunological processes and further not skeletal muscle-related articles were excluded (Supplementary Figure 1).
Data Analysis
All data included in the figures of the review were transferred to PathVisio (Version 3.3.0, Department of Bioinformatics, Maastricht University, Netherlands) for creating molecular interactions and pathways. All pathways are published online for free on WikiPathways.
Results
Hypoxia Keeps Satellite Cells in Their Undifferentiated State
Hypoxia regulates the identity and progression of satellite cells by regulating gene expression through NOTCH (Gustafsson et al., 2005) and WNT (Majmundar et al., 2015) signaling which we will discuss now.
Under normoxia, microRNA1 and 206 (miR1 & miR206) downregulate PAX7 protein production leading to reduced self-renewal of the satellite cells (Chen et al., 2010; Dey et al., 2011), hypoxia allows PAX7 to regulate asymmetric self-renewal division of satellite cells, by reducing miR1 & miR206 expression. Thereby, hypoxia ensure a sufficiently large stem cell pool without affecting the overall proliferation (Liu et al., 2012). This mainly occurs due to activation of NOTCH and non-canonical WNT signaling pathways (Figure 1).
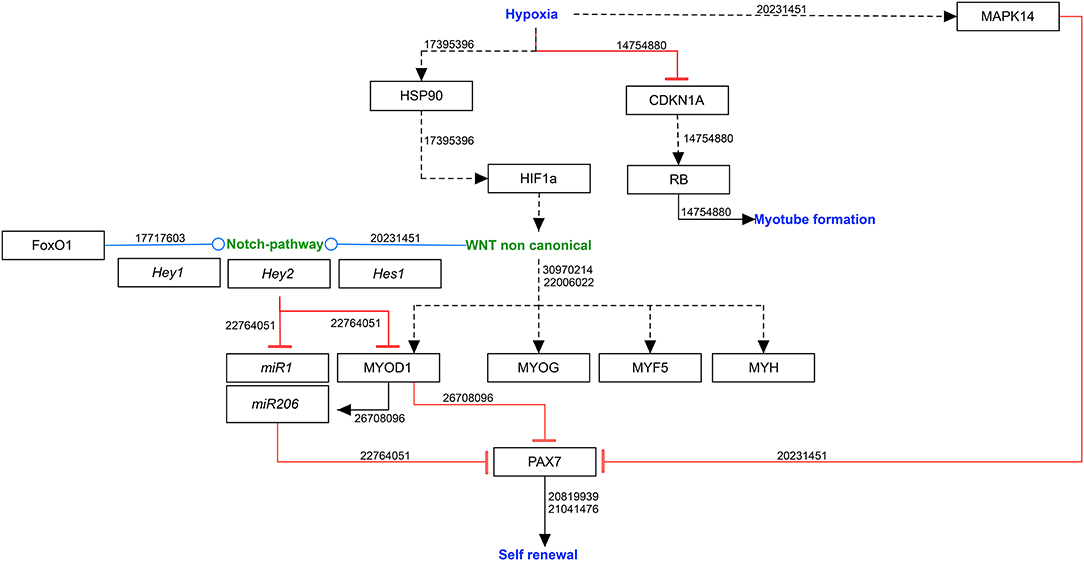
Figure 1. Molecular mechanisms involved in self-renewal of satellite cells in hypoxia. Black arrows: activation of the signaling pathway/protein/molecule. Blunt red arrow: inhibition of the signaling pathway/protein/molecule. Round blue arrow: interaction between two pathways. Numbers: PubMed-IDs. https://www.wikipathways.org/index.php/Pathway:WP5023.
NOTCH Signaling Pathway
The NOTCH signaling pathway is not only activated during embryonic muscle development to promote cell division, but also contributes to regeneration during muscle injury, which is associated with hypoxia as blood vessels are damaged. Gustafsson et al. reported a downregulation of myogenic differentiation in hypoxia through the activation of NOTCH signaling pathway, leading to an upregulation of HEY2 (hes related family bHLH transcription factor with YRPW motif 2) and therefore maintaining the myogenic cells in their undifferentiated state (Gustafsson et al., 2005). Acute hypoxia promotes the interaction between FOXO1 (forkhead box O1 transcription factor) and the NOTCH pathway upregulating the expression of NOTCH downstream transcription factor genes Hes1 (hes family bHLH transcription factor 1) and Hey1 (Kitamura et al., 2007). Furthermore, hypoxia promotes the division of primary murine myoblasts by a NOTCH-dependent upregulation of Hes1/Hey1, which in turn lead to a suppression of miR1 and miR206 in a MyoD1-independent manner (Liu et al., 2012).
Non-canonical WNT Signaling Pathway
The canonical and non-canonical WNT signaling pathways are major regulators of myogenesis (Chen et al., 2005; Borello et al., 2006). Of these, mainly the non-canonical WNT mediates the cellular response to hypoxia (Cirillo et al., 2017). While canonical WNT signaling mediates satellite cell division, non-canonical WNT signaling regulates the symmetric expansion of satellite cells and myofiber growth, resulting in hypertrophy (von Maltzahn et al., 2011). Low oxygen levels trigger non-canonical WNT signaling through at least two mechanisms: via protein kinase C (PKC), which activates calcium/calmodulin-dependent protein kinase II (CAMKII) and the RHOA/JNK (Ras homolog family member A/c-Jun N-terminal kinases) pathways, which leads to ATF/CREB (activating transcription factors/cAMP response element binding protein) activation (Veeman et al., 2003). Previous studies revealed Wnt7, Wnt9a and Wnt4 as important players in non-canonical pathway (Cirillo et al., 2017) and WNT4 upregulation leads to an increase of myogenin expressing cells (Leroux et al., 2010).
CDKN1A/B (p21/p27)
Although the main effect of non-canonical WNT signaling is the inhibition of differentiation, differentiating cells need to withdraw from the cell cycle, which is regulated by cyclin-dependent kinases (CDKs) and their inhibitors (CDKIs).
Cyclin-dependent kinase inhibitor 1 (CDKN1A, also known as p21) is a major target of TP53 and regulates cell cycle progression by inhibiting cyclin/CDK complexes (Di Carlo et al., 2004). Additionally, it leads to the accumulation of the tumor suppressor retinoblastoma protein (RB), which is necessary to establish a permanent post-mitotic state in myogenesis (Schneider et al., 1994; Novitch et al., 1996). In severe hypoxia (<1% oxygen), however, the CDKN1A as well as the RB accumulation is inhibited (Di Carlo et al., 2004).
Effects of Hypoxia on Proliferation of Skeletal Muscle Cells
Hypoxia with 2% oxygen stimulates the proliferation of mouse satellite cells (Urbani et al., 2012) and human primary myoblasts (Koning et al., 2011) in vitro by increasing the expression of the myogenic transcription factors MYF5 and MYOD1 (Koning et al., 2011).
Through induction of muscle-specific gene expression, quiescent satellite cells start to proliferate and subsequently differentiate into myotubes. The main muscle regulatory transcription factors (MRFs) are MYOD1, myogenic factor 5 (MYF5), myogenin (MYOG) and myogenic factor 6 (MYF6). Upon activation and proliferation, MYF5 and MYOD1 expression is upregulated in satellite cells and their expression remains high until late into differentiation (Megeney et al., 1996; Cornelison and Wold, 1997; Cooper et al., 1999).
Ogilvie et al. (2000) reported an effect of the hypoxia-induced hormone erythropoietin (EPO) on the proliferation of C2C12 cells and primary satellite cells. They observed that EPO could stimulate the proliferation of myoblasts and inhibit their differentiation by inducing Myf5 and MyoD1 expression (Ogilvie et al., 2000). Similar results were reported by Jazwa et al. who observed an influence of the hypoxia-regulated HMOX1 (heme oxygenase 1) on MRFs by increasing expression of MyoD1 and MyoG under ischemic conditions (Kozakowska et al., 2012; Jazwa et al., 2013). Jia et al. reported that EPO stimulates the recruitment or proliferation of satellite cells in injured muscle. Their data indicate that endogenous EPO may promote satellite cell survival in skeletal muscles by an increased expression of phosphatidylinositol 3 kinase (PI3K)-AKT-associated proteins (Jia et al., 2012).
In addition, GATA transcription factors can regulate differentiation, growth, and survival of a wide range of cell types (Molkentin, 2000). In myoblasts, GATA3 overexpression increases proliferation of undifferentiated C2C12 cells and can contribute to the proliferative response of EPO (Jia et al., 2012). In addition, it has been shown that the upregulated EPO in hypoxic conditions induces GATA3 and enables the activation of JAK2/STAT5a (Janus kinase 2/signal transducer and activator of transcription 5A) pathways (Jia et al., 2012), which is upregulated during early myogenic differentiation in vitro (Wang et al., 2008) (Figure 2).
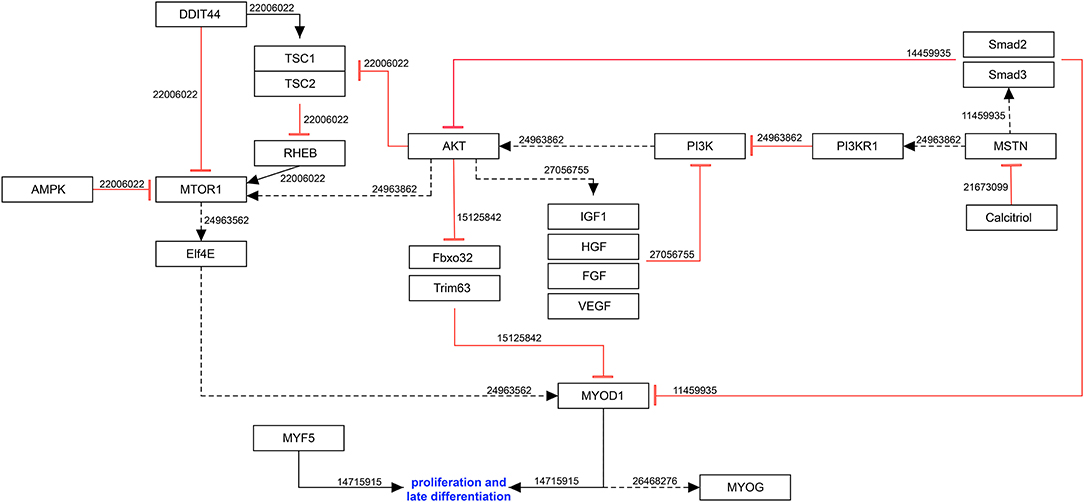
Figure 2. Molecular mechanisms involved in myogenic proliferation during hypoxia. Black arrow: activation of the signaling pathway/protein/molecule. Blunt red arrow: inhibition of the signaling pathway/protein/molecule. Numbers: PubMed-IDs. https://www.wikipathways.org/index.php/Pathway:WP5024.
Negative Feedback-Loop of Proliferation Controls Muscle Growth and Differentiation
Hypoxia also interferes with the negative feedback-loop of proliferation. For example, hypoxia causes myoblasts to release the transforming growth factor-β superfamily member myostatin (MSTN) (Hayot et al., 2011), which inhibits proliferation and enhances muscle growth by reducing protein degradation (Hauerslev et al., 2014).
Upregulation of MSTN leads to a negative loop which decreases the expression of MYOD1 (Langley et al., 2002) by binding to the ActRIIb (activin receptor type-2B) and activating SMAD2 (SMAD family member 2), which directly inhibits MYOD1 through AKT signaling inhibition (Lee and McPherron, 2001). During proliferation, AKT regulates the translation of MYOD1 (Hauerslev et al., 2014) by preventing the degradation of MYOD1 in FBXO32 (F-box protein 32) and TRIM63 (tripartite motif containing 63-dependent manner (Stitt et al., 2004). Altogether, MSTN reflects an oxygen-dependent key molecule in the regulation of myogenesis, especially during proliferation and differentiation (Rios et al., 2002).
HDAC9 Suppresses Myoblast Differentiation in Hypoxia
A recent study reported an increased expression of histone deacetylases (HDACs) including Hdac1, Hdac5, Hdac8 and Hdac9 in C2C12 cells after exposure to hypoxia (Zhang et al., 2019). The study mainly focused on HDAC9 and reported enhanced myogenesis and upregulation of MyoG and MyoD1 by Hdac9 inhibition. Additionally, the HDAC proteins were linked to autophagy by demonstrating a hypoxia-dependent enrichment of HDAC9 binding in the promoter region of autophagy-related genes Atg7, Becn1, Map1lc3a, and Map1lc3b (Zhang et al., 2019). Autophagy results in phosphorylation of GSK3ß (glycogen synthase kinase 3 beta) and was shown to directly regulate the canonical WNT pathway. Under hypoxia, phosphorylated GSK3ß and activated ß-catenin are both decreased and thus, myogenesis is impaired. These results imply that canonical Wnt signaling is negatively regulated by HDAC9 activation (Zhang et al., 2019).
PI3K-AKT Signaling Pathway Repression at Low Oxygen Levels Impedes Myoblast Differentiation
The PI3K-AKT-mTOR pathway, which participates in myogenic differentiation is downregulated due to oxygen deprivation (Ren et al., 2010). As described earlier, the PI3K-AKT signaling pathway prevents MYOD1 degradation and stimulates MYOD1 translation due to mTOR1 activation. Once the oxygen level drops, it stimulates the eukaryotic translation initiation factor 4E (ELF4E), which is normally bond to its repressor: the 4E-binding protein (Hauerslev et al., 2014). MTOR (mammalian target of rapamycin) depends on RHEB (Ras homolog enriched in brain) activation (Li et al., 2007). This Ras-related small GTPase is regulated within the cell by tumor-suppressant proteins that form the tuberous sclerosis complex (TSC) (Li et al., 2007). Under physiological condition, those complexes are inhibited by AKT, but when cells are exposed to stress or hypoxia, AMPK (AMP-activated protein kinase) and DDIT4 (DNA-damage-inducible transcript 4) pathways are activated, which inhibit RHEB (Wouters and Koritzinsky, 2008).
The PI3K-AKT signaling pathway is partially down regulated due to a reduced IGF-IR (IGF-I receptor) sensitivity at low oxygen levels (Majmundar et al., 2012).
Hypoxia, ROS- and Oxygen-Dependent microRNAs Regulation
The expression of small non-coding microRNAs (miRs), post-transcriptional regulators, can be ROS- as well as hypoxia - and oxygen-dependent. Accordingly, miR210 can also modulate the generation of reactive oxygen species (ROS) (Cicchillitti et al., 2012).
In myogenesis, eight miRs are currently known to be muscle tissue-specific and involved in myogenic processes: miR1, miR206, miR133A/B, miR208A/B, miR486, miR499 (Horak et al., 2016), miR26A (Lee et al., 2015) and miR210 (Cicchillitti et al., 2012), which are all controlled by MRFs. For example, miR1 and miR206, as described earlier, target PAX3 and PAX7 mRNA, respectively, and are both activated by MYOD1 (Horak et al., 2016). Together with miR133, mirR1 is also suggested to be required for proper somitogenesis (Chen et al., 2006). Additionally, there was the realization that some miRNAs are expressed tissue specific. Heart and skeletal muscle specific miRNA are called myomiR, including miR1, miR133 (Sempere et al., 2004), miR208A/B, miR499 and miR486 (van Rooij et al., 2007, 2009; Small et al., 2010). MiR206 holds a special role, since it is exclusively expressed in skeletal muscle cells (McCarthy et al., 2007). Moreover, changes in miR208b and miR499 expression during muscle atrophy showed a role in skeletal muscle plasticity (McCarthy et al., 2009).
One of the major targets activated by VEGF (vascular endothelial growth factor) during hypoxia is miR210. Even though miR210 has no influence on myotube number or fusion, it provides myotube survival in presence of mitochondrial dysfunction and oxidative stress by modifying mitochondrial metabolism through the modulation of ROS regeneration and direct repression of apoptosis-related genes such as CASP8AP2 (CASP8-associated protein 2) (Kim et al., 2009).
Effects of Hypoxia on Myogenic Differentiation
Hypoxia inhibits the differentiation of myoblasts. In contrast to self-renewal and proliferation, myogenic differentiation is divided into early and late phases, which are partly represented by different active molecules. During early differentiation, MYF5 and MYOD1 function as active regulatory muscle transcription factors. MYOD1 removes cells from the cell cycle and enhances the transcription of MYOG, which dominates the late differentiation, together with MRF4 (Zhou and Bornemann, 2001).
In 2004, Di Carlo et al. investigated the effect of severe hypoxia (<1% O2) on myogenic differentiation of C2C12 myoblasts. They observed growth arrest, and inhibition of MRFs expression and myogenic differentiation, due to decreased RB, CDKN1A, MYOD1, MYOG and MYHC1 (myosin heavy chain 1) and increased CDKN1B (Di Carlo et al., 2004). While hypoxic cultured myoblasts showed significantly impaired proliferation and differentiation (Di Carlo et al., 2004), Cirillo et al. described an upregulation of various MRFs due to hypoxic preconditioning of myogenic cells. Preconditioning induced a decrease of MYOD1 inhibition through downregulation of ID1 (inhibitor of differentiation/DNA binding 1) and MYOR (myogenic repressor) (Cirillo et al., 2017). The mitogen-activated protein kinase family member 14 (MAPK14) is involved in the terminal differentiation of myoblasts by activating transcription factors, such as MYOD1 and the myocyte enhancer factor-2 (MEF2). Hypoxia induces a decrease of p38-mediated phosphorylation in differentiating myoblasts, thus contributing to the initialization of the switch from differentiation to proliferation (Ren et al., 2010).
Epigenetic Regulation of Myogenic Cell Differentiation in Hypoxia
Recently, it was discovered that the histone demethylase KDM6A (lysine demethylase 6A) prevents cell differentiation by demethylation of H3K27 (histone H3 on lysine 27). Hypoxia can induce H3K27 methylation, which represses the transcription of late myogenic genes such as MyoG. The differential oxygen affinities of KDM6A and KDM6B suggests that the ability to promote H3K27 methylation and block differentiation is caused specifically by a loss of KDM6A activity within hypoxia. Indeed, the down-regulation of KDM6A, but not KDM6B, with different short hairpin RNAs phenocopied the effects of hypoxia on differentiation. Therefore, Abhishek et al. tried to directly increase KDM6A's oxygen affinity. By comparing the catalytic JmjC domains of KDM6A and KDM6B, they noted two non-conserved residues M1190 (KDM6A) → T1434 (KDM6B) and E1335 (KDM6A) → D1579 (KDM6B). A variant of KDM6A harboring these two KDM6B-like changes showed a 2-fold increased affinity of oxygen in vivo and was superior to the native KDM6A at rescuing differentiation under hypoxic conditions (Chakraborty et al., 2019).
Hypoxia-Induced Factor 1 and 2 Alpha
Hypoxia-inducible factor 1 alpha (HIF1A) is the most studied transcriptional regulator of the cellular and developmental response to hypoxia. For this reason, the Nobel Prize in Physiology and Medicine 2019 was awarded to the tree physician scientists who discovered and characterized HIF1, i.e., William G. Kaelin, Jr., Peter Ratcliffe and Gregg Semenza. Collectively, they have demonstrated that the response of gene expression to oxygen availability is directly coupled to oxygen levels in the animal cell, allowing immediate cellular responses to occur for oxygenation through the action of the HIF1 transcription factor complex.
The heterodimeric HIF1 transcription factor is under control of the oxygen-activated prolyl hydroxylase domain containing enzymes (PHD1, PHD2, PHD3), which act as oxygen sensors. The most common described prolyl hydroxylase is PHD2 (gene symbol EGLN1). In the presence of oxygen, the PHD-mediated prolyl hydroxylation leads to an ubiquitination and subsequent proteasomal degradation of HIF1A (Marxsen et al., 2004). During oxygen deprivation, the alpha-subunit is stabilized by the heat shock protein (HSP) HSP90 to prevent degradation (Kubis et al., 2005; Ono et al., 2006). Once stabilized, the alpha-subunit translocates to the nucleus and forms a heterodimer with its beta subunit. The newly formed complex bind to hypoxia response elements (HREs) in the promoter region of target genes, such as: genes to increase oxygen capacity in the blood (EPO, HMOX1), proangiogenic genes (VEGF, ADM) and metabolic genes associated with glycolysis and glucose uptake (Bracken et al., 2003).
Moreover, HIF1A activation, as well as hypoxia itself, induce BHLHE40 (Class E basic helix-loop-helix protein 40), a protein that is part of the TP53 signaling pathway. It was shown to repress myogenic differentiation by binding to the E-box sequence of the MyoG promoter, leading to reduced transcriptional activity of MyoD1 on MyoG (Wang et al., 2015). Moreover, HIF1A directly inhibits the canonical WNT signaling pathway and various MRFs, such as MYF5, MYOD1, MYOG, MRF6 and MYH (myosin heavy chain), leading to a repression of myogenic proliferation and differentiation (Sinha et al., 2019).
Another important member of the hypoxia-inducible transcription factors is HIF2A. Contrary to HIF1A, which is mainly expressed in myoblasts and regulates proliferation in hypoxic conditions, HIF2A is mainly expressed in quiescent SCs. Xie et al. did not detect HIF1A in PAX7+ quiescent satellite cells, whereas over 90% of these cells were HIF2A positive (Xie et al., 2018). Its major roles include enhancing the slow myofiber formation through acting downstream of peroxisome proliferator-activated receptor-γ coactivator 1α (PGC-1α) (Rasbach et al., 2010) and promoting stemness of satellite cell (Liu et al., 2012; Majmundar et al., 2012). Leukemia inhibitor factor (LIF) stimulates myoblast growth and fusion in vivo, acting directly on the side of injury and stimulating hypertrophy (Barnard et al., 1994; White et al., 2001). In addition, it was shown that HIF2A can directly activate the expression of LIF in colorectal cancer (Wu et al., 2015). However, it is not clear, if the mechanism through HIF2A activation also occurs in skeletal muscle regeneration.
Through a Pax7-specific double knockout of Hif1a and Hif2a in mice, Yang et al. could show the necessity of both transcription factors in self-renewal of satellite cells within hypoxic environments. While in normoxia a knockout of Hif1a/Hif2a did not affect muscle stem cell function, an oxygen level <1% showed decreased self-renewal and increased differentiation of myoblasts, without effects on the proliferation (Yang et al., 2017).
In skeletal muscle, both hypoxia-inducible transcription factors have a pro-angiogenetic function by inducing VEGF expression and the development of capillaries that express CD31 in vivo (Niemi et al., 2014). VEGF is not only essential for angiogenesis but also for skeletal muscle regeneration (Germani et al., 2003), even though it could have pro-fibrotic effects by inducing stress fiber formation in muscle-specific fibroblasts of dystrophic muscles (Gutpell and Hoffman, 2015).
In addition, HGF (hepatocyte growth factor) has been shown to have a major impact on myogenesis, as well as on adult regeneration. It leads to an upregulation of several signals such as the AKT signaling pathway (Rosova et al., 2008) and the CDKN1A (Hauerslev et al., 2014). Intriguingly, the promoter region of Hgf has a HRE element, and thus, binding of HIF1A leads to a downregulation of HGF in hypoxia (Flann et al., 2014) (Figure 3).
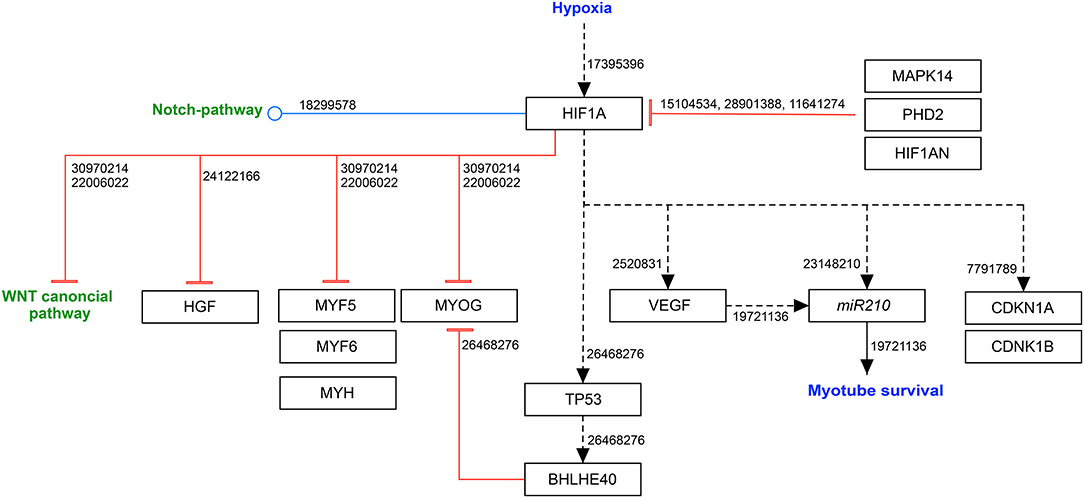
Figure 3. HIF1A modulates myogenic differentiation in hypoxia. Black arrow: activation of the signaling pathway/protein/molecule. Blunt red arrow: inhibition of the signaling pathway/protein/molecule. Round blue arrow: Interaction between two pathways. Numbers: PubMed-IDs. https://www.wikipathways.org/index.php/Pathway:WP5025.
The p38 signaling pathway has been shown to inhibit HIF1A expression. Thereby, HIMGB1 (high mobility group protein B1) is be involved in the activation of MAPK14 (p38) through RAGE (receptor for advanced glycation end-products) (Sorci et al., 2004).
Li et al. revealed an impact of estradiol (E2) on MAPK14. In a hypoxic environment, estradiol is active and binds to the estrogen receptor alpha (ERα), leading to MAPK14 phosphorylation (Li et al., 2017). MAPK14 additionally affects the activation of MYOD1 and MEF2 (myocyte enhancer factor-2) during differentiation (Keren et al., 2006).
HIF1AN (hypoxia-inducible factor 1-alpha inhibitor), an alpha-ketoglutarate-dependent dioxygenase, is the key element of negative loop regulation of HIF1A by hydroxylating the C-terminal transactivation domain of HIF1A. In hypoxia, HIF1AN is downregulated to allow HIF1A stabilization (Mahon et al., 2001). It also works as a negative regulator of NOTCH, which underlines the link between both pathways (Zheng et al., 2008).
In C2C12 cells in vitro, the inhibition of HIF1A leads to a decreased myoblast fusion, which is accompanied by a reduction of MyoG and Myh expression (Cirillo et al., 2017).
Summary and Conclusion
A reduction of oxygen levels occurs during embryogenesis and in several muscular diseases and is necessary for normal development and muscle regeneration. Cell culture studies demonstrate that myogenic differentiation is improved at oxygen levels of 2–10%. This mimics the physiological oxygen concentration in skeletal muscle tissue. Furthermore, a hypoxic preconditioning in <1% O2 has a positive effect on muscle regeneration. However, if myoblasts remain in an environment of <1% O2 over a longer period of time, it has negative and even toxic effects on myoblasts leading to apoptosis.
Generally, hypoxia prevents cell differentiation, as well as myotube growth, in muscular tissue by mainly influencing MRFs and is essential to keep satellite cells in their quiescent state. The most investigated factor that acts in low oxygen concentrations is HIF1A, which mediates the inhibition of myogenesis by involving WNT and NOTCH signaling pathways. In hypoxic environment, myogenic differentiation is additionally repressed by the inhibition of AKT/mTOR pathway as well as the induction of BHLHE40. Numerous factors and signaling pathways have proposed to modulate myogenesis during hypoxia, however this review only includes a small section, focusing onto protein and mRNA level. Metabolic and functional impacts were not discussed. Although, a deeper understanding and holistic comprehension is necessary to treat patients with extensive muscle damage associated with hypoxia.
Data Availability Statement
Publicly available datasets were analyzed in this study. This data can be found here: https://www.wikipathways.org/index.php/Pathway:WP5023; https://www.wikipathways.org/index.php/Pathway:WP5024; https://www.wikipathways.org/index.php/Pathway:WP5025.
Author Contributions
TP was part of the acquisition of the literature, was substantial contributor in developing the conception and analyzed the data. HW was substantial contributor in developing the conception and drafting of the manuscript. AA, CK, and WB substantively revised the manuscript. MS was part of the acquisition of the literature, substantially contributed to the developing the conception and drafting of the manuscript. All authors contributed to the article and approved the submitted version.
Conflict of Interest
The authors declare that the research was conducted in the absence of any commercial or financial relationships that could be construed as a potential conflict of interest.
Acknowledgments
We thank the WikiPathway team for continuous help during the creation of the pathways.
Supplementary Material
The Supplementary Material for this article can be found online at: https://www.frontiersin.org/articles/10.3389/fphys.2021.684899/full#supplementary-material
Supplementary Figure 1. PRISMA flow diagram for the systematic review.
References
Barnard, W., Bower, J., Brown, M. A., Murphy, M., and Austin, L. (1994). Leukemia inhibitory factor (LIF) infusion stimulates skeletal muscle regeneration after injury: injured muscle expresses lif mRNA. J. Neurol. Sci. 123, 108–113. doi: 10.1016/0022-510X(94)90211-9
Borello, U., Berarducci, B., Murphy, P., Bajard, L., Buffa, V., Piccolo, S., et al. (2006). The Wnt/beta-catenin pathway regulates Gli-mediated Myf5 expression during somitogenesis. Development 133, 3723–3732. doi: 10.1242/dev.02517
Bracken, C. P., Whitelaw, M. L., and Peet, D. J. (2003). The hypoxia-inducible factors: key transcriptional regulators of hypoxic responses. Cell. Mol. Life. Sci. 60, 1376–1393. doi: 10.1007/s00018-003-2370-y
Chakraborty, A. A., Laukka, T., Myllykoski, M., Ringel, A. E., Booker, M. A., Tolstorukov, M. Y., et al. (2019). Histone demethylase KDM6A directly senses oxygen to control chromatin and cell fate. Science 363, 1217–1222. doi: 10.1126/science.aaw1026
Chen, A. E., Ginty, D. D., and Fan, C. M. (2005). Protein kinase A signalling via CREB controls myogenesis induced by Wnt proteins. Nature 433, 317–322. doi: 10.1038/nature03126
Chen, J. F., Mandel, E. M., Thomson, J. M., Wu, Q., Callis, T. E., Hammond, S. M., et al. (2006). The role of microRNA-1 and microRNA-133 in skeletal muscle proliferation and differentiation. Nat. Genet. 38, 228–233. doi: 10.1038/ng1725
Chen, J. F., Tao, Y., Li, J., Deng, Z., Yan, Z., Xiao, X., et al. (2010). microRNA-1 and microRNA-206 regulate skeletal muscle satellite cell proliferation and differentiation by repressing Pax7. J. Cell Biol. 190, 867–879. doi: 10.1083/jcb.200911036
Cicchillitti, L., Di Stefano, V., Isaia, E., Crimaldi, L., Fasanaro, P., Ambrosino, V., et al. (2012). Hypoxia-inducible factor 1-alpha induces miR-210 in normoxic differentiating myoblasts. J. Biol. Chem. 287, 44761–44771. doi: 10.1074/jbc.M112.421255
Cirillo, F., Resmini, G., Ghiroldi, A., Piccoli, M., Bergante, S., Tettamanti, G., et al. (2017). Activation of the hypoxia-inducible factor 1alpha promotes myogenesis through the noncanonical Wnt pathway, leading to hypertrophic myotubes. FASEB J. 31, 2146–2156. doi: 10.1096/fj.201600878R
Cooper, R. N., Tajbakhsh, S., Mouly, V., Cossu, G., Buckingham, M., and Butler-Browne, G. S. (1999). In vivo satellite cell activation via Myf5 and MyoD in regenerating mouse skeletal muscle. J. Cell. Sci. 112 (Pt 17), 2895–2901. doi: 10.1242/jcs.112.17.2895
Cornelison, D. D., and Wold, B. J. (1997). Single-cell analysis of regulatory gene expression in quiescent and activated mouse skeletal muscle satellite cells. Dev. Biol. 191, 270–283. doi: 10.1006/dbio.1997.8721
Dey, B. K., Gagan, J., and Dutta, A. (2011). miR-206 and−486 induce myoblast differentiation by downregulating Pax7. Mol. Cell. Biol. 31, 203–214. doi: 10.1128/MCB.01009-10
Di Carlo, A., De Mori, R., Martelli, F., Pompilio, G., Capogrossi, M. C., and Germani, A. (2004). Hypoxia inhibits myogenic differentiation through accelerated MyoD degradation. J. Biol. Chem. 279, 16332–16338. doi: 10.1074/jbc.M313931200
Dunwoodie, S. L. (2009). The role of hypoxia in development of the Mammalian embryo. Dev. Cell 17, 755–773. doi: 10.1016/j.devcel.2009.11.008
Flann, K. L., Rathbone, C. R., Cole, L. C., Liu, X., Allen, R. E., and Rhoads, R. P. (2014). Hypoxia simultaneously alters satellite cell-mediated angiogenesis and hepatocyte growth factor expression. J. Cell Physiol. 229, 572–579. doi: 10.1002/jcp.24479
Germani, A., Di Carlo, A., Mangoni, A., Straino, S., Giacinti, C., Turrini, P., et al. (2003). Vascular endothelial growth factor modulates skeletal myoblast function. Am. J. Pathol. 163, 1417–1428. doi: 10.1016/S0002-9440(10)63499-2
Greenbaum, A. R., Etherington, P. J., Manek, S., O'Hare, D., Parker, K. H., Green, C. J., et al. (1997). Measurements of oxygenation and perfusion in skeletal muscle using multiple microelectrodes. J. Muscle Res. Cell Motil. 18, 149–159. doi: 10.1023/A:1018653521686
Gustafsson, M. V., Zheng, X., Pereira, T., Gradin, K., Jin, S., Lundkvist, J., et al. (2005). Hypoxia requires notch signaling to maintain the undifferentiated cell state. Dev. Cell 9, 617–628. doi: 10.1016/j.devcel.2005.09.010
Gutpell, K. M., and Hoffman, L. M. (2015). VEGF induces stress fiber formation in fibroblasts isolated from dystrophic muscle. J. Cell Commun. Signal. 9, 353–360. doi: 10.1007/s12079-015-0300-z
Hauerslev, S., Vissing, J., and Krag, T. O. (2014). Muscle atrophy reversed by growth factor activation of satellite cells in a mouse muscle atrophy model. PLoS ONE 9:e100594. doi: 10.1371/journal.pone.0100594
Hayot, M., Rodriguez, J., Vernus, B., Carnac, G., Jean, E., Allen, D., et al. (2011). Myostatin up-regulation is associated with the skeletal muscle response to hypoxic stimuli. Mol. Cell Endocrinol. 332, 38–47. doi: 10.1016/j.mce.2010.09.008
Horak, M., Novak, J., and Bienertova-Vasku, J. (2016). Muscle-specific microRNAs in skeletal muscle development. Dev. Biol. 410, 1–13. doi: 10.1016/j.ydbio.2015.12.013
Itoigawa, Y., Kishimoto, K. N., Okuno, H., Sano, H., Kaneko, K., and Itoi, E. (2010). Hypoxia induces adipogenic differentitation of myoblastic cell lines. Biochem. Biophys. Res. Commun. 399, 721–726. doi: 10.1016/j.bbrc.2010.08.007
Jazwa, A., Stepniewski, J., Zamykal, M., Jagodzinska, J., Meloni, M., Emanueli, C., et al. (2013). Pre-emptive hypoxia-regulated HO-1 gene therapy improves post-ischaemic limb perfusion and tissue regeneration in mice. Cardiovasc. Res. 97, 115–124. doi: 10.1093/cvr/cvs284
Jia, Y., Suzuki, N., Yamamoto, M., Gassmann, M., and Noguchi, C. T. (2012). Endogenous erythropoietin signaling facilitates skeletal muscle repair and recovery following pharmacologically induced damage. FASEB J. 26, 2847–2858. doi: 10.1096/fj.11-196618
Keren, A., Tamir, Y., and Bengal, E. (2006). The p38 MAPK signaling pathway: a major regulator of skeletal muscle development. Mol. Cell Endocrinol. 252, 224–230. doi: 10.1016/j.mce.2006.03.017
Kim, H. W., Haider, H. K., Jiang, S., and Ashraf, M. (2009). Ischemic preconditioning augments survival of stem cells via miR-210 expression by targeting caspase-8-associated protein 2. J. Biol. Chem. 284, 33161–33168. doi: 10.1074/jbc.M109.020925
Kitamura, T., Kitamura, Y. I., Funahashi, Y., Shawber, C. J., Castrillon, D. H., Kollipara, R., et al. (2007). A Foxo/Notch pathway controls myogenic differentiation and fiber type specification. J. Clin. Invest. 117, 2477–2485. doi: 10.1172/JCI32054
Koning, M., Werker, P. M., van Luyn, M. J., and Harmsen, M. C. (2011). Hypoxia promotes proliferation of human myogenic satellite cells: a potential benefactor in tissue engineering of skeletal muscle. Tissue Eng. Part A 17, 1747–1758. doi: 10.1089/ten.tea.2010.0624
Kozakowska, M., Ciesla, M., Stefanska, A., Skrzypek, K., Was, H., Jazwa, A., et al. (2012). Heme oxygenase-1 inhibits myoblast differentiation by targeting myomirs. Antioxid. Redox Signal. 16, 113–127. doi: 10.1089/ars.2011.3964
Kubis, H. P., Hanke, N., Scheibe, R. J., and Gros, G. (2005). Accumulation and nuclear import of HIF1 alpha during high and low oxygen concentration in skeletal muscle cells in primary culture. Biochim. Biophys. Acta 1745, 187–195. doi: 10.1016/j.bbamcr.2005.05.007
Langley, B., Thomas, M., Bishop, A., Sharma, M., Gilmour, S., and Kambadur, R. (2002). Myostatin inhibits myoblast differentiation by down-regulating MyoD expression. J. Biol. Chem. 277, 49831–49840. doi: 10.1074/jbc.M204291200
Lee, S. J., and McPherron, A. C. (2001). Regulation of myostatin activity and muscle growth. Proc. Natl. Acad. Sci. U. S. A. 98, 9306–9311. doi: 10.1073/pnas.151270098
Lee, S. W., Yang, J., Kim, S. Y., Jeong, H. K., Lee, J., Kim, W. J., et al. (2015). MicroRNA-26a induced by hypoxia targets HDAC6 in myogenic differentiation of embryonic stem cells. Nucleic Acids Res. 43, 2057–2073. doi: 10.1093/nar/gkv088
Leroux, L., Descamps, B., Tojais, N. F., Seguy, B., Oses, P., Moreau, C., et al. (2010). Hypoxia preconditioned mesenchymal stem cells improve vascular and skeletal muscle fiber regeneration after ischemia through a Wnt4-dependent pathway. Mol. Ther. 18, 1545–1552. doi: 10.1038/mt.2010.108
Li, Y., Liu, Y., Lu, Y., and Zhao, B. (2017). Inhibitory effects of 17beta-estradiol or a resveratrol dimer on hypoxia-inducible factor-1alpha in genioglossus myoblasts: involvement of ERalpha and its downstream p38 MAPK pathways. Int. J. Mol. Med. 40, 1347–1356. doi: 10.3892/ijmm.2017.3123
Li, Y., Wang, Y., Kim, E., Beemiller, P., Wang, C. Y., Swanson, J., et al. (2007). Bnip3 mediates the hypoxia-induced inhibition on mammalian target of rapamycin by interacting with Rheb. J. Biol. Chem. 282, 35803–35813. doi: 10.1074/jbc.M705231200
Liu, W., Wen, Y., Bi, P., Lai, X., Liu, X. S., Liu, X., et al. (2012). Hypoxia promotes satellite cell self-renewal and enhances the efficiency of myoblast transplantation. Development 139, 2857–2865. doi: 10.1242/dev.079665
Mahon, P. C., Hirota, K., and Semenza, G. L. (2001). FIH-1: a novel protein that interacts with HIF-1alpha and VHL to mediate repression of HIF-1 transcriptional activity. Genes Dev. 15, 2675–2686. doi: 10.1101/gad.924501
Majmundar, A. J., Lee, D. S., Skuli, N., Mesquita, R. C., Kim, M. N., Yodh, A. G., et al. (2015). HIF modulation of Wnt signaling regulates skeletal myogenesis in vivo. Development 142, 2405–2412. doi: 10.1242/dev.123026
Majmundar, A. J., Skuli, N., Mesquita, R. C., Kim, M. N., Yodh, A. G. M., et al. (2012). O(2)regulates skeletal muscle progenitor differentiation through phosphatidylinositol 3-kinase/AKT signaling. Mol. Cell Biol. 32, 36–49. doi: 10.1128/MCB.05857-11
Marxsen, J. H., Stengel, P., Doege, K., Heikkinen, P., Jokilehto, T., Wagner, T., et al. (2004). Hypoxia-inducible factor-1 (HIF-1) promotes its degradation by induction of HIF-alpha-prolyl-4-hydroxylases. Biochem. J. 381 (Pt 3), 761–767. doi: 10.1042/BJ20040620
McCarthy, J. J., Esser, K. A., and Andrade, F. H. (2007). MicroRNA-206 is overexpressed in the diaphragm but not the hindlimb muscle of mdx mouse. Am. J. Physiol. Cell Physiol. 293, C451–C457. doi: 10.1152/ajpcell.00077.2007
McCarthy, J. J., Esser, K. A., Peterson, C. A., and Dupont-Versteegden, E. E. (2009). Evidence of MyomiR network regulation of beta-myosin heavy chain gene expression during skeletal muscle atrophy. Physiol. Genom. 39, 219–226. doi: 10.1152/physiolgenomics.00042.2009
Megeney, L. A., Kablar, B., Garrett, K., Anderson, J. E., and Rudnicki, M. A. (1996). MyoD is required for myogenic stem cell function in adult skeletal muscle. Genes Dev. 10, 1173–1183. doi: 10.1101/gad.10.10.1173
Molkentin, J. D. (2000). The zinc finger-containing transcription factors GATA-4,−5, and−6. Ubiquitously expressed regulators of tissue-specific gene expression. J. Biol. Chem. 275, 38949–38952. doi: 10.1074/jbc.R000029200
Niemi, H., Honkonen, K., Korpisalo, P., Huusko, J., Kansanen, E., Merentie, M., et al. (2014). HIF-1alpha and HIF-2alpha induce angiogenesis and improve muscle energy recovery. Eur. J. Clin. Invest. 44, 989–999. doi: 10.1111/eci.12333
Norgren, L., Hiatt, W. R., Dormandy, J. A., Nehler, M. R., Harris, K. A., and Fowkes, F. G. (2007). Inter-Society Consensus for the Management of Peripheral Arterial Disease (TASC II). J. Vasc. Surg. 45(Suppl. S), S5–S67. doi: 10.1016/j.jvs.2006.12.037
Novitch, B. G., Mulligan, G. J., Jacks, T., and Lassar, A. B. (1996). Skeletal muscle cells lacking the retinoblastoma protein display defects in muscle gene expression and accumulate in S and G2 phases of the cell cycle. J. Cell Biol. 135, 441–456. doi: 10.1083/jcb.135.2.441
Ogilvie, M., Yu, X., Nicolas-Metral, V., Pulido, S. M., Liu, C., Ruegg, U. T., et al. (2000). Erythropoietin stimulates proliferation and interferes with differentiation of myoblasts. J. Biol. Chem. 275, 39754–39761. doi: 10.1074/jbc.M004999200
Ono, Y., Sensui, H., Sakamoto, Y., and Nagatomi, R. (2006). Knockdown of hypoxia-inducible factor-1alpha by siRNA inhibits C2C12 myoblast differentiation. J. Cell Biochem. 98, 642–649. doi: 10.1002/jcb.20804
Parmar, K., Mauch, P., Vergilio, J. A., Sackstein, R., and Down, J. D. (2007). Distribution of hematopoietic stem cells in the bone marrow according to regional hypoxia. Proc. Natl. Acad. Sci. U. S. A. 104, 5431–5436. doi: 10.1073/pnas.0701152104
Rasbach, K. A., Gupta, R. K., Ruas, J. L., Wu, J., Naseri, E., Estall, J. L., et al. (2010). PGC-1alpha regulates a HIF2alpha-dependent switch in skeletal muscle fiber types. Proc. Natl. Acad. Sci. U. S. A. 107, 21866–21871. doi: 10.1073/pnas.1016089107
Ren, H., Accili, D., and Duan, C. (2010). Hypoxia converts the myogenic action of insulin-like growth factors into mitogenic action by differentially regulating multiple signaling pathways. Proc. Natl. Acad. Sci. U. S. A. 107, 5857–5862. doi: 10.1073/pnas.0909570107
Rios, R., Carneiro, I., Arce, V. M., and Devesa, J. (2002). Myostatin is an inhibitor of myogenic differentiation. Am. J. Physiol. Cell Physiol. 282, C993–C999. doi: 10.1152/ajpcell.00372.2001
Rosova, I., Dao, M., Capoccia, B., Link, D., and Nolta, J. A. (2008). Hypoxic preconditioning results in increased motility and improved therapeutic potential of human mesenchymal stem cells. Stem Cells 26, 2173–2182. doi: 10.1634/stemcells.2007-1104
Schneider, J. W., Gu, W., Zhu, L., Mahdavi, V., and Nadal-Ginard, B. (1994). Reversal of terminal differentiation mediated by p107 in Rb-/- muscle cells. Science 264, 1467–1471. doi: 10.1126/science.8197461
Sempere, L. F., Freemantle, S., Pitha-Rowe, I., Moss, E., Dmitrovsky, E., and Ambros, V. (2004). Expression profiling of mammalian microRNAs uncovers a subset of brain-expressed microRNAs with possible roles in murine and human neuronal differentiation. Genome Biol. 5:R13. doi: 10.1186/gb-2004-5-3-r13
Sinha, K. M., Tseng, C., Guo, P., Lu, A., Pan, H., Gao, X., et al. (2019). Hypoxia-inducible factor 1alpha (HIF-1alpha) is a major determinant in the enhanced function of muscle-derived progenitors from MRL/MpJ mice. FASEB J. 33:fj201801794R. doi: 10.1096/fj.201801794R
Sliwicka, E., Cison, T., Kasprzak, Z., Nowak, A., and Pilaczynska-Szczesniak, L. (2017). Serum irisin and myostatin levels after 2 weeks of high-altitude climbing. PLoS ONE 12:e0181259. doi: 10.1371/journal.pone.0181259
Small, E. M., O'Rourke, J. R., Moresi, V., Sutherland, L. B., McAnally, J., Gerard, R. D., et al. (2010). Regulation of PI3-kinase/Akt signaling by muscle-enriched microRNA-486. Proc. Natl. Acad. Sci. U. S. A. 107, 4218–4223. doi: 10.1073/pnas.1000300107
Sorci, G., Riuzzi, F., Arcuri, C., Giambanco, I., and Donato, R. (2004). Amphoterin stimulates myogenesis and counteracts the antimyogenic factors basic fibroblast growth factor and S100B via RAGE binding. Mol. Cell Biol. 24, 4880–4894. doi: 10.1128/MCB.24.11.4880-4894.2004
Stitt, T. N., Drujan, D., Clarke, B. A., Panaro, F., Timofeyva, Y., Kline, W. O., et al. (2004). The IGF-1/PI3K/Akt pathway prevents expression of muscle atrophy-induced ubiquitin ligases by inhibiting FOXO transcription factors. Mol. Cell 14, 395–403. doi: 10.1016/S1097-2765(04)00211-4
Urbani, L., Piccoli, M., Franzin, C., Pozzobon, M., and De Coppi, P. (2012). Hypoxia increases mouse satellite cell clone proliferation maintaining both in vitro and in vivo heterogeneity and myogenic potential. PLoS ONE 7:e49860. doi: 10.1371/journal.pone.0049860
van Rooij, E., Quiat, D., Johnson, B. A., Sutherland, L. B., Qi, X., Richardson, J. A., et al. (2009). A family of microRNAs encoded by myosin genes governs myosin expression and muscle performance. Dev. Cell 17, 662–673. doi: 10.1016/j.devcel.2009.10.013
van Rooij, E., Sutherland, L. B., Qi, X., Richardson, J. A., Hill, J., and Olson, E. N. (2007). Control of stress-dependent cardiac growth and gene expression by a microRNA. Science 316, 575–579. doi: 10.1126/science.1139089
Veeman, M. T., Axelrod, J. D., and Moon, R. T. (2003). A second canon. Functions and mechanisms of beta-catenin-independent Wnt signaling. Dev. Cell 5, 367–377. doi: 10.1016/S1534-5807(03)00266-1
von Maltzahn, J., Bentzinger, C. F., and Rudnicki, M. A. (2011). Wnt7a-Fzd7 signalling directly activates the Akt/mTOR anabolic growth pathway in skeletal muscle. Nat. Cell Biol. 14, 186–191. doi: 10.1038/ncb2404
Wang, C., Liu, W., Liu, Z., Chen, L., Liu, X., and Kuang, S. (2015). Hypoxia inhibits myogenic differentiation through p53 protein-dependent induction of Bhlhe40 Protein. J. Biol. Chem. 290, 29707–29716. doi: 10.1074/jbc.M115.688671
Wang, K., Wang, C., Xiao, F., Wang, H., and Wu, Z. (2008). JAK2/STAT2/STAT3 are required for myogenic differentiation. J. Biol. Chem. 283, 34029–34036. doi: 10.1074/jbc.M803012200
White, J. D., Davies, M., and Grounds, M. D. (2001). Leukaemia inhibitory factor increases myoblast replication and survival and affects extracellular matrix production: combined in vivo and in vitro studies in post-natal skeletal muscle. Cell Tissue Res. 306, 129–141. doi: 10.1007/s004410100432
Wouters, B. G., and Koritzinsky, M. (2008). Hypoxia signalling through mTOR and the unfolded protein response in cancer. Nat. Rev. Cancer 8, 851–864. doi: 10.1038/nrc2501
Wu, L., Yu, H., Zhao, Y., Zhang, C., Wang, J., Yue, X., et al. (2015). HIF-2α mediates hypoxia-induced LIF expression in human colorectal cancer cells. Oncotarget 6, 4406–4417. doi: 10.18632/oncotarget.3017
Xie, L., Yin, A., Nichenko, A. S., Beedle, A. M., Call, J. A., and Yin, H. (2018). Transient HIF2A inhibition promotes satellite cell proliferation and muscle regeneration. J. Clin. Invest. 128, 2339–2355. doi: 10.1172/JCI96208
Yang, X., Yang, S., Wang, C., and Kuang, S. (2017). The hypoxia-inducible factors HIF1alpha and HIF2alpha are dispensable for embryonic muscle development but essential for postnatal muscle regeneration. J. Biol. Chem. 292, 5981–5991. doi: 10.1074/jbc.M116.756312
Yun, Z., Lin, Q., and Giaccia, A. J. (2005). Adaptive myogenesis under hypoxia. Mol. Cell Biol. 25, 3040–3055. doi: 10.1128/MCB.25.8.3040-3055.2005
Zammit, P. S., Golding, J. P., Nagata, Y., Hudon, V., Partridge, T. A., and Beauchamp, J. R. (2004). Muscle satellite cells adopt divergent fates: a mechanism for self-renewal? J. Cell Biol. 166, 347–357. doi: 10.1083/jcb.200312007
Zhang, Z., Zhang, L., Zhou, Y., Li, L., Zhao, J., Qin, W., et al. (2019). Increase in HDAC9 suppresses myoblast differentiation via epigenetic regulation of autophagy in hypoxia. Cell Death Dis. 10:552. doi: 10.1038/s41419-019-1763-2
Zheng, X., Linke, S., Dias, J. M., Zheng, X., Gradin, K., Wallis, T. P., et al. (2008). Interaction with factor inhibiting HIF-1 defines an additional mode of cross-coupling between the Notch and hypoxia signaling pathways. Proc. Natl. Acad. Sci. U. S. A. 105, 3368–3373. doi: 10.1073/pnas.0711591105
Keywords: hypoxia-inducible factor 1 alpha, HIF1A, muscle regeneration, satellite cells, fusion
Citation: Pircher T, Wackerhage H, Aszodi A, Kammerlander C, Böcker W and Saller MM (2021) Hypoxic Signaling in Skeletal Muscle Maintenance and Regeneration: A Systematic Review. Front. Physiol. 12:684899. doi: 10.3389/fphys.2021.684899
Received: 24 March 2021; Accepted: 26 May 2021;
Published: 23 June 2021.
Edited by:
Giorgos K. Sakkas, University of Thessaly, GreeceReviewed by:
Feng Yue, Purdue University, United StatesFrancesca Cencetti, University of Florence, Italy
Copyright © 2021 Pircher, Wackerhage, Aszodi, Kammerlander, Böcker and Saller. This is an open-access article distributed under the terms of the Creative Commons Attribution License (CC BY). The use, distribution or reproduction in other forums is permitted, provided the original author(s) and the copyright owner(s) are credited and that the original publication in this journal is cited, in accordance with accepted academic practice. No use, distribution or reproduction is permitted which does not comply with these terms.
*Correspondence: Maximilian Michael Saller, bWF4aW1pbGlhbi5zYWxsZXImI3gwMDA0MDttZWQudW5pLW11ZW5jaGVuLmRl