- 1Department of Neurosurgery, University Hospital Zurich, University of Zurich, Zurich, Switzerland
- 2Clinical Neuroscience Center, University Hospital Zurich, University of Zurich, Zurich, Switzerland
- 3Department of Neurosurgery, Careggi University Hospital, University of Florence, Florence, Italy
- 4Department of Neurology, University Hospital Zurich, University of Zurich, Zurich, Switzerland
- 5Department of Neuroradiology, University Hospital Zurich, University of Zurich, Zurich, Switzerland
Introduction: Wallerian degeneration and diaschisis are considered separate remote entities following ischemic stroke. They may, however, share common neurophysiological denominators, since they are both related to disruption of fiber tracts and brain atrophy over time. Therefore, with advanced multimodal neuroimaging, we investigate Wallerian degeneration and its association with diaschisis.
Methods: In order to determine different characteristics of Wallerian degeneration, we conducted examinations on seventeen patients with chronic unilateral ischemic stroke and persisting large vessel occlusion, conducting high-resolution anatomical magnetic resonance imaging (MRI) and blood oxygenation-level dependent cerebrovascular reactivity (BOLD-CVR) tests, as well as Diamox 15(O)–H2O–PET hemodynamic examinations. Wallerian degeneration was determined using a cerebral peduncle asymmetry index (% difference of volume of ipsilateral and contralateral cerebral peduncle) of more than two standard deviations away from the average of age-matched, healthy subjects (Here a cerebral peduncle asymmetry index > 11%). Diaschisis was derived from BOLD-CVR to assess the presence of ipsilateral thalamus diaschisis and/or crossed cerebellar diaschisis.
Results: Wallerian degeneration, found in 8 (47%) subjects, had a strong association with ipsilateral thalamic volume reduction (r2 = 0.60) and corticospinal-tract involvement of stroke (p < 0.001). It was also associated with ipsilateral thalamic diaschisis (p = 0.021), No cerebral peduncular hemodynamic differences were found in patients with Wallerian degeneration. In particular, no CBF decrease or BOLD-CVR impairment was found.
Conclusion: We show a strong association between Wallerian degeneration and ipsilateral thalamic diaschisis, indicating a structural pathophysiological relationship.
Introduction
Ischemic stroke can lead to neurophysiological and structural brain tissue changes, distant from the acute, primary focal lesion. These changes can be seen as post-stroke phenomena, known as Wallerian degeneration and diaschisis (i.e., crossed cerebellar diaschisis and ipsilateral thalamic diaschisis).
Wallerian degeneration is a secondary retrograde degeneration of descending fiber tracts or anterograde trans-synaptic degeneration after acute ischemic stroke and is considered a pure structural phenomenon (Zhang et al., 2012). Diaschisis on the other hand, encompasses remote neurophysiological changes, caused by neuronal deactivation, due to a supratentorial lesion (e.g., ischemic stroke). Although both have a similar pathophysiological origin (i.e., disruption of fiber tracts), the main imaging feature of Wallerian degeneration is structural change (i.e., atrophy), whereas diaschisis displays functional–i.e., neurophysiological–changes, such as a local reduction in cerebral blood flow and hypometabolism (Baron et al., 1981; Pantano et al., 1986; Sebök et al., 2018; van Niftrik et al., 2019). In addition, we have recently reported novel hemodynamic and structural imaging aspects related to diaschisis. Both ipsilateral thalamic diaschisis as well as crossed cerebellar diaschisis could be well detected with novel blood oxygenation-level dependent cerebrovascular reactivity (BOLD-CVR) (Sebök et al., 2018; van Niftrik et al., 2019). Ipsilateral thalamic diaschisis exhibited impaired BOLD-CVR in and thalamic atrophy whereas presence of crossed cerebellar diaschisis detected with BOLD-CVR showed an association with hemodynamic impairment, thereby further supporting the concept of a vascular component (Sebök et al., 2021; van Niftrik et al., 2019; von Bieberstein et al., 2020).
From a time course perspective, Wallerian degeneration can be detected as soon as a couple of days in young children (Mazumdar et al., 2003), whereas for adults it is usually first detected 2–3 weeks after the initial stroke. Diaschisis, on the other hand, may be found within hours or even minutes (Kamouchi et al., 2004; Zhang et al., 2012), and may even “disappear” over time (Feeney and Baron, 1986). This may, in some cases, be related to timely blood flow restoration (Sobesky et al., 2005). Interestingly, brain regions, such as the cerebellum and thalamus, where diaschisis persists 2–3 weeks after a stroke also show atrophy, thereby further indicating that both phenomena share a somewhat common pathophysiological pathway related to fibertract disruption (Tien and Ashdown, 1992; van Niftrik et al., 2019). However, the aforementioned local hemodynamic changes in diaschisis have not been studied for Wallerian degeneration.
Therefore, to better understand the pathophysiology of Wallerian degeneration, we investigate novel structural and hemodynamic determinants for Wallerian degeneration and its association with diaschisis. In order to achieve this, we quantified atrophy of the cerebral peduncle on high-resolution T1-weighted images of patients with chronic (>3 weeks) anterior circulation stroke, as compared to an age-matched group of subacute stroke patients (<2 weeks) and a healthy control group. Measuring cerebral peduncular atrophy is a good indicator of Wallerian degeneration because its fibers run in parallel and it is localized remotely from all stroke lesions (Mark et al., 2008; Burke et al., 2014). Hemodynamic features were investigated using the combination of BOLD-CVR and Diamox challenged 15O(H2O)-Positron Emission Tomography (PET).
Materials and Methods
Subjects and Ethical Approval
All data can be made available upon request to the corresponding author (BvN). For this study, we considered data from subjects with chronic (>3 weeks) unilateral symptomatic persisting internal carotid artery or middle cerebral artery occlusion or stenosis, who participated in an ongoing prospective BOLD-CVR–Diamox challenged H2O PET comparison study at the University Hospital Zurich. A maximal of 6 weeks was allowed between both measurements. Subjects with any cerebral peduncular, cerebellar or thalamic lesions on initial or follow-up imaging were excluded. Subjects were also excluded when they showcased new neurological symptoms between the BOLD-CVR and Diamox challenged H2O PET as well as endovascular and surgical revascularization between both scans. Prior to enrollment of subjects in the prospective database, the research ethics board of the canton Zurich, Switzerland (KEK-ZH-Nr. 2012-0427) approved this study and informed consent was obtained from all subjects.
To determine cerebral peduncular atrophy, we included age-matched, healthy subjects. These subjects were enrolled based on having a negative history of neurological disease and neurological symptoms. Subsequently, age-matched subjects with acute/subacute stroke (onset of first stroke symptoms < 2 weeks) were also included to assess the validity of manually masking the cerebral peduncle. Subjects with stroke onset between 2 and 3 weeks were excluded due to potential overlap.
This resulted in three study groups:
1. Patients with chronic symptomatic steno-occlusive disease (>3 weeks), i.e., chronic group.
2. Patients with acute/subacute symptomatic steno-occlusive disease (<2 weeks), i.e., acute/subacute group.
3. Healthy cohort.
Experimental Protocol
Assessment of Cerebral Peduncular Atrophy
In all patients, a three dimensional (3D) high-resolution T1-weighted Magnetization Prepared Rapid Acquisition Gradient Echo (MP RAGE) image was acquired. Acquisition parameters of the high resolution T1-weighted MP Rage were: voxel size 0.8 × 0.8 × 1.0 mm3 with a field of view 230 × 230 × 176 mm and a scan matrix of 288 × 288 × 176, TR/TE/TI 2200/5.14/900 ms, flip angle 8°.
The left and right cerebral peduncles were manually masked, based on a slight modification of a previously published method (Figure 1) (Mark et al., 2008). Each high-resolution T1-weighted image was separately uploaded in Iplan software (Brainlab, Erlangen, Germany). The medial border of the cerebral peduncle was a straight line drawn through the first point at the corner of the peduncle and the ventral midbrain, and the second point at the lateral sulcus of the midbrain on axial level. The outer margin was then outlined. If any of these structures were not possible to discriminate, the outline on the adjacent layer (either upper or lower) was used as a reference in order to draw the shape. The lower boundary of the cerebral peduncular mask was the ponto-mesencephalic junction, whereas the upper boundary was defined by the mammillary body. The area of the whole cerebral peduncle was then checked in a sagittal plane. These maps were verified by an experienced neuroradiologist, blinded toward the patient groups.
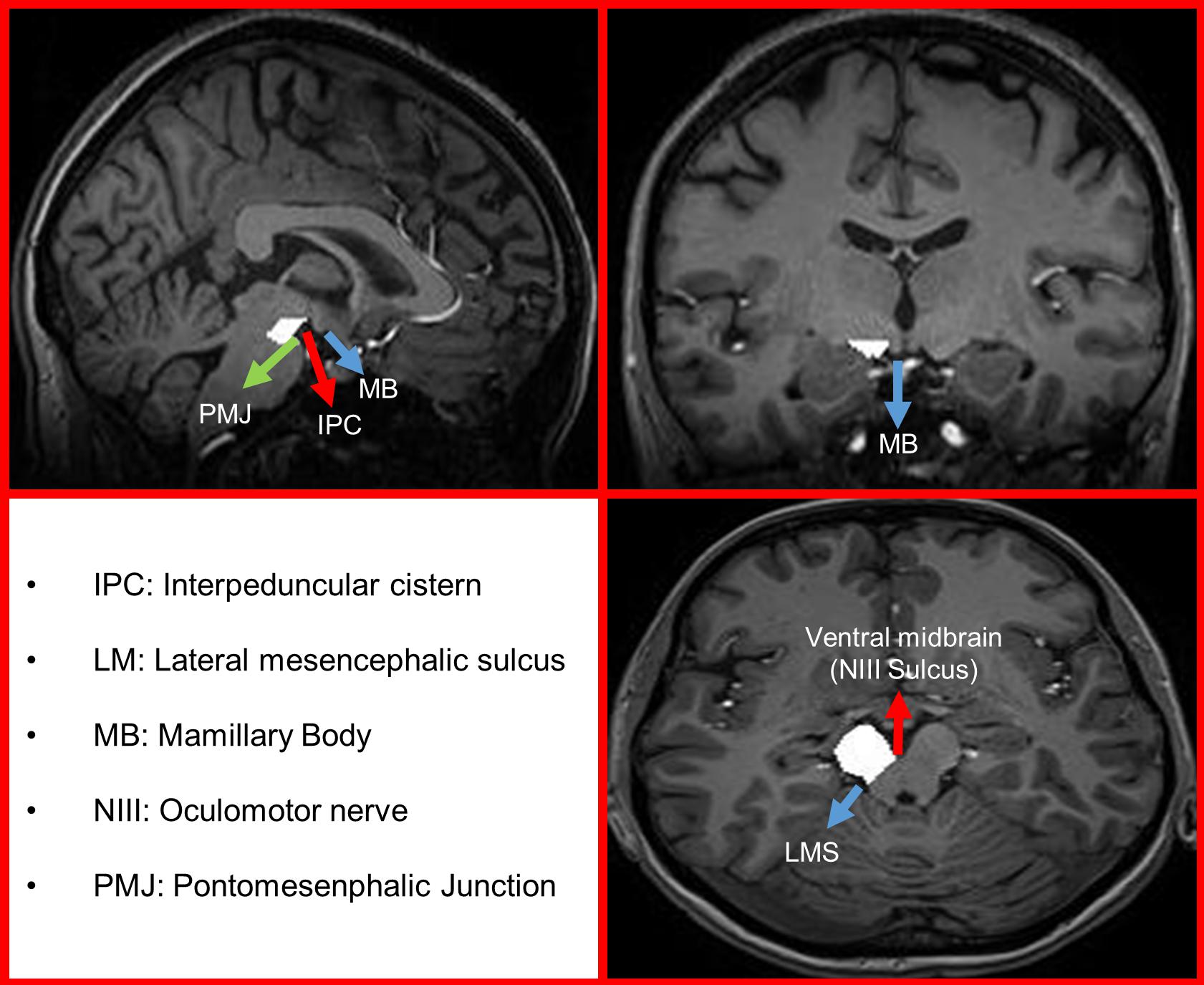
Figure 1. Examplary image of manual masking of a right cerebellar peduncle following Mark et al. (2008).
The cerebral peduncular asymmetry index was calculated as follows:
Wallerian degeneration was deemed present if the volumetric measurements asymmetry index of the cerebral peduncle was more than plus two standard deviations larger than that of the healthy cohort. An index rather than the quantitative value was chosen, to correct for anatomical differences between the subjects.
BOLD-CVR and (15O)-H2O PET Study
MRI: magnetic resonance imaging data were acquired on a 3-tesla Skyra VD13 (Siemens Healthcare, Erlangen, Germany) with a 32-channel head coil. All BOLD fMRI: functional magnetic resonance imaging scans were acquired with the following parameters: axial two-dimensional (2D) single-shot echo-planar imaging sequence planned on the anterior commissure-posterior commissure line plus 20° (on a sagittal image). The voxel size for the BOLD fMRI: functional magnetic resonance imaging scans was 3 × 3 × 3 mm3 with an acquisition matrix 64 × 64 × 35 ascending interleaved slice acquisition, slice gap 0.3 mm, GRAPPA factor 2 with 32 ref. lines, repetition time (TR)/echo time (TE) 2,000/30 ms, flip angle 85°, bandwidth 2,368 Hz/Px and a field of view 192 × 192 mm2. PET data were acquired on a full ring PET/CT-scanner in 3D mode (PET/CT Discovery STE, GE Healthcare, Chicago, IL, United States). The acquisition parameters and method of processing of the all images is extensively described in previous work (van Niftrik et al., 2017, 2018; Fierstra et al., 2018; Sebök et al., 2018).
To determine BOLD-CVR, we deployed a short, controlled hypercapnic stimulus of ∼10 mmHg above resting CO2 levels using the RespiractTM (Slessarev et al., 2007).
Blood oxygenation-level dependent cerebrovascular reactivity, defined as the percentage BOLD signal change per mmHg CO2 change, was calculated using a linear regression on a voxel-by-voxel basis between the BOLD signal time course and the CO2 time course.
Quantitative BOLD-CVR values were separately determined for the whole brain, ipsilateral supratentorial hemisphere, contralateral supratentorial hemisphere, both thalami, both cerebral peduncles, and both cerebellar hemispheres. For the cerebellum and thalamus, patient individual masks were separately extracted from the subjects’ specific subcortical anatomic parcellation, using Freesurfer software1. The technical details of these procedures are described in prior publications (Dale et al., 1999; Fischl et al., 2004). For the healthy cohort, a similar BOLD-CVR analysis was done. Average and standard deviations were calculated, of the cerebellar and thalamic asymmetry indices of the healthy control group (Sebök et al., 2021). Diaschisis (i.e., crossed cerebellar diaschisis and ipsilateral thalamic diaschisis) was deemed present if the asymmetry index in BOLD-CVR was more than plus two standard deviations larger than that of the healthy cohort average (Sebök et al., 2018; van Niftrik et al., 2019).
Concerning H2O–PET imaging, due to its qualitative nature, only difference analyses were conducted, which require hemispheric comparison rather than quantitative measurements (Jiang et al., 2010; Fierstra et al., 2018). Supratentorial hemodynamic status was evaluated as the hemispheric difference of the H2O PET image before and after Diamox (i.e., PET baseline and PET Diamox, respectively) and PET CVR (percent difference PET baseline and Diamox).
Stroke Volume and Location
Using Iplan software (Brainlab, Erlangen, Germany), stroke lesions were manually outlined with Diffusion weighted imaging, T2-Fluid-attentuated-inversion recovery imaging and T1-weighted imaging. In case of multiple stroke lesions, the total stroke volume would embody the sum of all stroke lesions combined. The primary manual masking of the strokes was done using DWI and FLAIR weighted images and then checked on the high-resolution T1 weighted image to correct potential extramarginal masking on lower resolution imaging. Based on the T1-weighted imaging properties, stroke volumes were calculated as the sum of the number of voxels.
Each lesion was also separately analyzed for corticospinal tract involvement. Using Freesurfer free segmentation software (see text footnote 1) (Fischl et al., 2004), the binary masks of the patient individual paracentral lobule and precentral gyrus (gray and white matter) were extracted, and this software was also used to get the individual patient maps for the cerebellum and thalamus. The binary stroke volume map was used as an overlay and corticospinal tract involvement was considered present if the stroke lesion coincided with these regions.
Statistical Analysis
We performed the statistical analysis using in-house scripts written in Matlab R2016b (the MathWorks, Inc., Natick, Massachusetts, United States2). First, evaluation of normal distribution per variable was done using the Shapiro-Wilk test. Averages of normally distributed, continuous variables from the group exhibiting Wallerian degeneration and the group without Wallerian degeneration were compared by an independent Student’s two tailed t-test, where p < 0.05 was considered significant. Non-normal distributed, categorical ordinal and dichotomous variables were analyzed with the Mann–Whitney-U test. All normally distributed, continuous variables are reported as mean ± standard deviation. Non-normally distributed variables, as well as categorical ordinal variables are presented as median (interquartile range), whereas dichotomous variables are shown as frequency (%, percentage).
To test the relationship of Wallerian degeneration with factors related to long-term volumetric and hemodynamic measurements related to diaschisis, a correlation analysis was done. For normal distributed variables, a Pearson correlation was performed. Other variables were studied using the Spearman Rank-order Correlation. Here, a p < 0.05 was deemed significant.
Results
Seventeen patients with chronic stroke (>3 weeks) met the inclusion criteria and were included for further analysis. Thirteen subjects with acute/subacute stroke and 17 age-matched, healthy subjects were included. The mean age was 58 ± 5 years and 70% of the participants were men. The mean time delay between BOLD-CVR and PET measurements was 16 ± 22 days with a maximum of 38 days. The relevant baseline characteristics of all healthy subjects are shown in Table 1, while all relevant characteristics of all patients with chronic stroke are shown in Table 2. No significant difference between subjects with acute/subacute stroke in baseline parameters and stroke volume was found.
Structural Features of Wallerian Degeneration
Volumetric measurements of the left and right cerebral peduncle in healthy subjects resulted in 2.08 ± 0.29 cm3 for the right cerebral peduncle and 2.11 ± 0.33 cm3 for the left peduncle. Consequently, the peduncular asymmetry index for healthy subjects was 1.0 ± 5.0%. Wallerian degeneration was then considered present in patients having a peduncular asymmetry index larger than 11% (i.e., exceeding the mean cerebral peduncular asymmetry index of the healthy control group by plus two (2 × 5) standard deviations, see section “Materials and Methods”). In the chronic group, eight (47%) had cerebral peduncular asymmetry indices larger than 11% and were classified as having Wallerian degeneration. In comparison, in the acute/subacute group, none of the cerebral peduncular asymmetry indices reached 11%. An exemplary patient with Wallerian degeneration is depicted in Figure 2.
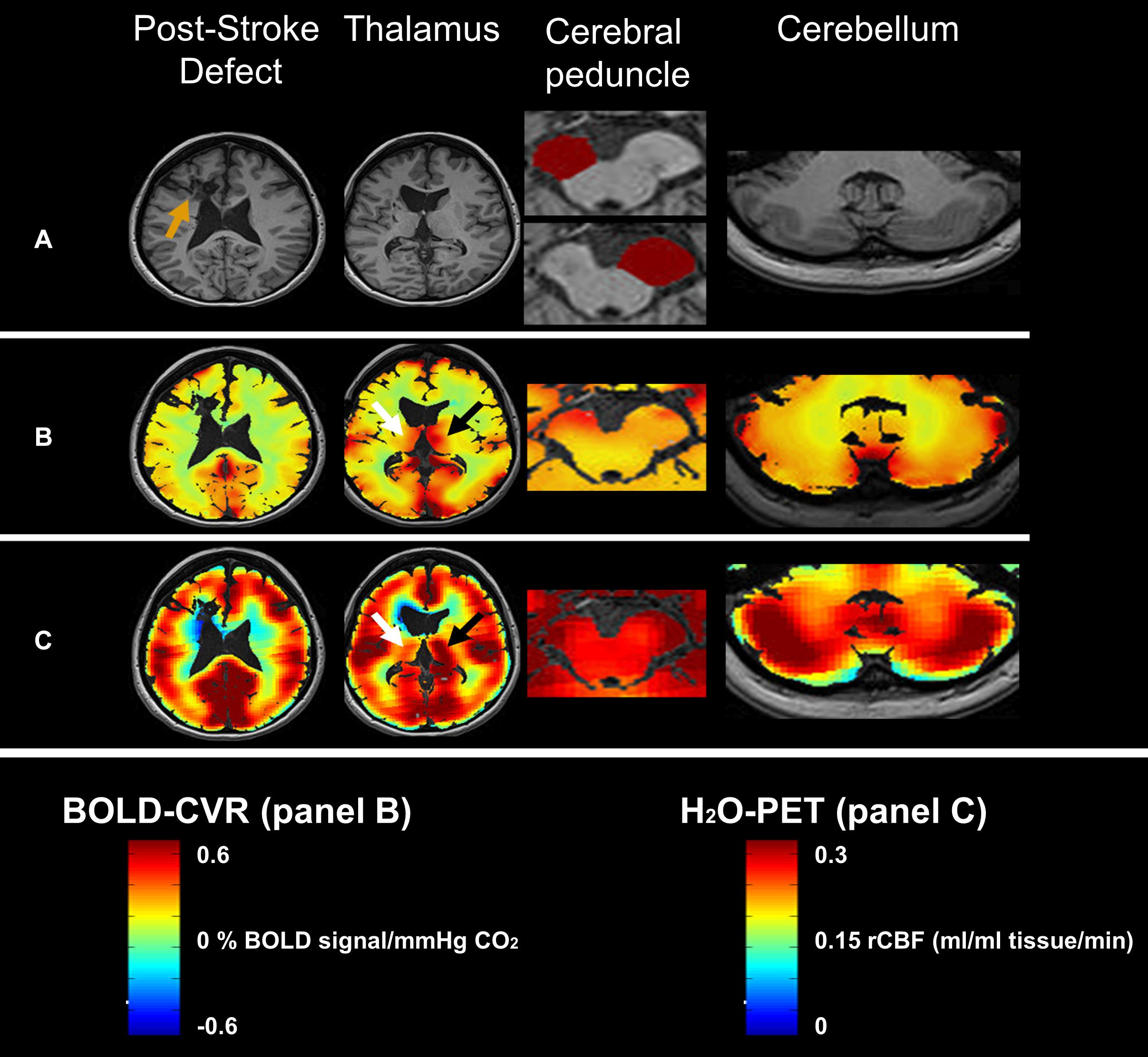
Figure 2. Illustrative image of a 39-year old female patient with Wallerian degeneration (91 weeks post-stroke). Panel (A) shows the T1 image of the post-stroke defect (orange arrow), thalamus, left and right cerebral peduncle masks and cerebellum. Note the large difference between the ipsilateral (top: 1.21 cm3) and contralateral cerebral peduncle (bottom: 1.65 cm3) with a cerebral peduncle asymmetry index of −37%. Panel (B) shows the equivalent BOLD-CVR images. Ipsilateral thalamic diaschisis can be seen (white arrow vs black arrow) as a asymmetry in BOLD-CVR (i.e. ipsilateral decrease). No clear BOLD-CVR asymmetry was seen in the cerebral peduncle or cerebellum. Panel (C) shows the equivalent 15O-H2O-PET cerebral blood flow images. Here also, thalamic asymmetry can be appreciated without blood flow differences in the cerebral peduncles or cerebellum. Abbreviations: BOLD: blood oxygenation-level dependent, cm: cubic centimeter, min: minute, ml: milliliter, mmHg: millimeter mercury, PET- Positron Emission Tomography, rCBF: relative cerebral blood flow.
On average, chronic patients had a markedly lower ipsilateral cerebral peduncular volume in comparison to healthy subjects (1.75 ± 0.33 cm3, p = 0.003), which was more pronounced in the patients with Wallerian degeneration (1.52 ± 0.26 cm3, p > 0.001) and not found in the patients without Wallerian degeneration (1.98 ± 0.21 cm3, p = 0.25). Between patients with and without Wallerian Degeneration, only the volume of the ipsilateral cerebral peduncle differed significantly (p = 0.002).
No volumetric differences in the cerebral peduncles were found between healthy subjects, acute/subacute patients and chronic patients without Wallerian degeneration.
Interestingly, stroke volume did not differ between patients with or without Wallerian degeneration; however, as may be expected, the corticospinal tract involvement within the stroke location was seen more often in the group with Wallerian degeneration.
Hemodynamic Features of Wallerian Degeneration
Mean BOLD-CVR of the healthy cohort for the left and right peduncle was 0.19 ± 0.08, compared to the mean CVR of 0.16 ± 0.09 (p = 0.21) for the ipsilateral cerebral peduncle, and a mean of 0.17 ± 0.10 (p = 0.41) for the contralateral cerebral peduncle in the chronic stroke patients.
Table 3 shows the hemodynamic features of Wallerian degeneration. Within the cerebral peduncle, BOLD-CVR did not show a difference between chronic stroke patients with Wallerian degeneration and patients without Wallerian degeneration, nor was there a difference in the asymmetry index. Similarly, both the baseline H2O–PET and the acetazolamide challenged PET did not show a difference for the cerebellar peduncle.
Wallerian Degeneration and Diaschisis
Table 3 shows the correlation analysis between Wallerian degeneration and diaschisis. Within the group of chronic patients, ipsilateral thalamic diaschisis was found in nine (53%) patients and crossed cerebellar diaschisis was found in seven (41%). Wallerian degeneration was often found in association with ipsilateral thalamic diaschisis (p = 0.021) and so these patients showed a marked increase in neurophysiological and structural variables associated with the thalamus (e.g., an increase in the BOLD-CVR asymmetry index, as well as an increase in both PET asymmetry indices and an decrease in ipsilateral thalamic volume–see Table 3). Moreover, there was a strong positive correlation between the thalamic volume asymmetry index and cerebral peduncular volume asymmetry index (r = 0.77, r2 = 0.59, p > 0.001–Figure 3), with more than half of the variance explained (r2 = 0.60). As for the association between crossed cerebellar diaschisis and Wallerian degeneration, the BOLD-CVR and PET cerebellar asymmetry indices did not differ between both groups, nor was there a correlation to be found between the cerebellar volume asymmetry index and the cerebral peduncular asymmetry index (r = −0.03, p = 0.93).
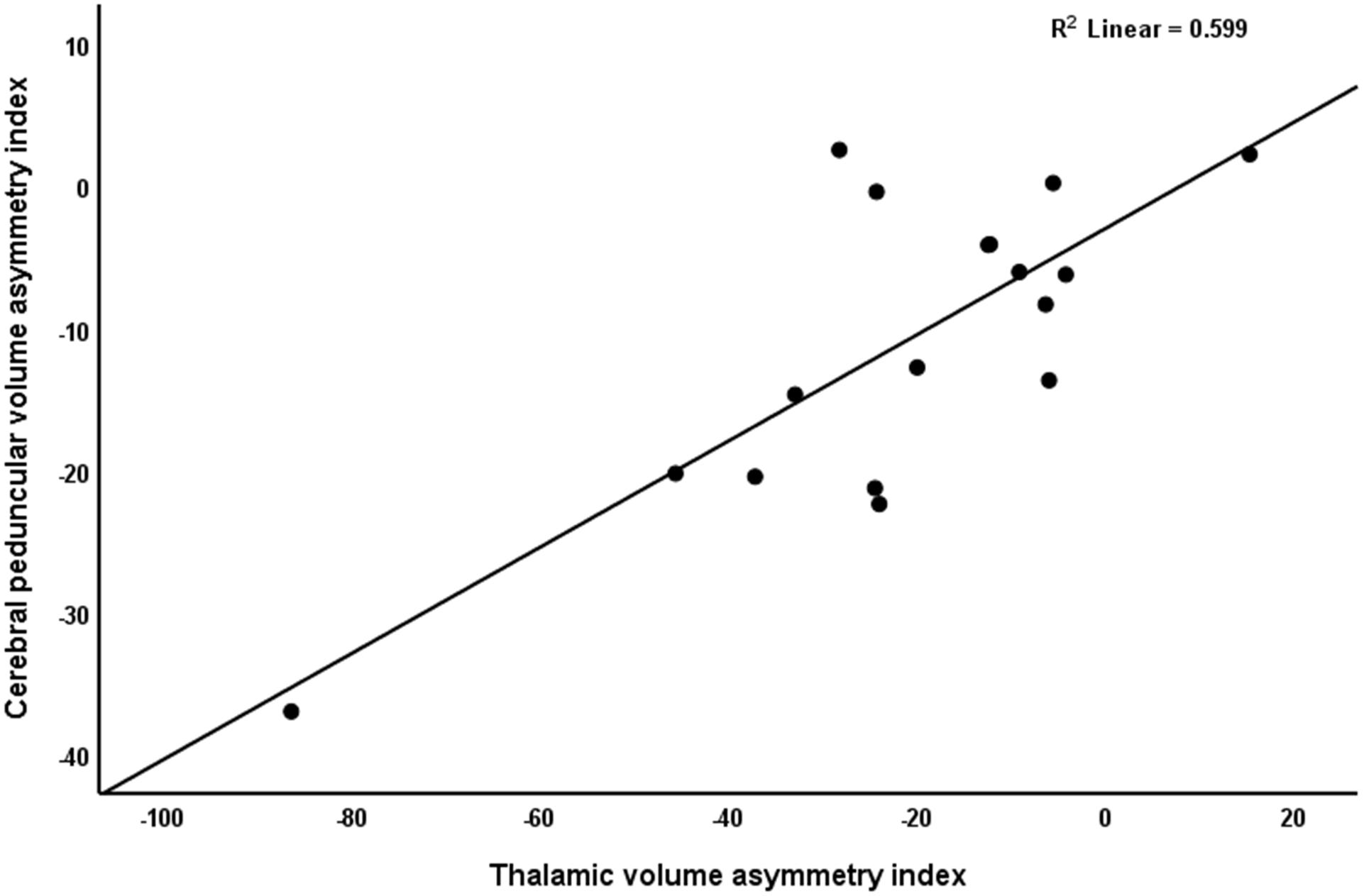
Figure 3. Correlation between cerebral peduncular volume asymmetry index and thalamic volume asymmetry index. Values around zero represent no ipsilateral-contralateral difference. Negative values represent a smaller ipsilateral volume. Note the strong positive relationship between both variables.
Discussion
Main Findings
Wallerian degeneration and diaschisis have a common pathophysiological origin (i.e., disruption of fiber tracts). Moreover, persisting diaschisis also causes atrophy, which is the main feature of Wallerian degeneration, thereby perhaps indicating that both are features of a common pathophysiological pathway. However, local hemodynamic changes commonly seen in diaschisis, as well as its association with diaschisis, have not been studied for Wallerian degeneration. In this study, we show that cerebral peduncles showing Wallerian degeneration, do not display hemodynamic features usually found in diaschisis. In particular, no CBF decrease or BOLD-CVR impairment were found. This may be related to the small sample size of this study. We do, however, show a strong association between Wallerian degeneration and ipsilateral thalamic diaschisis, indicating a structural relationship between both pathophysiological entities.
The Study of Wallerian Degeneration
In 1849, Augustus Waller observed that following transection of the hypoglossal and glossopharyngeal nerve, the distal portion of the nerve undergoes progressive degeneration (Waller, 1850). Initially found in the peripheral nervous system, where Wallerian degeneration takes only days, the existence of Wallerian degeneration was subsequently discovered in the central nervous system, where it occurred in 2–8 days in young children (Mazumdar et al., 2003) and in adults 2–3 weeks after the initial acute stroke (Warabi et al., 1987; Kuhn et al., 1989; Pujol et al., 1990; Castillo and Mukherji, 1999; Uchino et al., 2004; Vargas and Barres, 2007). Our study found a 47% incidence of Wallerian degeneration in the chronic stroke group, which is similar to the incidence reported by previous publications (Pujol et al., 1990; Uchino et al., 2004). In comparison, none of the subjects within the acute/subacute group showed a large enough cerebral peduncle volume asymmetry to surpass the average from healthy subjects by two standard deviations (i.e., was classified as having Wallerian degeneration), indicating our method to be valid for the masking of the cerebral peduncle.
In this study, the presence of Wallerian degeneration was independent of stroke volume, but showed a strong association with corticospinal tract involvement of the stroke lesion. Moreover, we found a strong association between the presence of Wallerian degeneration and neurological outcome. This has also been found by others, but is in disagreement with some historical papers (Schiemanck et al., 2005, 2006; Mark et al., 2008; Schaechter et al., 2009; Cassidy et al., 2018). In earlier work, infarct volume was correlated with motor outcome, but later studies suggested that corticospinal fiber tract involvement of stroke was a stronger predictor for motor outcome (Saver et al., 1999; Schaechter et al., 2009; Burke et al., 2014; Cassidy et al., 2018). Infarct volume in itself is an inexact variable, which does not say anything about the infarct location (Cassidy et al., 2018). Moreover, the predictive value of stroke lesion volume has more merit in the acute/subacute stroke phase (Stinear et al., 2007; Mark et al., 2008; Cassidy et al., 2018). As Wallerian degeneration is seen in patients with strokes involving the corticospinal fiber tract, it has been hypothesized that the association between Wallerian degeneration and worse neurological improvement was mostly due to the extent of the corticospinal tract involvement (Warabi et al., 1990; Liang et al., 2007, 2008; Lindberg et al., 2007; Puig et al., 2010). The corticospinal tract is the largest fiber tract in the cerebral peduncle and has a direct relationship to motor function. However, determining the integrity of the corticospinal tract supratentorially did not show a strong correlation and Wallerian degeneration was subsequently even identified as an independent measurement of motor impairment and greater disability (Mark et al., 2008; Burke et al., 2014). This might be due to the diversity of the corticospinal tract superior to the cerebral peduncle. The corticospinal tract represents the main motor output pathway and portions arise from different areas like the precentral gyrus, premotor cortex, cingulate motor areas and supplementary motor area (Puig et al., 2017). Only taking the portion descending from the precentral gyrus into account, is an oversimplification of the underlying anatomy. Because all these tracts converge and are first aligned in parallel in the cerebral peduncle, the cerebral peduncle may be the most optimal location to test corticospinal integrity as a whole.
The Association With Diaschisis
Diaschisis is a process primarily showing remote neurophysiological changes after a supratentorial lesion (Carrera and Tononi, 2014). Areas with diaschisis experience a decrease in cerebral blood flow, metabolism and BOLD-CVR and can be found directly after the stroke (Baron et al., 1981; De Reuck et al., 1995; Kamouchi et al., 2004; Sebök et al., 2021). These classical hemodynamic features, normally found in diaschisis, were not found in the cerebral peduncle of subjects with Wallerian degeneration. Specifically, no local difference in BOLD-CVR and cerebral blood flow could be detected, indicating a different pathophysiological mechanism for Wallerian degeneration. However, these findings need to be interpreted with caution. Physiologically speaking, a plausible cause for this difference could lie in the fact that white matter fiber tracts are usually much less perfused than other regions, like the thalamus or cerebellum. Smaller changes, in small regions like the cerebral peduncle, would therefore have gone unnoticed. Secondly, the cerebral peduncles are located posterior of the interpeduncular cisterns. It is known that the BOLD signal in regions close to the cerebrospinal fluid can be influenced by artifacts (Thomas et al., 2013). Such artifacts could mask small but significant hemodynamic differences within the cerebral peduncles. This is even more pronounced for H2O–PET imaging, as the resolution of those images is significantly lower than that of BOLD-CVR (see Figure 2). Furthermore, despite that both thalami and cerebellum are perfused by the posterior circulation, anatomical variation may account for variability in thalamic perfusion and BOLD-CVR.
The primary feature of Wallerian degeneration is a reduction in volume, due to degeneration of descending fiber tracts or trans-synaptic degeneration (Zhang et al., 2012). Diaschisis was thought to be a reversible phenomenon, but atrophy of the area presenting long-term diaschisis has also been found (Pantano et al., 1986; Tien and Ashdown, 1992; van Niftrik et al., 2019). This is more clearly discernable in the thalamus of patients with ipsilateral thalamic diaschisis, than it is discernable in the cerebellum of patients with crossed cerebellar diaschisis (van Niftrik et al., 2019). Our data shows a strong association between thalamic volume decrease and cerebral peduncle volume decrease. Such associations were not found for the cerebellum. This makes it more likely that the majority of ipsilateral thalamic diaschisis is due to disruption of the thalamo-cortico or cortico-thalamic tracts or indirect cerebral peduncular loop fibers. It is less likely to be due to the disruption of the afferent cerebellar-pontine-thalamic tracts. Other tracts, like the frontopontine, temporopontine, parietopontine, and occipitopontine fiber tracts, which are associated with crossed cerebellar diaschisis, also project parallel through the cerebral peduncle (Liu et al., 2007; Kamali et al., 2010). However, it is expected that direct injury of only one of those tracts may results in smaller atrophy–potentially not quantifiable–as discrete Wallerian degeneration of those tracts remains absent. This might explain why the presence of crossed cerebellar diaschisis seems to be independent of Wallerian degeneration.
Limitations
In this preliminary study, we have only included 17 subjects with chronic stroke. Therefore, the results should be interpreted with caution. In particular, the small study population most likely resulted in a lack of statistical power in order to sensitively detect hemodynamic differences between Wallerian degeneration and diaschisis. Therefore, a validation study in a larger cohort with correction for other factors should be done. In this study, the cerebral peduncle was manually masked, whereas the cerebellum and thalamus could be masked using patient individual masks obtained from Freesurfer software. This could have resulted in some erroneous measurement. However, as none of the cerebral peduncular asymmetry indices of patients in the acute/subacute stroke group exceeded the threshold of measuring two standard deviations higher than the mean of healthy subjects, we believe the masking was valid. However, optimally, masking should occur without human involvement to get the most unbiased measurements.
Conclusion
Our data indicate a strong association between Wallerian degeneration and ipsilateral thalamic diaschisis, indicating a structural relationship between both pathophysiological entities.
Data Availability Statement
The raw data supporting the conclusions of this article will be made available by the authors, without undue reservation.
Ethics Statement
The studies involving human participants were reviewed and approved by KEK-ZH-Nr. 2012-0427. The patients/participants provided their written informed consent to participate in this study.
Author Contributions
CN, MS, LR, and JF: study the design. CN, MS, GM, SW, and CS: data acquisition. CN, MS, GM, SW, CS, AL, LR, and JF: data analysis and interpretation and manuscript contribution. All authors contributed to the article and approved the submitted version.
Funding
The author(s) disclosed a receipt of the following financial support for the research, authorship, and/or publication of this article. This project was funded by the Clinical Research Priority Program of the University of Zurich (UZH CRPP Stroke) and the Swiss National Science Foundation (PP00P3_170683). JF is also supported by the Swiss Cancer League. Susanne Wegener received funding by the Swiss National Science Foundation (SNSF PP00P3_170683).
Conflict of Interest
The authors declare that the research was conducted in the absence of any commercial or financial relationships that could be construed as a potential conflict of interest.
Footnotes
References
Baron, J. C., Bousser, M. G., Comar, D., and Castaigne, P. (1981). “Crossed cerebellar diaschisis” in human supratentorial brain infarction. Trans. Am. Neurol. Assoc. 105, 459–461.
Burke, E., Dodakian, L., See, J., Mckenzie, A., Riley, J. D., Le, V., et al. (2014). A multimodal approach to understanding motor impairment and disability after stroke. J. Neurol. 261, 1178–1186. doi: 10.1007/s00415-014-7341-8
Carrera, E., and Tononi, G. (2014). Diaschisis: past, present, future. Brain 137, 2408–2422. doi: 10.1093/brain/awu101
Cassidy, J. M., Tran, G., Quinlan, E. B., and Cramer, S. C. (2018). Neuroimaging identifies patients most likely to respond to a restorative stroke therapy. Stroke 49, 433–438. doi: 10.1161/strokeaha.117.018844
Castillo, M., and Mukherji, S. K. (1999). Early abnormalities related to postinfarction Wallerian degeneration: evaluation with MR diffusion-weighted imaging. J. Comput. Assist. Tomogr. 23, 1004–1007. doi: 10.1097/00004728-199911000-00034
Dale, A. M., Fischl, B., and Sereno, M. I. (1999). Cortical surface-based analysis. I. Segmentation and surface reconstruction. Neuroimage 9, 179–194.
De Reuck, J., Decoo, D., Lemahieu, I., Strijckmans, K., Goethals, P., and Van Maele, G. (1995). Ipsilateral thalamic diaschisis after middle cerebral artery infarction. J. Neurol. Sci. 134, 130–135. doi: 10.1016/0022-510x(95)00229-2
Fierstra, J., Van Niftrik, C., Warnock, G., Wegener, S., Piccirelli, M., Pangalu, A., et al. (2018). Staging hemodynamic failure with blood oxygen-level–dependent functional magnetic resonance imaging cerebrovascular reactivity: a comparison versus gold standard (15 O-)H 2 O-positron emission tomography. Stroke. 49, 621–629. doi: 10.1161/STROKEAHA.117.020010
Fischl, B., Salat, D. H., Van Der Kouwe, A. J., Makris, N., Segonne, F., Quinn, B. T., et al. (2004). Sequence-independent segmentation of magnetic resonance images. Neuroimage 23(Suppl. 1) S69–S84.
Jiang, T. T., Videen, T. O., Grubb, R. L. Jr., Powers, W. J., and Derdeyn, C. P. (2010). Cerebellum as the normal reference for the detection of increased cerebral oxygen extraction. J. Cereb. Blood Flow Metab. 30, 1767–1776. doi: 10.1038/jcbfm.2010.43
Kamali, A., Kramer, L. A., Frye, R. E., Butler, I. J., and Hasan, K. M. (2010). Diffusion tensor tractography of the human brain cortico-ponto-cerebellar pathways: a quantitative preliminary study. J. Magn. Reson. Imaging 32, 809–817. doi: 10.1002/jmri.22330
Kamouchi, M., Fujishima, M., Saku, Y., Ibayashi, S., and Iida, M. (2004). Crossed cerebellar hypoperfusion in hyperacute ischemic stroke. J. Neurol. Sci. 225, 65–69. doi: 10.1016/j.jns.2004.07.004
Kuhn, M. J., Mikulis, D. J., Ayoub, D. M., Kosofsky, B. E., Davis, K. R., and Taveras, J. M. (1989). Wallerian degeneration after cerebral infarction: evaluation with sequential MR imaging. Radiology 172, 179–182. doi: 10.1148/radiology.172.1.2740501
Liang, Z., Zeng, J., Liu, S., Ling, X., Xu, A., Yu, J., et al. (2007). A prospective study of secondary degeneration following subcortical infarction using diffusion tensor imaging. J. Neurol. Neurosurg. Psychiatry 78, 581–586. doi: 10.1136/jnnp.2006.099077
Liang, Z., Zeng, J., Zhang, C., Liu, S., Ling, X., Xu, A., et al. (2008). Longitudinal investigations on the anterograde and retrograde degeneration in the pyramidal tract following pontine infarction with diffusion tensor imaging. Cerebrovasc. Dis. 25, 209–216. doi: 10.1159/000113858
Lindberg, P. G., Skejo, P. H., Rounis, E., Nagy, Z., Schmitz, C., Wernegren, H., et al. (2007). Wallerian degeneration of the corticofugal tracts in chronic stroke: a pilot study relating diffusion tensor imaging, transcranial magnetic stimulation, and hand function. Neurorehabil. Neural Repair 21, 551–560. doi: 10.1177/1545968307301886
Liu, Y., Karonen, J. O., Nuutinen, J., Vanninen, E., Kuikka, J. T., and Vanninen, R. L. (2007). Crossed cerebellar diaschisis in acute ischemic stroke: a study with serial SPECT and MRI. J. Cereb. Blood Flow Metab. 27, 1724–1732. doi: 10.1038/sj.jcbfm.9600467
Mark, V. W., Taub, E., Perkins, C., Gauthier, L. V., Uswatte, G., and Ogorek, J. (2008). Poststroke cerebral peduncular atrophy correlates with a measure of corticospinal tract injury in the cerebral hemisphere. AJNR Am. J. Neuroradiol. 29, 354–358. doi: 10.3174/ajnr.a0811
Mazumdar, A., Mukherjee, P., Miller, J. H., Malde, H., and Mckinstry, R. C. (2003). Diffusion-weighted imaging of acute corticospinal tract injury preceding Wallerian degeneration in the maturing human brain. AJNR Am. J. Neuroradiol. 24, 1057–1066.
Pantano, P., Baron, J. C., Samson, Y., Bousser, M. G., Derouesne, C., and Comar, D. (1986). Crossed cerebellar diaschisis. Further Studies. Brain 109(Pt 4), 677–694.
Puig, J., Blasco, G., Schlaug, G., Stinear, C. M., Daunis, I. E. P., Biarnes, C., et al. (2017). Diffusion tensor imaging as a prognostic biomarker for motor recovery and rehabilitation after stroke. Neuroradiology 59, 343–351. doi: 10.1007/s00234-017-1816-0
Puig, J., Pedraza, S., Blasco, G., Daunis, I. E. J., Prats, A., Prados, F., et al. (2010). Wallerian degeneration in the corticospinal tract evaluated by diffusion tensor imaging correlates with motor deficit 30 days after middle cerebral artery ischemic stroke. AJNR Am. J. Neuroradiol. 31, 1324–1330. doi: 10.3174/ajnr.a2038
Pujol, J., Marti-Vilalta, J. L., Junque, C., Vendrell, P., Fernandez, J., and Capdevila, A. (1990). Wallerian degeneration of the pyramidal tract in capsular infarction studied by magnetic resonance imaging. Stroke 21, 404–409. doi: 10.1161/01.str.21.3.404
Saver, J. L., Johnston, K. C., Homer, D., Wityk, R., Koroshetz, W., Truskowski, L. L., et al. (1999). Infarct volume as a surrogate or auxiliary outcome measure in ischemic stroke clinical trials. The RANTTAS investigators. Stroke 30, 293–298. doi: 10.1161/01.str.30.2.293
Schaechter, J. D., Fricker, Z. P., Perdue, K. L., Helmer, K. G., Vangel, M. G., Greve, D. N., et al. (2009). Microstructural status of ipsilesional and contralesional corticospinal tract correlates with motor skill in chronic stroke patients. Hum. Brain Mapp. 30, 3461–3474. doi: 10.1002/hbm.20770
Schiemanck, S. K., Kwakkel, G., Post, M. W., Kappelle, L. J., and Prevo, A. J. (2006). Predicting long-term independency in activities of daily living after middle cerebral artery stroke: does information from MRI have added predictive value compared with clinical information? Stroke 37, 1050–1054. doi: 10.1161/01.str.0000206462.09410.6f
Schiemanck, S. K., Post, M. W., Witkamp, T. D., Kappelle, L. J., and Prevo, A. J. (2005). Relationship between ischemic lesion volume and functional status in the 2nd week after middle cerebral artery stroke. Neurorehabil. Neural Repair 19, 133–138. doi: 10.1177/154596830501900207
Sebök, M., Van Niftrik, C. H. B., Piccirelli, M., Bozinov, O., Wegener, S., Esposito, G., et al. (2018). BOLD cerebrovascular reactivity as a novel marker for crossed cerebellar diaschisis. Neurology 91, e1328–e1337.
Sebök, M., Van Niftrik, C. H. B., Piccirelli, M., Muscas, G., Pangalu, A., Wegener, S., et al. (2021). Crossed cerebellar diaschisis in patients with symptomatic unilateral anterior circulation stroke is associated with hemodynamic impairment in the ipsilateral MCA territory. J. Magn. Reson. Imaging 53, 1190–1197. doi: 10.1002/jmri.27410
Sebök, M., Van Niftrik, C. H. B., Piccirelli, M., Muscas, G., Pangalu, A., Wegener, S., et al. (2021). Crossed cerebellar diaschisis in patients with symptomatic unilateral anterior circulation stroke is associated with hemodynamic impairment in the ipsilateral MCA territory. J. Magn. Reson. Imaging 53, 1190–1197. doi: 10.1002/jmri.27410
Slessarev, M., Han, J., Mardimae, A., Prisman, E., Preiss, D., Volgyesi, G., et al. (2007). Prospective targeting and control of end-tidal CO2 and O2 concentrations. J. Physiol. 581, 1207–1219. doi: 10.1113/jphysiol.2007.129395
Sobesky, J., Thiel, A., Ghaemi, M., Hilker, R. H., Rudolf, J., Jacobs, A. H., et al. (2005). Crossed cerebellar diaschisis in acute human stroke: a PET study of serial changes and response to supratentorial reperfusion. J. Cereb. Blood Flow Metab. 25, 1685–1691. doi: 10.1038/sj.jcbfm.9600162
Stinear, C. M., Barber, P. A., Smale, P. R., Coxon, J. P., Fleming, M. K., and Byblow, W. D. (2007). Functional potential in chronic stroke patients depends on corticospinal tract integrity. Brain 130, 170–180. doi: 10.1093/brain/awl333
Thomas, B. P., Liu, P., Aslan, S., King, K. S., Van Osch, M. J., and Lu, H. (2013). Physiologic underpinnings of negative BOLD cerebrovascular reactivity in brain ventricles. Neuroimage 83, 505–512. doi: 10.1016/j.neuroimage.2013.07.005
Tien, R. D., and Ashdown, B. C. (1992). Crossed cerebellar diaschisis and crossed cerebellar atrophy: correlation of MR findings, clinical symptoms, and supratentorial diseases in 26 patients. AJR Am J Roentgenol. 158, 1155–1159. doi: 10.2214/ajr.158.5.1566683
Uchino, A., Sawada, A., Takase, Y., Egashira, R., and Kudo, S. (2004). Transient detection of early wallerian degeneration on diffusion-weighted MRI after an acute cerebrovascular accident. Neuroradiology 46, 183–188. doi: 10.1007/s00234-003-1159-x
van Niftrik, C. H. B., Piccirelli, M., Bozinov, O., Maldaner, N., Strittmatter, C., Pangalu, A., et al. (2018). Impact of baseline CO2 on Blood-Oxygenation-Level-Dependent MRI measurements of cerebrovascular reactivity and task-evoked signal activation. Magn. Reson. Imaging 49, 123–130. doi: 10.1016/j.mri.2018.02.002
van Niftrik, C. H. B., Piccirelli, M., Bozinov, O., Pangalu, A., Fisher, J. A., Valavanis, A., et al. (2017). Iterative analysis of cerebrovascular reactivity dynamic response by temporal decomposition. Brain Behav. 7:e00705. doi: 10.1002/brb3.705
van Niftrik, C. H. B., Sebok, M., Muscas, G., Piccirelli, M., Serra, C., Krayenbuhl, N., et al. (2019). Characterizing ipsilateral thalamic diaschisis in symptomatic cerebrovascular steno-occlusive patients. J. Cereb. Blood Flow Metab. 40, 563–573. doi: 10.1177/0271678x19830532
Vargas, M. E., and Barres, B. A. (2007). Why is Wallerian degeneration in the CNS so slow? Annu. Rev. Neurosci. 30, 153–179. doi: 10.1146/annurev.neuro.30.051606.094354
von Bieberstein, L., Van Niftrik, C. H. B., Sebök, M., El Amki, M., Piccirelli, M., Stippich, C., et al. (2020). Crossed cerebellar diaschisis indicates hemodynamic compromise in ischemic stroke patients. Transl. Stroke Res. 12, 39–48. doi: 10.1007/s12975-020-00821-0
Waller, A. (1850). Experiments on the section of the glossopharyngeal and hypoglossal nerves of the frog, and observations of the alterations prodced thereby in the structure of their primitive fibers. Philos. Trans. R. Soc. Lond. 140, 423–429. doi: 10.1098/rstl.1850.0021
Warabi, T., Inoue, K., Noda, H., and Murakami, S. (1990). Recovery of voluntary movement in hemiplegic patients. Correlation with degenerative shrinkage of the cerebral peduncles in CT images. Brain 113(Pt 1), 177–189. doi: 10.1093/brain/113.1.177
Warabi, T., Miyasaka, K., Inoue, K., and Nakamura, N. (1987). Computed tomographic studies of the basis pedunculi in chronic hemiplegic patients: topographic correlation between cerebral lesion and midbrain shrinkage. Neuroradiology 29, 409–415. doi: 10.1007/bf00341735
Keywords: blood oxygenation-level dependent, functional magnetic resonance imaging (fMRI), Wallerian degeneration, diaschisis, H2O–PET, cerebrovascular reactivity
Citation: van Niftrik CHB, Sebök M, Muscas G, Wegener S, Luft AR, Stippich C, Regli L and Fierstra J (2021) Investigating the Association of Wallerian Degeneration and Diaschisis After Ischemic Stroke With BOLD Cerebrovascular Reactivity. Front. Physiol. 12:645157. doi: 10.3389/fphys.2021.645157
Received: 22 December 2020; Accepted: 24 May 2021;
Published: 24 June 2021.
Edited by:
James Duffin, University of Toronto, CanadaReviewed by:
Joshua Shimony, Washington University School of Medicine in St. Louis, United StatesAlexandre Krainik, Centre Hospitalier Universitaire de Grenoble, France
Copyright © 2021 van Niftrik, Sebök, Muscas, Wegener, Luft, Stippich, Regli and Fierstra. This is an open-access article distributed under the terms of the Creative Commons Attribution License (CC BY). The use, distribution or reproduction in other forums is permitted, provided the original author(s) and the copyright owner(s) are credited and that the original publication in this journal is cited, in accordance with accepted academic practice. No use, distribution or reproduction is permitted which does not comply with these terms.
*Correspondence: C. H. B. van Niftrik, YmFzLnZhbm5pZnRyaWtAdXN6LmNo