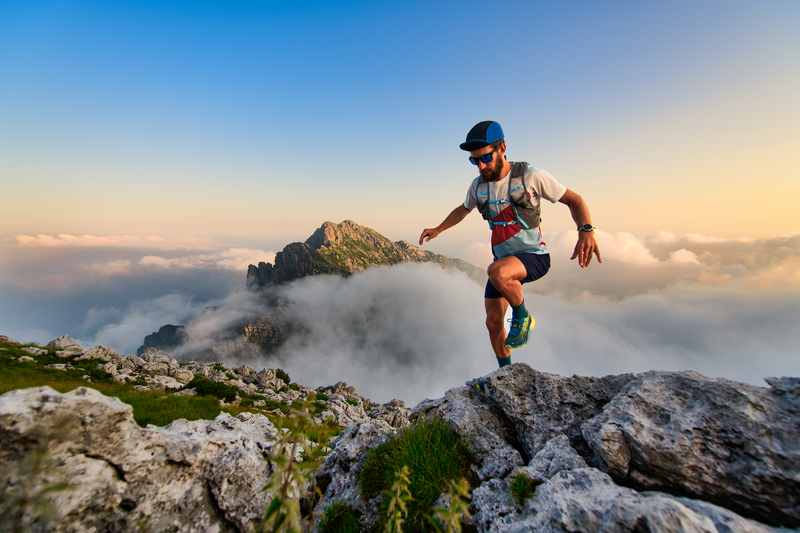
95% of researchers rate our articles as excellent or good
Learn more about the work of our research integrity team to safeguard the quality of each article we publish.
Find out more
ORIGINAL RESEARCH article
Front. Physiol. , 27 April 2021
Sec. Membrane Physiology and Membrane Biophysics
Volume 12 - 2021 | https://doi.org/10.3389/fphys.2021.656647
Currently, the role of Pannexin-1, a homomeric membrane hemichannel on the neuron cell membrane, in the development of spinal cord injury (SCI) is largely unknown. Herein, we assessed the contribution of Panx1 in the development of SCI. The SCI in vitro model was established using rat primary spinal neurocytes treated with hydrogen peroxide (H2O2). Effects of Panx1 overexpression or depletion in spinal neurocytes were analyzed by lentivirus-mediated transfection of Panx1 and interference sh-Panx1. Decreased cell viability was seen in SCI cells, which was further enhanced under Panx1 overexpression and mitigated by Panx1 deficiency. H2O2 induced an increase of intracellular Ca2+ signal and upregulated level of the proapoptotic protein Bax, and apoptosis pathway proteins including cleaved Caspase-3 and PARP1, which was enhanced by Panx1 overexpression or attenuated by Panx1 depletion. On the other hand, H2O2 treatment suppressed the level of antiapoptotic protein Bcl-2, which was further decreased by Panx1 overexpression or mitigated by Panx1 depletion. The results indicate that Panx1 was involved in the intracellular Ca2+ overload of SCI cells by accelerating extracellular Ca2+ influx, which promoted the apoptosis of spinal neurocytes through Ca2+ dependent pathways, thus aggravating the secondary injury of SCI.
Spinal cord injury (SCI) is one major cause of morbidity worldwide with impacts on quality of life and the healthcare system. The pathological outcomes of SCI mainly include damage to the white and gray matter of the spinal cord, which is caused by primary injury and subsequent progressive secondary injury (Colak et al., 2009; Dray et al., 2009). Secondary injury is a complex cascade reaction amplification process of self-destruction caused by primary injury, including ischemia and hypoxia, immune-inflammatory response, excitatory toxicity, free radical damage, lipid peroxidation, which was the main cause of aggravating neurological dysfunction and had reversibility and regulation (Ueno and Yamashita, 2008). Therefore, prevention and intervention of secondary injury is the core content of early SCI treatment (Boulenguez and Vinay, 2009).
In recent years, many scholars have confirmed that cell apoptosis, as well as cell necrosis, exist in the tissues after spinal cord injury. Cell necrosis is caused by primary injury, while cell apoptosis is caused by secondary injury (Yong et al., 1998). It was found that a large number of nerve cell apoptosis existed after SCI. And intracellular calcium ions ([Ca2+]i), plays an important role in the process of apoptosis. Previous studies have shown that [Ca2+]i is the initial messenger of Endoplasmic Reticulum Stress (ERS) reactive apoptosis, although the specific mechanism of ERS is largely unknown (Shen et al., 2002).
In 2000, Panchin found a coding sequence in vertebrates and named it Pannexin (Panx), which is homologous to the innexin gene of the invertebrate. There are three subtypes of Panx: Panx1, Panx2, and Panx3 (Panchin et al., 2000). Panx1 is abundantly expressed in many organs including the brain, spinal cord, bone (Penuela et al., 2007). Panx1 protein mainly functions by forming a homomer hemichannel (Locovei et al., 2006). And Panx1 channels are permeable to ions and molecules up to 1 kDa (Yang et al., 2020), which is large, non-selective plasma membrane channel that permit the movement of molecules and ions, including ATP to the extracellular space and Ca2+ release from ER (Vanden Abeele et al., 2006; Michalski et al., 2020). Vanden Abeele et al. demonstrated that Panx1 channels in the endoplasmic reticulum facilitates the movement of Ca2+ (Vanden Abeele et al., 2006). Yang et al. provided evidences that plasma membrane Panx1 channels were directly permeable to Ca2+, and facilitated increases in [Ca2+]i (Yang et al., 2020).
In the study of cerebral ischemia and anoxia injury, Thompson et al. also found that the Panx1 channel played an important role in cerebral ischemia and anoxia injury by regulating the transmission of intracellular and extracellular Ca2+ and ATP, and inducing neurocytes apoptosis and inflammatory response through various signal pathways (Thompson et al., 2006). However, a contrary evidence proposed by Harcha et al. showed that although panx1 channel located in the endoplasmic reticulum might be permeable to Ca2+, the cell membrane was not permeable to Ca2+ in the Hela cells. This led us to investigate the contribution of Panx1 to and its role in the Ca2+ overload and development of SCI in vitro. We reported here that Panx1 involved in the intracellular Ca2+ overload of SCI cells and promoted the apoptosis of spinal neurocytes.
The embryonic spinal cord was dissected from embryonic day-15 (E15) Sprague-Dawley rat pups under the dissecting microscope (Olympus, Japan), and the spinal membrane and dorsal root ganglia were removed, followed by treatment with 0.15% trypsin (Gibco, United States) for 7 min at 37°C to detach the spinal neurocytes from primary tissues. Cells were washed three times with HBSS, suspended and centrifuged at 1,000rpm for 3min, and resuspended in NPC Medium and seeded on glass coverslips coated with poly-L-lysine in a 6-well plate at a density of 3 × 105 cells/well. The cultures were maintained at 37°C under a humidified atmosphere of 5% CO2. This study was approved by the Institutional Animal Care and Use Committee of Fujian Medical University.
We used immunofluorescence staining of neuronal nuclei (NeuN) and β-tubulin III to identify the primary spinal neurocytes and observed cell apoptosis by the staining of Hoechst33258. After culturing for 5–7 days, cells were fixed with 4% paraformaldehyde in PBS for 10min at room temperature and washed three times with PBS. Then the fixed cells were incubated with 1% BSA for 30 min to block the unspecific binding of the antibodies and permeabilized with 0.1% Triton X-100 solution in PBS for 10min at room temperature. Following by incubating cells in the primary antibody including anti-NeuN (1:200, ABclonal, United States) and anti-β-tubulin III (1:400, ABclonal, United States) overnight at 4°C. Alexa 594 goat anti-rabbit (1:1,000, Abcam, United Kingdom) and Alexa 488 goat anti-rat (1:1,000, Abcam, United Kingdom) was added and incubated at room temperature for 1 h to visualize NeuN and anti-β-tubulin III. Finally, the cell nuclei were stained with Hoechst33258 (Beyotime, China) for 15 min and observed under a fluorescence microscope.
Cells were seeded in a 96-well and treated with H2O2 (100, 300, 500, and 700 μM) in a serum-free NPC medium to establish the SCI cell model. The viability of spinal neurocytes was detected at 0.5, 1, 3, 5, and 7 h after H2O2 exposure using Cell Counting kit-8 (CCK-8) according to the manufacturer’s instruction. The optimal condition for establishing the SCI model was 700 μM H2O2 exposure for 3 h (data not shown).
Overexpression and knockdown of Panx1 were achieved using LV5-Panx1 and Sh-Panx1 recombinant lentivirus. The constructions of the recombinant lentivirus were as follows: GPLV5/EF-1αF/GFP+Puro (LV5) and GPLV3/H1/GFP+Puro (LV3) were purchased from Gene Pharma Co., Ltd. (Shanghai, China). The primer of Panx1 was designed and synthesized with the homologous sequences of NotI and BamHI added to the 5′ end of the forward and reverse primers by Sangon Co., Ltd. (Shanghai, China). The primer sequence was as follows: Primer (+): GGCCGCGCCACCATGGCCATCGCC, Primer (−): GATCCTTAGCAGGATGAATTCAGAAG. The Panx1 gene was amplified by PCR (ABI, United States), and the Panx1 gene fragment was recovered by gel electrophoresis. DNA endonuclease NotI and BamHI were used to perform enzyme digestion of LV5 at 37°C for 2 h. The linear carrier LV5 was recovered by a gel recovery kit. And then the ClonExpress® Entry One Step Cloning Kit was used to clone Panx1 into a linearized LV5 vector. The reaction system was as follows: 5 × CE Entry Buffer 4 μl, LV5 1 μl, Panx1 2 μl, Exnase Entry 2 μl, ddH2O 11 μl. After being well mixed, the reaction was performed at 37°C for 30 min. The reaction tube was placed in an ice bath immediately after the reaction had been completed and cooled for 5 min. After that, the cloned products were transformed into the Top10 competent cells of E. coli, and LV5-Panx1 were extracted with the plasmid extract kit (TIANGEN, China), identified by enzyme digestion, and the positive clones were sent for sequencing. The Lenti Sh-Panx1 plasmid was produced similarly. The target sequence is GCTCCGACCTGAAGTTTAT- CA. GATCC was added to the 5′ end of the forward primer, which was complementary to the sticky end formed after BamHI enzyme digestion, and AATTC is added to the 5′ end of the reverse primer to compliment the sticky end formed by the EcoRI enzyme digestion. After the annealing reaction, a sh-DNA template with a concentration of 10 μM was obtained. Enzyme digestion of LV3 was performed by EcoRI and BamHI. Then the sh-DNA gene was cloned into the vector LV3. The reaction system was as follows: 10 × T4 Ligase Buffer 2 μl, LV3 1 μl, sh-DNA template (100 nM) 1 μl, T4 DNA Ligase (5 Weiss U/μl) 1 μl, ddH2O 15 μl. The reaction was performed at 22°C for 1 h. After transformation, the plasmids were extracted, identified by enzyme digestion, and sent to sequencing. LV5-Panx1 and Sh-Panx1 were co-transfected with the package plasmids (pGag/Pol, pRev, pvsv-g) into 293T cells to produce the recombinant lentivirus LV5-Panx1 and Sh-Panx1, respectively. Empty LV5 and LV3 plasmids were also co-transfected with the package plasmids into 293T cells to produce the LV-con and sh-con as control lentivirus. Virus titer and multiplicity of infections were detected. After the production of LV5-Panx1, Sh-Panx1 and control recombinant lentivirus, they were added to the medium of the primary spinal cord cells and the overexpression and knockdown of Panx1 in the cells were detected by western blot.
The viability of spinal neurocytes was measured using the Cell Counting Kit-8 (Qiagen, United States) (QS) according to the manufacturer’s instructions. Briefly, cells were seeded on a 96-well plate at a density of 1 × 104/ well, after the establishment of SCI and transfected with LV5-Panx1, Sh-Panx1, and control lentivirus, at 3, 8, 24, 48, and 72 h time points, the medium was removed, and 10 μl CCK-8 reagent in 90 μl medium were added. Following incubated at 37°C for 2 h, the absorbances were read at 450 nm on the microplate reader (Bio-Rad, United States). All experiments were run in duplicate and were repeated at least three times.
Cells (NC, LV-con, sh-con, LV5-Panx1, and sh-Panx1) were seeded on a 6-well plate at a density of 1 × 106/ well. At 24 h after H2O2 exposure, cell apoptosis was detected by an annexin V-apoptosis kit. The cells were washed and incubated for 15 min at room temperature in the presence of annexin V-APC and propidium iodide (PI). A flow cytometer (Accuri C6, United States) was used for detection. PI fluorescence was performed using the FL2 channel, while AnnexinV-APC fluorescence was performed using the FL4 channel. 20,000 cells in the portal were collected and apoptosis was analyzed in each group.
Primary neurocytes were seeded in a 28.2 mm dish. At 24 h after H2O2 exposure, the BBcellProbeTMF04 probe (1:200) was added and incubated at 37°C for 40 min. Laser confocal microscopy (Carl Zeiss, Germany) was used to detect intracellular Ca2+ signal in each group. The change of Ca2+ signal was inversely proportional to the intensity of red fluorescence. Image J was used for semi-quantitative analysis of fluorescence intensity in each group.
The cells were diluted to 1 × 105 cell/mL and were incubated for 24 h in six-well plates (Falcon, BD Biosciences). After the establishment of SCI and transfected with LV5-Panx1, Sh-Panx1, and control lentivirus for 24 h. Total RNA of rat spinal neurocytes was extracted by Trizol (Invitrogen, United States). The PrimeScriptTM RT reagent Kit with gDNA Eraser Kit (TaKaRa, Japan) was used to conduct a Reverse Transcription reaction to synthesize cDNA. The primers of β-actin, Bcl-2, Bax, Caspase3, PARP1 were designed and synthesized by Sangon Co., Ltd. (Shanghai, China), and the sequence was shown in the Table 1. Real-time PCR reaction solution was prepared according to the instruction of SYBR® Premix Ex TaqTM (Tli RNaseH Plus) (TaKaRa, Japan). Reaction conditions were set on the fluorescence quantitative PCR apparatus (LightCycler/LightCycler480 System, Roche, Switzerland): denaturation at 95°C for 30 s. PCR at 95°C for 5 s, 60°C for 30 s, 40 cycles. Dissolution at 95°C for 5 s, 60°C for 1 min. The Ct value of the target gene and β-actin of each group was generated by the fluorescence quantitative PCR apparatus, and each gene expression of relative quantity was calculated by the formula: F = 2–ΔΔCt, and the normal cells were used as the control.
The levels of Bcl-2, Bax, Caspase3, and PARP1, regulatory genes involved in the intracellular Ca2+ homeostasis, and apoptosis pathways, were determined by western blot analysis. Cells were harvested and lysed for western blot analysis. Lysates were prepared as previously described (Zhang et al., 2019). Membranes were incubated with the following primary antibodies: anti Bcl-2 (1:800, ABclonal, United States), anti Bax (1:800, ABclonal, United States), anti Caspase3 (1:1,000, ABclonal, United States), anti PARP1 (1:1,000, ABclonal, United States). HRP-conjugated secondary Abs were applied, and a supersensitive ECL Chemiluminescence Kit (Beyotime, China) was used to detect proteins. anti-β-actin (1:1,600, ABclonal, United States) was used as a loading control.
Data are analyzed using SPSS 20.0 software (SPSS Inc., Chicago, IL, United States). All experiments were performed at least three times. Data were presented as the mean ± SD. Student’s t-test and/or ANOVA were performed for statistical analyses, and probability values < 0.05 indicated statistically significant differences.
SCI primary spinal neurocytes showed reduced cell viability when compared with normal cells at 24, 48, and 72 h time points after H2O2 treatment. Panx1 overexpression (LV5-Panx1) further reduced cell viability when compared with control SCI cells (SCI + LV-con) and SCI cells (Figure 1A). On the other hand, Panx1 depletion reversed the cell injury and the Panx1 depletion cells showed increased cell viability when compared with control SCI cells (SCI + sh-con) and SCI cells (Figure 1B).
Figure 1. Cell viability of the rat primary spinal neurocytes SCI model was reduced by Panx1 overexpression and increased by Panx1 depletion, in vitro. (A) SCI primary spinal neurocytes showed reduced cell viability when compared with normal cells at 24 h, 48 h, and 72 h time points after H2O2 treatment. Panx1 overexpression (LV5-Panx1) further reduced cell viability when compared with control cells (LV-con) and normal cells after H2O2 treatment. (B) Panx1 depletion (sh-Panx1) promoted cell viability at 24, 48, and 72 h time points after H2O2 treatment when compared with control cells (sh-con) and normal cells after H2O2 treatment.
Cell apoptosis induced by H2O2 was measured by Hoechst33258 staining under fluorescence microscopy (Figures 2A,B) and flow cytometry (FCM) with Annexin V-APC/PI staining (Figures 2C,D). Increased number of apoptotic cells were shown in SCI model comparing with untreated normal cells (Figure 2A), Panx1 overexpression (LV5-Panx1) further increased the number of apoptotic cells at 24 h time point after H2O2 treatment, when compared with control cells (LV-con) and normal cells after H2O2 treatment. On the other hand, a reduced number of apoptotic cells in SCI model cells with Panx1 depletion (sh-Panx1) were observed when compared with cells transfected with sh-con or SCI model cells (Figures 2A,B). Our finding suggested that Panx1 increased the cell apoptosis of the primary spinal neurocytes after H2O2 treatment, therefore increased SCI in the cells, in vitro. Besides, Annexin V apoptosis analysis demonstrated an increased apoptotic cell population in the Panx1 overexpression cells compared with cells transfected with LV-con or treated with a vehicle. On the other hand, a reduced number of apoptotic cells in the SCI model with Panx1 depletion (sh-Panx1) were observed when compared with cells transfected with sh-con or treated with vehicle, this suggested Panx1 increased cell apoptosis in the rat primary spinal neurocytes SCI model, in vitro (Figures 2C,D). Together, we showed that Panx1 decreased cell viability, induced upregulation of apoptosis in the SCI model, in vitro.
Figure 2. Panx1 increased cell apoptosis in the rat primary spinal neurocytes SCI model, in vitro. (A) Cell apoptosis induced by H2O2 was measured by Hoechst33258 staining under fluorescence microscopy, β-tubulin III served as a neuron cell surface maker. The nuclei of apoptotic cells were dense or fragmented. Increased number of apoptotic cells were shown in SCI model comparing with untreated normal cells, Panx1 overexpression (LV5-Panx1) further increased the number of apoptotic cells at 24 h time point after H2O2 treatment, while Panx1 depletion reverses the effect when compared with control cells (LV-con, sh-con) and normal cells after H2O2 treatment. (B) The apoptotic index was measured as the percentage of apoptotic cells and bodies per all cells. mean ± SD was used, n = 5, *p < 0.05, **p < 0.01. (C) Annexin V/Propidium Iodide (PI) apoptosis analysis demonstrated an increased apoptotic cell population in the SCI cells at 24 h time point after H2O2 treatment, Panx1 overexpression (LV5-Panx1) further increased the number of apoptotic cells at 24 h time point after H2O2 treatment, while Panx1 depletion reverses the effect when compared with control cells (LV-con, sh-con) and normal cells after H2O2 treatment. (D) The apoptotic index was measured using flow cytometry (Q3), mean ± SD was used, n = 3, **p < 0.01.
Increased intracellular Ca2+ is linked to neuronal apoptosis. We detect intracellular Ca2+ signal with a confocal microscope. The red fluorescence intensity was inversely proportional to the change of the intracellular Ca2+ signal. Our results showed elevated intracellular Ca2+ signal in SCI model cells comparing with untreated normal cells. Panx1 overexpression (LV5-Panx1) enhanced the intracellular Ca2+ signal while Panx1 depletion reversed this effect compared with control cells (LV-con, sh-con) and normal cells at 24 h time point after H2O2 treatment (Figure 3). This finding suggested that Panx1 increased cell apoptosis via the upregulation of intracellular Ca2+ in the SCI model, in vitro.
Figure 3. Panx1 increased the intracellular Ca2+ signal of SCI cells. (A) We detect the intracellular Ca2+ signal with a confocal microscope. The red fluorescence intensity was inversely proportional to that of the intracellular Ca2+ signal. Normal cells without treatment served as control. Elevated intracellular Ca2+ signal was shown in SCI model cells comparing with untreated normal cells. Panx1 overexpression (LV5-Panx1) enhanced the intracellular Ca2+ level while Panx1 depletion reversed this effect compared with control cells (LV-con, sh-con) and normal cells at 24 h time point after H2O2 treatment. (B) A densitometric quantification of optical density was performed by Image J and expressed as IntDen relative to the area. It was inversely proportional to that of the intracellular Ca2+ level. Mean ± SD was used, n = 3, **p < 0.01.
SCI cells showed elevated mRNA and protein levels of proapoptotic protein Bax, and apoptosis pathway proteins including cleaved Caspase-3 and PARP1, as well as the decreased levels ofantiapoptotic protein Bcl-2. Panx1 overexpression (LV5-Panx1) further enhanced the level of Bax, Caspase-3 and PARP1, but suppressed the level of Bcl-2, when compared to SCI cells or SCI cells transfected with LV-con. On the other hand, Panx1 depletion (sh-Panx1) reduced the levels of Bax, Caspase-3 and PARP1 but increased the level of Bcl-2, when compared to SCI cells or SCI cells transfected with sh-con (Figure 4).
Figure 4. Panx1 increased the level of Bax, cleaved Caspase-3 and PARP1 but suppressed the level of Bcl-2. mRNA and protein level of Bcl-2, Bax, Caspase-3, and PARP1were analyzed by RT-PCR (A) and western blot (B) at 24 h time point after H2O2 treatment. (C) Quantification of protein bands using ImageJ Gel Analysis program. SCI cells showed elevated mRNA and protein level of proapoptotic protein Bax, and apoptosis pathway proteins including cleaved Caspase-3 and PARP1, as well as the decreased level of antiapoptotic protein Bcl-2. Panx1 overexpression (LV5-Panx1) further enhanced the level of Bax, Caspase-3 and PARP1, but suppressed the level of Bcl-2, when compared to SCI cells or SCI cells transfected with LV-con. On the other hand, Panx1 depletion (sh-Panx1) reduced the levels of Bax, Caspase-3 and PARP1 but increased the level of Bcl-2, when compared to SCI cells or SCI cells transfected with sh-con. Mean ± SD was used, n = 3, * p < 0.05, **p < 0.01.
To identify the spinal primary neurocytes, we used immunofluorescence staining of specific NeuN and tubulin-III to label the cells. NeuN and tubulin-III double-positive cells were identified as the spinal primary neurocytes. Hoechst33258 served as the nuclear counterstain. The percentage of spinal cord neurocytes in the isolated primary cells was identified to be 90% (Supplementary Figure 1).
In this study, we established the SCI model of rat primary spinal cord cells. We showed that decreased cell viability and apoptosis were seen in SCI cells, which was further enhanced under Panx1 overexpression and mitigated by Panx1 deficiency. H2O2 induced an increase of intracellular Ca2+ signal and upregulated levels of Bax, Caspase-3, and PARP1, which was enhanced by Panx1 overexpression or attenuated by Panx1 depletion. The results indicated that Panx1 involved in the intracellular Ca2+ overload of SCI cells, which led to endoplasmic reticulum stress (ERS) and promoted the apoptosis of spinal neurocytes through the endoplasmic reticulum pathway, thus aggravating the secondary injury of SCI.
Nerve cell apoptosis was frequently seen after SCI. Glial cell apoptosis was observed in the damaged area at 4–9 days after SCI (Li et al., 1996, 2000). And Crowe et al. (1997) [1][5] reported a large number of apoptotic cells in the degenerative nerve fiber bundles of monkeys. Apoptosis of neurocytes was found around the damaged area in the spinal cord of patients who died of SCI in 3 h to 2 months, with degenerative changes occurred in adjacent segments (Emery et al., 1998). Besides, studies have confirmed that neurocytes necrosis was dominant in the early stage of SCI in rats, and the apoptosis of neurocytes began in 6 h after injury, which could last for 2 weeks or even longer (Liu et al., 2020). Panx1 was correlated with the inflammatory response (Pelegrin and Surprenant, 2006; Orellana et al., 2013), Pyroptosis (Kayagaki et al., 2011, 2013; Hagar et al., 2013; de Gassart and Martinon, 2015; Yang et al., 2015), and autophagy (Ayna et al., 2012; Martins et al., 2014) after nervous system injury, which promoted apoptosis in the cells. These studies in line with our data that showed overexpression of Panx1 increased the apoptosis rate in SCI cells, which were migrated by Panx1 depletion. Therefore, it was revealed that Panx1 was connected to the apoptosis of nerve cells after SCI.
Ca2+ was linked to neuronal cell apoptosis. The increased intracellular Ca2+ in neurons could trigger the arachidonic acid metabolism cascade and activate the xanthine oxidation system through oxidative stress (Lukic-Panin et al., 2007), causing the formation of a large number of free radicals, forming a vicious cycle, and finally activating the Ca2+ dependent endonuclease, initiating the neuronal apoptosis process, leading to neuronal apoptosis (Guven et al., 2011). The activated Panx1 channel could result in increased intracellular Ca2+. Yang et al. (2020) provided several lines of evidence that suggested that plasma membrane Panx1 channels were directly permeable to Ca2+, and facilitated increases in [Ca2+]i. These included no Ca2+ response to TNF when Panx1 was silenced by siRNA or the channel was inhibited using the Panx1 specific inhibitor peptide PxIL2P. In addition, increases in [Ca2+]i are associated with IL-1β -synthesis, that can be ablated following reductions in [Ca2+]i by blocking the Panx1 channel (Yang et al., 2020). These evidences support our data that showed Panx1 overexpression promoted [Ca2+]i and resulted in cell apoptosis. While depletion of Panx1 improved the viability of neurocytes after SCI and reduced [Ca2+]i, therefore had a protective effect on oxidative stress injury of neurocytes.
Bcl-2 and Bax were two typical regulatory genes (Kale et al., 2018; Kalkavan and Green, 2018), which participated in the maintenance of intracellular Ca2+ homeostasis. Bcl-2 exerted a direct effect on the Ca2+ handling in the ER by regulating the movement of Ca2+ through the ER membrane. Overexpression of proapoptotic Bax or knock-down Bcl-2 increased Ca2+ and augmented the number of releasable ER Ca2+ (Xu et al., 2008; Chiu et al., 2018). However, Bax is mostly distributed in the cytoplasm in the form of an inactive monomer through forming a heterodimer with Bcl-2, which blocked the anti-apoptotic function of Bcl-2 (Hetz et al., 2006). Therefore, the ratio of Bcl-2 to Bax is of great significance in determining whether the cells enter the state of apoptosis.
Caspase-3 was the main effector Caspase, a common downstream effector protein of various apoptosis pathways, which played a central role in the apoptosis process and was known as the “death executive protease” (Rogers et al., 2017). When the Ca2+ homeostasis of the endoplasmic reticulum was disrupted or excessive protein accumulation in the endoplasmic reticulum, Caspase-12 might indirectly activate caspase-3, which was triggered by ERS, but the specific mechanism is not clear and needs to be confirmed by further studies (Lamb et al., 2006; Tan et al., 2006; Kim et al., 2008). Activated cleaved-Caspase-3 cleaved the DNA repair protein PARP-1 in the nucleus, causing cell disintegration and eventually leading to cell apoptosis (Alano et al., 2010; Ba and Garg, 2011). Our results demonstrated SCI activated Panx1, which led to the increase of intracellular Ca2+. B-cell lymphoma-2 (Bcl-2) family of proteins, including pro-apoptotic and anti-apoptotic members as well, associated with the neuronal Ca2+ homeostasis and mediated the intrinsic apoptosis pathway by controlling mitochondrial outer membrane (MOM) integrity. Bax as one of the “pro-apoptotic” Bcl-2 family proteins played a critical role in the apoptosis pathway after the neuronal injury (D’Orsi et al., 2017). Our results indicated that increased intracellular Ca2+ after SCI resulted in elevated Bax level in spinal cord cells, which bonded to the antiapoptotic protein Bcl-2, decreased its level, and initiated the intrinsic apoptosis pathway, including the increase of cleaved caspase-3, and PARP-1.
Our study demonstrated for the first time that Panx1 increased cell apoptosis in SCI spinal cord cells in vitro. This effect was associated with intracellular Ca2+ overload, and in turn, activated the cell apoptosis pathways. Our findings suggested that the Panx1 channel was a promising new therapeutic target for the treatment of SCI. One of the limitations in the present study is that we cannot identify whether the increase intracellular Ca2+ induced by SCI is from extracellular Ca2+ or Ca2+ release from ER. Further studies are needed to identify the mechanisms of Panx1 channel mediated control of [Ca2+]i.
The original contributions presented in the study are included in the article/Supplementary Material, further inquiries can be directed to the corresponding author/s.
YH designed and performed the experiments, interpreted the results, and prepared the manuscript. JinL and XC helped in performing experiments. JiaL conceived, designed, and oversaw the project. All authors have read and approved the manuscript for publication.
This work was supported by the Joint project of the Department of Health and Medical Education (grant no. 2019-WJ-30).
The authors declare that the research was conducted in the absence of any commercial or financial relationships that could be construed as a potential conflict of interest.
The Supplementary Material for this article can be found online at: https://www.frontiersin.org/articles/10.3389/fphys.2021.656647/full#supplementary-material
Supplementary Figure 1 | Identification of isolated spinal primary neurocytes. The spinal primary neurocytes were isolated from rat embryos. (a,b) NeuN and tubulin-III staining double-positive cells were identified as the spinal primary neurocytes. (c) Hoechst33258 served as the nuclear counterstain. (d) Merged image of (a–c). The percentage of spinal cord neurocytes in the isolated primary cells was 90%.
Alano, C. C., Garnier, P., Ying, W., Higashi, Y., Kauppinen, T. M., and Swanson, R. A. (2010). NAD+ depletion is necessary and sufficient for poly(ADP-ribose) polymerase-1-mediated neuronal death. J. Neurosci. 30, 2967–2978. doi: 10.1523/jneurosci.5552-09.2010
Ayna, G., Krysko, D. V., Kaczmarek, A., Petrovski, G., Vandenabeele, P., and Fésüs, L. (2012). ATP release from dying autophagic cells and their phagocytosis are crucial for inflammasome activation in macrophages. PLoS One 7:e40069. doi: 10.1371/journal.pone.0040069
Ba, X., and Garg, N. J. (2011). Signaling mechanism of poly(ADP-ribose) polymerase-1 (PARP-1) in inflammatory diseases. Am. J. Pathol. 178, 946–955. doi: 10.1016/j.ajpath.2010.12.004
Boulenguez, P., and Vinay, L. (2009). Strategies to restore motor functions after spinal cord injury. Curr. Opin. Neurobiol. 19, 587–600. doi: 10.1016/j.conb.2009.10.005
Chiu, W. T., Chang, H. A., Lin, Y. H., Lin, Y. S., Chang, H. T., Lin, H. H., et al. (2018). Bcl(-)2 regulates store-operated Ca(2+) entry to modulate ER stress-induced apoptosis. Cell Death Discov. 4:37.
Colak, A., Kaya, M., Karaoğlan, A., Sağmanligil, A., Akdemir, O., Sahan, E., et al. (2009). Calpain inhibitor AK 295 inhibits calpain-induced apoptosis and improves neurologic function after traumatic spinal cord injury in rats. Neurocirugia 20, 245–254. doi: 10.1016/s1130-1473(09)70163-0
Crowe, M. J., Bresnahan, J. C., Shuman, S. L., Masters, J. N., and Beattie, M. S. (1997). Apoptosis and delayed degeneration after spinal cord injury in rats and monkeys. Nat. Med. 3, 73–76. doi: 10.1038/nm0197-73
D’Orsi, B., Mateyka, J., and Prehn, J. H. M. (2017). Control of mitochondrial physiology and cell death by the Bcl-2 family proteins Bax and Bok. Neurochem. Int. 109, 162–170. doi: 10.1016/j.neuint.2017.03.010
de Gassart, A., and Martinon, F. (2015). Pyroptosis: caspase-11 unlocks the gates of death. Immunity 43, 835–837. doi: 10.1016/j.immuni.2015.10.024
Dray, C., Rougon, G., and Debarbieux, F. (2009). Quantitative analysis by in vivo imaging of the dynamics of vascular and axonal networks in injured mouse spinal cord. Proc. Natl. Acad. Sci. U.S.A. 106, 9459–9464. doi: 10.1073/pnas.0900222106
Emery, E., Aldana, P., Bunge, M. B., Puckett, W., Srinivasan, A., Keane, R. W., et al. (1998). Apoptosis after traumatic human spinal cord injury. J. Neurosurg. 89, 911–920. doi: 10.3171/jns.1998.89.6.0911
Guven, H., Cilliler, A. E., Koker, C., Sarikaya, S. A., and Comoglu, S. S. (2011). Association of serum calcium levels with clinical severity of acute ischemic stroke. Acta Neurol. Belg. 111, 45–49.
Hagar, J. A., Powell, D. A., Aachoui, Y., Ernst, R. K., and Miao, E. A. (2013). Cytoplasmic LPS activates caspase-11: implications in TLR4-independent endotoxic shock. Science 341, 1250–1253. doi: 10.1126/science.1240988
Hetz, C., Bernasconi, P., Fisher, J., Lee, A. H., Bassik, M. C., Antonsson, B., et al. (2006). Proapoptotic BAX and BAK modulate the unfolded protein response by a direct interaction with IRE1alpha. Science 312, 572–576. doi: 10.1126/science.1123480
Kale, J., Osterlund, E. J., and Andrews, D. W. (2018). BCL-2 family proteins: changing partners in the dance towards death. Cell Death Differ. 25, 65–80. doi: 10.1038/cdd.2017.186
Kalkavan, H., and Green, D. R. (2018). MOMP, cell suicide as a BCL-2 family business. Cell Death Differ. 25, 46–55. doi: 10.1038/cdd.2017.179
Kayagaki, N., Warming, S., Lamkanfi, M., Vande Walle, L., Louie, S., Dong, J., et al. (2011). Non-canonical inflammasome activation targets caspase-11. Nature 479, 117–121.
Kayagaki, N., Wong, M. T., Stowe, I. B., Ramani, S. R., Gonzalez, L. C., Akashi-Takamura, S., et al. (2013). Noncanonical inflammasome activation by intracellular LPS independent of TLR4. Science 341, 1246–1249. doi: 10.1126/science.1240248
Kim, H. R., Lee, G. H., Ha, K. C., Ahn, T., Moon, J. Y., Lee, B. J., et al. (2008). Bax inhibitor-1 Is a pH-dependent regulator of Ca2+ channel activity in the endoplasmic reticulum. J. Biol. Chem. 283, 15946–15955. doi: 10.1074/jbc.m800075200
Lamb, H. K., Mee, C., Xu, W., Liu, L., Blond, S., Cooper, A., et al. (2006). The affinity of a major Ca2+ binding site on GRP78 is differentially enhanced by ADP and ATP. J. Biol. Chem. 281, 8796–8805. doi: 10.1074/jbc.m503964200
Li, G. L., Brodin, G., Farooque, M., Funa, K., Holtz, A., Wang, W. L., et al. (1996). Apoptosis and expression of Bcl-2 after compression trauma to rat spinal cord. J. Neuropathol. Exp. Neurol. 55, 280–289. doi: 10.1097/00005072-199603000-00003
Li, G. L., Farooque, M., and Olsson, Y. (2000). Changes of Fas and Fas ligand immunoreactivity after compression trauma to rat spinal cord. Acta Neuropathol. 100, 75–81. doi: 10.1007/s004010051195
Liu, J., Liu, Z., Liu, G., Gao, K., Zhou, H., Zhao, Y., et al. (2020). Spinal cord injury and its underlying mechanism in rats with temporal lobe epilepsy. Exp. Ther. Med. 19, 2103–2112.
Locovei, S., Bao, L., and Dahl, G. (2006). Pannexin 1 in erythrocytes: function without a gap. Proc. Natl. Acad. Sci. U.S.A. 103, 7655–7659. doi: 10.1073/pnas.0601037103
Lukic-Panin, V., Kamiya, T., Zhang, H., Hayashi, T., Tsuchiya, A., Sehara, Y., et al. (2007). Prevention of neuronal damage by calcium channel blockers with antioxidative effects after transient focal ischemia in rats. Brain Res. 1176, 143–150. doi: 10.1016/j.brainres.2007.07.038
Martins, I., Wang, Y., Michaud, M., Ma, Y., Sukkurwala, A. Q., Shen, S., et al. (2014). Molecular mechanisms of ATP secretion during immunogenic cell death. Cell Death Differ. 21, 79–91. doi: 10.1038/cdd.2013.75
Michalski, K., Syrjanen, J. L., Henze, E., Kumpf, J., Furukawa, H., and Kawate, T. (2020). The Cryo-EM structure of pannexin 1 reveals unique motifs for ion selection and inhibition. Elife 9:e54670.
Orellana, J. A., Montero, T. D., and von Bernhardi, R. (2013). Astrocytes inhibit nitric oxide-dependent Ca(2+) dynamics in activated microglia: involvement of ATP released via pannexin 1 channels. Glia 61, 2023–2037. doi: 10.1002/glia.22573
Panchin, Y., Kelmanson, I., Matz, M., Lukyanov, K., Usman, N., and Lukyanov, S. (2000). A ubiquitous family of putative gap junction molecules. Curr. Biol. 10, R473–R474.
Pelegrin, P., and Surprenant, A. (2006). Pannexin-1 mediates large pore formation and interleukin-1beta release by the ATP-gated P2X7 receptor. EMBO J. 25, 5071–5082. doi: 10.1038/sj.emboj.7601378
Penuela, S., Bhalla, R., Gong, X. Q., Cowan, K. N., Celetti, S. J., Cowan, B. J., et al. (2007). Pannexin 1 and pannexin 3 are glycoproteins that exhibit many distinct characteristics from the connexin family of gap junction proteins. J. Cell Sci. 120(Pt 21), 3772–3783. doi: 10.1242/jcs.009514
Rogers, C., Fernandes-Alnemri, T., Mayes, L., Alnemri, D., Cingolani, G., and Alnemri, E. S. (2017). Cleavage of DFNA5 by caspase-3 during apoptosis mediates progression to secondary necrotic/pyroptotic cell death. Nat. Commun. 8:14128.
Shen, Z. Y., Shen, W. Y., Chen, M. H., Shen, J., Cai, W. J., and Yi, Z. (2002). Nitric oxide and calcium ions in apoptotic esophageal carcinoma cells induced by arsenite. World J. Gastroenterol. 8, 40–43. doi: 10.3748/wjg.v8.i1.40
Tan, Y., Dourdin, N., Wu, C., De Veyra, T., Elce, J. S., and Greer, P. A. (2006). Ubiquitous calpains promote caspase-12 and JNK activation during endoplasmic reticulum stress-induced apoptosis. J. Biol. Chem. 281, 16016–16024. doi: 10.1074/jbc.m601299200
Thompson, R. J., Zhou, N., and MacVicar, B. A. (2006). Ischemia opens neuronal gap junction hemichannels. Science 312, 924–927. doi: 10.1126/science.1126241
Ueno, M., and Yamashita, T. (2008). Strategies for regenerating injured axons after spinal cord injury – insights from brain development. Biologics 2, 253–264. doi: 10.2147/btt.s2715
Vanden Abeele, F., Bidaux, G., Gordienko, D., Beck, B., Panchin, Y. V., Baranova, A. V., et al. (2006). Functional implications of calcium permeability of the channel formed by pannexin 1. J. Cell Biol. 174, 535–546. doi: 10.1083/jcb.200601115
Xu, C., Xu, W., Palmer, A. E., and Reed, J. C. (2008). BI-1 regulates endoplasmic reticulum Ca2+ homeostasis downstream of Bcl-2 family proteins. J. Biol. Chem. 283, 11477–11484. doi: 10.1074/jbc.m708385200
Yang, D., He, Y., Muñoz-Planillo, R., Liu, Q., and Núñez, G. (2015). Caspase-11 requires the pannexin-1 channel and the purinergic P2X7 pore to mediate pyroptosis and endotoxic shock. Immunity 43, 923–932. doi: 10.1016/j.immuni.2015.10.009
Yang, Y., Delalio, L. J., Best, A. K., Macal, E., Milstein, J., Donnelly, I., et al. (2020). Endothelial pannexin 1 channels control inflammation by regulating intracellular calcium. J. Immunol. 204, 2995–3007. doi: 10.4049/jimmunol.1901089
Yong, C., Arnold, P. M., Zoubine, M. N., Citron, B. A., Watanabe, I., Berman, N. E., et al. (1998). Apoptosis in cellular compartments of rat spinal cord after severe contusion injury. J. Neurotrauma 15, 459–472. doi: 10.1089/neu.1998.15.459
Keywords: pannexin-1 channels, spinal cord injury, apoptosis, Ca2+ influx, neurocytes
Citation: Huang Y, Lin J, Chen X and Lin J (2021) Pannexin-1 Contributes to the Apoptosis of Spinal Neurocytes in Spinal Cord Injury. Front. Physiol. 12:656647. doi: 10.3389/fphys.2021.656647
Received: 22 January 2021; Accepted: 06 April 2021;
Published: 27 April 2021.
Edited by:
Guido Santos-Rosales, University Hospital Erlangen, GermanyReviewed by:
Juan C. Saez, Pontificia Universidad Católica de Chile, ChileCopyright © 2021 Huang, Lin, Chen and Lin. This is an open-access article distributed under the terms of the Creative Commons Attribution License (CC BY). The use, distribution or reproduction in other forums is permitted, provided the original author(s) and the copyright owner(s) are credited and that the original publication in this journal is cited, in accordance with accepted academic practice. No use, distribution or reproduction is permitted which does not comply with these terms.
*Correspondence: Jianhua Lin, amlhbmh1YWxAMTI2LmNvbQ==
Disclaimer: All claims expressed in this article are solely those of the authors and do not necessarily represent those of their affiliated organizations, or those of the publisher, the editors and the reviewers. Any product that may be evaluated in this article or claim that may be made by its manufacturer is not guaranteed or endorsed by the publisher.
Research integrity at Frontiers
Learn more about the work of our research integrity team to safeguard the quality of each article we publish.