- 1Department of Pharmacology, Wayne State University School of Medicine, Detroit, MI, United States
- 2Center for Molecular Medicine and Genetics, Wayne State University School of Medicine, Detroit, MI, United States
- 3Department of Emergency Medicine, University of Michigan Medical School, Ann Arbor, MI, United States
- 4Office of the Vice President of Research, Wayne State University, Detroit, MI, United States
- 5Institute of Environmental Health Sciences, Wayne State University, Detroit, MI, United States
- 6Department of Obstetrics and Gynecology, Wayne State University, Detroit, MI, United States
Traumatic brain injuries (TBIs) caused by a sudden impact to the head alter behavior and impair physical and cognitive function. Besides the severity, type and area of the brain affected, the outcome of TBI is also influenced by the patient’s biological sex. Previous studies reporting mitochondrial dysfunction mainly focused on exponential reactive oxygen species (ROS) generation, increased mitochondrial membrane potential, and altered mitochondrial dynamics as a key player in the outcome to brain injury. In this study, we evaluated the effect of a near-infrared (NIR) light exposure on gene expression in a Drosophila TBI model. NIR interacts with cytochrome c oxidase (COX) of the electron transport chain to reduce mitochondrial membrane potential hyperpolarization, attenuate ROS generation, and apoptosis. We subjected w1118 male and female flies to TBI using a high-impact trauma (HIT) device and subsequently exposed the isolated fly brains to a COX-inhibitory wavelength of 750 nm for 2 hours (hr). Genome-wide 3′-mRNA-sequencing of fly brains revealed that injured w1118 females exhibit greater changes in transcription compared to males at 1, 2, and 4 hours (hr) after TBI. Inhibiting COX by exposure to NIR downregulates gene expression in injured females but has minimal effect in injured males. Our results suggest that mitochondrial COX modulation with NIR alters gene expression in Drosophila following TBI and the response to injury and NIR exposure varies by biological sex.
Introduction
Traumatic brain injury (TBI) results from a violent blow or jolt to the head causing a wide range of physical and psychological effects (Finnie and Blumbergs, 2002; Ciuffreda et al., 2016; Dale Horne, 2018). There are estimated to be about 2.5 million TBI cases every year that require emergency department visits or result in death (Taylor et al., 2017). The most common causes of TBI include sports, motor vehicle accidents, falls, and violence (Hiebert et al., 2015) with the symptoms of the injury depending on the type and area of the brain affected (Morries et al., 2015). TBI is a heterogenous disorder consisting of primary damage resulting from direct mechanical forces followed by secondary damage centered on mitochondrial dysfunction leading to neuronal death (Cheng et al., 2012).
Mitochondria are membrane-bound organelles distributed throughout the brain cytosol and responsible for energy production using the electron transport chain (ETC) (Kim et al., 2017). The ETC is a series of protein complexes made of NADH dehydrogenase (complex I), succinate dehydrogenase (complex II), ubiquinone, bc1-complex (complex III), cytochrome c (Cytc), and cytochrome c oxidase (COX; complex IV) (Hüttemann et al., 2008). Complexes I, III, and IV pump protons across the inner mitochondrial membrane to generate the mitochondrial membrane potential (ΔΨm) (Hüttemann et al., 2008) which is utilized by ATP synthase (complex V) to synthesize ATP from ADP and phosphate (Hüttemann et al., 2008; Sanderson et al., 2013). An optimal physiological ΔΨm between 120–140 mV allows efficient ATP production and minimal reactive oxygen species (ROS) generation from complexes I and III (Sanderson et al., 2013). Disruption of ΔΨm is considered as an indicator of mitochondrial damage causing decreased respiration, decreased ATP production, increased ROS generation and induction of apoptosis by efflux of macromolecules like Cytc and caspase-9/caspase-3 cascade activation (Singh et al., 2006; Watts, 2016).
Mitochondrial impairment has been shown to play a key role in several neurodegenerative disorders including Alzheimer’s disease (AD), Parkinson’s disease (PD), amyotrophic lateral sclerosis (ALS), ischemic brain injury, and stroke (Reddy, 2009; Sanderson et al., 2013, 2018; Strubakos et al., 2020). Following TBI using a controlled cortical impact model, mitochondrial dysfunction and calcium perturbation was observed in male rats (Xiong et al., 1997). A significant decrease in mitochondrial oxidative phosphorylation and calcium buffering capacity was observed in male mice 3 hr post-TBI (Singh et al., 2006). These mice also show structural damage to isolated mitochondria and an increase in oxidative stress (Singh et al., 2006). A Drosophila model of TBI also showed significant decrease in ATP production which was observed 24 hr after injury (Sen et al., 2017). It is evident that production of ROS, hyperpolarization of ΔΨm beyond the physiological range, and caspase activation induce mitochondrial damage following TBI (Lifshitz et al., 2004; Kim et al., 2017). Additionally, biological sex has been shown to influence mitochondrial function (Demarest and McCarthy, 2015; Ventura-Clapier et al., 2017) and the outcome to TBI (Leitgeb et al., 2011; Gupte et al., 2019) but the role of sex-differences in mitochondrial function in response to TBI has not been studied. In a previous study, we observed sex differences in mitochondrial gene transcription and oxidation in Drosophila subjected to trauma using the high-impact trauma device (HIT-device) (Katzenberger et al., 2015; Shah et al., 2020). Thus, efforts to attenuate mitochondrial damage have been increasingly studied in TBI, as mitochondrial maintenance could possibly preserve brain function (Watts, 2016). However, most pharmacological approaches to circumvent mitochondrial damage suffer from a critical issue: the ability of drugs to cross the blood-brain barrier and attain effective concentrations in the injured tissue (Sanderson et al., 2013).
In the current study, we explore a non-pharmacological approach of preserving mitochondrial damage in TBI using near-infrared (NIR) light. Photobiomodulation (PBM) or the use of NIR has been studied as a therapeutic alternative in animal and human TBI to protect tissue from dying, increase mitochondrial function, improve blood flow, stimulate healing and tissue oxygenation (Naeser et al., 2011; Hamblin, 2018). PBM involves shining red or NIR light onto the head where the light penetrates into the brain and is absorbed by specific chromophores (Hamblin, 2018). NIR has been shown to interact with cytochrome c oxidase (COX), the terminal complex of the ETC (Sanderson et al., 2018; Strubakos et al., 2020). COX contains several chromophores including two copper centers that act as photoacceptors for NIR and absorb light in the range of 700–1000 nm (Karu and Afanas’eva, 1995). Many studies in animal models of TBI using NIR from light-emitting diodes (LEDs) have found improved neurological and cognitive function and reduced inflammation and cell death in brain post-exposure (Naeser et al., 2011; Hamblin, 2018). Drosophila exposed to 670 nm NIR showed increased ATP production and reduced inflammation with age (Begum et al., 2015) whereas irradiating Drosophila pink1 mutants at 808 nm rescued mitochondrial defects (Vos et al., 2013). Recent studies in animal models of stroke and ischemia/reperfusion injury have found that NIR exposure at 750 nm inhibits COX activity (Sanderson et al., 2018), reduces mitochondrial respiratory and ΔΨm, and prevents ROS generation (Sanderson et al., 2018; Strubakos et al., 2020). In line with this evidence, we sought to investigate the effect of modulating mitochondrial COX activity with 750 nm NIR exposure in both sexes of a Drosophila model of mild traumatic brain injury (mTBI). Drosophila brain is enclosed in an exoskeleton consisting of chitin which strongly absorbs NIR and although previous studies found other wavelengths to penetrate fly and mammalian tissues effectively, we found 750 nm NIR penetration through the exoskeleton ineffective. Thus, we isolated fly brains from w1118 male and female flies inflicted with trauma using the HIT-device at control, 1, 2, and 4 hr post-TBI and exposed them to NIR at 750 nm for 2 hr. Post-treatment, we assessed change in gene expression separated by sex and found an overall downregulation in transcription in w1118 females in response to NIR exposure. As compared to w1118 females, we saw fewer changes in w1118 males in response to TBI and NIR exposure. These data suggest that outcome to TBI differs between sexes in Drosophila exposed to NIR, which could be a result of sex-differences in mitochondrial function. This is the first study to investigate the response of mitochondrial COX inhibition using NIR light at a single wavelength in both sexes post-TBI within 4 hr.
Materials and Methods
Fly Stocks and Crosses
w1118 stock were obtained from the Bloomington Drosophila Stock Center and repo-GFP stock was a gift from Dr. Laura Buttitta (University of Michigan). Fly stocks were stored at 25°C at constant humidity and fed with standard sugar/yeast/agar medium. All assays were performed on adult mated flies (10–14 days old).
Traumatic Brain Injury
Both sexes of w1118 and repo-GFP flies were subjected to a single strike full body trauma using a modified HIT device with the impact arm constrained to a 45° angle (Katzenberger et al., 2013; Sen et al., 2017). This device inflicts mild TBI to the flies. No more than 50 flies were placed in a plastic vial before being confined to the bottom quarter of the vial by a stationary cotton ball. Upon deflection and release of the spring, the vial rapidly contacts a styrofoam pad delivering a mechanical force to the flies as they contact the vial wall and rebound causing closed head trauma.
NIR Light Emitting Diodes and Exposure
Diodes (epoxy lens type infrared illuminator LED750-66-60, Roithner Lasertechnik, Vienna, Austria) were mounted on heat sinks (black aluminum, 47 × 20 for LED array 60 chips) together with a small fan (EC3010M05X; Evercool, New Taipei City, Taiwan) operated in reverse mode to avoid any heating of the brain tissue (Sanderson et al., 2018). The diodes were operated with an energy density of 100 mW/cm2. Brains were dissected from control flies or flies exposed to trauma 1, 2, and 4 hr after injury. Approximately 10–15 fly brains were dissected for each condition and placed in a 35 mm petri dish containing cold Schneider’s media. The petri dish was then placed under the 750 nm diode within 2 cm distance and exposed to NIR for 2 hr. Post-exposure, brains were immediately processed for either RNA collection or confocal imaging.
RNA Isolation
Total RNA was extracted from w1118 single fly brains using QIAzol® lysis reagent and Direct-zolTM RNA MicroPrep kit (Zymo Research) following manufacturer’s instructions.
3′-mRNA Expression Analysis
Expression analysis was conducted in collaboration with the Wayne State University Genome Sciences Core. Three biological replicates were used for each condition (Shah et al., 2020).
QuantSeq 3′-mRNA-Seq Library Prep Kit FWD for Illumina (Lexogen) was used to generate libraries of sequences close to the 3′ end of polyadenylated RNA from 15 ng of total RNA isolated from single fly brain in 5 μl of nuclease-free water following the low-input protocol. Library aliquots were assessed for overall quality using the ScreenTape for the Agilent 2200 TapeStation and quantified using QubitTM 1X dsDNA HS Assay kit (Invitrogen). Barcoded libraries were normalized to 2 nM before sequencing at 300 pM on one lane of a NovaSeq 6000 SP flow cell. After de-multiplexing with Illumina’s CASAVA 1.8.2 software, the 50 bp reads were aligned to the Drosophila genome (Build dm3) with STAR_2.4 (Dobin et al., 2013) and tabulated for each gene region (Anders et al., 2015). Differential gene expression analysis was used to compare transcriptome changes between conditions using edgeR v.3.22.3 (Robinson et al., 2010) and transcripts were defined as significantly differentially expressed at absolute log2 fold change (| log2 FC|) > 1 with an false discovery rate (FDR) < 0.05. Significant gene expression changes were submitted for gene ontology (GO) analyses using RDAVID (Fresno and Fernandez, 2013) for the following categories: GOTERM_BP_ALL, GOTERM_MF_ALL, UP_KEYWORDS, GOTERM_BP_DIRECT, and GOTERM_MF_DIRECT.
Heatmaps
Heatmaps were generated using Java Treeview (Saldanha, 2004). Counts representing the number of reads mapped to each gene were obtained using HTSeq (Anders et al., 2015) from STAR alignments (Dobin et al., 2013) before normalization. To normalize, a scaling factor was determined by dividing the uniquely mapped reads for each sample by the sample with the highest uniquely mapped number of reads. The scaling factor was multiplied to each gene count for the sample. The log2 of the normalized averaged counts for all three replicates is represented for each condition on the orange scale (0–10). The log2 fold change, represented on yellow-blue scale (0–6), for each gene is obtained from differential expression analysis across all three replicates (Robinson et al., 2010). Genes significant (| log2 FC| > 1, p-value < 0.05) in at least one time point are indicated in black text.
Confocal Imaging
Between 10–15 adult repo-GFP flies for each sex (control and every time point post-TBI) were inflicted with TBI using the HIT-device (Katzenberger et al., 2013; Shah et al., 2020). Flies were anesthetized with CO2 before brains were dissected in 1×PBS (phosphate-buffered saline) and fixed for 5 min with 4% PFA (paraformaldehyde). Fixed brains were mounted using Prolong gold antifade mounting media to visualize changes in GFP expression using a confocal microscope (Zeiss LSM 800) at the Microscopy, Imaging and Cytometry Resources Core at Wayne State University, School of Medicine. Average fluorescence intensity for all brains in each condition was calculated using ImageJ (Schneider et al., 2012) in a blinded study. All data are represented as means ± SEM. Statistical analyses (One-way ANOVA with Dunnett’s multiple comparisons test) were performed using GraphPad Prism to compute statistical significance (p < 0.05) between groups.
Results
Gene Transcription in TBI Inflicted Flies Is Downregulated After Exposure to NIR
Several studies in experimental models of brain injury have shown structural and functional damage to mitochondria being an early event in TBI which leads to activation of cell death pathways (Fischer et al., 2016). In a previous study, we demonstrated an upregulation in gene transcription involved with immune response, cytoskeleton organization and apoptosis after injury in Drosophila (Shah et al., 2020). Moreover, we have also shown sex-differences in mitochondrial stress and gene transcription in response to TBI (Shah et al., 2020). To identify gene expression changes after attenuation of mitochondria response by COX-inhibitory NIR exposure, we generated 3′-mRNA-Seq libraries from isolated w1118 male and female fly brains at control and 1-, 2-, and 4-hr post-injury (single strike) time points. Differential gene expression analysis shows significant changes in both sexes after TBI and NIR exposure (Figure 1) with females (Figures 1A–C) exhibiting more transcriptional changes than males (Figures 1D–F). Gene expression changes in response to TBI were less pronounced in both sexes exposed to NIR as compared to flies not treated with NIR (Shah et al., 2020) at all 3 time-points (| log FC| > 2; p-value < 0.05).
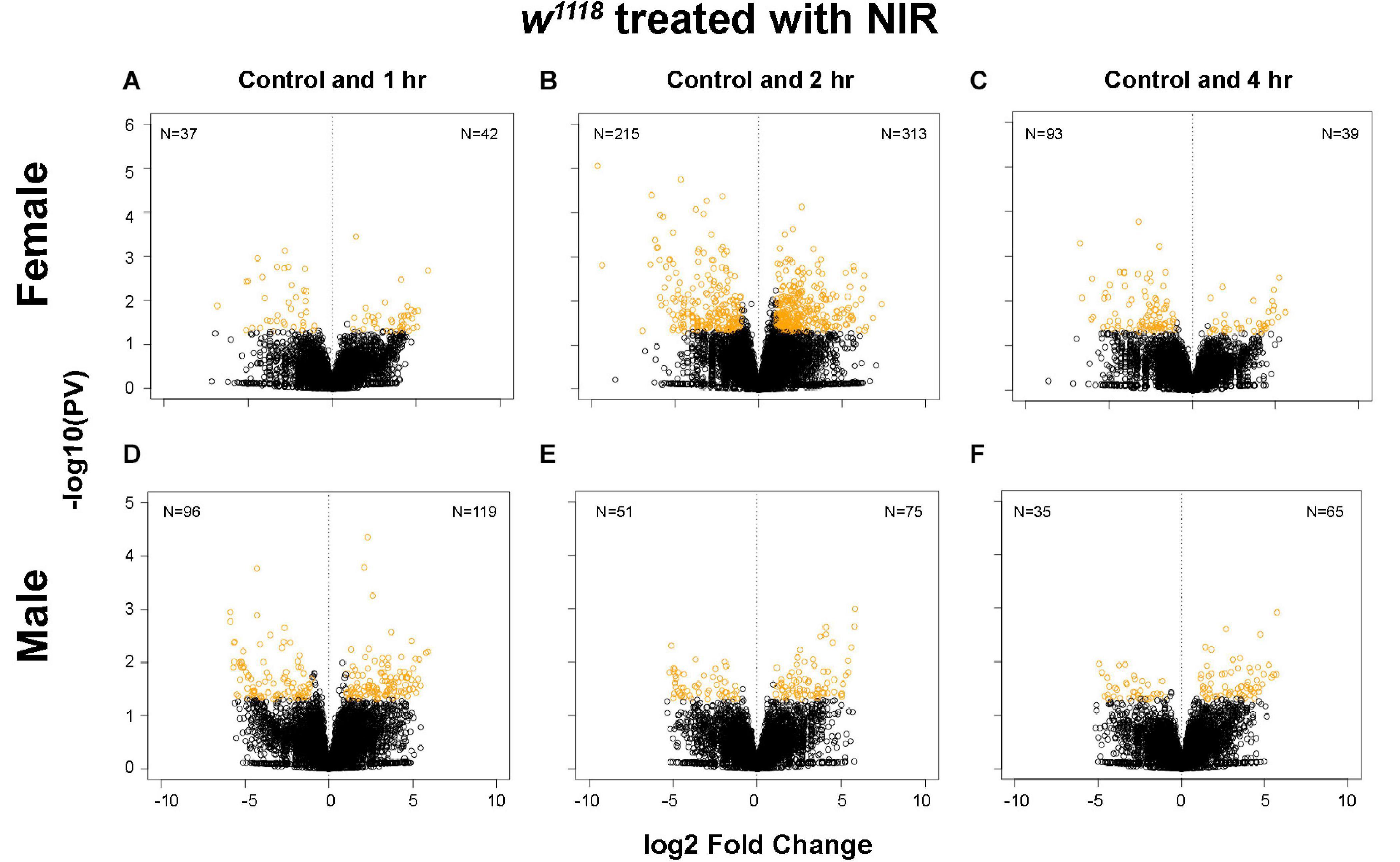
Figure 1. Plot depicting transcriptional changes after NIR exposure in w1118 injured male and female flies. Volcano plots depicting log2 fold change and –log10(PV:p-value) of differentially expressed genes at 1, 2, and 4 hr after injury and subsequent NIR exposure compared to control for females (A–C) and males (D–F). The number of significantly upregulated and downregulated gene changes are indicated in each plot (| log2FC| > 1; p-value < 0.05). Injured w1118 females show more transcriptional changes in response to NIR exposure than males.
Significant genes identified from 3′-mRNA-Seq were classified for their biological functions using RDAVID (Huang et al., 2009; Fresno and Fernandez, 2013) and several GO categories were found to be changed in both sexes exposed to NIR in response to TBI (Figure 2 and Tables 1, 2). A subset of GO categories significantly altered in both sexes are indicated in Figure 2 wherein it is observed that females have more processes affected than males at all three time-points. In w1118 females exposed to NIR, the highest number of significant categories (FDR < 0.05) were altered 2 hr after injury (41 GO terms) (Figure 2A) and we also observed significant changes in GO terms for “Humoral immune response,” “Defense response,” “Response to stress,” and “Detection of light stimulus” (Figure 2A, Table 1, and Supplementary Data 1). In our previous study, we observed GO processes including “Immune response,” “Mitochondrial organization,” and “Programmed cell death” significantly altered after TBI in w1118 females untreated with NIR (Shah et al., 2020). Here, we found no change in any of these processes in females treated with NIR after TBI indicating a positive effect of the COX-inhibitory NIR exposure that could help minimize aberrant gene transcription after brain injury. However, for w1118 males exposed to NIR, there were fewer significant changes observed than for females, and gene expression related to “nervous system development” and “neurogenesis” was altered after NIR exposure (Figure 2B, Table 2, and Supplementary Data 1). Previous studies involving the use of PBM for TBI patients have also reported downregulation of immune response and upregulation of neurogenesis as an effect of NIR treatment (Hennessy and Hamblin, 2017; Santos et al., 2018). There was no overlap in biological processes affected across all three TBI time-points shared by both sexes. Additionally, we also exposed control fly brains to NIR for both sexes and found that female controls had more significant GO enrichment (18 categories) as compared to male controls (7 categories) (Supplementary Data 1). For female controls, GO terms like “Neurogenesis,” “Oxidation-Reduction Process,” and “Intracellular Transport” were altered whereas for male controls “Nuclear Transport” and “Nucleic Acid Metabolic Process” were changed in response to NIR exposure. Neither sexes showed alteration in immune response or mitochondrial organization (Supplementary Data 1).
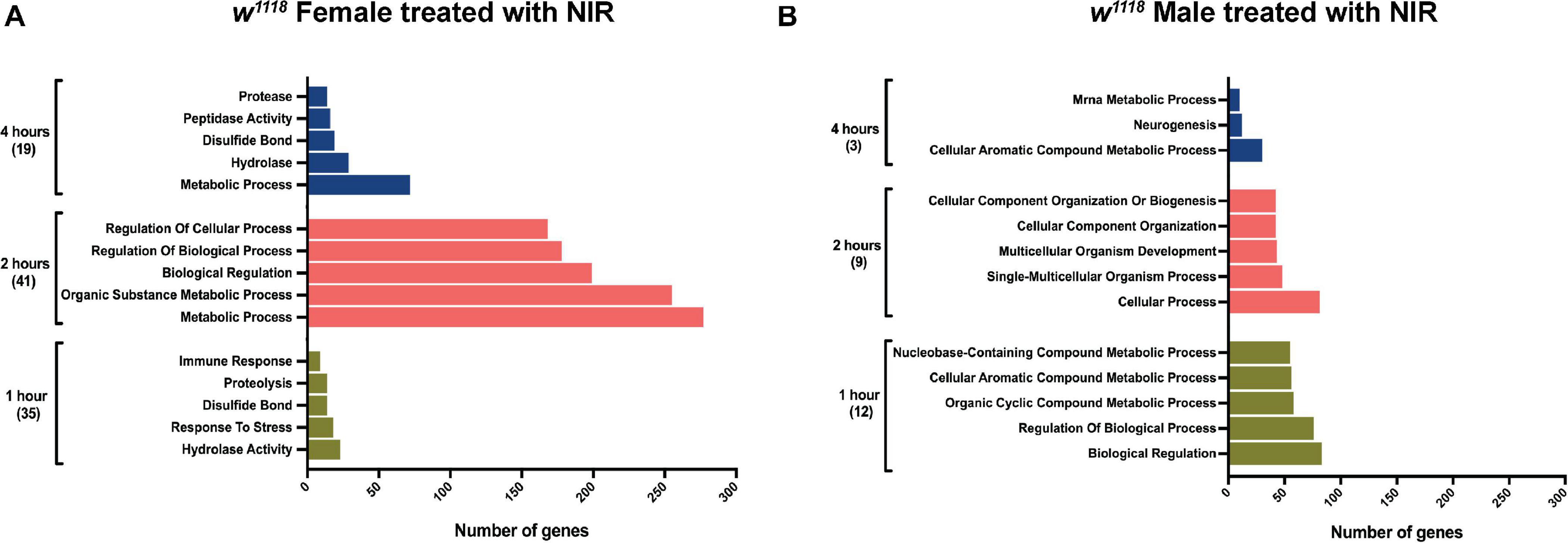
Figure 2. Gene ontology (GO) enrichment in w1118 injured flies exposed to NIR. Significant genes (| log2FC| > 1; p-value < 0.05) identified from sequencing were classified for their biological functions using RDAVID. The plot shows top five biological processes enriched in injured females (A) and males (B) after NIR exposure at each time-point. The total number of GO terms differentially regulated at each time-point is indicated in parenthesis.
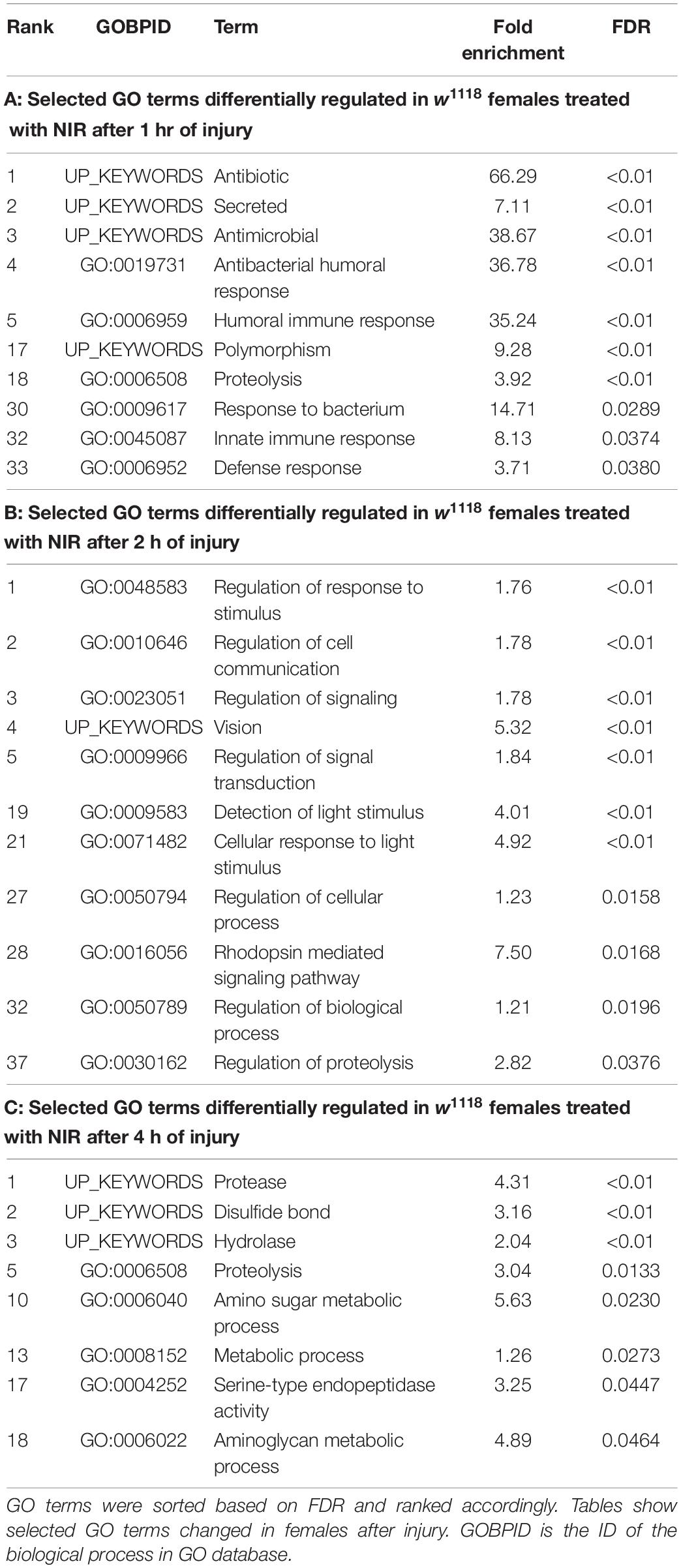
Table 1. Gene ontology terms significantly (FDR < 0.05) changed in response to traumatic brain injury in w1118 female flies treated with NIR.
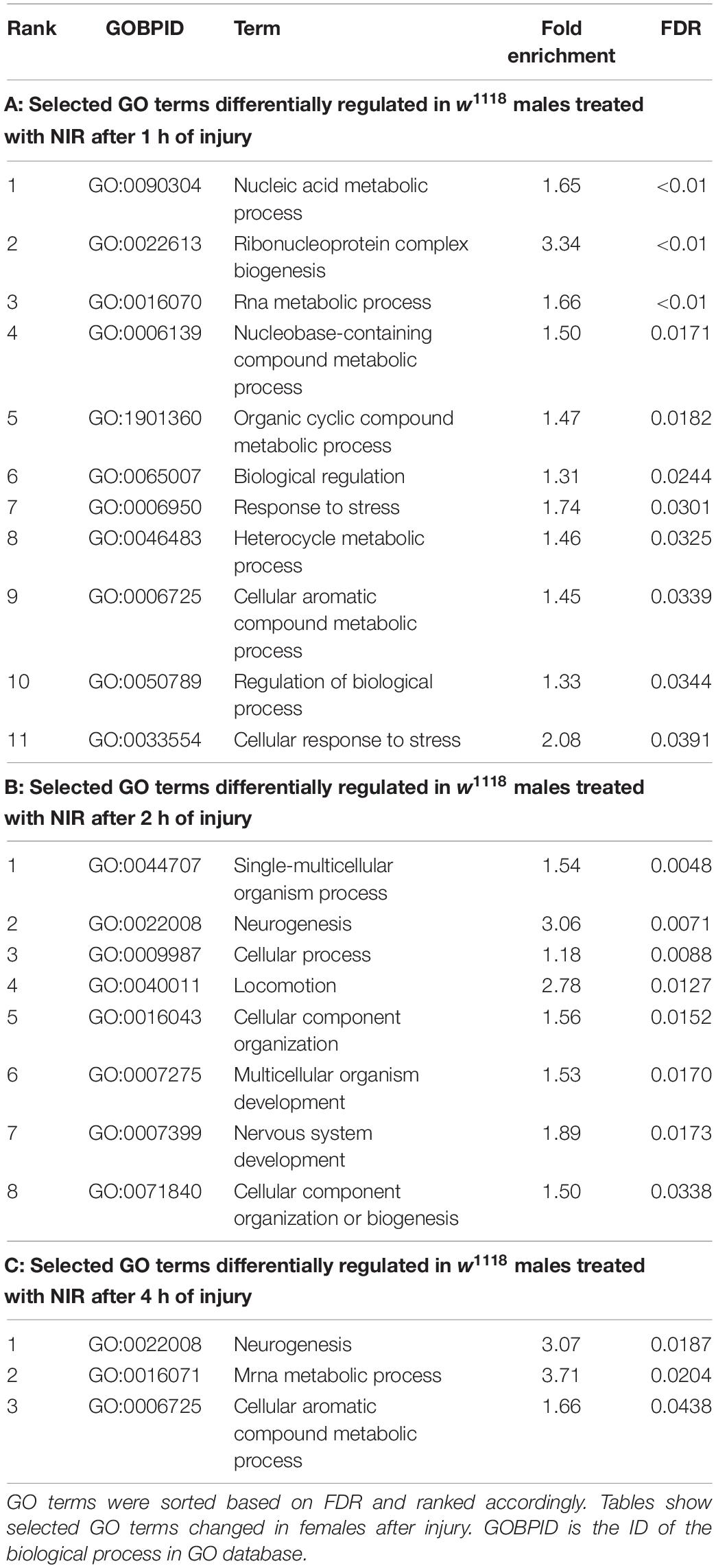
Table 2. Gene ontology terms significantly (FDR < 0.05) changed in response to traumatic brain injury in w1118 male flies treated with NIR.
These data suggest that while NIR exposure could be neuroprotective in brain injury, the effect of this exposure varies by biological sex in Drosophila. Male and female flies show differences in gene transcription after injury and such differences are also prevalent after exposure to COX-inhibitory NIR. Overall, both sexes exhibit fewer transcriptional changes in response to TBI after exposure to COX-inhibitory NIR.
NIR Treatment Downregulates Immune Gene Transcription in Injured Flies
Brain trauma triggers immune system activation, which helps protect tissue against damage (Plesnila, 2016), but long-term inflammation may contribute to neurological deterioration and cognitive decline (Henry et al., 2020). TBI induced neuroinflammation and pathology have also been linked to an increased risk of developing neurodegenerative disorders like AD, PD, and chronic traumatic encephalopathy (CTE) (McKee and Lukens, 2016). We have previously reported an upregulation of immune gene expression in female flies within 4 hr of injury (Shah et al., 2020) and there have been several reports of an upregulated immune response in male flies days, weeks or months after injury (Katzenberger et al., 2016; van Alphen et al., 2018; Swanson et al., 2020). In animal models of TBI, exposure to NIR light has been shown to downregulate pro-inflammatory cytokines and upregulate anti-inflammatory cytokines (Moreira et al., 2009; Khuman et al., 2012; Quirk et al., 2012; Zhang et al., 2014). Based on this evidence, we aimed to explore the effect of modulating mitochondrial COX using NIR exposure at 750 nm on immune gene expression in male and female fly brains inflicted with TBI.
The Drosophila immune system is regulated by the Toll, Immunodeficiency (Imd) and Janus Kinase protein and the Signal Transducer and Activator of Transcription (JAK-STAT) pathways (West and Silverman, 2017). We looked at transcriptional changes in genes involved with these pathways and observed that w1118 flies exposed to NIR post-injury exhibit fewer alterations to immune genes than those untreated with NIR (Figure 3). In females, we have previously seen a significant upregulation of genes encoding anti-fungal peptide Drs (Drosomycin) and anti-bacterial peptides Diptericin (DptA, DptB), Cecropin (CecA1, CecA2, CecB, and CecC), and Attacin (Att, AttB, and AttC) (Figure 3A) after injury. w1118 females exposed to NIR show an upregulation in transcript levels of CecB, AttC, DptB, CecA1, and Dro in the immediate time frame after injury and are mostly unchanged by 4 hr. The Drosophila NF-κB transcription factor Rel (Relish) (Hetru and Hoffmann, 2009), a downstream component of the immune deficiency pathway, was significantly upregulated in injured females not exposed to NIR but unchanged after NIR treatment. The NF-κB pathway functions in the host defense of Drosophila to control the expression of genes encoding immune-responsive peptides and proteins (Hetru and Hoffmann, 2009). Expression of Mtk (Metchnikowin), an antimicrobial peptide, was seen to be significantly increased in females after TBI but is unchanged in injured females exposed to NIR. Loss of Mtk has been shown to reduce mortality and behavioral deficits and improve lifespan in TBI exposed flies (Swanson et al., 2020).
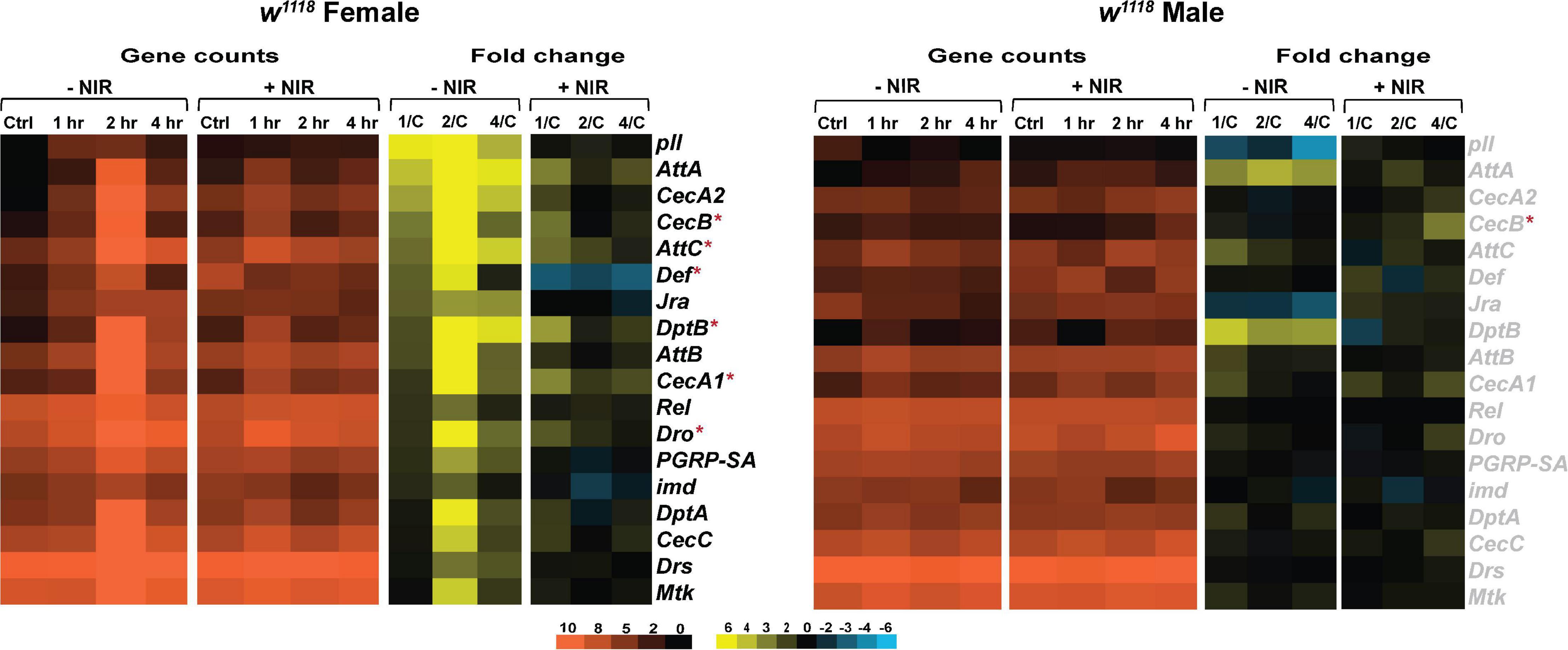
Figure 3. Immune response gene expression is downregulated in injured w1118 flies with post-NIR exposure. Heatmaps depicting immune response gene expression changes in w1118 females and males at control, 1, 2, and 4 hr after injury and NIR exposure. The orange scale represents average normalized counts for three replicates in each of the indicated groups. Yellow-blue scale shows fold change for each gene at 1-, 2-, and 4-hr post-injury compared to control. The genes indicated in black font are significantly changed in injured flies not exposed to NIR whereas red asterisk (*) indicates significant alteration in injured flies exposed to NIR (| log2FC| > 1, p-value < 0.05). 1/C: Fold change at 1 hr compared to control; 2/C: Fold change at 2 hr compared to control and 4/C: Fold change at 4 hr compared to control. (–NIR: not exposed to NIR and +NIR: exposed to NIR).
w1118 males show no transcriptional change in response to injury with or without exposure to NIR for immune response (Figure 3B). We have observed significant upregulation in CecB in injured males exposed to NIR 4-hr after injury. Although not significantly induced after injury, we have seen consistently high transcription of AttA and DptB in injured males not exposed to NIR.
Trauma-induced changes in glial gene expression is a conserved feature of mammalian (Allen and Barres, 2009; Chung et al., 2015) and Drosophila models (van Alphen et al., 2018; Swanson et al., 2020). In flies, glia are able to perform immune related functions and dysregulation of immune signaling in glial cells has been implicated in neurodegeneration (Petersen et al., 2012, 2013). In this study, we utilized the Gal4/UAS system to drive GFP expression in glia using the glial marker Repo driving Gal4 and the reporter gene UAS-GFP. Thus, in addition to gene transcription, we looked at change in GFP reporter expression in glial cells after injury and exposure to NIR in both sexes. We have observed differences in GFP expression and repo transcription in both sexes post-TBI (Figure 4). In females (Figures 4A,B) and males (Figures 4D,E), we saw a significant increase in GFP expression 1, 4, and 24 hr after injury but no change at 2 hr compared to control. At all three time-points, the observed increase in GFP expression is significantly decreased after NIR exposure in both sexes. We did observe a significant decrease in GFP expression at 2 hr in males but the regulation of this phasic pattern is not understood. Sex-differences were also observed in repo transcription with injured females (Figure 4C) exhibiting significant upregulation 1 hr after injury whereas injured males (Figure 4F) showed significant downregulation 2 hr after injury.
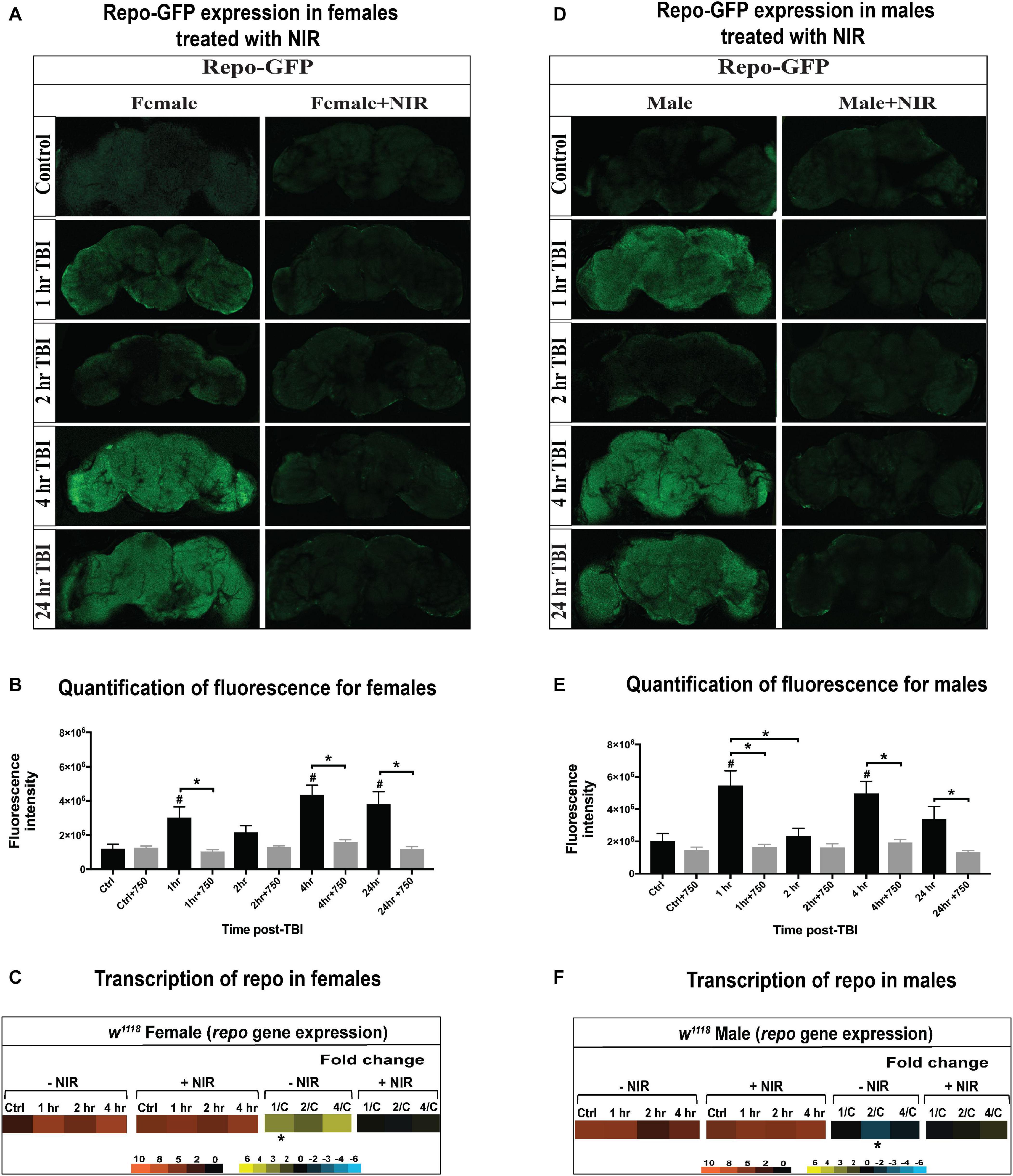
Figure 4. GFP-tagged repo expression in w1118 male and female flies. Confocal images showing change in GFP expression in Repo-GFP flies at control, 1, 2, 4, and 24 hr after TBI in females (A) and males (D) with and without exposure to NIR. About 10–15 brains were imaged for each condition and representative images from each group are shown here. Average fluorescence intensity for each time-point (10–15 brains) was assessed using ImageJ marking whole brain area as region of interest (B,E). A significant increase (One-way ANOVA, Dunnett test, p-value < 0.05) in GFP expression was observed for both sexes at 1, 4, and 24 hr after injury. GFP expression was unchanged in both sexes inflicted with TBI after NIR exposure. Repo transcription was significantly upregulated (| log2FC| > 1, p-value < 0.05) in females 1 hr after injury (C) and downregulated in males (F) 2 hr after injury. NIR exposure had no change in repo transcription in both sexes.
Overall, male and female flies exhibit differences in immune response activation after brain injury with females exhibiting a more immediate alteration in gene transcription than males. Injured males do exhibit an apparent upregulation of immune response as seen by repo-GFP expression, but this response is discordant with repo gene transcription which did not change in males, likely due to changes in protein dynamics (degradation or turnover) not captured by transcriptional data. Inhibiting COX-activity after injury prevents the aberrant activation of immune response gene transcription in females but has no effect on males. It is likely that sexual dimorphism in immune response contributes to the differences in response to injury and NIR exposure.
Injured Flies Exposed to NIR Exhibit Downregulation in Mitochondrial Gene Transcription
From meeting the increased demand for ATP production to initiating apoptotic signals for clearance of cell debris, mitochondrial function is crucial to the repair process after brain injury (Lifshitz et al., 2004; Hiebert et al., 2015). However, this upregulation in mitochondrial process is also accompanied by an increased generation of ROS, hyperpolarization of ΔΨm and mitochondrial dysfunction leading to neurodegeneration (Liesa et al., 2009; Shah et al., 2019, 2020). Sexual dimorphism in mitochondrial metabolism is also found to play a crucial role in development of pathologies following injury (Gupte et al., 2019). We have previously shown sex-differences in mitochondrial stress and gene transcription after TBI in Drosophila (Shah et al., 2020) and we here wanted to explore the effects of inhibiting mitochondrial COX activity on gene expression in both sexes of injured flies.
Transcription of genes involved in mitochondrial oxidative phosphorylation, biogenesis, transport and translation is significantly upregulated in injured w1118 females (Figure 5). We have seen increased expression of SdhA and SdhB (Succinate dehydrogenase, subunit A and B), subunits of the succinate dehydrogenase complex of the ETC after injury in females. Surf1 (Surfeit 1), involved in the assembly of COX is also upregulated in injured females. Increased transcription of ETC genes could indicate upregulation of mitochondrial activity to increase production of ATP (Sen et al., 2017). There is significant upregulation of mitochondrial ribosomal genes like mRpL43, mRpS25, mRpL46, mRpL1, mRpS21, and mRpL35 after injury in females. Upregulation in transcription of these genes could indicate alterations in mitochondrial dynamics to clear damage and dysfunctional mitochondria after injury and restore homeostasis. Interestingly, COX inhibition with NIR exposure in females did not have significant transcriptional effects after TBI. There was significant downregulation observed only in mRpL43 after injury in NIR exposed females. Similar to immune processes, we observed limited changes in injured w1118 males with or without NIR exposure (Figure 5). mRpL43 was significantly downregulated in injured males but unchanged after NIR exposure whereas mRpL35 was significantly upregulated after NIR treatment only. It is possible that in Drosophila, similar to humans, mitochondrial function and metabolism vary by sex, which may explain the variations observed between both sexes in response to injury.
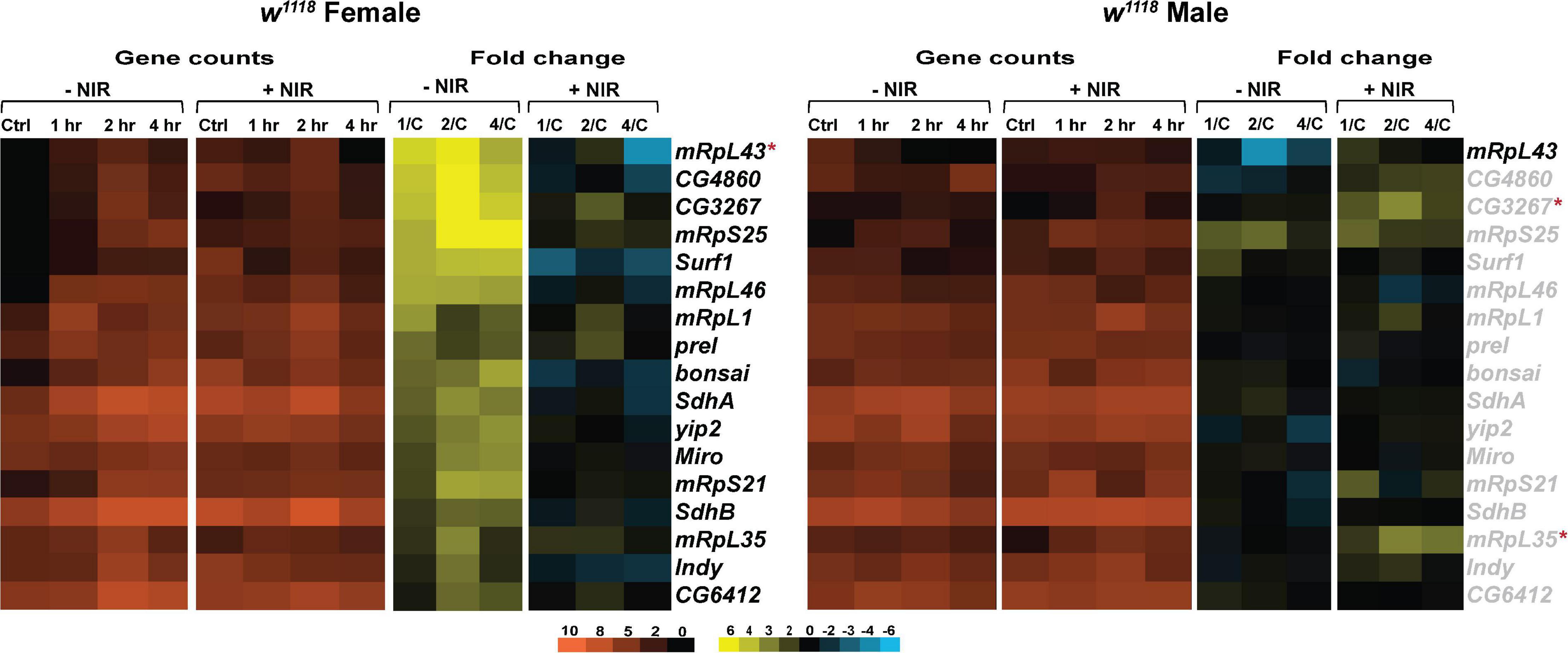
Figure 5. NIR exposure downregulates mitochondrial gene transcription in w1118 flies subjected to TBI. Heatmaps depicting mitochondrial gene expression changes in w1118 females and males at control, 1, 2, and 4 hr after injury and NIR exposure. The orange scale represents average normalized counts for three replicates in each of the indicated groups. Yellow-blue scale shows fold change for each gene at 1-, 2-, and 4-hr post-injury compared to control. The genes indicated in black font are significantly changed in injured flies not exposed to NIR whereas red asterisk (*) indicates significant alteration in injured flies exposed to NIR (| log2FC| > 1, p-value < 0.05). 1/C: Fold change at 1 hr compared to control; 2/C: Fold change at 2 hr compared to control and 4/C: Fold change at 4 hr compared to control. (–NIR: not exposed to NIR and +NIR: exposed to NIR).
These data suggest that modulating mitochondrial COX within the immediate early period following brain injury could prevent mitochondrial damage. However, additional studies that determine ΔΨm, oxygen consumption, ATP production and superoxide production after NIR exposure will be useful to support the observed changes in gene transcription reported here.
Cytoskeletal Gene Transcription Is Downregulated in Injured Flies After NIR Exposure
Mitochondria move along the axons in both directions using the microtubules and motor proteins (Bartolak-Suki et al., 2017). These interactions between the cytoskeleton and mitochondria are essential for maintaining mitochondrial morphology (Boldogh and Pon, 2006). The twisting and shearing of axons caused by the movement of brain within the skull during an injury is known to cause mechanical deformation on the neuronal cytoskeleton (Hill et al., 2016). Following TBI, Tau, a microtubule associated protein is found to be hyperphosphorylated and impact cytoskeletal integrity and mitochondrial transport (Sivanandam and Thakur, 2012; Edwards et al., 2019). Thus, we wanted to look at the effects of exposure to COX-inhibitory NIR on tau and cytoskeletal gene transcription in injured flies of both sexes.
As observed in immune response and mitochondrial gene transcription, we saw significant upregulation in expression of cytoskeletal genes in injured females (Figure 6). w1118 injured females exhibit significant upregulation of kinases involved in Tau phosphorylation like lok (loki) and Cdk5alpha (Cdk5 activator-like protein) after injury (Figure 6). Cyclin-dependent kinases were shown to be involved in mitophagy in cell culture models (Moskal et al., 2020). Microtubule integrity depends largely on tubulin polymerization (Bartolak-Suki et al., 2017) and we found a significant upregulation in alphaTub84D (α-Tubulin at 84D) and betaTub97EF (β-Tubulin at 97EF), both involved in polymerization of microtubules. In w1118 injured males (Figure 6), we observed very little transcriptional change with only pbl (pebble), involved in actin cytoskeleton reorganization being significantly downregulated whereas pbl was upregulated in injured females. Exposure to NIR resulted in significant alteration in Tip60 (Tat interactive protein 60kDa), cher (cheerio) and Cdk5alpha in females. DCTN5-p25 (Dynactin 5, p25 subunit), a motor protein involved in retrograde axonal transport was significantly upregulated in injured females but unchanged after NIR exposure. DCTN5-p25 was significantly upregulated after NIR exposure in injured males.
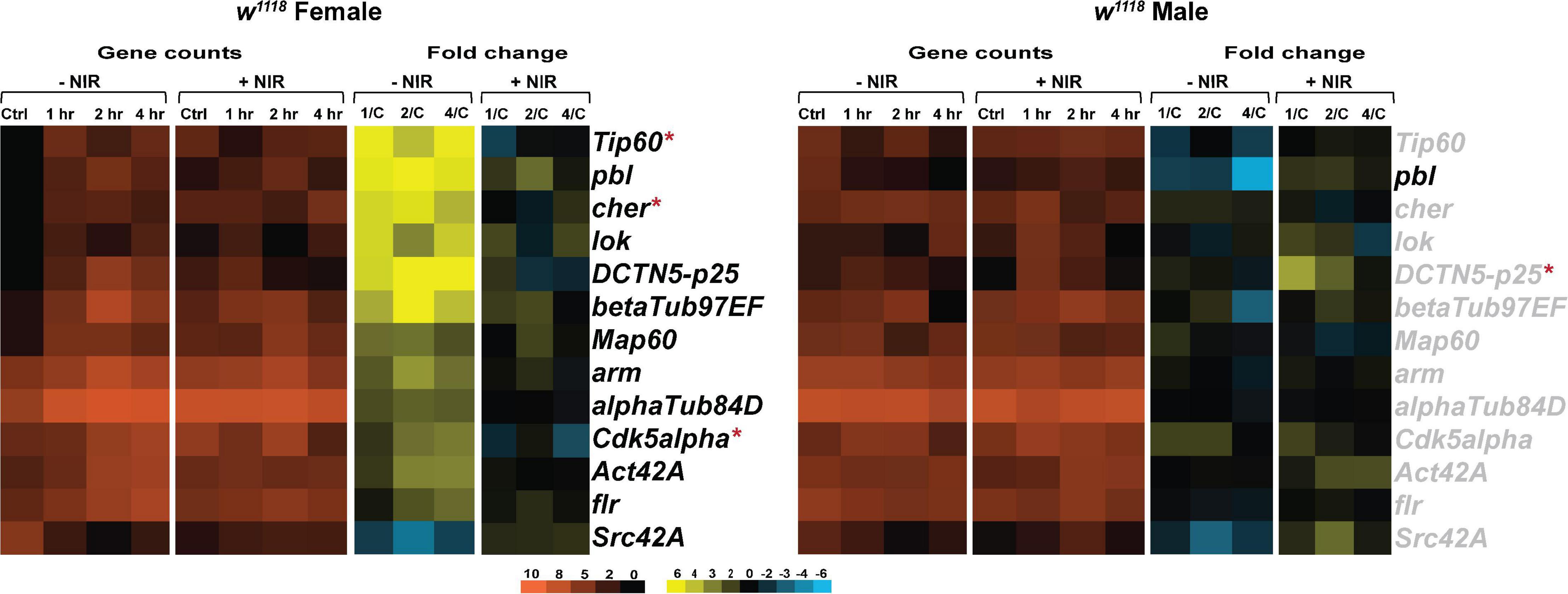
Figure 6. Cytoskeletal gene transcription is downregulated after NIR exposure. Heatmaps depicting cytoskeletal gene expression changes in w1118 females and males at control, 1, 2, and 4 hr after injury and NIR exposure. The orange scale represents average normalized counts for three replicates in each of the indicated groups. Yellow-blue scale shows fold change for each gene at 1-, 2-, and 4-hr post-injury compared to control. The genes indicated in black font are significantly changed in injured flies not exposed to NIR whereas red asterisk (*) indicates significant alteration in injured flies exposed to NIR (| log2FC| > 1, p-value < 0.05). 1/C: Fold change at 1 hr compared to control; 2/C: Fold change at 2 hr compared to control and 4/C: Fold change at 4 hr compared to control. (–NIR: not exposed to NIR and +NIR: exposed to NIR).
Our data suggests that brain injury affects cytoskeletal integrity differently in male and female flies and NIR exposure limits the damage sustained by trauma in both sexes.
Discussion
Pharmacological therapies for brain injury are primarily focused on modulating major neurotransmitter processes to enhance the neurocognitive sequelae of TBI (Morries et al., 2015). Unfortunately, little has been found to reverse TBI damage caused by mitochondrial dysfunction, a well-known cause of neuronal death (Hiebert et al., 2015; Morries et al., 2015). In this study we employed NIR light, a non-pharmacological approach to minimize mitochondrial damage after TBI in Drosophila. NIR treatment has been shown to provide benefit in animal models of ischemia/reperfusion injury (Sanderson et al., 2018), spinal cord injury (Giacci et al., 2014), stroke (Strubakos et al., 2020), optic nerve injury (Giacci et al., 2014), and in human trials of TBI (Naeser et al., 2014). Sex differences in outcome to TBI have been observed in flies (Shah et al., 2020) and there exist strong evidence indicating sex differences in mitochondria could also have an effect on TBI response (Conley et al., 2014; Gupte et al., 2019). Hence, we assessed the effects of modulating mitochondrial COX using NIR exposure on gene transcription in the immediate time frame after brain injury in both sexes. The fly brain is enclosed in a cuticle composed of chitin which strongly absorbs the 750 nm infrared radiation (Klocke et al., 2011), so we sequenced 3′mRNA libraries of isolated w1118 fly brains at control, 1, 2, and 4 hr after TBI. Our results suggest that inhibiting COX activity downregulates gene expression in injured females whereas injured males exhibit minimal changes with or without NIR treatment.
Different studies have suggested a positive effect of PBM treatment in TBI patients (Naeser et al., 2014; Poiani et al., 2018; Carneiro et al., 2019). NIR between 700–1000 nm readily penetrates the scalp and skull and has the potential to improve cellular activity of compromised brain tissue (Santos et al., 2018). Most studies employing NIR treatment have focused on evaluating the efficacy of this exposure on neuropsychological defects like impaired cognition and mood arising from brain injury (Poiani et al., 2018; Santos et al., 2018) but none have looked at the effect of NIR on gene transcription. Since failing mitochondria can place the cell in energy crisis, mitochondrial dysfunction after injury could affect gene transcription, an energy demanding process (Muir et al., 2016). Thus, we looked at gene transcription profile to identify the effect of mitochondrial damage on brain transcriptome post-TBI and COX inhibition. It should be noted that most PBM studies conducted to date used COX-activating NIR, such as 810 nm, and no COX-inhibitory wavelengths were known until their recent discovery by the Hüttemann laboratory (Sanderson et al., 2018). Therefore, the goal of this study was to assess (a) the change in gene expression in response to COX-inhibitory NIR exposure and (b) whether the response varies in both sexes. Contrary to other studies, we have made use of a single COX-inhibitory wavelength (750 nm) to assess genome-wide changes in gene expression in TBI inflicted fly brains. We specifically looked at alteration in genes involved in immune response, mitochondrial function and cytoskeleton after TBI.
Brain injury causes a prolonged activation of inflammatory responses, which exacerbates primary damage occurring within hours of injury (McKee and Lukens, 2016; Plesnila, 2016; Dinet et al., 2019). Efforts to manipulate genes or pathways that can either trigger anti-inflammatory responses or inhibit pro-inflammatory processes have been underway as means to curb this extensive brain damage (Griffin, 2011; McKee and Lukens, 2016). A growing body of evidence also links mitochondrial dysfunction with increased incidences of immune activation (Walker et al., 2014; Breda et al., 2019). Here, we see an increased expression of genes involved in the Drosophila immune system after injury in females and most of these upregulated genes are unchanged after NIR exposure. Previous studies have also noted protective effects of PBM on immune system in TBI models (Moreira et al., 2009; Hennessy and Hamblin, 2017) but the underlying cause for differences between males and females as observed here remains unknown. Drosophila males have been shown to have increased immune gene transcription days or weeks after injury (Katzenberger et al., 2016; Swanson et al., 2020), so it is likely that an upregulated gene expression could be observed if longer time points are assessed. Sexual dimorphism in mitochondria (Demarest and McCarthy, 2015; Gupte et al., 2019) could also be influencing the secondary damage cascades after brain injury but further studies are required to explore such differences in flies. It should also be noted that the NIR wavelength (COX-inhibiting) and energy density employed in this study is different to previously published reports (Weinrich et al., 2017), which could also have an impact on the response. Weinrich et al. (2017) showed that lifelong 670-nm exposure in Drosophila extends lifespan and improves aged mobility. However, our initial assessments determined that chitin absorbed NIR at the 750-nm exposure, thus raising the possibility that different wavelengths have varying penetration through tissues. Due to this we were unable to assess behavioral function or survival post-TBI in NIR-exposed flies, however our focus was to assess gene expression changes in the brain after injury.
One of the most pronounced effect of TBI is axonal damage which impacts the cytoskeletal integrity and structure (Fitzpatrick et al., 1998; Saatman and Burkhardt, 2009). The cytoskeleton regulates mitochondrial positioning and transport and this interaction of mitochondria with microtubules is a tightly regulated process (Anesti and Scorrano, 2006; Boldogh and Pon, 2006). An additional level of regulation is accomplished by microtubule-associated proteins (MAP’s) like Tau (Ebneth et al., 1998) and pathological Tau, a common finding in TBI patients and animals models of brain injury (Collins-Praino and Corrigan, 2017; Castellani and Perry, 2019), inhibits mitochondrial transport (Shahpasand et al., 2012). In this study, we observed an upregulation in several cytoskeletal genes in injured females but no change in tau transcription in both sexes after TBI. NIR exposure appears to show an immediate response in females but minimal change in males. Similar trend in gene expression is also observed in mitochondrial gene expression for both sexes after injury and subsequent NIR exposure. Since tau transcription was unaffected by brain injury, we also exposed TBI inflicted Tau knockout flies to NIR and observed mostly downregulation or no change in gene expression in both sexes (Supplementary Data 1). The synergistic effect of absence of tau and NIR exposure in injured flies highlights the role of tau expression and mitochondrial dysfunction in TBI outcome.
In conclusion, we have shown that exposure to COX-inhibitory NIR light within the immediate timeframe after brain injury downregulates gene expression in injured flies. The response to TBI and the subsequent modulation of mitochondrial COX by exposure to NIR varies by biological sex with females exhibiting a more pronounced effect than males in Drosophila. Although the cause of these transcriptional differences remains unknown, the presence of metabolic tissues, sex-specific genes or brain architecture could be potential factors requiring further studies. In this study, we were unable to assess lifespan or behavioral measures in NIR-exposed flies due to the absorbance property of chitin, but we present genome-wide transcriptional changes in Drosophila brains to decipher gene networks or pathways that are affected by TBI and NIR. Finally, we propose that a detailed understanding of the disease mechanism at the mitochondrial level after acute stress (Sanderson et al., 2013, 2018) will make possible use of COX-inhibitory and -activating NIR at the early and late time periods following tissue injury, respectively, to limit ΔΨm hyperpolarization and thus ROS early to suppress cell death, whereas at later chronic stages of the disease, COX-activating NIR may be useful to enhance tissue remodeling and repair.
Data Availability Statement
The datasets presented in this study can be found in online repositories. The names of the repository/repositories and accession number(s) can be found in the article/Supplementary Material. Gene expression data are available in the GEO database under accession number GSE140663 (w1118 without NIR exposure) and GSE158061 (w1118 with NIR exposure).
Author Contributions
DR, MH, and KG conceived the project. ES and KG conceptualized the content. ES performed the data analysis and wrote the manuscript. All authors edited the manuscript and assisted with data analysis.
Funding
This work was funded by the National Institutes of Health [Grant Nos. 5R01ES012933 (DR), 5P30ES020957 (DR), and 1UG3OD023285 (DR)]. In addition, the Office of the Vice President for Research at Wayne State University supports KG and provided pilot funds for DR.
Conflict of Interest
MH and TS are co-founders of Mitovation Inc., that develops infrared light therapy for ischemia/reperfusion injury applications.
The remaining authors declare that the research was conducted in the absence of any commercial or financial relationships that could be construed as a potential conflict of interest.
Supplementary Material
The Supplementary Material for this article can be found online at: https://www.frontiersin.org/articles/10.3389/fphys.2021.628777/full#supplementary-material
Supplementary Data Sheet 1 | Gene Ontology tables.
References
Allen, N. J., and Barres, B. A. (2009). Neuroscience: glia - more than just brain glue. Nature 457, 675–677. doi: 10.1038/457675a
Anders, S., Pyl, P. T., and Huber, W. (2015). HTSeq–a Python framework to work with high-throughput sequencing data. Bioinformatics 31, 166–169. doi: 10.1093/bioinformatics/btu638
Anesti, V., and Scorrano, L. (2006). The relationship between mitochondrial shape and function and the cytoskeleton. Biochim. Biophys. Acta 1757, 692–699. doi: 10.1016/j.bbabio.2006.04.013
Bartolak-Suki, E., Imsirovic, J., Nishibori, Y., Krishnan, R., and Suki, B. (2017). Regulation of mitochondrial structure and dynamics by the cytoskeleton and mechanical factors. Int. J. Mol. Sci. 18:1812. doi: 10.3390/ijms18081812
Begum, R., Calaza, K., Kam, J. H., Salt, T. E., Hogg, C., and Jeffery, G. (2015). Near-infrared light increases ATP, extends lifespan and improves mobility in aged Drosophila melanogaster. Biol. Lett. 11:20150073. doi: 10.1098/rsbl.2015.0073
Boldogh, I. R., and Pon, L. A. (2006). Interactions of mitochondria with the actin cytoskeleton. Biochim. Biophys. Acta 1763, 450–462. doi: 10.1016/j.bbamcr.2006.02.014
Breda, C. N. S., Davanzo, G. G., Basso, P. J., Saraiva Camara, N. O., and Moraes-Vieira, P. M. M. (2019). Mitochondria as central hub of the immune system. Redox Biol. 26:101255. doi: 10.1016/j.redox.2019.101255
Carneiro, A. M. C., Poiani, G. C., Zaninnoto, A. L., Lazo Osorio, R., Oliveira, M. L., Paiva, W. S., et al. (2019). Transcranial photobiomodulation therapy in the cognitive rehabilitation of patients with cranioencephalic trauma. Photobiomodul Photomed Laser Surg. 37, 657–666. doi: 10.1089/photob.2019.4683
Castellani, R. J., and Perry, G. (2019). Tau biology, tauopathy, traumatic brain injury, and diagnostic challenges. J. Alzheimers Dis. 67, 447–467. doi: 10.3233/JAD-180721
Cheng, G., Kong, R. H., Zhang, L. M., and Zhang, J. N. (2012). Mitochondria in traumatic brain injury and mitochondrial-targeted multipotential therapeutic strategies. Br. J. Pharmacol. 167, 699–719. doi: 10.1111/j.1476-5381.2012.02025.x
Chung, W. S., Welsh, C. A., Barres, B. A., and Stevens, B. (2015). Do glia drive synaptic and cognitive impairment in disease? Nat. Neurosci. 18, 1539–1545. doi: 10.1038/nn.4142
Ciuffreda, K. J., Ludlam, D. P., Yadav, N. K., and Thiagarajan, P. (2016). Traumatic brain injury. Adv. Ophthalmol. Optom. 1, 307–333. doi: 10.1016/j.yaoo.2016.03.013
Collins-Praino, L. E., and Corrigan, F. (2017). Does neuroinflammation drive the relationship between tau hyperphosphorylation and dementia development following traumatic brain injury? Brain Behav. Immun. 60, 369–382. doi: 10.1016/j.bbi.2016.09.027
Conley, Y. P., Okonkwo, D. O., Deslouches, S., Alexander, S., Puccio, A. M., Beers, S. R., et al. (2014). Mitochondrial polymorphisms impact outcomes after severe traumatic brain injury. J. Neurotrauma 31, 34–41. doi: 10.1089/neu.2013.2855
Demarest, T. G., and McCarthy, M. M. (2015). Sex differences in mitochondrial (dys)function: implications for neuroprotection. J. Bioenerg. Biomembr. 47, 173–188. doi: 10.1007/s10863-014-9583-7
Dinet, V., Petry, K. G., and Badaut, J. (2019). Brain-immune interactions and neuroinflammation after traumatic brain injury. Front. Neurosci. 13:1178. doi: 10.3389/fnins.2019.01178
Dobin, A., Davis, C. A., Schlesinger, F., Drenkow, J., Zaleski, C., Jha, S., et al. (2013). STAR: ultrafast universal RNA-seq aligner. Bioinformatics 29, 15–21. doi: 10.1093/bioinformatics/bts635
Ebneth, A., Godemann, R., Stamer, K., Illenberger, S., Trinczek, B., and Mandelkow, E. (1998). Overexpression of tau protein inhibits kinesin-dependent trafficking of vesicles, mitochondria, and endoplasmic reticulum: implications for Alzheimer’s disease. J. Cell Biol. 143, 777–794. doi: 10.1083/jcb.143.3.777
Edwards, G. III, Zhao, J., Dash, P. K., Soto, C., and Moreno-Gonzalez, I. (2019). Traumatic brain injury induces tau aggregation and spreading. J. Neurotrauma 37, 80–92. doi: 10.1089/neu.2018.6348
Finnie, J. W., and Blumbergs, P. C. (2002). Traumatic brain injury. Vet Pathol. 39, 679–689. doi: 10.1354/vp.39-6-679
Fischer, T. D., Hylin, M. J., Zhao, J., Moore, A. N., Waxham, M. N., and Dash, P. K. (2016). Altered mitochondrial dynamics and TBI pathophysiology. Front. Syst. Neurosci. 10:29. doi: 10.3389/fnsys.2016.00029
Fitzpatrick, M. O., Dewar, D., Teasdale, G. M., and Graham, D. I. (1998). The neuronal cytoskeleton in acute brain injury. Br. J. Neurosurg. 12, 313–317. doi: 10.1080/02688699844808
Fresno, C., and Fernandez, E. A. (2013). RDAVIDWebService: a versatile R interface to DAVID. Bioinformatics 29, 2810–2811. doi: 10.1093/bioinformatics/btt487
Giacci, M. K., Wheeler, L., Lovett, S., Dishington, E., Majda, B., Bartlett, C. A., et al. (2014). Differential effects of 670 and 830 nm red near infrared irradiation therapy: a comparative study of optic nerve injury, retinal degeneration, traumatic brain and spinal cord injury. PLoS One 9:e104565. doi: 10.1371/journal.pone.0104565
Griffin, G. D. (2011). The injured brain: TBI, mTBI, the immune system, and infection: connecting the dots. Mil Med. 176, 364–368. doi: 10.7205/milmed-d-10-00021
Gupte, R., Brooks, W., Vukas, R., Pierce, J., and Harris, J. (2019). Sex differences in traumatic brain injury: what we know and what we should know. J. Neurotrauma 36, 3063–3091. doi: 10.1089/neu.2018.6171
Hamblin, M. R. (2018). Photobiomodulation for traumatic brain injury and stroke. J. Neurosci. Res. 96, 731–743. doi: 10.1002/jnr.24190
Hennessy, M., and Hamblin, M. R. (2017). Photobiomodulation and the brain: a new paradigm. J. Opt. 19:013003. doi: 10.1088/2040-8986/19/1/013003
Henry, R. J., Ritzel, R. M., Barrett, J. P., Doran, S. J., Jiao, Y., Leach, J. B., et al. (2020). Microglial Depletion with CSF1R inhibitor during chronic phase of experimental traumatic brain injury reduces neurodegeneration and neurological deficits. J. Neurosci. 40, 2960–2974. doi: 10.1523/JNEUROSCI.2402-19.2020
Hetru, C., and Hoffmann, J. A. (2009). NF-kappaB in the immune response of Drosophila. Cold Spring Harb. Perspect Biol. 1:a000232. doi: 10.1101/cshperspect.a000232
Hiebert, J. B., Shen, Q., Thimmesch, A. R., and Pierce, J. D. (2015). Traumatic brain injury and mitochondrial dysfunction. Am. J. Med. Sci. 350, 132–138. doi: 10.1097/MAJ.0000000000000506
Hill, C. S., Coleman, M. P., and Menon, D. K. (2016). Traumatic axonal injury: mechanisms and translational opportunities. Trends Neurosci. 39, 311–324. doi: 10.1016/j.tins.2016.03.002
Huang, D. W., Sherman, B. T., and Lempicki, R. A. (2009). Bioinformatics enrichment tools: paths toward the comprehensive functional analysis of large gene lists. Nucleic Acids Res. 37, 1–13. doi: 10.1093/nar/gkn923
Hüttemann, M., Lee, I., Pecinova, A., Pecina, P., Przyklenk, K., and Doan, J. W. (2008). Regulation of oxidative phosphorylation, the mitochondrial membrane potential, and their role in human disease. J. Bioenerg. Biomembr. 40, 445–456. doi: 10.1007/s10863-008-9169-3
Karu, T. I., and Afanas’eva, N. I. (1995). [Cytochrome c oxidase as the primary photoacceptor upon laser exposure of cultured cells to visible and near IR-range light]. Dokl Akad Nauk 342, 693–695.
Katzenberger, R. J., Ganetzky, B., and Wassarman, D. A. (2016). Age and diet affect genetically separable secondary injuries that cause acute mortality following traumatic brain injury in Drosophila. G3 (Bethesda) 6, 4151–4166. doi: 10.1534/g3.116.036194
Katzenberger, R. J., Loewen, C. A., Bockstruck, R. T., Woods, M. A., Ganetzky, B., and Wassarman, D. A. A. (2015). Method to inflict closed head traumatic brain injury in Drosophila. J. Vis. Exp. 100:e52905. doi: 10.3791/52905
Katzenberger, R. J., Loewen, C. A., Wassarman, D. R., Petersen, A. J., Ganetzky, B., and Wassarman, D. A. A. (2013). Drosophila model of closed head traumatic brain injury. Proc. Natl. Acad. Sci. U.S.A. 110, E4152–E4159. doi: 10.1073/pnas.1316895110
Khuman, J., Zhang, J., Park, J., Carroll, J. D., Donahue, C., and Whalen, M. J. (2012). Low-level laser light therapy improves cognitive deficits and inhibits microglial activation after controlled cortical impact in mice. J. Neurotrauma 29, 408–417. doi: 10.1089/neu.2010.1745
Kim, S., Han, S. C., Gallan, A. J., and Hayes, J. P. (2017). Neurometabolic indicators of mitochondrial dysfunction in repetitive mild traumatic brain injury. Concussion 2:CNC48. doi: 10.2217/cnc-2017-0013
Klocke, D., Schmitz, A., Soltner, H., Bousack, H., and Schmitz, H. (2011). Infrared receptors in pyrophilous (”fire loving”) insects as model for new un-cooled infrared sensors. Beilstein J. Nanotechnol. 2, 186–197. doi: 10.3762/bjnano.2.22
Leitgeb, J., Mauritz, W., Brazinova, A., Janciak, I., Majdan, M., Wilbacher, I., et al. (2011). Effects of gender on outcomes after traumatic brain injury. J. Trauma. 71, 1620–1626. doi: 10.1097/TA.0b013e318226ea0e
Liesa, M., Palacin, M., and Zorzano, A. (2009). Mitochondrial dynamics in mammalian health and disease. Physiol. Rev. 89, 799–845. doi: 10.1152/physrev.00030.2008
Lifshitz, J., Sullivan, P. G., Hovda, D. A., Wieloch, T., and McIntosh, T. K. (2004). Mitochondrial damage and dysfunction in traumatic brain injury. Mitochondrion 4, 705–713. doi: 10.1016/j.mito.2004.07.021
McKee, C. A., and Lukens, J. R. (2016). Emerging roles for the immune system in traumatic brain injury. Front. Immunol. 7:556. doi: 10.3389/fimmu.2016.00556
Moreira, M. S., Velasco, I. T., Ferreira, L. S., Ariga, S. K., Barbeiro, D. F., Meneguzzo, D. T., et al. (2009). Effect of phototherapy with low intensity laser on local and systemic immunomodulation following focal brain damage in rat. J. Photochem. Photobiol. B 97, 145–151. doi: 10.1016/j.jphotobiol.2009.09.002
Morries, L. D., Cassano, P., and Henderson, T. A. (2015). Treatments for traumatic brain injury with emphasis on transcranial near-infrared laser phototherapy. Neuropsychiatr. Dis. Treat. 11, 2159–2175. doi: 10.2147/NDT.S65809
Moskal, N., Riccio, V., Bashkurov, M., Taddese, R., Datti, A., and Lewis, P. N. (2020). Angus McQuibban G. ROCK inhibitors upregulate the neuroprotective Parkin-mediated mitophagy pathway. Nat. Commun. 11:88. doi: 10.1038/s41467-019-13781-3
Muir, R., Diot, A., and Poulton, J. (2016). Mitochondrial content is central to nuclear gene expression: profound implications for human health. Bioessays 38, 150–156. doi: 10.1002/bies.201500105
Naeser, M. A., Saltmarche, A., Krengel, M. H., Hamblin, M. R., and Knight, J. A. (2011). Improved cognitive function after transcranial, light-emitting diode treatments in chronic, traumatic brain injury: two case reports. Photomed. Laser Surg. 29, 351–358. doi: 10.1089/pho.2010.2814
Naeser, M. A., Zafonte, R., Krengel, M. H., Martin, P. I., Frazier, J., Hamblin, M. R., et al. (2014). Significant improvements in cognitive performance post-transcranial, red/near-infrared light-emitting diode treatments in chronic, mild traumatic brain injury: open-protocol study. J. Neurotrauma 31, 1008–1017. doi: 10.1089/neu.2013.3244
Petersen, A. J., Katzenberger, R. J., and Wassarman, D. A. (2013). The innate immune response transcription factor relish is necessary for neurodegeneration in a Drosophila model of ataxia-telangiectasia. Genetics 194, 133–142. doi: 10.1534/genetics.113.150854
Petersen, A. J., Rimkus, S. A., and Wassarman, D. A. (2012). ATM kinase inhibition in glial cells activates the innate immune response and causes neurodegeneration in Drosophila. Proc. Natl. Acad. Sci. U.S.A. 109, E656–E664. doi: 10.1073/pnas.1110470109
Plesnila, N. (2016). The immune system in traumatic brain injury. Curr. Opin. Pharmacol. 26, 110–117. doi: 10.1016/j.coph.2015.10.008
Poiani, G., Zaninotto, A. L., Carneiro, A. M. C., Zangaro, R. A., Salgado, A. S. I., Parreira, R. B., et al. (2018). Photobiomodulation using low-level laser therapy (LLLT) for patients with chronic traumatic brain injury: a randomized controlled trial study protocol. Trials 19:17. doi: 10.1186/s13063-017-2414-5
Quirk, B. J., Torbey, M., Buchmann, E., Verma, S., and Whelan, H. T. (2012). Near-infrared photobiomodulation in an animal model of traumatic brain injury: improvements at the behavioral and biochemical levels. Photomed. Laser Surg. 30, 523–529. doi: 10.1089/pho.2012.3261
Reddy, P. H. (2009). Role of mitochondria in neurodegenerative diseases: mitochondria as a therapeutic target in Alzheimer’s disease. CNS Spectr. 14(8 Suppl 7), 8–13;discussion6–8. doi: 10.1017/s1092852900024901
Robinson, M. D., McCarthy, D. J., and Smyth, G. K. (2010). edgeR: a Bioconductor package for differential expression analysis of digital gene expression data. Bioinformatics 26, 139–140. doi: 10.1093/bioinformatics/btp616
Saatman, K. E. S. G., and Burkhardt, M. F. (2009). “Axonal damage due to traumatic brain injury,” in Handbook of Neurochemistry and Molecular Neurobiology, eds A. Lajtha, G. E. Gibson, and G. A. Dienel (Boston, MA: Springer).
Saldanha, A. J. (2004). Java Treeview–extensible visualization of microarray data. Bioinformatics 20, 3246–3248. doi: 10.1093/bioinformatics/bth349
Sanderson, T. H., Reynolds, C. A., Kumar, R., Przyklenk, K., and Hüttemann, M. (2013). Molecular mechanisms of ischemia-reperfusion injury in brain: pivotal role of the mitochondrial membrane potential in reactive oxygen species generation. Mol. Neurobiol. 47, 9–23. doi: 10.1007/s12035-012-8344-z
Sanderson, T. H., Wider, J. M., Lee, I., Reynolds, C. A., Liu, J., Lepore, B., et al. (2018). Inhibitory modulation of cytochrome c oxidase activity with specific near-infrared light wavelengths attenuates brain ischemia/reperfusion injury. Sci. Rep. 8:3481. doi: 10.1038/s41598-018-21869-x
Santos, J., Zaninotto, A. L. C., Zangaro, R. A., Carneiro, A. M. C., Neville, I. S., de Andrade, A. F., et al. (2018). Effects of transcranial LED therapy on the cognitive rehabilitation for diffuse axonal injury due to severe acute traumatic brain injury: study protocol for a randomized controlled trial. Trials 19:249. doi: 10.1186/s13063-018-2632-5
Schneider, C. A., Rasband, W. S., and Eliceiri, K. W. N. I. H. (2012). Image to ImageJ: 25 years of image analysis. Nat. Methods 9, 671–675. doi: 10.1038/nmeth.2089
Sen, A., Gurdziel, K., Liu, J., Qu, W., Nuga, O. O., Burl, R. B., et al. (2017). Smooth, an hnRNP-L homolog, might decrease mitochondrial metabolism by post-transcriptional regulation of isocitrate dehydrogenase (Idh) and other metabolic genes in the sub-acute phase of traumatic brain injury. Front. Genet. 8:175. doi: 10.3389/fgene.2017.00175
Shah, E. J., Gurdziel, K., and Ruden, D. M. (2019). Mammalian models of traumatic brain injury and a place for Drosophila in TBI research. Front. Neurosci. 13:409. doi: 10.3389/fnins.2019.00409
Shah, E. J., Gurdziel, K., and Ruden, D. M. (2020). Drosophila exhibit divergent sex-based responses in transcription and motor function after traumatic brain injury. Front. Neurol. 11:511. doi: 10.3389/fneur.2020.00511
Shahpasand, K., Uemura, I., Saito, T., Asano, T., Hata, K., Shibata, K., et al. (2012). Regulation of mitochondrial transport and inter-microtubule spacing by tau phosphorylation at the sites hyperphosphorylated in Alzheimer’s disease. J. Neurosci. 32, 2430–2441. doi: 10.1523/JNEUROSCI.5927-11.2012
Singh, I. N., Sullivan, P. G., Deng, Y., Mbye, L. H., and Hall, E. D. (2006). Time course of post-traumatic mitochondrial oxidative damage and dysfunction in a mouse model of focal traumatic brain injury: implications for neuroprotective therapy. J. Cereb. Blood Flow. Metab. 26, 1407–1418. doi: 10.1038/sj.jcbfm.9600297
Sivanandam, T. M., and Thakur, M. K. (2012). Traumatic brain injury: a risk factor for Alzheimer’s disease. Neurosci. Biobehav. Rev. 36, 1376–1381. doi: 10.1016/j.neubiorev.2012.02.013
Strubakos, C. D., Malik, M., Wider, J. M., Lee, I., Reynolds, C. A., Mitsias, P., et al. (2020). Non-invasive treatment with near-infrared light: a novel mechanisms-based strategy that evokes sustained reduction in brain injury after stroke. J. Cereb. Blood Flow. Metab. 40, 833–844. doi: 10.1177/0271678X19845149
Swanson, L. C., Rimkus, S. A., Ganetzky, B., and Wassarman, D. A. (2020). Loss of the antimicrobial peptide mechnikowin protects against traumatic brain injury outcomes in Drosophila melanogaster. G3 (Bethesda) 10, 3109–3119. doi: 10.1534/g3.120.401377
Taylor, C. A., Bell, J. M., Breiding, M. J., and Xu, L. (2017). Traumatic brain injury–related emergency department visits, hospitalizations, and deaths — United States, 2007 and 2013. Surveil. Summ. 66, 1–16.
van Alphen, B., Stewart, S., Iwanaszko, M., Fangke, X., Bang, E., Rozenfeld, S., et al. (2018). Glial immune-related pathways as mediators of closed head TBI effects on behavior in Drosophila. BioRxiv [Preprint] doi: 10.1101/422535
Ventura-Clapier, R., Moulin, M., Piquereau, J., Lemaire, C., Mericskay, M., Veksler, V., et al. (2017). Mitochondria: a central target for sex differences in pathologies. Clin. Sci. (Lond) 131, 803–822. doi: 10.1042/CS20160485
Vos, M., Lovisa, B., Geens, A., Morais, V. A., Wagnieres, G., van den Bergh, H., et al. (2013). Near-infrared 808 nm light boosts complex IV-dependent respiration and rescues a Parkinson-related pink1 model. PLoS One 8:e78562. doi: 10.1371/journal.pone.0078562
Walker, M. A., Volpi, S., Sims, K. B., Walter, J. E., and Traggiai, E. (2014). Powering the immune system: mitochondria in immune function and deficiency. J. Immunol. Res. 2014:164309. doi: 10.1155/2014/164309
Watts, L. T. (2016). Stimulating mitochondria to protect the brain following traumatic brain injury. Neural. Regen. Res. 11, 1403–1404. doi: 10.4103/1673-5374.191205
Weinrich, T. W., Coyne, A., Salt, T. E., Hogg, C., and Jeffery, G. (2017). Improving mitochondrial function significantly reduces metabolic, visual, motor and cognitive decline in aged Drosophila melanogaster. Neurobiol. Aging 60, 34–43. doi: 10.1016/j.neurobiolaging.2017.08.016
West, C., and Silverman, N. (2017). Drosophilosophical: re-thinking adaptive immunity in the fly. Cell 169, 188–190. doi: 10.1016/j.cell.2017.03.032
Xiong, Y., Gu, Q., Peterson, P. L., Muizelaar, J. P., and Lee, C. P. (1997). Mitochondrial dysfunction and calcium perturbation induced by traumatic brain injury. J. Neurotrauma. 14, 23–34. doi: 10.1089/neu.1997.14.23
Keywords: traumatic brain injury, sex-differences, gene expression, near-infrared light, mitochondria
Citation: Shah EJ, Hüttemann M, Sanderson TH, Gurdziel K and Ruden DM (2021) Inhibiting Mitochondrial Cytochrome c Oxidase Downregulates Gene Transcription After Traumatic Brain Injury in Drosophila. Front. Physiol. 12:628777. doi: 10.3389/fphys.2021.628777
Received: 12 November 2020; Accepted: 23 February 2021;
Published: 15 March 2021.
Edited by:
Cesare Indiveri, University of Calabria, ItalyReviewed by:
Pamela J. VandeVord, Virginia Tech, United StatesLeonardo Salviati, University of Padua, Italy
Copyright © 2021 Shah, Hüttemann, Sanderson, Gurdziel and Ruden. This is an open-access article distributed under the terms of the Creative Commons Attribution License (CC BY). The use, distribution or reproduction in other forums is permitted, provided the original author(s) and the copyright owner(s) are credited and that the original publication in this journal is cited, in accordance with accepted academic practice. No use, distribution or reproduction is permitted which does not comply with these terms.
*Correspondence: Katherine Gurdziel, Z3VyZHppZWxAd2F5bmUuZWR1; Douglas M. Ruden, ZG91Z2xhcy5ydWRlbkBnbWFpbC5jb20=
†These authors share senior authorship