- 1Centre for Lipid Research, Key Laboratory of Molecular Biology for Infectious Diseases, Ministry of Education, Department of Infectious Diseases, Institute for Viral Hepatitis, The Second Affiliated Hospital, Chongqing Medical University, Chongqing, China
- 2National Clinical Research Center for Aging and Medicine, Huashan Hospital, Fudan University, Shanghai, China
- 3John Moorhead Research Laboratory, Centre for Nephrology, University College London Medical School, Royal Free Campus, University College London, London, United Kingdom
Dietary lipids absorbed in the intestine are closely related to the development of metabolic syndrome. CD36 is a multi-functional scavenger receptor with multiple ligands, which plays important roles in developing hyperlipidemia, insulin resistance, and metabolic syndrome. In the intestine, CD36 is abundant on the brush border membrane of the enterocytes mainly localized in proximal intestine. This review recapitulates the update and current advances on the importance of intestinal CD36 in sensing dietary lipids and regulating intestinal lipids uptake, synthesis and transport, and regulating intestinal hormones secretion. However, further studies are still needed to demonstrate the complex interactions between intestinal CD36 and dietary lipids, as well as its importance in diet associated metabolic syndrome.
Introduction
Diet associated metabolic syndrome (MetS) has become a global public health problem. MetS is a cluster of metabolic disorders that include abdominal obesity, dyslipidemia, hypertension, elevated blood glucose, and liver steatosis. The MetS greatly increases the risk of heart disease, stroke, diabetes, non-alcoholic fatty liver disease (NAFLD), and all-cause mortality. A positive correlation has been described between the risk of MetS and dietary lipid content. Since about 95% of dietary lipids are absorbed in the small intestine, the small intestine could play an essential role in MetS etiology.
CD36, a highly glycosylated transmembrane protein also known as fatty acid (FA) translocase (FAT), platelet GPIV, GP88, and scavenger receptor class B type 2 (SR-B2), is a multi-functional scavenger receptor with multiple ligands (Chen et al., 2019).
In humans, variants in the CD36 gene have been associated with lipid and glucose metabolism abnormality and altered susceptibility to metabolic syndrome and diabetes-associated coronary disease (Love-Gregory et al., 2016). Moreover, CD36 deficient is the only genetic deficiency state among scavenger receptors in humans. Since a patient with CD36 deficiency was found in Japan in the 1990s, numerous studies have reported that the patients with CD36 deficiency had the typical metabolic features of MetS, such as dyslipidemia, including postprandial hypertriglyceridemia, insulin resistance, and hypertension (Love-Gregory and Abumrad, 2011). These phenotypes have also been observed in CD36 knockout (CD36KO) mice (Shu et al., 2020). In addition, one strain of spontaneously hypertensive rats lacks CD36 and shows metabolic phenotypes of insulin resistance and high free fatty acid (FFA) levels, which are ameliorated by the transgenic overexpression of CD36 (Aitman et al., 1999; Pravenec et al., 2001). Here we will review recent findings on the importance of CD36 in sensing dietary lipids and regulating lipids uptake, synthesis, and transport in the intestine.
CD36 Gene, Structure, Function and Distribution
The human CD36 gene is located on the long arm of chromosome 7 (7q21.11). The gene is ∼46 kb long and includes 17 exons and 18 introns. At least 23 alternative transcripts are known for CD36, yielding different transcripts in different tissue types (Pietka et al., 2014). There are four protein isoforms generated by alternative splicing of the CD36 gene1.
Human CD36 contains ∼472 amino acids, which contains two transmembrane domains, a large extracellular region containing ligand-binding sites, and a short cytoplasmic tail at the N-terminal and C-terminal. The extracellular domain of CD36 forms two hydrophobic cavities that mediate the uptake of hydrophobic molecules such as FA, cholesterol, and phospholipids. CD36 also harbors a CD36, LIMP-2, Emp sequence homologous (CLESH) domain that can interact with thrombospond-1 (TSP1) repeat 2 (TSR2) domain of TSP1 (Klenotic et al., 2013). Moreover, in CD36, the lysine-cluster region can bind to negatively charged ligands, such as oxidized low-density lipoprotein (ox-LDL), apoptotic cells, and advanced oxidation protein products (AOPPs). With the interaction with these multiple ligands, CD36 plays essential roles in regulating lipids metabolism, angiogenesis, adhesion, apoptosis and inflammation/immune response (Zhao et al., 2018a).
CD36 undergoes multiple post-translational modifications, including phosphorylation, glycosylation, palmitoylation, acetylation, ubiquitylation, and disulfide bonding. These modifications control CD36 expression, maturation and subcellular localization in cells (Luiken et al., 2016).
CD36 is expressed in multiple cell types, including platelets, monocytes/macrophages, intestinal epithelial cells, microvascular endothelial cells, smooth muscle cells, adipose tissues, skeletal muscles, and cardiomyocytes. In the intestine, CD36 is highly expressed on the brush border membrane of the enterocytes mainly localized in the proximal intestine (duodenum and jejunum). It seems to be little or absent in the ileum and colon, whereas it presents in blood vessels throughout the intestine (Cifarelli et al., 2017). It is demonstrated that CD36 is important in mediating the uptake of long-chain FA (LCFA) in skeletal muscle and adipose tissues (Pietka et al., 2012). However, the role of CD36 in the intestine is more complex.
The Role of Intestinal CD36 in Sensing Dietary Lipids
CD36 Regulates Dietary Fat Intake
In humans, the CD36 gene polymorphism, which causes a decrease of CD36 protein expression, is directly associated with the capability to detect dietary FA and the preference for fat-rich diet (Keller et al., 2012; Pepino et al., 2012; Fujii et al., 2019; Bajit et al., 2020). Inhibition of CD36 on ventromedial hypothalamus (VMH) neurons leads to insensitivity of neurons to FA loadings, which may cause more food intake and body weight gain, development of fatty liver and insulin resistance in rats, suggesting CD36 is a critical molecular in both neuronal FA sensing and the regulation of metabolic homeostasis (Le Foll et al., 2015). On the taste bud cells (TBC) in the tongue, CD36 binding to dietary FA launches the signal transmission to the central nervous system producing fat taste perception and cephalic phase secretion of insulin and bile acids (Laugerette et al., 2005; El-Yassimi et al., 2008). It is also reported that the defect of CD36, both in humans and animal models decreases the release of FA-induced Ca2+ signaling and serotonin in TBC, which may promote more fat intake in these subjects (Ozdener et al., 2014). On the enterocytes of the proximal small intestine, CD36 mediates the conversion of diet-derived FA into a cellular lipid messenger oleoylethanolamide (OEA), which consequently activates peroxisome proliferator activated receptor alpha (PPARα) to prolong across-meal satiety (Schwartz et al., 2008). Accordingly, the prolonging meal interval induced by a lipid emulsion was absent in CD36 null mutants, indicating that CD36 may regulate the fat intake via modulating OEA production. Thus these findings provide evidence supporting that CD36 may act as a dietary FA sensor to regulate dietary fat intake and lipid homeostasis.
CD36 Regulates Intestinal Hormones
In addition to the absorption of dietary nutrients, the intestine is well-recognized as a virtual organ with endocrine functions. Although enteroendocrine cells (EECs) account for a small population of intestinal epithelial cells (Haber et al., 2017), they produce and release several kinds of intestinal peptides and hormones (Gribble and Reimann, 2016), including cholecystokinin (CCK) secreted by I cells, secretin by S cells, glucose-dependent insulinotropic polypeptide (GIP) by K cells and glucagon-like peptide-1 (GLP-1) by L cells. These intestinal hormones widely participate in nutrients digestion and regulation of energy balance. CCK regulates gallbladder contraction, gastrointestinal secretions, and promotes fat absorption (Kato et al., 2021). Secretin regulates the pH of the intestinal contents by stimulating water and bicarbonate secretion, and it can also synergize with CCK to induce pancreatic secretions (Afroze et al., 2013). GLP-1 and GIP are identified as incretins which enhance insulin secretion in β-cells, decrease blood glucose, and regulate nutrient absorption (Holst, 2007; Sandoval and D’Alessio, 2015; Campbell, 2020). In addition, all the peptides inhibit gastrointestinal motility and promote satiety, contributing to the regulation of fat intake (Holst, 2007; Cheng et al., 2011; Dockray, 2012; Wang et al., 2015).
CD36 has been found to be expressed on the membrane of EECs. Recent studies have demonstrated its important role in regulating the secretion of multiple intestinal hormones in response to dietary lipid loading (Lobo et al., 2001; Sundaresan et al., 2013; Little et al., 2014). CD36 regulates the release of CCK by activating cAMP/CaM-KII, and it mediates secretin release via activating cAMP-PKA pathway (Sundaresan et al., 2013). In vivo and in vitro, CD36 deficiency significantly decreases the release of CCK and secretin both in the fasted and oil-loaded state (Sundaresan et al., 2013). CD36 has also been reported to interact with incretins, including GLPs and GIP. The secretion of GLP-1 and GIP to a high-fat meal was significantly reduced in human subjects carrying CD36 rs3211938 (G/T) which decreases CD36 level by approximately 50% (Shibao et al., 2018). In the meantime, the secretion of ghrelin, a gastric peptide regulating growth and energy balance, was unchanged in rs3211938 subjects (Shibao et al., 2018), suggesting that CD36 may not regulates the secretion of ghrelin.
In turn, the intestinal peptides/hormones can regulate the expression and function of intestinal CD36 (Little et al., 2014). Secretin and CCK can act on their receptors on enterocytes to up-regulate CD36 expression, promoting intestinal lipid absorption (Sekar and Chow, 2014; Demenis et al., 2017). Consistently, the CD36 levels were reduced in secretin receptor-deficient mice (Sekar and Chow, 2014). GLP-1 inhibits the expression of CD36 via activating protein kinase A (PKA) in human macrophages and rodents’ cardiomyocytes (Dai et al., 2014; Wu et al., 2018). Interestingly, GLP-2, a product of the same gene gcg as GLP-1, promotes lipid absorption by increasing the expression of fully glycosylated CD36 in the small intestine, which involves the intestinal-epithelial insulin-like growth factor-1 receptor (Hsieh et al., 2009; Mellitzer and Gradwohl, 2011; Xiao et al., 2015; Markovic et al., 2020; Figure 1).
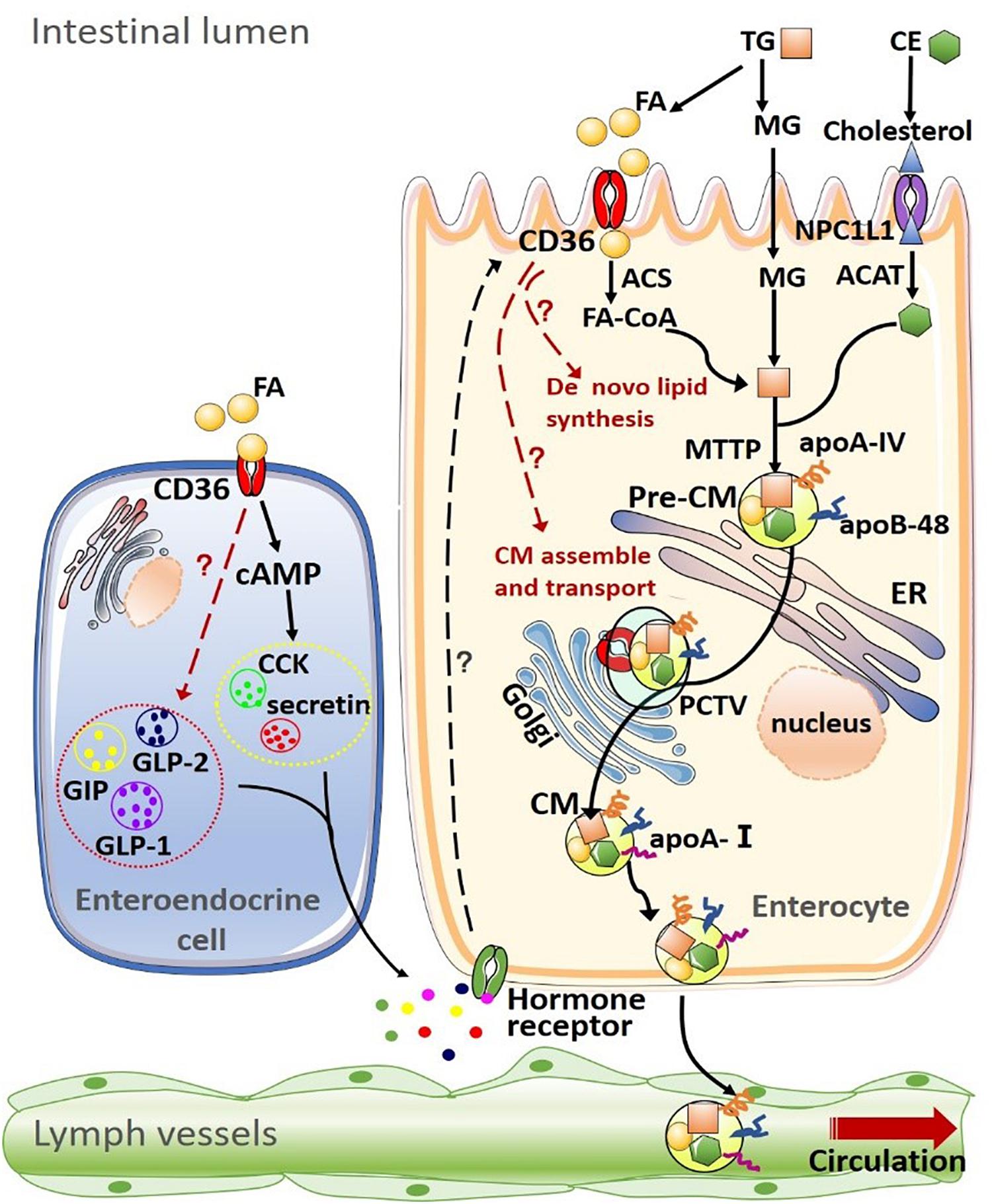
Figure 1. Summary of CD36 on lipid uptake, de novo synthesis transport and intestinal hormone in the intestine. (1) Dietary lipids, such as triglyceride (TG) and cholesteryl esters (CE) are completely digested by pancreatic enzymes in the intestinal lumen, producing fatty acids (FAs), monoacylglycerols (MAGs), cholesterol. The uptake of FAs and MAGs into the enterocyte can be driven by the concentration gradient or facilitated by other proteins such as CD36. Cholesterol uptake is mediated by Niemann-Pick C1-like 1 (NPC1L1) protein. After being taken up by enterocytes, products of lipid digestion are re-esterified by a well-coordinated group of proteins [including acyl-CoA synthetase (ACS) and acyl-CoA:cholesterol acyltransferase (ACAT)] and assembled into TG and CE. Intestinal lipids are then packaged into pre-chylomicrons (pre-CM) with apolipoprotein A-IV (apoA-IV) and apolipoprotein B-48 (apoB-48) or stored as intracellular lipid droplets. Microsomal triglyceride transfer protein (MTP) mediates the packaging of lipids into pre-CM, which are transported from the endoplasmic reticulum to the Golgi apparatus for maturation via the pre-chylomicron transport vesicle (PCTV). Mature chylomicrons (CM) are then secreted into intestinal lymph trunk and transported by the lymphatic system into the circulation. (2) CD36 on the enteroendocrine cell (EEC) mediates the secretion of multiple intestinal hormones, including cholecystokinin (CCK), secretin, glucose-dependent insulinotropic polypeptide (GIP), glucagon-like peptide-1 (GLP-1), and glucagon-like peptide-1 (GLP-2). In turn, these intestinal peptides/hormones act on their receptors on enterocytes to modulate CD36 expression and function.
The Role of CD36 in Regulating Lipids Homeostasis in the Intestine
CD36 in the Absorption of Dietary Triglycerides/FA
Lipid absorption in the intestine involves the digestion and absorption of triglycerides (TG), phospholipids, cholesterol esters, and fat-soluble vitamins. TG are the majority of dietary lipids, which account for about 90% of dietary lipids. In the lumen of duodenum, dietary TG is hydrolyzed into FA, mostly LCFA (16∼20 carbon atoms), monoglyceride (MG) and glycerol. The short-chain FA, medium-chain FA and glycerol can be directly absorbed into the portal system, whereas LCFA and very-long-chain FA need FA transporters to be absorbed and transported to the bloodstream.
CD36 is one of the important proteins involved in facilitating the absorption of LCFA. In the intestine, CD36 expression is abundant in the apical membrane of enterocytes in the duodenum and jejunum, the main sites of lipids absorption. Goudriaan et al. (2002) determined the TG absorption in CD36KO mice by administering an intragastric olive oil bolus with 3H-labeled triolein and the amount of 3H label in plasma, after blocking serum lipoprotein clearance by i.v. injections of Triton WR 1339. The plasma appearance of 3H-label in CD36KO mice is not different from that in wild-type (WT) mice. These results suggest that CD36 deficiency does not affect intestinal TG absorption after an acute lipid load. Drover et al. (2005) further monitored the TG absorption in a high-fat diet-fed mice model and showed that fecal TG levels were comparable in CD36-deficient mice and WT controls. Also, using the sucrose polybehenate method, which evaluates overall TG absorption from the ratio of fecal fat to a non-absorbable marker, it showed that no significant difference was observed between CD36KO and WT mice (Drover et al., 2005). These data indicate no global defect in overall TG absorption in CD36-deficient mice.
On the other hand, Nassir et al. (2007) isolated enterocytes from the proximal and distal small intestine of CD36KO and WT mice. Compared with WT mice, the enterocytes of the proximal intestine from CD36KO mice exhibit 50% reduction of the uptake of [3H]-oleate-BSA (C18:1). The mass spectrometry technique of shotgun lipidomics also shows that the oleic acid enrichment of mucosal lipids is delayed in the proximal intestine of CD36 KO mice, compared with WT mice, after gavage with olive oil. These data support that CD36 is important for LCFA uptake in the proximal intestine. Interestingly, in vivo, it is reported that the absorption of 14C-labeled palmitic acid is comparable between CD36KO mice and WT mice after gavage of olive oil with 14C-labeled palmitic acid (Goudriaan et al., 2002). One possibility could be that the LCFA absorption is enhanced in the distal intestine in compensation to the reduced LCFA uptake in the proximal intestine of CD36KO mice, which may explain comparable TG absorption between CD36KO and WT mice. It is also possible that other intestinal FA transporters, including fatty acid transport proteins (FATPs) and fatty acid binding proteins (FABPs) could compensate for reduced LCFA uptake in the absence of CD36 (Chassen et al., 2018). However, these hypotheses need to be validated in future works using mice models with the deficiency of these genes in the intestine.
Moreover, recent studies show that the absorption of C24:0 FA is completely abolished in intestine of CD36KO mice fed a high-fat diet (Drover et al., 2008), highlights the key role of intestinal CD36 in the absorption of fatty acids containing very long saturated acyl chains.
CD36 in the Absorption of Dietary Cholesterol
Using the fecal dual-isotope method, it is demonstrated that there is no significant difference in cholesterol absorption between CD36KO and WT mice 24-h after gavage with 14C-cholesterol and 3H-sitosterol (Nauli et al., 2006). However, when mice were intraduodenally infused with a lipid emulsion containing [3H] triolein and [14C] cholesterol, compared with WT mice, the CD36KO mice exhibited significant accumulation of recovered [14C] cholesterol in the small intestinal lumen and significant reduction of recovered [14C] cholesterol in the lymph at the end of 6-h infusion (Nauli et al., 2006). It seems that while CD36 deficiency reduced the absorption of dietary cholesterol in a short time, it does not affect global dietary cholesterol absorption for a long time.
Using primary enterocytes, it is also demonstrated that CD36 deficiency reduces cholesterol uptake (60%) in proximal intestine but not in the distal intestine (Nassir et al., 2007). Thus it is suggested that CD36 mediates the cholesterol absorption in the intestine, but its absence can be compensated by many factors, including slower gastrointestinal emptying, a longer length of small intestine and the presence of other cholesterol transporters. Consistently, it is demonstrated that CD36KO mice exhibits significantly higher levels of Niemann-Pick C1 Like 1 (NPC1L1) in the distal intestine compared with WT mice (Nassir et al., 2007). Since NPC1L1 is a key molecule to the cholesterol absorption in the intestine (Zhang et al., 2018), it is proposed that intestinal CD36 may also be involved in the regulation of cholesterol absorption via NPC1L1 pathway.
CD36 Regulates de novo Lipogenesis and Lipids Transport in the Intestine
Once FA is taken up by binding proteins/transporters at the apical surface of enterocytes, it can be converted to the corresponding acyl-CoA, which is rapidly resynthesized into TG. This resynthesized TG together with endogenous TG are packaged with apolipoproteins (apo) (apoA and apoB-48) into chylomicrons (CM) (Nauli et al., 2006) in the ER of intestinal mucosal cells. They are then transported to the basolateral side of enterocytes and secreted into the extracellular space of intestinal epithelial cells, collected by lymph. Lymph goes through thoracic duct and into left brachiocephalic vein and blood. In the blood, it gets apo C and E from high-density lipoprotein (HDL).
In patients with CD36-deficiency, TG levels in very low-density lipoprotein (VLDL) and low-density lipoprotein (LDL) fractions are higher than controls in fasting state. After oral fat loading (OFL), the levels of TG in CM, VLDL, and LDL are enhanced further in the patients with CD36 deficiency compared with controls. The TG concentrations of apoB-48-containing fractions are also higher in the patients than controls, during fasting and postprandial state (Masuda et al., 2009). These increases in TG, apoB-48, FFA, and free glycerol after OFL suggest that CD36 might be involved in the production and/or transport of lipids of intestine in response to fat loading.
In animal models, Masuda et al. (2009) showed that the TG, FFA and free glycerol levels in the intestinal lymph of CD36-KO mice were significantly increased after gavage of olive oil compared with WT mice. Interestingly, Drover et al. (2005) and Goudriaan et al. (2002) showed that the recovery of [3H]-labeled TG infused into the duodenum was reduced in the intestinal lymph of CD36KO mice compared with WT mice (Nauli et al., 2006). It is known that the intestinal TG is derived from both exogenous and endogenous sources, and the endogenously synthesized intestinal TG accounts for more than half of total lymphatic TG during the postprandial state (Shiau et al., 1985). Thus, these data suggest that while the exogenously synthesized TG derived from dietary source is decreased in the intestine of CD36KO mice, the endogenously synthesized intestinal TG is increased, contributing to an enhanced total intestinal TG production in CD36KO mice during postprandial state. The enhanced intestinal TG production may consequently cause postprandial hyperlipidemia in subjects with CD36-deficiency.
It is also reported that CD36 ablation causes lipid accumulation in the enterocytes of the upper villi of the proximal small intestine after an acute lipids load and with a HFD treatment, indicating the lipid homeostasis is disrupted in intestinal enterocytes of CD36-null mice (Drover et al., 2005; Masuda et al., 2009). Since the lipids uptake defects in the proximal intestine of CD36KO mice, the accumulated lipids in intestine of CD36-null mice may be attributed to enhanced de novo lipids synthesis and/or decreased lipids transport/secretion into the lymph.
Consistently, the mRNA level of fatty acid synthase (FAS), a key enzyme involved in the biosynthesis of FFA, is also significantly increased in the intestinal epithelium of CD36KO mice, both in the fasting state and postprandial states, when compared with WT mice. The mRNA level of stearoyl-CoA desaturase 1 (SCD1), a lipogenic enzyme in the biosynthesis of monounsaturated fatty acids [mainly oleate (C18:1) and palmitoleate (C16:1)], is increased significantly in CD36KO mice in the postprandial state (Masuda et al., 2009). On the other hand, it is suggested that CD36 is necessary for inducing the expression of apoB48, microsomal triglyceride-transfer protein (MTP) in response to LCFA loading, and the formation and maturation of pre-chylomicron (pre-CM) transport vesicle, which are key steps of CM formation/transport (Tran et al., 2011). The average size of the lymph lipoprotein particles of CD36KO mice was significantly smaller than that of WT mice in the lipid feeding state. The lymphatic apoB amounts are also lower in CD36KO mice than WT mice during both fasting and postprandial states (Masuda et al., 2009). These data support that CD36 deficiency promotes de novo lipid synthesis and blunt CM formation/transport in the intestine. However, it remains unclear exactly how CD36 might regulate intestinal de novo lipids synthesis and CM production (Figure 1).
Notably, in addition to regulating LCFA transport into the lymphatic vessel under physiologic conditions, CD36 may contribute to abnormal LCFA absorption under pathological conditions. In patients with liver cirrhosis, it is suggested that the increased expression of glycosylated CD36 in intestinal endothelial cells may promote alternative LCFA transport into blood capillary vessels, rather than into lymphatic vessels because of the backpressure of these vessels as a result of portal hypertension (Yamamoto et al., 2012).
The Regulation of Lipids on Intestinal CD36
CD36 has been identified as a receptor for various nutrients and its products, for example, advanced glycation end products (AGEs), modified-lipoproteins and LCFA. In turn, multiple nutrients and its derivatives also modulate CD36 expression and function (Shi et al., 2019).
In patients with hyperglycemia and/or hyperlipidemia, the CD36 expression is significantly increased in vascular lesions and kidneys compared with control subjects (Shu et al., 2020). In duodenal mucosa biopsies from human, the CD36 expression is also positively correlated with increasing body mass index (BMI), suggesting the dysregulation of CD36 responses to fat in obesity (Little et al., 2014). In addition, HFD significantly increased CD36 expression in the jejunum of mice (Lynes et al., 2011).
Fatty acid and its products promote CD36 transcription by activating NF-KappaB pathway or peroxisome proliferator-activated receptors (PPARs) or TR4 pathway (Xie et al., 2009; Jiang et al., 2019). The ox-LDL promote CD36 transcription by activating PPARs signaling pathway contributing to foam cell formation in macrophages (Gautam and Banerjee, 2011). Glucose also promotes CD36 translation via increasing ribosomal reinitiation following enhanced translation of an ORF downstream of the CD36 5′-untranslated region (UTR) (Griffin et al., 2001).
In addition, recent studies indicate that nutrients regulate CD36 expression and translocation at the post-translational levels. FA increases poly-ubiquitination of CD36, contributing to reduce of CD36 protein levels and decrease of cellular fatty acid uptake in C2C12 myotubes and CHO cells (Tran et al., 2011). Interestingly, treatment of FA in cardiomyocytes promotes the translocation of CD36 from endosomes to the sarcolemma by activating vacuolar-type H+-ATPase, rather than alter myocellular protein levels of CD36 (Liu et al., 2017). FA supply can increase the O-linked N-acetylglucosamine (O-GlcNAc) modification of CD36 via hexosamine biosynthesis pathway, resulting in an increase of CD36 expression on cell membranes of gastric cancer cells (Jiang et al., 2019). We have previously demonstrated that FA and HFD promote CD36 palmitoylation and distribution in the plasma membranes of hepatocytes, contributing to NAFLD development and progression (Zhao et al., 2018b). Recent studies also demonstrate the importance of FA in regulating the internalization of CD36, which facilitates the transport of FA into adipocytes (Hao et al., 2020). During this process, binding of FA to CD36 activates its downstream kinase Lyn, which promotes the Tyr91 phosphorylation and inactivation of palmitoyl acyltransferase DHHC5, the palmitoyl acyltransferase of CD36 (Wang et al., 2019). CD36 then gets depalmitoylated by acyl-protein thioesterase 1 (APT1) and recruits another tyrosine kinase SYK to phosphorylate c-Jun N-terminal kinase (JNK) and VAVs to initiate delivery of FAs into cells. These observations underscore the notion that the regulation of FA on CD36 expression/function is tissue-specific and/or via different mechanisms.
Conclusion
With the recognition of the gut-liver axis, the gut-pancreas axis, and the gut-brain axis, the key roles of the intestine in the maintenance of energy homeostasis and diet-related metabolic disorders have been well-accepted. Numerous studies have reported that CD36 is closely related to the development of Mets, atherosclerosis, NAFLD and diabetes. Although the intestinal CD36 is involved in mediating dietary lipids uptake, it seems to play an essential role in the transduction of lipid signals into epithelial cells regulating de novo lipid synthesis, lipids transport and gastrointestinal hormones production/secretion in response to dietary lipid loading. However, much knowledge of CD36 in the intestine is now from humans and animal models where CD36 is less functional or absent systemically. Thus, the intestinal-specific CD36 knockout models are needed to validate the importance of intestinal CD36 in sensing dietary lipids and regulating lipid homeostasis in the future. Moreover, it remains unclear how CD36 regulates intestinal lipoprotein/hormone production and secretion in the fasting and postprandial states. It is also unclear how fat loading in the intestine regulates CD36 expression and post-translational modifications (Figure 1). Further studies are needed to demonstrate the complex interactions between intestinal CD36 and dietary lipids.
Author Contributions
LZ, YuL, QD, and YaL wrote the manuscript. YC and XR revised the manuscript. All authors contributed to the article and approved the submitted version.
Funding
This work was supported by the National Natural Science Foundation of China (32030054, 81970510, and 31971084), the Talent Project of Chongqing (CQYC2019050790), the Science and Technology Research Program of Chongqing Municipal Education Commission (KJQN201900438), the 111 Project of China (D20028), and National Key R&D Program of China (2018YFC1312700).
Conflict of Interest
The authors declare that the research was conducted in the absence of any commercial or financial relationships that could be construed as a potential conflict of interest.
Footnotes
References
Afroze, S., Meng, F., Jensen, K., McDaniel, K., Rahal, K., Onori, P., et al. (2013). The physiological roles of secretin and its receptor. Ann. Transl. Med. 1:29. doi: 10.3978/j.issn.2305-5839.2012.12.01
Aitman, T., Glazier, A., Wallace, C., Cooper, L., Norsworthy, P., Wahid, F., et al. (1999). Identification of Cd36 (Fat) as an insulin-resistance gene causing defective fatty acid and glucose metabolism in hypertensive rats. Nat. Genet. 21, 76–83. doi: 10.1038/5013
Bajit, H., Ait Si Mohammed, O., Guennoun, Y., Benaich, S., Bouaiti, E., Belghiti, H., et al. (2020). Single-nucleotide polymorphism rs1761667 in the CD36 gene is associated with orosensory perception of a fatty acid in obese and normal-weight Moroccan subjects. J. Nutr. Sci. 9:e24. doi: 10.1017/jns.2020.18
Campbell, J. E. (2020). Targeting the GIPR for obesity: to agonize or antagonize? Potential mechanisms. Mol. Metab. 2020:101139. doi: 10.1016/j.molmet.2020.101139
Chassen, S., Ferchaud-Roucher, V., Gupta, M., Jansson, T., and Powell, T. (2018). Alterations in placental long chain polyunsaturated fatty acid metabolism in human intrauterine growth restriction. Clin. Sci. 132, 595–607. doi: 10.1042/cs20171340
Chen, Y., Yang, M., Huang, W., Chen, W., Zhao, Y., Schulte, M., et al. (2019). Mitochondrial metabolic reprogramming by CD36 signaling drives macrophage inflammatory responses. Circ. Res. 125, 1087–1102. doi: 10.1161/circresaha.119.315833
Cheng, C. Y., Chu, J. Y., and Chow, B. K. (2011). Central and peripheral administration of secretin inhibits food intake in mice through the activation of the melanocortin system. Neuropsychopharmacology 36, 459–471. doi: 10.1038/npp.2010.178
Cifarelli, V., Ivanov, S., Xie, Y., Son, N., Saunders, B., Pietka, T., et al. (2017). CD36 deficiency impairs the small intestinal barrier and induces subclinical inflammation in mice. Cell. Mol. Gastroenterol. Hepatol. 3, 82–98. doi: 10.1016/j.jcmgh.2016.09.001
Dai, Y., Dai, D., Wang, X., Ding, Z., Li, C., and Mehta, J. L. (2014). GLP-1 agonists inhibit ox-LDL uptake in macrophages by activating protein kinase A. J. Cardiovasc. Pharmacol. 64, 47–52. doi: 10.1097/FJC.0000000000000087
Demenis, C., McLaughlin, J., and Smith, C. P. (2017). Sulfated cholecystokinin-8 promotes CD36-mediated fatty acid uptake into primary mouse duodenal enterocytes. Front. Physiol. 8:660. doi: 10.3389/fphys.2017.00660
Dockray, G. J. (2012). Cholecystokinin. Curr. Opin. Endocrinol. Diabetes Obes. 19, 8–12. doi: 10.1097/MED.0b013e32834eb77d
Drover, V., Ajmal, M., Nassir, F., Davidson, N., Nauli, A., Sahoo, D., et al. (2005). CD36 deficiency impairs intestinal lipid secretion and clearance of chylomicrons from the blood. J. Clin. Invest. 115, 1290–1297. doi: 10.1172/jci21514
Drover, V., Nguyen, D., Bastie, C., Darlington, Y., Abumrad, N., Pessin, J., et al. (2008). CD36 mediates both cellular uptake of very long chain fatty acids and their intestinal absorption in mice. J. Biol. Chem. 283, 13108–13115. doi: 10.1074/jbc.M708086200
El-Yassimi, A., Hichami, A., Besnard, P., and Khan, N. A. (2008). Linoleic acid induces calcium signaling, Src kinase phosphorylation, and neurotransmitter release in mouse CD36-positive gustatory cells. J. Biol. Chem. 283, 12949–12959. doi: 10.1074/jbc.M707478200
Fujii, R., Hishida, A., Suzuki, K., Imaeda, N., Goto, C., Hamajima, N., et al. (2019). Cluster of differentiation 36 gene polymorphism (rs1761667) is associated with dietary MUFA intake and hypertension in a Japanese population. Br. J. Nutr. 121, 1215–1222. doi: 10.1017/s0007114519000679
Gautam, S., and Banerjee, M. (2011). The macrophage Ox-LDL receptor, CD36 and its association with type II diabetes mellitus. Mol. Genet. Metab. 102, 389–398. doi: 10.1016/j.ymgme.2010.12.012
Goudriaan, J., Dahlmans, V., Febbraio, M., Teusink, B., Romijn, J., Havekes, L., et al. (2002). Intestinal lipid absorption is not affected in CD36 deficient mice. Mol. Cell. Biochem. 239, 199–202. doi: 10.1023/a:1020575412789
Gribble, F. M., and Reimann, F. (2016). Enteroendocrine cells: chemosensors in the intestinal epithelium. Annu. Rev. Physiol. 78, 277–299. doi: 10.1146/annurev-physiol-021115-105439
Griffin, E., Re, A., Hamel, N., Fu, C., Bush, H., McCaffrey, T., et al. (2001). A link between diabetes and atherosclerosis: glucose regulates expression of CD36 at the level of translation. Nat. Med. 7, 840–846. doi: 10.1038/89969
Haber, A. L., Biton, M., Rogel, N., Herbst, R. H., Shekhar, K., Smillie, C., et al. (2017). A single-cell survey of the small intestinal epithelium. Nature 551, 333–339. doi: 10.1038/nature24489
Hao, J., Wang, J., Guo, H., Zhao, Y., Sun, H., Li, Y., et al. (2020). CD36 facilitates fatty acid uptake by dynamic palmitoylation-regulated endocytosis. Nat. Commun. 11:4765. doi: 10.1038/s41467-020-18565-8
Holst, J. J. (2007). The physiology of glucagon-like peptide 1. Physiol. Rev. 87, 1409–1439. doi: 10.1152/physrev.00034.2006
Hsieh, J., Longuet, C., Maida, A., Bahrami, J., Xu, E., Baker, C. L., et al. (2009). Glucagon-like peptide-2 increases intestinal lipid absorption and chylomicron production via CD36. Gastroenterology 137, 997–1005. doi: 10.1053/j.gastro.2009.05.051
Jiang, M., Wu, N., Xu, B., Chu, Y., Li, X., Su, S., et al. (2019). Fatty acid-induced CD36 expression via O-GlcNAcylation drives gastric cancer metastasis. Theranostics 9, 5359–5373. doi: 10.7150/thno.34024
Kato, T., Harada, N., Ikeguchi, E., Sankoda, A., Hatoko, T., Lu, X., et al. (2021). Gene expression of nutrient-sensing molecules in I cells of CCK reporter male mice. J. Mol. Endocrinol. 66, 11–22. doi: 10.1530/JME-20-0134
Keller, K. L., Liang, L. C., Sakimura, J., May, D., van Belle, C., Breen, C., et al. (2012). Common variants in the CD36 gene are associated with oral fat perception, fat preferences, and obesity in African Americans. Obesity 20, 1066–1073. doi: 10.1038/oby.2011.374
Klenotic, P., Page, R., Li, W., Amick, J., Misra, S., and Silverstein, R. (2013). Molecular basis of antiangiogenic thrombospondin-1 type 1 repeat domain interactions with CD36. Arterioscleros. Thromb. Vasc. Biol. 33, 1655–1662. doi: 10.1161/atvbaha.113.301523
Laugerette, F., Passilly-Degrace, P., Patris, B., Niot, I., Febbraio, M., Montmayeur, J. P., et al. (2005). CD36 involvement in orosensory detection of dietary lipids, spontaneous fat preference, and digestive secretions. J. Clin. Invest. 115, 3177–3184. doi: 10.1172/JCI25299
Le Foll, C., Dunn-Meynell, A. A., and Levin, B. E. (2015). Role of FAT/CD36 in fatty acid sensing, energy, and glucose homeostasis regulation in DIO and DR rats. Am. J. Physiol. Regul. Integr. Comp. Physiol. 308, R188–R198. doi: 10.1152/ajpregu.00367.2014
Little, T. J., Isaacs, N. J., Young, R. L., Ott, R., Nguyen, N. Q., Rayner, C. K., et al. (2014). Characterization of duodenal expression and localization of fatty acid-sensing receptors in humans: relationships with body mass index. Am. J. Physiol. Gastrointest Liver Physiol. 307, G958–G967. doi: 10.1152/ajpgi.00134.2014
Liu, Y., Steinbusch, L., Nabben, M., Kapsokalyvas, D., van Zandvoort, M., Schönleitner, P., et al. (2017). Palmitate-induced vacuolar-Type H-ATPase inhibition feeds forward into insulin resistance and contractile dysfunction. Diabetes 66, 1521–1534. doi: 10.2337/db16-0727
Lobo, M. V., Huerta, L., Ruiz-Velasco, N., Teixeiro, E., de la Cueva, P., Celdran, A., et al. (2001). Localization of the lipid receptors CD36 and CLA-1/SR-BI in the human gastrointestinal tract: towards the identification of receptors mediating the intestinal absorption of dietary lipids. J. Histochem. Cytochem. 49, 1253–1260. doi: 10.1177/002215540104901007
Love-Gregory, L., and Abumrad, N. (2011). CD36 genetics and the metabolic complications of obesity. Curr. Opin. Clin. Nutr. Metab. Care 14, 527–534. doi: 10.1097/MCO.0b013e32834bbac9
Love-Gregory, L., Kraja, A., Allum, F., Aslibekyan, S., Hedman, Å, Duan, Y., et al. (2016). Higher chylomicron remnants and LDL particle numbers associate with CD36 SNPs and DNA methylation sites that reduce CD36. J. Lipid Res. 57, 2176–2184. doi: 10.1194/jlr.P065250
Luiken, J., Chanda, D., Nabben, M., Neumann, D., and Glatz, J. (2016). Post-translational modifications of CD36 (SR-B2): implications for regulation of myocellular fatty acid uptake. Biochim. Biophys. Acta 1862, 2253–2258. doi: 10.1016/j.bbadis.2016.09.004
Lynes, M., Narisawa, S., Millán, J., and Widmaier, E. (2011). Interactions between CD36 and global intestinal alkaline phosphatase in mouse small intestine and effects of high-fat diet. Am. J. Physiol. Regul. Integrat. Comparat. Physiol. 301, R1738–R1747. doi: 10.1152/ajpregu.00235.2011
Markovic, M. A., Srikrishnaraj, A., Tsang, D., and Brubaker, P. L. (2020). Requirement for the intestinal epithelial insulin-like growth factor-1 receptor in the intestinal responses to glucagon-like peptide-2 and dietary fat. FASEB J. 34, 6628–6640. doi: 10.1096/fj.202000169R
Masuda, D., Hirano, K., Oku, H., Sandoval, J., Kawase, R., Yuasa-Kawase, M., et al. (2009). Chylomicron remnants are increased in the postprandial state in CD36 deficiency. J. Lipid Res. 50, 999–1011. doi: 10.1194/jlr.P700032-JLR200
Mellitzer, G., and Gradwohl, G. (2011). Enteroendocrine cells and lipid absorption. Curr. Opin. Lipidol. 22, 171–175. doi: 10.1097/MOL.0b013e32834622a2
Nassir, F., Wilson, B., Han, X., Gross, R., and Abumrad, N. (2007). CD36 is important for fatty acid and cholesterol uptake by the proximal but not distal intestine. J. Biol. Chem. 282, 19493–19501. doi: 10.1074/jbc.M703330200
Nauli, A., Nassir, F., Zheng, S., Yang, Q., Lo, C., Vonlehmden, S., et al. (2006). CD36 is important for chylomicron formation and secretion and may mediate cholesterol uptake in the proximal intestine. Gastroenterology 131, 1197–1207. doi: 10.1053/j.gastro.2006.08.012
Ozdener, M. H., Subramaniam, S., Sundaresan, S., Sery, O., Hashimoto, T., Asakawa, Y., et al. (2014). CD36- and GPR120-mediated Ca+ signaling in human taste bud cells mediates differential responses to fatty acids and is altered in obese mice. Gastroenterology 146, 995–1005. doi: 10.1053/j.gastro.2014.01.006
Pepino, M. Y., Love-Gregory, L., Klein, S., and Abumrad, N. A. (2012). The fatty acid translocase gene CD36 and lingual lipase influence oral sensitivity to fat in obese subjects. J. Lipid Res. 53, 561–566. doi: 10.1194/jlr.M021873
Pietka, T., Schappe, T., Conte, C., Fabbrini, E., Patterson, B., Klein, S., et al. (2014). Adipose and muscle tissue profile of CD36 transcripts in obese subjects highlights the role of CD36 in fatty acid homeostasis and insulin resistance. Diabetes Care 37, 1990–1997. doi: 10.2337/dc13-2835
Pietka, T., Sulkin, M., Kuda, O., Wang, W., Zhou, D., Yamada, K., et al. (2012). CD36 protein influences myocardial Ca2+ homeostasis and phospholipid metabolism: conduction anomalies in CD36-deficient mice during fasting. J. Biol. Chem. 287, 38901–38912. doi: 10.1074/jbc.M112.413609
Pravenec, M., Landa, V., Zidek, V., Musilova, A., Kren, V., Kazdova, L., et al. (2001). Transgenic rescue of defective Cd36 ameliorates insulin resistance in spontaneously hypertensive rats. Nat. Genet. 27, 156–158. doi: 10.1038/84777
Sandoval, D. A., and D’Alessio, D. A. (2015). Physiology of proglucagon peptides: role of glucagon and GLP-1 in health and disease. Physiol. Rev. 95, 513–548. doi: 10.1152/physrev.00013.2014
Schwartz, G. J., Fu, J., Astarita, G., Li, X., Gaetani, S., Campolongo, P., et al. (2008). The lipid messenger OEA links dietary fat intake to satiety. Cell Metab. 8, 281–288. doi: 10.1016/j.cmet.2008.08.005
Sekar, R., and Chow, B. K. (2014). Secretin receptor-knockout mice are resistant to high-fat diet-induced obesity and exhibit impaired intestinal lipid absorption. FASEB J. 28, 3494–3505. doi: 10.1096/fj.13-247536
Shi, J., Sun, S., Liao, Y., Tang, J., Xu, X., Qin, B., et al. (2019). Advanced oxidation protein products induce G1 phase arrest in intestinal epithelial cells via a RAGE/CD36-JNK-p27kip1 mediated pathway. Redox Biol. 25:101196. doi: 10.1016/j.redox.2019.101196
Shiau, Y., Popper, D., Reed, M., Umstetter, C., Capuzzi, D., and Levine, G. (1985). Intestinal triglycerides are derived from both endogenous and exogenous sources. Am. J. Physiol. 248, G164–G169. doi: 10.1152/ajpgi.1985.248.2.G164
Shibao, C. A., Celedonio, J. E., Tamboli, R., Sidani, R., Love-Gregory, L., Pietka, T., et al. (2018). CD36 modulates fasting and preabsorptive hormone and bile acid levels. J. Clin. Endocrinol. Metab. 103, 1856–1866. doi: 10.1210/jc.2017-01982
Shu, H., Peng, Y., Hang, W., Nie, J., Zhou, N., and Wang, D. (2020). The role of CD36 in cardiovascular disease. Cardiovasc. Res. 2020:cvaa319. doi: 10.1093/cvr/cvaa319
Sundaresan, S., Shahid, R., Riehl, T. E., Chandra, R., Nassir, F., Stenson, W. F., et al. (2013). CD36-dependent signaling mediates fatty acid-induced gut release of secretin and cholecystokinin. FASEB J. 27, 1191–1202. doi: 10.1096/fj.12-217703
Tran, T., Poirier, H., Clément, L., Nassir, F., Pelsers, M., Petit, V., et al. (2011). Luminal lipid regulates CD36 levels and downstream signaling to stimulate chylomicron synthesis. J. Biol. Chem. 286, 25201–25210. doi: 10.1074/jbc.M111.233551
Wang, J., Hao, J., Wang, X., Guo, H., Sun, H., Lai, X., et al. (2019). DHHC4 and DHHC5 facilitate fatty acid uptake by palmitoylating and targeting CD36 to the plasma membrane. Cell Rep. 26, 209–221.e5. doi: 10.1016/j.celrep.2018.12.022
Wang, X. F., Liu, J. J., Xia, J., Liu, J., Mirabella, V., and Pang, Z. P. (2015). Endogenous Glucagon-like Peptide-1 suppresses high-fat food intake by reducing synaptic drive onto mesolimbic dopamine neurons. Cell Rep. 12, 726–733. doi: 10.1016/j.celrep.2015.06.062
Wu, L., Wang, K., Wang, W., Wen, Z., Wang, P., Liu, L., et al. (2018). Glucagon-like peptide-1 ameliorates cardiac lipotoxicity in diabetic cardiomyopathy via the PPARα pathway. Aging Cell 17:e12763. doi: 10.1111/acel.12763
Xiao, C., Dash, S., Morgantini, C., Adeli, K., and Lewis, G. F. (2015). Gut peptides are novel regulators of intestinal lipoprotein secretion: experimental and pharmacological manipulation of lipoprotein metabolism. Diabetes 64, 2310–2318. doi: 10.2337/db14-1706
Xie, S., Lee, Y., Kim, E., Chen, L., Ni, J., Fang, L., et al. (2009). TR4 nuclear receptor functions as a fatty acid sensor to modulate CD36 expression and foam cell formation. Proc. Natl. Acad. Sci. U.S.A. 106, 13353–13358. doi: 10.1073/pnas.0905724106
Yamamoto, Y., Hiasa, Y., Murakami, H., Ikeda, Y., Yamanishi, H., Abe, M., et al. (2012). Rapid alternative absorption of dietary long-chain fatty acids with upregulation of intestinal glycosylated CD36 in liver cirrhosis. Am. J. Clin. Nutr. 96, 90–101. doi: 10.3945/ajcn.111.033084
Zhang, Y., Fu, Z., Wei, J., Qi, W., Baituola, G., Luo, J., et al. (2018). LIMA1A variant promotes low plasma LDL cholesterol and decreases intestinal cholesterol absorption. Science 360, 1087–1092. doi: 10.1126/science.aao6575
Zhao, L., Varghese, Z., Moorhead, J., Chen, Y., and Ruan, X. (2018a). CD36 and lipid metabolism in the evolution of atherosclerosis. Br. Med. Bull. 126, 101–112. doi: 10.1093/bmb/ldy006
Keywords: CD36, dietary lipid, intestine, lipid homeostasis, intestinal hormones
Citation: Zhao L, Li Y, Ding Q, Li Y, Chen Y and Ruan XZ (2021) CD36 Senses Dietary Lipids and Regulates Lipids Homeostasis in the Intestine. Front. Physiol. 12:669279. doi: 10.3389/fphys.2021.669279
Received: 18 February 2021; Accepted: 22 March 2021;
Published: 28 April 2021.
Edited by:
Eugenia Morselli, Pontificia Universidad Católica de Chile, ChileReviewed by:
Kimberly K. Buhman, Purdue University, United StatesNeale David Ridgway, Dalhousie University, Canada
Copyright © 2021 Zhao, Li, Ding, Li, Chen and Ruan. This is an open-access article distributed under the terms of the Creative Commons Attribution License (CC BY). The use, distribution or reproduction in other forums is permitted, provided the original author(s) and the copyright owner(s) are credited and that the original publication in this journal is cited, in accordance with accepted academic practice. No use, distribution or reproduction is permitted which does not comply with these terms.
*Correspondence: Yaxi Chen, Y2hlbnlheGlAY3FtdS5lZHUuY24=; Xiong Z. Ruan, eGlvbmd6cnVhbkBnbWFpbC5jb20=; eC5ydWFuQHVjbC5hYy51aw==