- Medical Research Council-Versus Arthritis Centre for Musculoskeletal Ageing Research and Nottingham National Institute for Health Research Nottingham Biomedical Research Centre, School of Medicine, University of Nottingham, Derby, United Kingdom
Maintenance of skeletal muscle mass throughout the life course is key for the regulation of health, with physical activity a critical component of this, in part, due to its influence upon key hormones such as testosterone, estrogen, growth hormone (GH), and insulin-like growth factor (IGF). Despite the importance of these hormones for the regulation of skeletal muscle mass in response to different types of exercise, their interaction with the processes controlling muscle mass remain unclear. This review presents evidence on the importance of these hormones in the regulation of skeletal muscle mass and their responses, and involvement in muscle adaptation to resistance exercise. Highlighting the key role testosterone plays as a primary anabolic hormone in muscle adaptation following exercise training, through its interaction with anabolic signaling pathways and other hormones via the androgen receptor (AR), this review also describes the potential importance of fluctuations in other hormones such as GH and IGF-1 in concert with dietary amino acid availability; and the role of estrogen, under the influence of the menstrual cycle and menopause, being especially important in adaptive exercise responses in women. Finally, the downstream mechanisms by which these hormones impact regulation of muscle protein turnover (synthesis and breakdown), and thus muscle mass are discussed. Advances in our understanding of hormones that impact protein turnover throughout life offers great relevance, not just for athletes, but also for the general and clinical populations alike.
Introduction
Skeletal muscle accounts for ~40–45% of total body mass (Romagnoli et al., 2020). Following a rapid post-natal growth phase, skeletal muscle mass is typically maintained at a steady state in adulthood through a controlled balance between muscle protein synthesis (MPS) and breakdown (MPB)—unless in the presence of physiological (exercise) or pathological (age or disease) stimuli. The mitigation of age and disease-related muscle wasting and dysfunction remains a major research effort. Even today after significant efforts to develop pharmaceutical strategies to mitigate muscle wasting (Sepulveda et al., 2015), contractile activity in the form of resistance exercise (RE) remains the most efficacious intervention. RE training (RET) leads to muscle hypertrophy through a sustained elevation of MPS (Hooper et al., 2017)—responses which are driven by a combination of mechanical overload, and an associated release of hormones; e.g., androgens and insulin-like growth factor-1 (IGF-1) (Romagnoli et al., 2020) and by muscle mechano-sensitive signals.
While the fundamental roles of hormones in muscle development and their decline in aging are well-established, the impact of physiological fluctuations (e.g., due to circadian rhythms or transient increases following bouts of RE) in hormones remains unclear (Schroeder et al., 2013). RE induces marked anabolic hormone responses, in particular those involving testosterone, growth hormone (GH) and IGF-1 (Spiering et al., 2008). These hormonal elevations in response to RE take place in a unique physiological environment, whereby acute elevations in circulating blood hormone concentrations, resulting from a combination of either e.g., increased secretion, reduced hepatic clearance, reduced plasma volume or reduced degradation rates—interact with receptors on the target tissue cell membranes or with nuclear/cytoplasmic receptors located within the target tissue (e.g., steroid receptors); which, alongside mechano-signaling, initiates a sequence of molecular events, leading to muscle adaptive responses such as an increase in MPS and/or a decrease in MPB (Kraemer and Ratamess, 2005). Given the apparent complexity of RE-induced hormonal responses and their impact on muscle adaptation, we aim to provide an update on advances in this area.
Testosterone
The principal androgen, testosterone, is an anabolic-androgenic steroid hormone which is synthesized from cholesterol—produced mainly in Leydig cells in men, and the ovary (25%) and adrenalzona fasciculata (25%) in women, via conversion from progesterone, with the remaining ~50% being produced from circulating androstenedione (Burger, 2002). Homeostatic processes maintain systemic testosterone levels within the range of 7.7–29.4 nmol.L−1 in healthy young men and 0.1–1.7 nmol.L−1 in healthy menstruating women under 40 y (Handelsman et al., 2018). In contrast, there are no differences observed between men and women in relation to intramuscular testosterone concentrations and steroidogenic enzymes (Vingren et al., 2008). Systemic testosterone is taken up by the muscle through its binding to membrane-bound or cytoplasmic androgen receptors (AR), in turn stimulating subsequent myocellular signaling (Vingren et al., 2010; Kraemer et al., 2020) and altering the expression of thousands of genes, many of which are involved in the regulation of skeletal muscle structure, fiber type (Dubois et al., 2014), intramyocellular metabolism (White et al., 2013), and mRNA transcription (MacLean et al., 2008) (Figure 1). Once bound to the AR, testosterone is irreversibly converted to dihydrotestosterone (DHT) through the enzymatic action of 5-α reductase (Wilborn et al., 2010). It is generally accepted that DHT is the more potent hormone due to its receptor binding kinetics (Ly et al., 2001); however, testosterone has also been shown to regulate a multitude of ergogenic, anabolic, and anti-catabolic functions in skeletal muscle, without prior conversion to DHT (Bhasin et al., 2003). Testosterone also effects the development of bone, connective and neural tissues (Hoffman et al., 2009), leading to increased muscle strength, power, endurance, and hypertrophy in a dose-dependent manner (Sinha-Hikim et al., 2006; Kraemer et al., 2017). The importance of androgens for mediating muscle growth are substantiated through numerous lines of evidence, including: (1) that exogenous administration, potentiates gains in muscle strength and muscle mass (Gharahdaghi et al., 2019); (2) that gonadotropin-releasing hormone analogs, which inhibit endogenous testosterone release, prevent gains in muscle strength and attenuate gains in muscle mass (Kvorning et al., 2007); and (3) that AR antagonists, which inhibit endogenous testosterone from binding to the AR, impair radical muscle growth during synergist overload (Inoue et al., 1994). Together, these findings suggest a significant role for testosterone in regulating adult muscle growth in response to mechanical loading (i.e., RE).
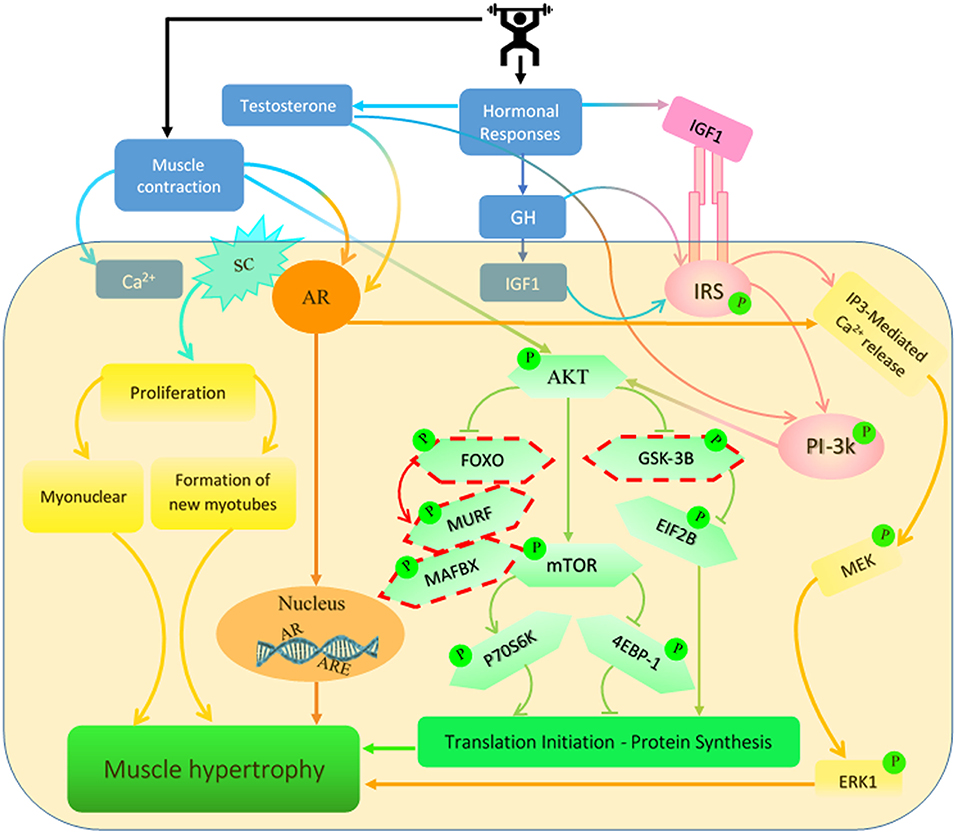
Figure 1. Signaling pathways regulated by testosterone, growth hormone (GH) and insulin-like growth factor 1 (IGF-1) are induced by resistance exercise (RE). RE has been shown to increase the concentration of these hormones which activate several different signaling pathways in the muscle. These pathways lead to increases in muscle protein synthesis (MPS) and net protein accretion which result in an increase in muscle mass. SC, satellite cell; AR, androgen receptor; IRS, insulin receptor substrate; ARE, androgen response element. *Dashed outline represents inhibitory protein cascades.
Exercise-Induced Testosterone Release, and Links With Muscle Adaptation by Sex and Age
The relationship between RE and testosterone responses have been extensively reviewed in young men (Ahtiainen et al., 2003, 2005; West et al., 2009; West and Phillips, 2012), with the majority of studies suggesting that it is the acute transient elevations in testosterone that likely drive the proposed hormonal adaptations associated with muscle growth. For example, immediately following RE, serum testosterone levels peak [~from 13 (resting levels) to 38 (at ~30 mins) nmol.L−1] with a concomitant upregulation of AR mRNA and protein content within the muscle (Willoughby and Taylor, 2004; Hooper et al., 2017). It seems high intensity RE stimulates basophilic cells of the anterior pituitary to release luteinizing hormone (LH) from gonadotrophs in the anterior pituitary which then acts as the primary regulator of testosterone secretion from the Leydig cells of the testes (Fry and Kraemer, 1997). High affinity binding of LH at the Leydig cells of the testes activates a cyclic adenosine mono phosphate (cAMP) mechanism, resulting in increased testosterone synthesis (Dufau and Catt, 1979; Fry and Kraemer, 1997). This activation is dependent on the frequency and amplitude of LH secretion, with pulses occurring in the human at the rate of ~8–14 pulses.day−1 in men. Secretion of LH is principally regulated by LH releasing hormone (LHRH) via portal circulation from the hypothalamus. High affinity binding of LHRH in the anterior pituitary activates LH secretion by a calcium-dependent mechanism, resulting in LH secretion (Dufau and Catt, 1979; Fry and Kraemer, 1997). Further, early increased circulating testosterone levels during RE are also LH-independent and it seems they may be directly stimulated via increases in lactate levels induced by an increase in the production of cAMP in testicular tissues (Lin et al., 2001). However, the mechanisms of lactate action on testosterone production by Leydig cells are not clear yet. While the acute response of testosterone returns to baseline rapidly post exercise and has been shown to not be elevated chronically following repeated bouts of RE (Hooper et al., 2017); the acute upregulation of AR mRNA and protein content can last up to 1–2 days post RE (Ratamess et al., 2005), thereby augmenting testosterone uptake into the muscle, and potentiating the anabolic effects of testosterone over longer periods (Murphy and Koehler, 2020; Tinline-Goodfellow et al., 2020). It therefore may be that the combined effects of acute testosterone elevation post exercise and sustained AR upregulation in the muscle may represent an additional mechanism through which RE might regulate muscle growth. Notably, while some studies have indicated correlative relationships between RE-induced elevations in testosterone and muscle strength and hypertrophy (Hansen et al., 2001; Ahtiainen et al., 2003, 2005), this remains equivocal (West et al., 2009; West and Phillips, 2012) perhaps since the magnitude of acute responses in young males can be influenced by many factors e.g., timing of sampling etc. Additionally, there is evidence that RE loads of <70% 1-RM (Tremblay et al., 2004; Yarrow et al., 2007; Fry and Lohnes, 2010; Hough et al., 2011); programs incorporating only upper body exercises, even at a relatively high intensity and volume (Migiano et al., 2010); and those with the long rest periods between repetitions do not stimulate a significant post exercise testosterone response (McCaulley et al., 2009), despite post exercise increases in MPS, anabolic signaling and associated muscle growth adaptive responses being observed (West et al., 2009; West and Phillips, 2012). In an attempt to better understand the discrepancies between testosterone and muscle adaptive responses, Phillips and colleagues devised a unique experimental approach, whereby they compared a “high” vs. “low” hormone environment (induced by working distinct muscle bulk) (West et al., 2010). Despite the contrasting hormonal profiles and significantly different acute testosterone responses in these environments, muscle mass and strength gains were comparable, suggesting that the role of testosterone (and other hormones) in exercise induced muscle adaptation is minimal. Conversely, other studies using similar procedures reported that the “high” hormone environment potentiated muscle strength gains after 9 weeks RET (Hansen et al., 2001) and acute AR content 180 min after RE (Spiering et al., 2009), which was associated with increased MPS, muscle growth and recovery (Sheffield-Moore, 2000). The differences in outcomes between these studies may be driven by the experimental design (different biopsy location; i.e., biceps brachii vs. vastus lateralis and different testosterone inducing exercise regimes, which resulted in different peak testosterone; i.e., 27 (West et al., 2010) vs. 38 nmol.L−1 (Spiering et al., 2009) and also the time course of muscle sampling (between 3 and 4 h post-RE). These differences may be important since the duration and magnitude of testosterone and AR elevation and AR exposure to testosterone appear to play a crucial role in skeletal muscle adaptations both in vitro (Bloomer et al., 2000) and in vivo (Antonio et al., 1999; Ferrando et al., 2002). In turn, as the pituitary-gonadal axis works in a negative feedback loop, increasing AR content will likely result in enhanced tissue uptake of testosterone, thus lowering circulating testosterone. Reductions in circulating testosterone concentrations (due to enhanced cellular uptake) are monitored by the hypothalamus, which releases gonadotropin-releasing hormone (GnRH) to stimulate LH secretion, then testosterone synthesis/secretion (Kraemer et al., 2006). Herein, it is suggested that AR protein content itself may be critical in RE-induced skeletal muscle protein accretion, with AR content, and not circulating testosterone being more closely associated with adaptations in muscle mass (Morton et al., 2018) and fiber CSA (Mitchell et al., 2013) in young men.
Whilst the majority of investigations into the role of testosterone in muscle adaptive response have been performed in males (reflecting male biology), the importance of circulating concentrations of testosterone in adult women should not be underestimated based on its biological role in the conversion of progesterone to the principal oestrogens—oestradiol and oestrone (Cui et al., 2013). That being said, the importance of testosterone in women remains unclear since while there is an indispensable role e.g., on bone health, in older males (Mohamad et al., 2016), a reduction in testosterone generally does not occur independently of other hormones (such as the oestrogens) in females (e.g., following the menopause) (Chakravarti et al., 1976). Moreover, females do not have Leydig cells; the cells which are likely the source of the acute RE-induced increase in testosterone in men (Kvorning et al., 2007). That said, increases in testosterone in females have been reported in response to RE in some (Nindl et al., 2001; Copeland et al., 2002), but not all (Marx et al., 2001; Linnamo et al., 2005) studies, albeit with claims of no, or limited, effects of acute testosterone elevations in relation to muscle growth in women (Kraemer et al., 2017). Therefore, the links between the testosterone response and exercise adaptation in women remain contentious and require further investigation.
Finally, it is important when overviewing the role of testosterone in controlling muscle mass, to consider older adults. With aging, there is a linear decline in bioavailable circulating testosterone in both men and women (Kraemer et al., 1998; Hakkinen et al., 2000), with these reductions leading to osteoporosis in both sexes (Mohamad et al., 2016). Testosterone is ~98% bound to serum proteins (sex hormone-binding globulin (SHBG) and albumin) and only 1–2% of testosterone is unbound or free. Because testosterone is bound to SHBG with high affinity, it is not available to most tissues for action. Given the concentration of SHBG increases across the lifespan in men (Liu et al., 2007) and only increase after ~60 y in women (Maggio et al., 2008), bioavailable testosterone (free plus albumin-bound testosterone) concentrations decline even more markedly than total testosterone levels with aging (Matsumoto, 2002). This reduction can eventually lead to very low resting concentrations of circulating testosterone particularly in men, creating the so-called andropause (Vingren et al., 2010). Intriguingly, this reduction in testosterone tracks with the gradual decline in muscle mass observed with age, i.e., ~1–3% decline in circulating testosterone and 1–2% loss of muscle mass in men (Vingren et al., 2010; Gharahdaghi et al., 2019), perhaps suggesting declines in endogenous testosterone may be linked to loss of muscle mass. Moreover, while RE in older men (> 59 yr old) still elicits an acute elevation in circulating testosterone, the magnitude of elevation is smaller than that of younger men performing the same RE (Kraemer et al., 1998; Hakkinen et al., 2000), i.e., ~1 nmol.L−1 in young vs. ~0.1 nmol.L−1 in older men (Brook et al., 2016), ostensibly leading to reduced muscle AR content, MPS and ultimately blunted muscle adaptive responses to chronic RET (Brook et al., 2016; Gharahdaghi et al., 2019). With provision of exogenous testosterone helping to restore this blunting somewhat (Gharahdaghi et al., 2019), the influence of testosterone on muscle may be small and permissive in the young, but the need for hormonal input for the control of muscle mass may be more important as we age to overcome age-related deficits in the responsiveness of older muscle to exercise training.
Metabolic and Molecular Effects of Testosterone in Skeletal Muscle
Anabolic effects of AR and testosterone upregulation after RE occur through a combination of both genomic i.e., transcriptional capacity, and non-genomic i.e., translational efficiency, pathways (Kraemer et al., 2020). RE and androgens up-regulate muscle AR content via distinct mechanisms; RE increases AR mRNA transcription via RhoA (a member of the Rho family of small GTPases which is involved in muscle transcription factor) and serum response factor (MADS-box transcription factor which is essential for muscle-specific gene expression) signaling (Lee et al., 2003); in contrast, testosterone increases muscle AR via enhanced AR mRNA association with polyribosomes, increasing AR mRNA translation (Mora and Mahesh, 1999) and doubling AR half-life from ~3.1 to 6.6 h (Syms et al., 1985). Due to these contrasting mechanisms of action, a combination of RE and RE-induced testosterone secretion will likely potentiate post exercise AR responses for longer, thereby augmenting adaptive muscle growth. Conversely, impaired testosterone responsiveness to RE in older adults, likely attenuates the AR response, due to lack of testosterone mediated AR increases, and subsequently, limits muscle mass gains with RET. When testosterone binds to the AR, the AR transforms, dimerizes and translocates to the nucleus, binding to androgen-response elements (ARE) therein, as a homodimer. Activation of these AREs stimulates the transcription of protein targets and other anabolic systems, such as the local production of IGF-1 which is related to muscle protein accretion through a decrease in IGFBP-4 mRNA concentration coupled with an increase in IGF-1 mRNA (Bamman et al., 2001; West et al., 2010) (see IGF-1 section). In addition to these genomic signaling pathways, testosterone is thought to independently activate the Akt/mTOR/S6K1 pathway, which represents an integrated step in the hypertrophic response (Basualto-Alarcón et al., 2013); and also through activation of G protein-linked membrane receptors, which result in calcium dependent phosphorylation of ERK1/2 (a mitogen-activated protein kinases), potentially leading to the phosphorylation of transcription factors associated with cellular growth (Kadi, 2008). However, transient activation of ERK1/2 induced by testosterone was not found to be directly related to the hypertrophic signaling cascade; though activated ERK can phosphorylate co-activators of the intracellular receptor at the nuclear level (Bratton et al., 2012), through potentiation of estrogen receptor activation function 1 (AF-1) by Src/JNK (serine 118-Independent pathway) which promotes cellular growth (Feng et al., 2001; Estrada et al., 2003). In sum, the combined effects of RE and RE-induced testosterone release induced upregulation of AR anabolism is driven via genomic and non-genomic signaling pathways which likely augment protein turnover in muscle resulting in increases in net protein accretion and hypertrophy (Wolfe et al., 2000; Roberts et al., 2018).
Metabolic and Molecular Effects of Exercise on Oestrogen in Skeletal Muscle
Oestrogens are steroid hormones, primarily produced in the ovaries from testosterone via an aromatase enzyme, of which women have four times the amount compared with men, until the menopause (Hansen and Kjaer, 2014). While less studied in this sphere, endogenous oestrogens seem to have a metabolic role in regulating skeletal muscle; for instance, being critical for the regrowth of atrophied skeletal muscle (Sitnick et al., 2006)- an action mediated by the estrogen receptors, located within skeletal muscle tissue that function as transcription factors (Hansen and Kjaer, 2014). Indeed, as with testosterone (and perhaps as a function of its metabolic regulation through testosterone), estrogen is believed to be important in the regulation of both muscle function (Chidi-Ogbolu and Baar, 2019) and hypertrophy in response to exercise—with rapid changes in systemic concentration occurring immediately post RE, that are dependent upon RE intensity (Copeland et al., 2002), however, baseline circulating concentrations are unaltered following chronic RET for up to 6 months (Gil et al., 2012; Yoon et al., 2018). It is reported that RE acutely augments the activity of the aromatase enzyme which results in an increase in the biosynthesis of estrogen from androgens (Nelson and Bulun, 2001; Luk et al., 2015); in turn explaining the effects of RE-induced testosterone increase on an increase in estrogen levels in women (Luk et al., 2015). The effects of acute estrogen release may relate to a reduction in exercise-induced muscle damage and improved recovery (Hansen, 2018), possibly via its indirect antioxidant properties and stabilization of cell membranes (Paroo et al., 2002) and decreased post-exercise production of protein chaperones- i.e., heat shock protein (HSP) 72 (Paroo et al., 1999) and HSP70 (Enns and Tiidus, 2010). HSPs act as an index of cellular damage and activate inflammatory cell populations (e.g., neutrophils and macrophages) thereby regulating the extent of inflammatory responses after muscle injury (Senf et al., 2013). In addition, estrogen is also known to activate insulin/IGF-1 (Lee et al., 2004) and PI3K/Akt (Mangan et al., 2014) pathways, potentially enhancing the mechanisms regulating MPS (Hansen et al., 2012) and consequently muscle growth (Smith et al., 2014). The latter is suggested to occur through increased expression of Pax7 and MyoD transcription factors (Thomas et al., 2010; Sambasivan et al., 2011) which induce satellite cell expansion, differentiation, and self-renewal of muscle function and mass (Kitajima and Ono, 2016; Chidi-Ogbolu and Baar, 2019). Given that estrogen stimulates post-RE myogenesis, decreased estrogen levels in post-menopausal women may be a contributing factor to the development of sarcopenia, diminishing the rate of muscle repair and adaptive capacity in older women (Thomas et al., 2010). Indeed estrogen replacement has been shown to attenuate the age-related decline in muscle mass observed in postmenopausal women (Enns and Tiidus, 2010). However, the proposed effects of estrogen may be defined by the stage of the menstrual cycle. For example, low estrogen in the early follicular stage, may negatively affect RE-induced increases in estrogen levels (Hansen et al., 2012), while, in the luteal phase where circulating progesterone is relatively high, may also counteract the sensitizing effects of estrogen on muscle impairing any benefit of acute RE-induced during these phases (Hansen, 2018). In contrast, RET in the late part of the follicular phase, when circulating estrogen is enhanced, appears to result in increased fiber type II CSA, nuclei to fiber ratio and muscle mass, compared to RET during luteal phase (Sung et al., 2014; Wikström-Frisén et al., 2017). However, this has not been confirmed (Miller et al., 2006; Sakamaki-Sunaga et al., 2016) as no differences between follicular phase and luteal phase RET responses have also been observed, at least with regard to strength gains and hypertrophy; and as such, the role of estrogen in mediating responses to RE, remains unclear. Future trials are needed to clarify the effects of the oestrogens on muscle biology under different conditions e.g., phase of menstrual cycle, pre or post-menopause, and the response to nutrition (fasting/feeding) and exercise training (Hansen, 2018).
Growth Hormone (GH), Exercise, and Effects on Muscle Metabolism
Human growth hormone (GH) is secreted from somatotroph cells of the anterior pituitary. It is released in 6–8 bursts.day−1, with negligible secretion outside of these bursts, and is under the control of the hypothalamic hormones: GH-releasing hormone (GHRH), which promotes secretion, and somatostatin which inhibits release of GH (Giustina et al., 2008). Following GH release, which induces the hepatic generation of IGF-1, circulating levels of IGF-1 and GH, feedback to the hypothalamus to inhibit further GH secretion (Daughaday, 2000). RE is the most potent physiological stimulus for GH release in both men (Nicholls and Holt, 2016; Fink et al., 2017) and women (Hymer et al., 2001), but little is known about how RE alters somatotroph content and function. GH begins to rise 10–20 min after commencing RE and peaks at the end of the RE (Gibney et al., 2007; Fink et al., 2018a), returning to baseline values around 60 min post RE (Häkkinen and Pakarinen, 1995; Fink et al., 2018a). RE increases the amplitude of each GH pulse rather than the frequency. Accordingly, whole-body RE induces GH increases from basal levels of 5 ug.L−1 (Fink et al., 2018b) to 24 ug.L−1 (Kraemer et al., 1990) while localized RE of individual muscle groups (biceps and triceps) leads to increases of only half of this; up to 12 ug.L−1 (Fink et al., 2018a). These increases in post RE GH levels are blunted in older adults, and a progressive decline in GH secretion and clearance is observed after the age of 40 y (Zaccaria et al., 1999). In younger adults, exercise-induced GH release is relatively non-specific occurring in response to both RE and aerobic exercise (e.g., 60% VO2 max) (Godfrey et al., 2003). It has also been reported that when lactate is elevated beyond anaerobic threshold, which is associated with greater demands on anaerobic glycolysis, the hypothalamus is highly stimulated (Kraemer et al., 1990, 1993; Hartman et al., 1993). This is further supported during RE protocols of moderate intensity (10-RM vs. 5-RM) with short rest periods (1 vs. 3 min) between sets (Hoffman et al., 2003), which result in higher circulating concentrations of GH in both men and women (Kraemer et al., 1993). The exact mechanism of exercise-induced GH release remain ill-defined, however are likely driven via higher intensities of RE directly stimulating the anterior pituitary, facilitated via increasing circulating of catecholamines, lactate, nitric oxide and changes in acid-base balance (Godfrey et al., 2003). Increases in post RE GH levels have also been associated with increased estrogen levels in women; i.e., area under curve (AUC) of increase in GH after RE was higher in midluteal phase than increases in early follicular phases of menstrual cycle (Nakamura et al., 2011). It seems there is a close interplay between estrogen levels and GH secretion and prevailing estrogen concentrations may modulate both GH secretion and action (Leung et al., 2004). This effect of estrogen may be due to a combination of a reduction of somatostatin's inhibitory tone, amplification of endogenous GHRH levels or its pituitary actions, and activation of additional mechanisms; e.g., estrogen stimulates GH secretion by decreasing liver secretion of IGF-1, resulting in stimulation of the pituitary to synthesize and secrete GH (Cook, 2004; Nakamura and Aizawa, 2017).
The physiological relevance of increases in GH levels after RE may be increases in protein synthesis and its ability to aid in muscle repair (Gibney et al., 2007; Liu et al., 2008) and impact on muscle mass (Hermansen et al., 2017), without any impact on muscle function (Hermansen et al., 2017). It was reported that there is a correlation between acute RE-induced GH increases and long term muscle and fiber type I and II hypertrophy (McCall et al., 1999). GH per se has also been associated with whole body protein synthesis (e.g., reflecting in part, connective tissues); but directly, may not augment MPS (Doessing et al., 2010; West et al., 2010). Conversely, acute GH infusion studies (eliciting a similar to post RE increase in GH levels) have shown this hormone may have a role in stimulating MPS (Fryburg et al., 1991; Fryburg and Barrett, 1993) while associated increases in insulin inhibit MPB (Fryburg et al., 1992). Thus, while GH is a positive regulator of extracellular matrix (ECM) synthesis (Kragstrup et al., 2011) which is important in morphogenesis (Rozario and DeSimone, 2010), there is still debate surrounding its role in the regulation of muscle mass in adults; however what may be key is the lack of effect on muscle function regardless of its impact on growth pathways and MPS.
In terms of mechanisms, following release, GH binds to its receptor leading to the recruitment and phosphorylation of Janus kinase 2 (JAK2) and its most recognized downstream target, signal transducer and activator of transcription 5 (STAT5) (Jørgensen et al., 2006). In addition, GH stimulates the IRS1/Akt (Costoya et al., 1999; Consitt et al., 2017) and mitogen-activated protein kinase (MAPK) pathways which are thought to be the main pathways contributing to GH/IGF-1-induced muscle hypertrophy via p42/p44 and p38 pathways (Consitt et al., 2017) (Figure 1). The activation of Akt results in skeletal muscle growth/maintenance since it controls the phosphorylation of a number of substrates involved in MPS including mTOR (and its downstream targets 4E-binding protein 1 (4E-BP1) and p70S6 kinase) and glycogen synthase kinase 3β (GSK3β), as well as, the inhibition of protein degradation via the forkhead transcription factor (FOXO) pathway (Consitt et al., 2017). To support these molecular effects that GH has on muscle mass, GH receptor knock-out results in a decrease in myofiber CSA and muscle mass loss in mice (Sotiropoulos et al., 2006). Nevertheless, as these mice had a reduction in circulating IGF-1 and tissue IGF-1 expression; at least in part GH dependent, it is difficult to separate the effects of the two hormones (Velloso, 2008) and further investigations are needed to clarify the main effects of GH on muscle growth in adults, in particular after RE. However, the main muscle anabolic effects of GH are believed to be indirect—via inducing the hepatic generation of IGF-1 triggering the IGF-1-Akt-mTOR pathway; in turn resulting in MPS augmentation and as a consequence muscle maintenance and growth (Sandri et al., 2013; Schiaffino et al., 2013).
IGF-1, Exercise, and Effects on Muscle Metabolism
As previously discussed, GH acts through its receptor; however, many effects linked to RE and muscle growth are believed to act indirectly through an increase in hepatic release of IGF-1. IGF-1 can also promote muscle growth in the absence of GH; and unlike GH, IGF-1 is critical for intrauterine growth (Velloso, 2008). A liver-specific knockout mouse exhibited some postnatal growth reduction, but not as severe as with global IGF knockout (Baker et al., 1993; Tahimic et al., 2013). Bikle et al. also showed muscle atrophy was more pronounced after ablation of muscle IGF-1 production than when hepatic IGF-1 production was suppressed (Bikle et al., 2015); exhibiting circulating levels of IGF-1 (i.e., endocrine factor) do not effect overall growth responses (Ohlsson et al., 2000; Velloso, 2008). This implies that locally produced, autocrine/paracrine IGF-1 plays an important role in both pre- and postnatal growth. The local production of IGF-1 is controlled primarily by GH and other hormones (e.g., parathyroid and thyroid hormones) (Bikle et al., 2015); suggesting GH's effect on growth may be mediated in part via increased local IGF-1 production and/or action. These results indicated GH has local effects that may be independent of increased levels of the circulating IGF-I (Ohlsson et al., 2009). However, a role for circulating liver-derived IGF-I could not be excluded. Reflecting this, it has been reported that IGF-1 levels are associated with improvements in handgrip strength and physical performance as well as life-span (Birnie et al., 2012; Yusuf et al., 2020); in addition, higher circulating IGF-1 has been linked with increases in MPS, muscle free fatty acid utilization, and improvements insulin sensitivity (Kraemer et al., 2017), which may explain its often linked importance in exercise training adaptations. Systemic IGF-1 levels are rapidly increased in humans in response to RE from ~45 (resting levels) to 65 nM (immediately after RE) (Schwarz et al., 1996; West et al., 2009; Ogasawara et al., 2013) and return to baseline levels ~30 min after RE (Kraemer et al., 2017), which may play an important role for exercise-induced hypertrophy (Kido et al., 2016), neurogenesis (Trejo et al., 2001), and improved muscle strength with RET (Bjersing et al., 2017) by improving translational efficiency (Schiaffino and Mammucari, 2011) and satellite cell proliferation (Velloso, 2008) in muscle and in the central nervous system (CNS) (Mainardi et al., 2015). GH-induced IGF-1 released from the liver in response to RE is involved in two negative feedback loops. One directly affects the somatotropic cells of the anterior pituitary, itself inhibiting further release of GH, whilst the other affects GH releasing hormone and somatostatin release from the hypothalamus to reduce the secretion of GH. Repeated bouts of RE resulted in an exercise-induced GH response to each acute exercise episode, thereby increasing the 24-h secretion of GH and then IGF-1. Thus, exercise counters negative feedback and so IGF-1 secretion is maintained or increased (Godfrey et al., 2003). Further, to what we already showed [i.e., testosterone increased IGF-1 gene expression during RET (Gharahdaghi et al., 2019)], both testosterone and estrogen blunted IGF-I feedback-dependent inhibition of GH secretion (Veldhuis et al., 2004, 2005); and as it was reported in prepubertal boys, lead to increase in GH and then IGF-1 levels; which in turn exhibit the further and indirect anabolic links between androgens and muscle growth (Mauras et al., 2003). Like testosterone levels, older adults experience a lower basal level of IGF-1 (the so-called somatopause which refers to the diminishment of the GH-IGF-1 system) which attenuates post-RE levels of IGF-1 (Kraemer et al., 1999). However, RE increases IGF-1 mRNA and IGF-1 peptide production in younger adults which result in increases in muscle DNA and protein content (Adams and Haddad, 1996). Increased expression of IGF-1 in muscle leads to muscle hypertrophy in mice; which is independent of effects of circulating levels of IGF-1 (Coleman et al., 1995). Therefore, serum levels of IGF-1 (resting levels or acutely after RE) may not be a good reflection of local effects of IGF-1 (Bartke and Darcy, 2017; Van Nieuwpoort et al., 2018), especially in those tissues that have capabilities of producing the hormone themselves, such as skeletal muscle (Barclay et al., 2019). Indeed, circulating IGF-1 levels have even been shown to decrease during periods of active muscle building, likely due to a redistribution of IGF-1 from the circulation into the muscle (Arnarson et al., 2015). If such a sequestration of IGF-1 into muscle increases during RE (with a decrease in cellular GH receptors), it might occur as a result of reduced GH-induced hepatic production (Eliakim et al., 1998) and it may be speculated that the effect would be more pronounced in individuals experiencing greater activation of intracellular muscle signaling and subsequent muscle hypertrophy and performance (Velloso, 2008; Arnarson et al., 2015; Morton et al., 2016). This suggests that intrinsic secretion (i.e. autocrine) of muscle IGF-1, beside circulating IGF-1, may be a determinant for switching on anabolic pathways (Morton et al., 2018) and fusion of satellite cells (Velloso, 2008).
Given the fairly short half-life of unbound IGF-1 in serum (i.e., 5–10 min), binding to an IGF binding protein (IGFBP-3 is the most prevalent) in serum or in ECM increases IGF-1 half-life to around 25 min (Allard and Duan, 2018). In addition, IGFBPs are important in potentiating IGF-1 anabolic signaling. The potentiating action occurs when the IGF-1-IGFBP binds to the target cell's ECM components, which results in activation of IGF-1 receptor (IGFR) and then IGF-1 enters the cell and triggers phosphoinositide 3-kinase (P13-K) to generate phosphatidylinositol-bisphosphate (PIP2) (Pinedo-Villanueva et al., 2019), leading to the production of phosphatidylinositol 3,4,5-trisphosphate (PIP3) (O'Neill et al., 2015). PIP3 is then free to bind to phosphoinositide-dependent kinase-1 (PDK1) which activates the Akt-mTORC1 pathway (Schiaffino and Mammucari, 2011) promoting ribosomal biogenesis and translation to permit increases in MPS and the formation myofibrillar proteins, which allows muscle mass growth (Menon et al., 2014; Wen et al., 2016) (Figure 1). Similar to GH, IGF-1 alone stimulates the IRS1/Akt (Costoya et al., 1999; Consitt et al., 2017) and mitogen-activated protein kinase (MAPK) pathways which are thought to be main pathways contributing to GH/IGF-1-induced muscle hypertrophy (Consitt et al., 2017). Also, RE-induced IGF1-Akt activation phosphorylates AS160 (Akt substrate of 160 kDa) resulting in enhanced GLUT4 translocation and glucose uptake, reflecting the mediator role of IGF-1 in glycaemic control via insulin-IGF-1-Akt pathway activation in muscle (Kido et al., 2016). Taken together, IGF-1 signaling, including prolonged Akt and AS160 phosphorylation, may be a specific signal response to acute RE; which transduces mechanical signals leading to anabolic responses and allow IGF-1 signaling to stimulate the competing processes of muscle cellular growth.
Conclusion
RE-induced increases in key endogenous steroid and peptide hormone responses are likely to be an integral part of the integrated response to acute exercise and exercise-induced muscle growth. The combined effects of RE and RE-induced androgen release lead to upregulation of anabolic signaling pathways which likely augment net protein accretion and hypertrophy. However, the anabolic effects of RE-induced GH release act indirectly via stimulation of hepatic-IGF-1 production; in turn resulting in the activation of anabolic signaling pathways, and muscle growth and maintenance.
Lower levels of these anabolic hormones in older adults induces anabolic resistance during RE which may partially explain their low sensitivity to a given anabolic stimulus. Hormonal patterns are obviously physiologically distinct in females and males, complicating true clarity of the isolated effects e.g., of the sex hormones (higher testosterone levels may play an important role for the adaption to RET in men; whereas in premenopausal women, estrogen may enhance the sensitivity to anabolic stimuli). Further studies are required to isolate clear hierarchical roles of the key anabolic hormones/peptides in regulating muscle growth in adults, in particular after RE, and to elucidate sex differences and their mechanisms.
Author Contributions
NG, DW, and PA drafted the manuscript and BP, NS, KS, DW, and PA helped in literature search and edited the manuscript. All authors approved the submitted version.
Funding
This research was supported by the MRC-Versus Arthritis Center for Musculoskeletal Aging Research [grant numbers MR/R502364/1, MR/P021220/1] and the National Institute for Health Research (NIHR) Nottingham Biomedical Research Center.
Conflict of Interest
The authors declare that the research was conducted in the absence of any commercial or financial relationships that could be construed as a potential conflict of interest.
References
Adams, G., and Haddad, F. (1996). The relationships among IGF-1, DNA content, and protein accumulation during skeletal muscle hypertrophy. J. Appl. Physiol. 81, 2509–2516. doi: 10.1152/jappl.1996.81.6.2509
Ahtiainen, J. P., Pakarinen, A., Alen, M., Kraemer, W. J., and Häkkinen, K. (2003). Muscle hypertrophy, hormonal adaptations and strength development during strength training in strength-trained and untrained men. Eur. J. Appl. Physiol. 89, 555–563. doi: 10.1007/s00421-003-0833-3
Ahtiainen, J. P., Pakarinen, A., Alen, M., Kraemer, W. J., and Häkkinen, K. (2005). Short vs. long rest period between the sets in hypertrophic resistance training: influence on muscle strength, size, and hormonal adaptations in trained men. J. Strength Conditioning Res. 19, 572–582. doi: 10.1519/15604.1
Allard, J. B., and Duan, C. (2018). IGF-binding proteins: why do they exist and why are there so many? Front. Endocrinolo. 9:117. doi: 10.3389/fendo.2018.00117
Antonio, J., Wilson, J. D., and George, F. W. (1999). Effects of castration and androgen treatment on androgen-receptor levels in rat skeletal muscles. J. Appl. Physiol. 87, 2016–2019. doi: 10.1152/jappl.1999.87.6.2016
Arnarson, A., Geirsdottir, O. G., Ramel, A., Jonsson, P., and Thorsdottir, I. (2015). Insulin-like growth factor-1 and resistance exercise in community dwelling old adults. J. Nutrition Health Aging 19, 856–860. doi: 10.1007/s12603-015-0547-3
Baker, J., Liu, J.-P., Robertson, E. J., and Efstratiadis, A. (1993). Role of insulin-like growth factors in embryonic and postnatal growth. Cell 75, 73–82. doi: 10.1016/S0092-8674(05)80085-6
Bamman, M. M., Shipp, J. R., Jiang, J., Gower, B. A., Hunter, G. R., Goodman, A., et al. (2001). Mechanical load increases muscle IGF-I and androgen receptor mRNA concentrations in humans. Am. J. Physiol. Endocrinol. Metabolism 280, 383–390. doi: 10.1152/ajpendo.2001.280.3.E383
Barclay, R. D., Mackenzie, R. W., Burd, N. A., Tyler, C. J., and Tillin, N. A. (2019). The role of the IGF-1 signalling cascade in muscle protein synthesis and anabolic resistance in aging skeletal muscle. Front. Nutrition 6:146. doi: 10.3389/fnut.2019.00146
Bartke, A., and Darcy, J. (2017). GH and aging: pitfalls and new insights. Best Pract. Res. Clin. Endocrinol. Metabol. 31, 113–125. doi: 10.1016/j.beem.2017.02.005
Basualto-Alarcón, C., Jorquera, G., Altamirano, F., Jaimovich, E., and Estrada, M. (2013). Testosterone signals through mTOR and androgen receptor to induce muscle hypertrophy. Med. Sci. Sports Exercise 45, 1712–1720. doi: 10.1249/MSS.0b013e31828cf5f3
Bhasin, S., Taylor, W. E., Singh, R., Artaza, J., Sinha-Hikim, I., Jasuja, R., et al. (2003). The mechanisms of androgen effects on body composition: mesenchymal pluripotent cell as the target of androgen action. J. Gerontol. Seri. A 58, 1103–1110. doi: 10.1093/gerona/58.12.M1103
Bikle, D. D., Tahimic, C., Chang, W., Wang, Y., Philippou, A., and Barton, E. R. (2015). Role of IGF-I signaling in muscle bone interactions. Bone 80, 79–88. doi: 10.1016/j.bone.2015.04.036
Birnie, K., Ben-Shlomo, Y., Holly, J. M., Gunnell, D., Ebrahim, S., Bayer, A., et al. (2012). Associations of insulin and insulin-like growth factors with physical performance in old age in the Boyd Orr and Caerphilly studies. PLoS ONE 7:30096. doi: 10.1371/journal.pone.0030096
Bjersing, J. L., Larsson, A., Palstam, A., Ernberg, M., Bileviciute-Ljungar, I., Löfgren, M., et al. (2017). Benefits of resistance exercise in lean women with fibromyalgia: involvement of IGF-1 and leptin. BMC Musculoskeletal Disord. 18:106. doi: 10.1186/s12891-017-1477-5
Bloomer, R. J., Sforzo, G. A., and Keller, B. A. (2000). Effects of meal form and composition on plasma testosterone, cortisol, and insulin following resistance exercise. Int. J. Sport Nutrition Exercise Metabol. 10, 415–424. doi: 10.1123/ijsnem.10.4.415
Bratton, M. R., Antoon, J. W., Duong, B. N., Frigo, D. E., Tilghman, S., Collins-Burow, B. M., et al. (2012). Gαo potentiates estrogen receptor α activity via the ERK signaling pathway. J. Endocrinol. 214, 45–54. doi: 10.1530/JOE-12-0097
Brook, M. S., Wilkinson, D. J., Mitchell, W. K., Lund, J. N., Phillips, B. E., Szewczyk, N. J., et al. (2016). Synchronous deficits in cumulative muscle protein synthesis and ribosomal biogenesis underlie age-related anabolic resistance to exercise in humans. J. Physiol. 594, 7399–7417. doi: 10.1113/JP272857
Burger, H. G. (2002). Androgen production in women. Fertility Sterility 77, 3–5. doi: 10.1016/S0015-0282(02)02985-0
Chakravarti, S., Collins, W., Forecast, J., Newton, J., Oram, D., and Studd, J. (1976). Hormonal profiles after the menopause. Br. Med. J. 2, 784–787. doi: 10.1136/bmj.2.6039.784
Chidi-Ogbolu, N., and Baar, K. (2019). Effect of estrogen on musculoskeletal performance and injury risk. Front. Physiol. 9:1834. doi: 10.3389/fphys.2018.01834
Coleman, M. E., DeMayo, F., Yin, K. C., Lee, H. M., Geske, R., Montgomery, C., et al. (1995). Myogenic vector expression of insulin-like growth factor I stimulates muscle cell differentiation and myofiber hypertrophy in transgenic mice. J. Biol. Chem. 270, 12109–12116. doi: 10.1074/jbc.270.20.12109
Consitt, L. A., Saneda, A., Saxena, G., List, E. O., and Kopchick, J. J. (2017). Mice overexpressing growth hormone exhibit increased skeletal muscle myostatin and MuRF1 with attenuation of muscle mass. Skeletal Muscle 7:17. doi: 10.1186/s13395-017-0133-y
Cook, D. M. (2004). Growth hormone and estrogen: a clinician's approach. J. Pediatric Endocrinol. Metabolism 17, 1273–1276.
Copeland, J. L., Consitt, L. A., and Tremblay, M. S. (2002). Hormonal responses to endurance and resistance exercise in females aged 19–69 years. J. Gerontol. Seri. A 57, 158–165. doi: 10.1093/gerona/57.4.B158
Costoya, J. A., Finidori, J. E., Moutoussamy, S., Señaris, R., Devesa, J., et al. (1999). Activation of growth hormone receptor delivers an antiapoptotic signal: evidence for a role of Akt in this pathway. Endocrinology 140, 5937–5943. doi: 10.1210/endo.140.12.7209
Cui, J., Shen, Y., and Li, R. (2013). Estrogen synthesis and signaling pathways during aging: from periphery to brain. Trends Mol. Med. 19, 197–209. doi: 10.1016/j.molmed.2012.12.007
Daughaday, W. H. (2000). Growth hormone axis overview–somatomedin hypothesis. Pediatr. Nephrol. 14, 537–540. doi: 10.1007/s004670000334
Doessing, S., Heinemeier, K. M., Holm, L., Mackey, A. L., Schjerling, P., Rennie, M., et al. (2010). Growth hormone stimulates the collagen synthesis in human tendon and skeletal muscle without affecting myofibrillar protein synthesis. J. Physiol. 588, 341–351. doi: 10.1113/jphysiol.2009.179325
Dubois, V., Laurent, M. R., Sinnesael, M., Cielen, N., Helsen, C., Clinckemalie, L., et al. (2014). A satellite cell-specific knockout of the androgen receptor reveals myostatin as a direct androgen target in skeletal muscle. FASEB J. 28, 2979–2994. doi: 10.1096/fj.14-249748
Dufau, M. L., and Catt, K. J. (1979). Gonadotropin receptors and regulation of steroidogenesis in the testis and ovary. Vitamins & Hormones. 36, 461–592. doi: 10.1016/S0083-6729(08)60989-9
Eliakim, A., Brasel, J. A., Mohan, S., Wong, W. L. T., and Cooper, D. M. (1998). Increased physical activity and the growth hormone-IGF-I axis in adolescent males. Am. J. Physiol. Regulatory Integrative Comparative Physiol. 275, 308–314. doi: 10.1152/ajpregu.1998.275.1.R308
Enns, D. L., and Tiidus, P. M. (2010). The influence of estrogen on skeletal muscle. Sports Med. 40, 41–58. doi: 10.2165/11319760-000000000-00000
Estrada, M., Espinosa, A., Müller, M., and Jaimovich, E. (2003). Testosterone stimulates intracellular calcium release and mitogen-activated protein kinases via a G protein-coupled receptor in skeletal muscle cells. Endocrinology 144, 3586–3597. doi: 10.1210/en.2002-0164
Feng, W., Webb, P., Nguyen, P., Liu, X., Li, J., Karin, M., et al. (2001). Potentiation of estrogen receptor activation function 1 (AF-1) by Src/JNK through a serine 118-independent pathway. Mol. Endocrinol. 15, 32–45. doi: 10.1210/mend.15.1.0590
Ferrando, A. A., Sheffield-Moore, M., Yeckel, C. W., Gilkison, C., Jiang, J., Achacosa, A., et al. (2002). Testosterone administration to older men improves muscle function: molecular and physiological mechanisms. Am. J. Physiol. Endocrinol. Metabol. 282, 601–607. doi: 10.1152/ajpendo.00362.2001
Fink, J., Kikuchi, N., and Nakazato, K. (2018a). Effects of rest intervals and training loads on metabolic stress and muscle hypertrophy. Clin. Physiol. Funct. Imaging 38, 261–268. doi: 10.1111/cpf.12409
Fink, J., Schoenfeld, B. J., and Nakazato, K. (2018b). The role of hormones in muscle hypertrophy. Physician Sportsmed. 46, 129–134. doi: 10.1080/00913847.2018.1406778
Fink, J. E., Schoenfeld, B. J., Kikuchi, N., and Nakazato, K. (2017). Acute and long-term responses to different rest intervals in low-load resistance training. Int. J. Sports Med. 38, 118–124. doi: 10.1055/s-0042-119204
Fry, A., and Lohnes, C. (2010). Acute testosterone and cortisol responses to high power resistance exercise. Human Physiol. 36, 457–461. doi: 10.1134/S0362119710040110
Fry, A. C., and Kraemer, W. J. (1997). Resistance exercise overtraining and overreaching. Sports Med. 23, 106–129. doi: 10.2165/00007256-199723020-00004
Fryburg, D. A., and Barrett, E. J. (1993). Growth hormone acutely stimulates skeletal muscle but not whole-body protein synthesis in humans. Metabolism 42, 1223–1227. doi: 10.1016/0026-0495(93)90285-V
Fryburg, D. A., Gelfand, R. A., and Barrett, E. J. (1991). Growth hormone acutely stimulates forearm muscle protein synthesis in normal humans. Am. J. Physiol. Endocrinol. Metabol. 260, E499–E504. doi: 10.1152/ajpendo.1991.260.3.E499
Fryburg, D. A., Louard, R. J., Gerow, K. E., Gelfand, R. A., and Barrett, E. J. (1992). Growth hormone stimulates skeletal muscle protein synthesis and antagonizes insulin's antiproteolytic action in humans. Diabetes 41, 424–429. doi: 10.2337/diab.41.4.424
Gharahdaghi, N., Rudrappa, S., Brook, M. S., Idris, I., Crossland, H., Hamrock, C., et al. (2019). Testosterone therapy induces molecular programming augmenting physiological adaptations to resistance exercise in older men. J. Cachexia Sarcopenia Muscle 10, 1276–1294. doi: 10.1002/jcsm.12472
Gibney, J., Healy, M.-L., and Sönksen, P. H. (2007). The growth hormone/insulin-like growth factor-I axis in exercise and sport. Endocrine Rev. 28, 603–624. doi: 10.1210/er.2006-0052
Gil, M. C. R., Timón, R., Toribio, A., Muñoz, D., Maynar, J., Caballero, M., et al. (2012). Effects of aerobic exercise on urinary estrogens and progestagens in pre and postmenopausal women. Eur. J. Appl. Physiol. 112, 357–364. doi: 10.1007/s00421-011-1982-4
Giustina, A., Mazziotti, G., and Canalis, E. (2008). Growth hormone, insulin-like growth factors, and the skeleton. Endocrine Rev. 29, 535–559. doi: 10.1210/er.2007-0036
Godfrey, R. J., Madgwick, Z., and Whyte, G. P. (2003). The exercise-induced growth hormone response in athletes. Sports Med. 33, 599–613. doi: 10.2165/00007256-200333080-00005
Häkkinen, K., and Pakarinen, A. (1995). Acute hormonal responses to heavy resistance exercise in men and women at different ages. Int. J. Sports Med. 16, 507–513. doi: 10.1055/s-2007-973045
Hakkinen, K., Pakarinen, A., Kraemer, W. J., Newton, R. U., and Alen, M. (2000). Basal concentrations and acute responses of serum hormones and strength development during heavy resistance training in middle-aged and elderly men and women. J. Gerontology-Biol. Sci. Med. Sci. 55, 95–105. doi: 10.1093/gerona/55.2.B95
Handelsman, D. J., Hirschberg, A. L., and Bermon, S. (2018). Circulating testosterone as the hormonal basis of sex differences in athletic performance. Endocrine Rev. 39, 803–829. doi: 10.1210/er.2018-00020
Hansen, M. (2018). Female hormones: do they influence muscle and tendon protein metabolism? Proc. Nutrition Soc. 77, 32–41. doi: 10.1017/S0029665117001951
Hansen, M., and Kjaer, M. (2014). Influence of sex and estrogen on musculotendinous protein turnover at rest and after exercise. Exercise Sport Sci. Rev. 42, 183–192. doi: 10.1249/JES.0000000000000026
Hansen, M., Skovgaard, D., Reitelseder, S., Holm, L., Langbjerg, H., and Kjaer, M. (2012). Effects of estrogen replacement and lower androgen status on skeletal muscle collagen and myofibrillar protein synthesis in postmenopausal women. J. Gerontol. Seri. A 67, 1005–1013. doi: 10.1093/gerona/gls007
Hansen, S., Kvorning, T., Kjaer, M., and Sjøgaard, G. (2001). The effect of short-term strength training on human skeletal muscle: the importance of physiologically elevated hormone levels. Scand. J. Med. Sci. Sports 11, 347–354. doi: 10.1034/j.1600-0838.2001.110606.x
Hartman, M. L., Iranmanesh, A., Thorner, M. O., and Veldhuis, J. D. (1993). Evaluation of pulsatile patterns of growth hormone release in humans: a brief review. Am. J. Human Biol. 5, 603–614. doi: 10.1002/ajhb.1310050603
Hermansen, K., Bengtsen, M., Kjær, M., Vestergaard, P., and Jørgensen, J. O. L. (2017). Impact of GH administration on athletic performance in healthy young adults: a systematic review and meta-analysis of placebo-controlled trials. Growth Hormone IGF Res. 34, 38–44. doi: 10.1016/j.ghir.2017.05.005
Hoffman, J. R., Im, J., Rundell, K. W., Kang, J., Nioka, S., Speiring, B. A., et al. (2003). Effect of muscle oxygenation during resistance exercise on anabolic hormone response. Med. Sci. Sports Exercise 35, 1929–1934. doi: 10.1249/01.MSS.0000093613.30362.DF
Hoffman, J. R., Kraemer, W. J., Bhasin, S., Storer, T., Ratamess, N. A., Haff, G. G., et al. (2009). Position stand on androgen and human growth hormone use. J. Strength Conditioning Res. 23, 1–59. doi: 10.1519/JSC.0b013e31819df2e6
Hooper, D. R., Kraemer, W. J., Focht, B. C., Volek, J. S., DuPont, W. H., Caldwell, L. K., et al. (2017). Endocrinological roles for testosterone in resistance exercise responses and adaptations. Sports Med. 47, 1709–1720. doi: 10.1007/s40279-017-0698-y
Hough, J. P., Papacosta, E., Wraith, E., and Gleeson, M. (2011). Plasma and salivary steroid hormone responses of men to high-intensity cycling and resistance exercise. J. Strength Conditioning Res. 25, 23–31. doi: 10.1519/JSC.0b013e3181fef8e7
Hymer, W. C., Kraemer, W. J., Nindl, B. C., Marx, J. O., Benson, D. E., Welsch, J. R., et al. (2001). Characteristics of circulating growth hormone in women after acute heavy resistance exercise. Am. J. Physiol. Endocrinol. Metabol. 281, 878–887. doi: 10.1152/ajpendo.2001.281.4.E878
Inoue, K., Yamasaki, S., Fushiki, T., Okada, Y., and Sugimoto, E. (1994). Androgen receptor antagonist suppresses exercise-induced hypertrophy of skeletal muscle. Eur. J. Appl. Physiol. Occupational Physiol. 69, 88–91. doi: 10.1007/BF00867933
Jørgensen, J. O., Jessen, N., Pedersen, S. B., Vestergaard, E., Gormsen, L., Lund, S. A., et al. (2006). GH receptor signaling in skeletal muscle and adipose tissue in human subjects following exposure to an intravenous GH bolus. Am. J. Physiol. Endocrinol. Metabol. 291, 899–905. doi: 10.1152/ajpendo.00024.2006
Kadi, F. (2008). Cellular and molecular mechanisms responsible for the action of testosterone on human skeletal muscle. A basis for illegal performance enhancement. Br. J. Pharmacol. 154, 522–528. doi: 10.1038/bjp.2008.118
Kido, K., Ato, S., Yokokawa, T., Makanae, Y., Sato, K., and Fujita, S. (2016). Acute resistance exercise-induced IGF 1 expression and subsequent GLUT 4 translocation. Physiol. Rep. 4:e12907. doi: 10.14814/phy2.12907
Kitajima, Y., and Ono, Y. (2016). Estrogens maintain skeletal muscle and satellite cell functions. J. Endocrinol. 229, 267–275. doi: 10.1530/JOE-15-0476
Kraemer, W. J., Fleck, S. J., Dziados, J. E., Harman, E. A., Marchitelli, L. J., Gordon, S., et al. (1993). Changes in hormonal concentrations after different heavy-resistance exercise protocols in women. J. Appl. Physiol. 75, 594–604. doi: 10.1152/jappl.1993.75.2.594
Kraemer, W. J., Häkkinen, K., Newton, R. U., McCormick, M., Nindl, B. C., Volek, J. S., et al. (1998). Acute hormonal responses to heavy resistance exercise in younger and older men. Eur. J. Appl. Physiol. Occupat. Physiol. 77, 206–211. doi: 10.1007/s004210050323
Kraemer, W. J., Häkkinen, K., Newton, R. U., Nindl, B. C., Volek, J. S., McCormick, M., et al. (1999). Effects of heavy-resistance training on hormonal response patterns in younger vs. older men. J. Appl. Physiol. 87, 982–992. doi: 10.1152/jappl.1999.87.3.982
Kraemer, W. J., Marchitelli, L., Gordon, S. E., Harman, E., Dziados, J. E., Mello, R., et al. (1990). Hormonal and growth factor responses to heavy resistance exercise protocols. J. Appl. Physiol. 69, 1442–1450. doi: 10.1152/jappl.1990.69.4.1442
Kraemer, W. J., and Ratamess, N. A. (2005). Hormonal responses and adaptations to resistance exercise and training. Sports Med. 35, 339–361. doi: 10.2165/00007256-200535040-00004
Kraemer, W. J., Ratamess, N. A., Hymer, W. C., Nindl, B. C., and Fragala, M. S. (2020). Growth Hormone (s), testosterone, insulin-like growth factors, and cortisol: roles and integration for cellular development and growth with exercise. Front. Endocrinol. 11:33. doi: 10.3389/fendo.2020.00033
Kraemer, W. J., Ratamess, N. A., and Nindl, B. C. (2017). Recovery responses of testosterone, growth hormone, and IGF-1 after resistance exercise. J. Appl. Physiol. 122, 549–558. doi: 10.1152/japplphysiol.00599.2016
Kraemer, W. J., Spiering, B. A., Volek, J. S., Ratamess, N. A., Sharman, M. J., Rubin, M. R., et al. (2006). Androgenic responses to resistance exercise: effects of feeding and L-carnitine. Med. Sci. Sports Exercise 38, 1288–1296. doi: 10.1249/01.mss.0000227314.85728.35
Kragstrup, T. W., Kjaer, M., and Mackey, A. (2011). Structural, biochemical, cellular, and functional changes in skeletal muscle extracellular matrix with aging. Scand. J. Med. Sci. Sports 21, 749–757. doi: 10.1111/j.1600-0838.2011.01377.x
Kvorning, T., Andersen, M., Brixen, K., Schjerling, P., Suetta, C., and Madsen, K. (2007). Suppression of testosterone does not blunt mRNA expression of myoD, myogenin, IGF, myostatin or androgen receptor post strength training in humans. J. Physiol. 578, 579–593. doi: 10.1113/jphysiol.2006.122671
Lee, S., Campomanes, C., Sikat, P., Greenfield, A., Allen, P., and McEwen, B. (2004). Estrogen induces phosphorylation of cyclic AMP response element binding (pCREB) in primary hippocampal cells in a time-dependent manner. Neuroscience 124, 549–560. doi: 10.1016/j.neuroscience.2003.11.035
Lee, W. J., Thompson, R. W., McClung, J. M., and Carson, J. A. (2003). Regulation of androgen receptor expression at the onset of functional overload in rat plantaris muscle. Am. J. Physiol. Regulat. Integrat. Comparat. Physiol. 285, 1076–1085. doi: 10.1152/ajpregu.00202.2003
Leung, K.-C., Johannsson, G., Leong, G. M., and Ho, K. K. (2004). Estrogen regulation of growth hormone action. Endocrine Rev. 25, 693–721. doi: 10.1210/er.2003-0035
Lin, H., Wang, S. W., Wang, R. Y., and Wang, P. S. (2001). Stimulatory effect of lactate on testosterone production by rat Leydig cells. J. Cell. Biochem. 83, 147–154. doi: 10.1002/jcb.1213
Linnamo, V., Pakarinen, A., Komi, P. V., Kraemer, W. J., and Häkkinen, K. (2005). Acute hormonal responses to submaximal and maximal heavy resistance and explosive exercises in men and women. J. Strength Conditioning Res. 19, 566–571. doi: 10.1519/R-15404.1
Liu, H., Bravata, D. M., Olkin, I., Friedlander, A., Liu, V., Roberts, B., et al. (2008). Systematic review: the effects of growth hormone on athletic performance. Annals Int. Med. 148, 747–758. doi: 10.7326/0003-4819-148-10-200805200-00215
Liu, P. Y., Beilin, J., Meier, C., Nguyen, T. V., Center, J. R., Leedman, P. J., et al. (2007). Age-related changes in serum testosterone and sex hormone binding globulin in Australian men: longitudinal analyses of two geographically separate regional cohorts. J. Clin. Endocrinol. Metabol. 92, 3599–3603. doi: 10.1210/jc.2007-0862
Luk, H. Y., Kraemer, W. J., Szivak, T. K., Flanagan, S. D., Hooper, D. R., Kupchak, B. R., et al. (2015). Acute resistance exercise stimulates sex-specific dimeric immunoreactive growth hormone responses. Growth Hormone IGF Res. 25, 136–140. doi: 10.1016/j.ghir.2015.02.002
Ly, L. P., Jimenez, M., Zhuang, T. N., Celermajer, D. S., Conway, A. J., and Handelsman, D. J. (2001). A double-blind, placebo-controlled, randomized clinical trial of transdermal dihydrotestosterone gel on muscular strength, mobility, and quality of life in older men with partial androgen deficiency. J. Clin. Endocrinol. Metabol. 86, 4078–4088. doi: 10.1210/jcem.86.9.7821
MacLean, H. E., Chiu, W. M., Notini, A. J., Axell, A.-M., Davey, R. A., McManus, J. F., et al. (2008). Impaired skeletal muscle development and function in male, but not female, genomic androgen receptor knockout mice. FASEB J. 22, 2676–2689. doi: 10.1096/fj.08-105726
Maggio, M., Lauretani, F., Basaria, S., Ceda, G., Bandinelli, S., Metter, E., et al. (2008). Sex hormone binding globulin levels across the adult lifespan in women—the role of body mass index and fasting insulin. J. Endocrinol. Invest. 31, 597–601. doi: 10.1007/BF03345608
Mainardi, M., Fusco, S., and Grassi, C. (2015). Modulation of hippocampal neural plasticity by glucose-related signaling. Neural plasticity 2015:657928. doi: 10.1155/2015/657928
Mangan, G., Bombardier, E., Mitchell, A., Quadrilatero, J., and Tiidus, P. (2014). Oestrogen-dependent satellite cell activation and proliferation following a running exercise occurs via the PI 3K signalling pathway and not IGF-1. Acta Physiol. 212, 75–85. doi: 10.1111/apha.12317
Marx, J. O., Ratamess, N. A., Nindl, B. C., Gotshalk, L. A., Volek, J. S., Dohi, K., et al. (2001). Low-volume circuit versus high-volume periodized resistance training in women. Med. Sci. Sports Exercise 33, 635–643. doi: 10.1097/00005768-200104000-00019
Matsumoto, A. M. (2002). Andropause: clinical implications of the decline in serum testosterone levels with aging in men. J. Gerontol. Seri. A 57, 76–99. doi: 10.1093/gerona/57.2.M76
Mauras, N., Rini, A., Welch, S., Sager, B., and Murphy, S. P. (2003). Synergistic effects of testosterone and growth hormone on protein metabolism and body composition in prepubertal boys. Metabolism 52, 964–969. doi: 10.1016/S0026-0495(03)00163-X
McCall, G. E., Byrnes, W. C., Fleck, S. J., Dickinson, A., and Kraemer, W. J. (1999). Acute and chronic hormonal responses to resistance training designed to promote muscle hypertrophy. Can. J. Appl. Physiol. 24, 96–107. doi: 10.1139/h99-009
McCaulley, G. O., McBride, J. M., Cormie, P., Hudson, M. B., Nuzzo, J. L., Quindry, J. C., et al. (2009). Acute hormonal and neuromuscular responses to hypertrophy, strength and power type resistance exercise. Eur. J. Appl. Physiol. 105, 695–704. doi: 10.1007/s00421-008-0951-z
Menon, S., Dibble, C. C., Talbott, G., Hoxhaj, G., Valvezan, A. J., Takahashi, H., et al. (2014). Spatial control of the TSC complex integrates insulin and nutrient regulation of mTORC1 at the lysosome. Cell 156, 771–785. doi: 10.1016/j.cell.2013.11.049
Migiano, M. J., Vingren, J. L., Volek, J. S., Maresh, C. M., Fragala, M. S., Ho, J.-Y., et al. (2010). Endocrine response patterns to acute unilateral and bilateral resistance exercise in men. J. Strength Conditioning Res. 24, 128–134. doi: 10.1519/JSC.0b013e3181a92dc5
Miller, B. F., Hansen, M., Olesen, J. L., Flyvbjerg, A., Schwarz, P., Babraj, J. A., et al. (2006). No effect of menstrual cycle on myofibrillar and connective tissue protein synthesis in contracting skeletal muscle. Am. J. Physiol. Endocrinol. Metabol. 290, 163–168. doi: 10.1152/ajpendo.00300.2005
Mitchell, C. J., Churchward-Venne, T. A., Bellamy, L., Parise, G., Baker, S. K., and Phillips, S. M. (2013). Muscular and systemic correlates of resistance training-induced muscle hypertrophy. PLoS ONE 8:78636. doi: 10.1371/journal.pone.0078636
Mohamad, N.-,v., Soelaiman, I.-N., and Chin, K.-Y. (2016). A concise review of testosterone and bone health. Clin. Interventions In Aging 11, 1317–1324. doi: 10.2147/CIA.S115472
Mora, G. R., and Mahesh, V. B. (1999). Autoregulation of the androgen receptor at the translational level:: Testosterone induces accumulation of androgen receptor mrna in the rat ventral prostate polyribosomes. Steroids 64, 587–591. doi: 10.1016/S0039-128X(99)00037-9
Morton, R. W., Oikawa, S. Y., Wavell, C. G., Mazara, N., McGlory, C., Quadrilatero, J., et al. (2016). Neither load nor systemic hormones determine resistance training-mediated hypertrophy or strength gains in resistance-trained young men. J. Appl. Physiol. 121, 129–138. doi: 10.1152/japplphysiol.00154.2016
Morton, R. W., Sato, K., Gallaugher, M. P., Oikawa, S. Y., McNicholas, P. D., Fujita, S., et al. (2018). Muscle androgen receptor content but not systemic hormones is associated with resistance training-induced skeletal muscle hypertrophy in healthy, young men. Front. Physiol. 9:1373. doi: 10.3389/fphys.2018.01373
Murphy, C., and Koehler, K. (2020). Caloric restriction induces anabolic resistance to resistance exercise. European J. Appl. Physiol. 120:1155–64. doi: 10.1007/s00421-020-04354-0
Nakamura, Y., and Aizawa, K. (2017). “Sex hormones, menstrual cycle and resistance exercise,” in Sex Hormones, Exercise and Women, eds A. C. Hackney (Cham: Springer), 243–256. doi: 10.1007/978-3-319-44558-8_14
Nakamura, Y., Aizawa, K., Imai, T., Kono, I., and Mesaki, N. (2011). Hormonal responses to resistance exercise during different menstrual cycle states. Med. Sci. Sports Exercise 43, 967–973. doi: 10.1249/MSS.0b013e3182019774
Nelson, L. R., and Bulun, S. E. (2001). Estrogen production and action. J. Am. Acad. Dermatol. 45, S116–S124. doi: 10.1067/mjd.2001.117432
Nicholls, A. R., and Holt, R. I. (2016). “Growth hormone and insulin-like growth factor-1,” in Sports Endocrinology, eds F. Lanfranco, and C.J. Strasburger (Basel: Karger Publishers), p. 101–114. doi: 10.1159/000445173
Nindl, B. C., Kraemer, W. J., Gotshalk, L. A., Marx, J. O., Volek, J. S., Bush, J. A., et al. (2001). Testosterone responses after resistance exercise in women: influence of regional fat distribution. Int. J. Sport Nutrition Exercise Metabol. 11, 451–465. doi: 10.1123/ijsnem.11.4.451
Ogasawara, R., Sato, K., Higashida, K., Nakazato, K., and Fujita, S. (2013). Ursolic acid stimulates mTORC1 signaling after resistance exercise in rat skeletal muscle. Am. J. Physiol. Endocrinol. Metabol. 305, 760–765. doi: 10.1152/ajpendo.00302.2013
Ohlsson, C., Mohan, S., Sjogren, K., Tivesten, A., Isgaard, J., Isaksson, O., et al. (2009). The role of liver-derived insulin-like growth factor-I. Endocrine Rev. 30, 494–535. doi: 10.1210/er.2009-0010
Ohlsson, C., Sjögren, K., Jansson, J.-O., and Isaksson, O. (2000). The relative importance of endocrine versus autocrine/paracrine insulin-like growth factor-I in the regulation of body growth. Pediatr. Nephrol. 14, 541–543. doi: 10.1007/s004670000348
O'Neill, B. T., Lauritzen, H. P., Hirshman, M. F., Smyth, G., Goodyear, L. J., and Kahn, C. R. (2015). Differential role of insulin/IGF-1 receptor signaling in muscle growth and glucose homeostasis. Cell Rep. 11, 1220–1235. doi: 10.1016/j.celrep.2015.04.037
Paroo, Z., Dipchand, E. S., and Noble, E. G. (2002). Estrogen attenuates postexercise HSP70 expression in skeletal muscle. Am. J. Physiol. Cell Physiol. 282, 245–251. doi: 10.1152/ajpcell.00336.2001
Paroo, Z., Tiidus, P. M., and Noble, E. G. (1999). Estrogen attenuates HSP 72 expression in acutely exercised male rodents. Eur. J. Appl. Physiol. Occupat. Physiol. 80, 180–184. doi: 10.1007/s004210050579
Pinedo-Villanueva, R., Westbury, L. D., Syddall, H. E., Sanchez-Santos, M. T., Dennison, E. M., Robinson, S. M., et al. (2019). Health care costs associated with muscle weakness: a UK population-based estimate. Calcified Tissue Int. 104, 137–144. doi: 10.1007/s00223-018-0478-1
Ratamess, N. A., Kraemer, W. J., Volek, J. S., Maresh, C. M., VanHeest, J. L., Sharman, M. J., et al. (2005). Androgen receptor content following heavy resistance exercise in men. J. Steroid Biochem. Mol. Biol. 93, 35–42. doi: 10.1016/j.jsbmb.2004.10.019
Roberts, M. D., Haun, C. T., Mobley, C. B., Mumford, P. W., Romero, M. A., Roberson, P. A., et al. (2018). Physiological differences between low versus high skeletal muscle hypertrophic responders to resistance exercise training: current perspectives and future research directions. Front. Physiol. 9:834. doi: 10.3389/fphys.2018.00834
Romagnoli, C., Zonefrati, R., Sharma, P., Innocenti, M., Cianferotti, L., and Brandi, M. L. (2020). Characterization of skeletal muscle endocrine control in an in vitro model of myogenesis. Calcified Tissue Int. 107, 18–30. doi: 10.1007/s00223-020-00678-3
Rozario, T., and DeSimone, D. W. (2010). The extracellular matrix in development and morphogenesis: a dynamic view. Dev. Biol. 341, 126–140. doi: 10.1016/j.ydbio.2009.10.026
Sakamaki-Sunaga, M., Min, S., Kamemoto, K., and Okamoto, T. (2016). Effects of menstrual phase–dependent resistance training frequency on muscular hypertrophy and strength. J. Strength Conditioning Res. 30, 1727–1734. doi: 10.1519/JSC.0000000000001250
Sambasivan, R., Yao, R., Kissenpfennig, A., Van Wittenberghe, L., Paldi, A., Gayraud-Morel, B., et al. (2011). Pax7-expressing satellite cells are indispensable for adult skeletal muscle regeneration. Development 138, 3647–3656. doi: 10.1242/dev.067587
Sandri, M., Barberi, L., Bijlsma, A., Blaauw, B., Dyar, K., Milan, G., et al. (2013). Signalling pathways regulating muscle mass in aging skeletal muscle. The role of the IGF1-Akt-mTOR-FoxO pathway. Biogerontology 14, 303–323. doi: 10.1007/s10522-013-9432-9
Schiaffino, S., Dyar, K. A., Ciciliot, S., Blaauw, B., and Sandri, M. (2013). Mechanisms regulating skeletal muscle growth and atrophy. FEBS J. 280, 4294–4314. doi: 10.1111/febs.12253
Schiaffino, S., and Mammucari, C. (2011). Regulation of skeletal muscle growth by the IGF1-Akt/PKB pathway: insights from genetic models. Skeletal muscle 1:4. doi: 10.1186/2044-5040-1-4
Schroeder, E. T., Villanueva, M., West, D. D., and Phillips, S. M. (2013). Are acute post–resistance exercise increases in testosterone, growth hormone, and IGF-1 necessary to stimulate skeletal muscle anabolism and hypertrophy? Med. Sci. Sports Exercise 45, 2044–2051. doi: 10.1249/MSS.0000000000000147
Schwarz, A. J., Brasel, J., Hintz, R. L., Mohan, S., and Cooper, D. (1996). Acute effect of brief low-and high-intensity exercise on circulating insulin-like growth factor (IGF) I, II, and IGF-binding protein-3 and its proteolysis in young healthy men. J. Clin. Endocrinol. Metabol. 81, 3492–3497. doi: 10.1210/jcem.81.10.8855791
Senf, S. M., Howard, T. M., Ahn, B., Ferreira, L. F., and Judge, A. R. (2013). Loss of the inducible Hsp70 delays the inflammatory response to skeletal muscle injury and severely impairs muscle regeneration. PLoS ONE 8:e62687. doi: 10.1371/journal.pone.0062687
Sepulveda, P. V., Bush, E. D., and Baar, K. (2015). Pharmacology of manipulating lean body mass. Clin. Exp. Pharmacol. Physiol. 42, 1–13. doi: 10.1111/1440-1681.12320
Sheffield-Moore, M. (2000). Androgens and the control of skeletal muscle protein synthesis. Annals Med. 32, 181–186. doi: 10.3109/07853890008998825
Sinha-Hikim, I., Cornford, M., Gaytan, H., Lee, M. L., and Bhasin, S. (2006). Effects of testosterone supplementation on skeletal muscle fiber hypertrophy and satellite cells in community-dwelling older men. J. Clin. Endocrinol. Metabol. 91, 3024–3033. doi: 10.1210/jc.2006-0357
Sitnick, M., Foley, A. M., Brown, M., and Spangenburg, E. E. (2006). Ovariectomy prevents the recovery of atrophied gastrocnemius skeletal muscle mass. J. Appl. Physiol. 100, 286–293. doi: 10.1152/japplphysiol.00869.2005
Smith, G. I., Yoshino, J., Reeds, D. N., Bradley, D., Burrows, R. E., Heisey, H. D., et al. (2014). Testosterone and progesterone, but not estradiol, stimulate muscle protein synthesis in postmenopausal women. J. Clin. Endocrinol. Metabol. 99, 256–265. doi: 10.1210/jc.2013-2835
Sotiropoulos, A., Ohanna, M., Kedzia, C., Menon, R. K., Kopchick, J. J., Kelly, P. A., et al. (2006). Growth hormone promotes skeletal muscle cell fusion independent of insulin-like growth factor 1 up-regulation. Proc. Natl. Acad. Sci. U.S.A. 103, 7315–7320. doi: 10.1073/pnas.0510033103
Spiering, B., Kraemer, W., Anderson, J., Armstrong, L., Nindl, B., Volek, J., et al. (2008). Manipulation of resistance exercise Programme variables determines the responses of cellular and molecular Signalling pathways. Sports Med. 38, 527–540. doi: 10.2165/00007256-200838070-00001
Spiering, B. A., Kraemer, W. J., Vingren, J. L., Ratamess, N. A., Anderson, J. M., Armstrong, L. E., et al. (2009). Elevated endogenous testosterone concentrations potentiate muscle androgen receptor responses to resistance exercise. J. Steroid Biochem. Mol. Biol. 114, 195–199. doi: 10.1016/j.jsbmb.2009.02.005
Sung, E., Han, A., Hinrichs, T., Vorgerd, M., Manchado, C., and Platen, P. (2014). Effects of follicular versus luteal phase-based strength training in young women. Springerplus 3:668. doi: 10.1186/2193-1801-3-668
Syms, A., Norris, J., Panko, W., and Smith, R. (1985). Mechanism of androgen-receptor augmentation. Analysis of receptor synthesis and degradation by the density-shift technique. J. Biol. Chem. 260, 455–461.
Tahimic, C. G., Wang, Y., and Bikle, D. D. (2013). Anabolic effects of IGF-1 signaling on the skeleton. Front. Endocrinol. 4:6. doi: 10.3389/fendo.2013.00006
Thomas, A., Bunyan, K., and Tiidus, P. (2010). Oestrogen receptor-alpha activation augments post-exercise myoblast proliferation. Acta Physiol. 198, 81–89. doi: 10.1111/j.1748-1716.2009.02033.x
Tinline-Goodfellow, C. T., West, D. W., Malowany, J. M., Gillen, J. B., and Moore, D. R. (2020). An acute reduction in habitual protein intake attenuates post exercise anabolism and may bias oxidation-derived protein requirements in resistance trained men. Front. Nutrition 7:55. doi: 10.3389/fnut.2020.00055
Trejo, J. L., Carro, E., and Torres-Aleman, I. (2001). Circulating insulin-like growth factor I mediates exercise-induced increases in the number of new neurons in the adult hippocampus. J. Neurosci. 21, 1628–1634. doi: 10.1523/JNEUROSCI.21-05-01628.2001
Tremblay, M. S., Copeland, J. L., and Van Helder, W. (2004). Effect of training status and exercise mode on endogenous steroid hormones in men. J. Appl. Physiol. 96, 531–539. doi: 10.1152/japplphysiol.00656.2003
Van Nieuwpoort, I., Vlot, M., Schaap, L., Lips, P., and Drent, M. (2018). The relationship between serum IGF-1, handgrip strength, physical performance and falls in elderly men and women. Eur. J. Endocrinol. 179, 73–84. doi: 10.1530/EJE-18-0076
Veldhuis, J. D., Anderson, S. M., Iranmanesh, A., and Bowers, C. Y. (2005). Testosterone blunts feedback inhibition of growth hormone secretion by experimentally elevated insulin-like growth factor-I concentrations. J. Clin. Endocrinol. Metabol. 90, 1613–1617. doi: 10.1210/jc.2004-1303
Veldhuis, J. D., Anderson, S. M., Patrie, J. T., and Bowers, C. Y. (2004). Estradiol supplementation in postmenopausal women doubles rebound-like release of growth hormone (GH) triggered by sequential infusion and withdrawal of somatostatin: evidence that estrogen facilitates endogenous GH-releasing hormone drive. J. Clin. Endocrinol. Metabol. 89, 121–127. doi: 10.1210/jc.2003-031291
Velloso, C. (2008). Regulation of muscle mass by growth hormone and IGF-I. Br. J. Pharmacol. 154, 557–568. doi: 10.1038/bjp.2008.153
Vingren, J. L., Kraemer, W. J., Hatfield, D. L., Anderson, J. M., Volek, J. S., Ratamess, N. A., et al. (2008). Effect of resistance exercise on muscle steroidogenesis. J. Appl. Physiol. 105, 1754–1760. doi: 10.1152/japplphysiol.91235.2008
Vingren, J. L., Kraemer, W. J., Ratamess, N. A., Anderson, J. M., Volek, J. S., and Maresh, C. M. (2010). Testosterone physiology in resistance exercise and training. Sports Med. 40, 1037–1053. doi: 10.2165/11536910-000000000-00000
Wen, Y., Alimov, A. P., and McCarthy, J. J. (2016). Ribosome biogenesis is necessary for skeletal muscle hypertrophy. Exercise Sport Sciences Rev. 44, 110–115. doi: 10.1249/JES.0000000000000082
West, D. W., Burd, N. A., Tang, J. E., Moore, D. R., Staples, A. W., Holwerda, A. M., et al. (2010). Elevations in ostensibly anabolic hormones with resistance exercise enhance neither training-induced muscle hypertrophy nor strength of the elbow flexors. J. Appl. Physiol. 108, 60–67. doi: 10.1152/japplphysiol.01147.2009
West, D. W., Kujbida, G. W., Moore, D. R., Atherton, P., Burd, N. A., Padzik, J. P., et al. (2009). Resistance exercise-induced increases in putative anabolic hormones do not enhance muscle protein synthesis or intracellular signalling in young men. J. Physiol. 587, 5239–5247. doi: 10.1113/jphysiol.2009.177220
West, D. W., and Phillips, S. M. (2012). Associations of exercise-induced hormone profiles and gains in strength and hypertrophy in a large cohort after weight training. Eur. J. Appl. Physiol. 112, 2693–2702. doi: 10.1007/s00421-011-2246-z
White, J. P., Gao, S., Puppa, M. J., Sato, S., Welle, S. L., and Carson, J. A. (2013). Testosterone regulation of Akt/mTORC1/FoxO3a signaling in skeletal muscle. Mol. Cell. Endocrinol. 365, 174–186. doi: 10.1016/j.mce.2012.10.019
Wikström-Frisén, L., Boraxbekk, C. J., and Henriksson-Larsen, K. (2017). Effects on power, strength and lean body mass of menstrual/oral contraceptive cycle based resistance training. J. Sports Med. Phys. Fitness 57, 43–52.
Wilborn, C., Taylor, L., Poole, C., Foster, C., Willoughby, D., and Kreider, R. (2010). Effects of a purported aromatase and 5 α-reductase inhibitor on hormone profiles in college-age men. Int. J. Sport Nutrition Exercise Metabol. 20, 457–465. doi: 10.1123/ijsnem.20.6.457
Willoughby, D. S., and Taylor, L. (2004). Effects of sequential bouts of resistance exercise on androgen receptor expression. Med. Sci. Sports Exercise 36, 1499–1506. doi: 10.1249/01.MSS.0000139795.83030.D1
Wolfe, R., Ferrando, A., Sheffield-Moore, M., and Urban, R. (2000). Testosterone and muscle protein metabolism. Mayo. Clinic. Proceedings. 75, 55–60. doi: 10.1016/S0025-6196(19)30644-5
Yarrow, J. F., Borsa, P. A., Borst, S. E., Sitren, H. S., Stevens, B. R., and White, L. J. (2007). Neuroendocrine responses to an acute bout of eccentric-enhanced resistance exercise. Med. Sci. Sports Exercise 39, 941–947. doi: 10.1097/mss.0b013e318043a249
Yoon, J.-R., Ha, G.-C., Ko, K.-J., and Kang, S.-J. (2018). Effects of exercise type on estrogen, tumor markers, immune function, antioxidant function, and physical fitness in postmenopausal obese women. J. Exerc. Rehabil. 14, 1032–1040. doi: 10.12965/jer.1836446.223
Yusuf, S., Joseph, P., Rangarajan, S., Islam, S., Mente, A., Hystad, P., et al. (2020). Modifiable risk factors, cardiovascular disease, and mortality in 155 722 individuals from 21 high-income, middle-income, and low-income countries (PURE): a prospective cohort study. Lancet 395, 795–808. doi: 10.1016/S0140-6736(19)32008-2
Keywords: hormone, resistance exercise, muscle growth, protein synthesis, hypertrophy
Citation: Gharahdaghi N, Phillips BE, Szewczyk NJ, Smith K, Wilkinson DJ and Atherton PJ (2021) Links Between Testosterone, Oestrogen, and the Growth Hormone/Insulin-Like Growth Factor Axis and Resistance Exercise Muscle Adaptations. Front. Physiol. 11:621226. doi: 10.3389/fphys.2020.621226
Received: 25 October 2020; Accepted: 18 December 2020;
Published: 15 January 2021.
Edited by:
James P. Fisher, The University of Auckland, New ZealandCopyright © 2021 Gharahdaghi, Phillips, Szewczyk, Smith, Wilkinson and Atherton. This is an open-access article distributed under the terms of the Creative Commons Attribution License (CC BY). The use, distribution or reproduction in other forums is permitted, provided the original author(s) and the copyright owner(s) are credited and that the original publication in this journal is cited, in accordance with accepted academic practice. No use, distribution or reproduction is permitted which does not comply with these terms.
*Correspondence: Philip J. Atherton, philip.atherton@nottingham.ac.uk; Daniel J. Wilkinson, Daniel.wilkinson@nottingham.ac.uk