- 1Farmacia Municipale 2, Azienda Sanitaria Locale (ASL) CN1, Fossano, Italy
- 2Anatomia Patologica, Hospital “Michele e Pietro Ferrero”, Verduno, Italy
- 3Department of Science, University of Basilicata, Potenza, Italy
- 4Department of Translational Medical Sciences, University of Naples Federico II, Napoli, Italy
SARS-CoV2 infection not only causes abnormal severe pneumonia but also induces other relevant pathophysiological effects on several tissues and organs. In this regard, the clinical complications observed in COVID-19 include acute coronary syndrome, pulmonary thromboembolism, myocarditis and, in the severe cases, the occurrence of disseminated intravascular coagulation. Literature on COVID-19 highlighted the central role of the Renin Angiotensin Aldosterone System in the determinism of SARS-CoV2 cellular internalization in the target tissues. Lung degeneration and respiratory distress appear to be dependent on the perturbance of physiological mechanisms, such as the uncontrolled release of pro-inflammatory cytokines, a dysregulation of the fibrinolytic coagulative cascade and the hyperactivation of immune effector cells. In this mini review, we address the physiology of Midkine, a growth factor able to bind heparin, and its pathophysiological potential role in COVID-19 determinism. Midkine increases in many inflammatory and autoimmune conditions and correlates with several dysfunctional immune-inflammatory responses that appear to show similarities with the pathophysiological elicited by SARS-CoV2. Midkine, together with its receptor, could facilitate the virus entry, fostering its accumulation and increasing its affinity with Ace2 receptor. We also focus on Netosis, a particular mechanism of pathogen clearance exerted by neutrophils, which under certain pathological condition becomes dysfunctional and can cause tissue damage. Moreover, we highlight the mechanism of autophagy that the new coronavirus could try to escape in order to replicate itself, as well as on pulmonary fibrosis induced by hypoxia and on the release of cytokines and mediators of inflammation, correlating the interplay between Midkine and SARS-CoV2.
Introduction
Severe acute respiratory syndrome coronavirus 2 (SARS-CoV2) infection not only causes abnormal severe pneumonia but also induces other relevant pathophysiological effects on several tissues and organs. In this regard, the cardiovascular complications observed in Corona Virus Disease of 2019 (COVID-19) include acute coronary syndrome, pulmonary thromboembolism, myocarditis and, in the severe cases, the occurrence of disseminated intravascular coagulation system (Vallamkondu et al., 2020; Verdecchia et al., 2020). Literature on COVID-19 highlighted the central role of the SAAR in SARS-CoV2 cellular internalization, particularly for the virus binding to angiotensin I converting enzyme 2 (ACE2) receptor expressed on the cell membrane of the tissues targeted by SARS-CoV2 (Hoffmann et al., 2020; Ingraham et al., 2020; Liu et al., 2020; Mycroft-West et al., 2020). Lung degeneration and respiratory distress appear to be dependent on the perturbance of host response mechanisms that could foster the uncontrolled release of pro-inflammatory cytokines, the dysregulation of the fibrinolytic coagulative cascade, as well as the hyper-activation of immune effector cells (Ackermann et al., 2020; Azkur et al., 2020; Becker, 2020; Stephen-Victor et al., 2020; Vallamkondu et al., 2020). Inflammation mediators, endothelial cells, neutrophils, and macrophages are responsible for the amplification of inflammation processes and concur to the cross talk between enzymatic cascades and signal pathways (Ackermann et al., 2020; Becker, 2020; Vallamkondu et al., 2020).
Midkine is a growth factor able to bind heparin and showing a physiological role in embryonic development (Kadomatsu et al., 1988). Midkine is poorly expressed in the adult organism cells, while is highly incremented in cancer cells and correlated with a less favorable prognosis in cancer patients (O’Brien et al., 1996; Maeda et al., 2007). Midkine has a crucial role in the interplay between kidney and lung (Salvati et al., 2011), is involved in inflammation (Weckbach et al., 2011), angiogenesis (Weckbach et al., 2012), tumor growth (Kadomatsu, 2005), vascular stenosis (Weckbach et al., 2011), renal (Sato et al., 2001), neurodegenerative (Kadomatsu, 2005; Takeuchi, 2014), and autoimmune diseases (Takada et al., 1997; Kadomatsu, 2005; Figure 1). It is of note that Midkine is significantly involved in inflammation determinism (Weckbach et al., 2011), is induced during inflammation process, and enhances the recruitment of inflammatory cells (Kadomatsu et al., 2013; Figure 1). Midkine is expressed in several pathological renal conditions including diabetic nephropathy (Figure 1) and can exacerbate several kidney diseases through leukocyte recruitment (Weckbach et al., 2011). Patients with rheumatoid arthritis highly expressed Midkine (Weckbach et al., 2011). Endothelial lesions caused increase expression of Midkine that has been observed in macrophages infiltrated into the injured vascular wall (Weckbach et al., 2011).
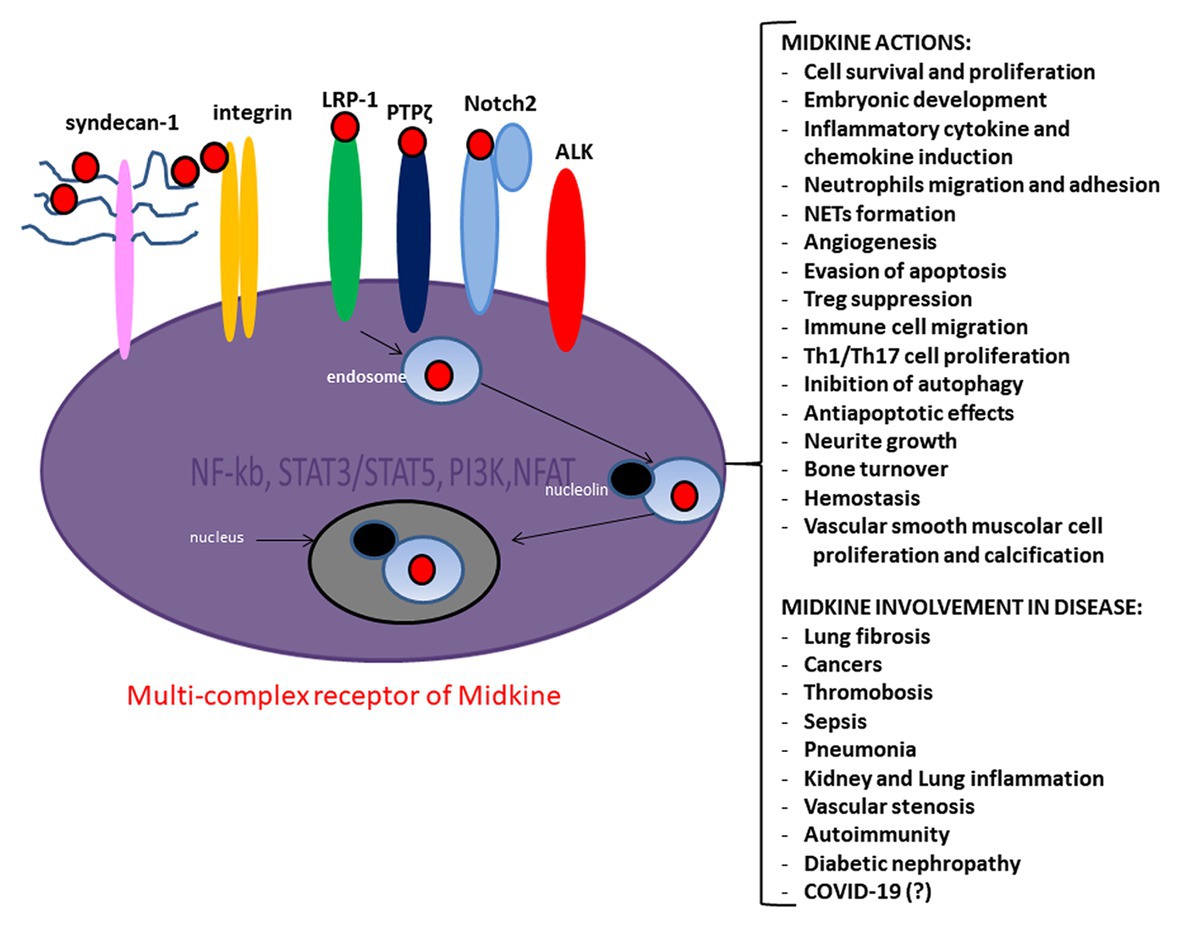
Figure 1. Midkine multi-complex receptor, actions and involvement in disease. Midkine multi-complex receptor includes Syndecan-n-1, low density lipoprotein receptor-related protein 1 (LRP-1), Neurogenic locus notch homolog protein 2 (Notch-2), integrins, protein tyrosine phosphatase zeta (PTP ζ), and Anaplastic lymphoma kinase (ALK). The signaling pathway of Midkine multi-complex receptor involves several molecules as the nuclear factor kappa-light-chain-enhancer of activated B cells (NF-kB), signal transducer and activator of transcription 3 and 5 (STAT3/STAT5), phosphatidylinositol 3-kinase (PI3K)/protein kinase B (AKT), and nuclear factor of activated T-cells (NFAT). Midkine promotes several actions ad is involved in various diseases.
Midkine can be easily detected by enzyme-linked immunosorbent assay (ELISA) in serum and urine (Ikematsu et al., 2000; Xia et al., 2016), and its tissue expression in histochemistry has been described (Kim et al., 2017).
Midkine is an important physiological mediator of Renin Angiotensin Aldosterone System (SAAR; Kadomatsu, 2010; Figure 1). SAAR regulates the migration and proliferation of smooth muscle cells and the extracellular matrix (ECM) production, the increased expression of adhesion proteins and pro-inflammatory cytokine production (Hoffmann et al., 2020; Ingraham et al., 2020; Liu et al., 2020). Plasma concentration of Midkine dramatically increased in patients with acute respiratory distress syndrome (ARDS; Zhang and Baker, 2017). Midkine appears to be overregulated upon mechanical stress in lung epithelial cells (Zhang et al., 2015; Zhang and Baker, 2017) and induces ACE2 level in the lung (Ezquerra et al., 2005; Kadomatsu, 2010). A recent study showed the interplay between Midkine and ACE2 in mechanically ventilated lung tissue (Huang S. et al., 2020). In addition, the overregulation of Midkine upon the mechanical stress was found in lung epithelial cells (Zhang et al., 2015; Zhang and Baker, 2017).
In this mini review, we focus the physiology of Midkine and its pathophysiological potential role in COVID-19, and we suggest to investigate Midkine as a putative biomarker of altered physiological conditions and/or a potential therapeutic target in the fight against pandemic COVID-19.
Midkine, Heparan Sulfate, and Extracellular Matrix: A Role For Virus Entry Facilitation?
The ECM contains proteoglycans that are very important for the structural integrity and tissue morphogenesis and homeostasis (Frantz et al., 2010). Heparan sulfate proteoglycans (HSPGs) are mainly present in the ECM and in the cell cytoplasmatic membrane and bind the Heparan sulfate (HS) chains (Lu et al., 2011). Syndecans (SDC) are HSPGs acting as regulators of cell migration, endocytosis, and cell signals (Beauvais and Rapraeger, 2003; Afratis et al., 2012; Christianson and Belting, 2014; Gallagher, 2015; Changyaleket et al., 2017). HS chains, according to their different degree of sulfation, can interfere with the growth factors/receptors interplay and promote the signal activation (Lu et al., 2011; Changyaleket et al., 2017). ADAM and ADAMTS metalloproteases and heparanase (Lu et al., 2011; Changyaleket et al., 2017) shed “soluble syndecans,” which interact with the microenvironment, where they are released (Lu et al., 2011; Changyaleket et al., 2017). Several viruses use highly sulfated proteoglycans to bind the membrane surface of target cells (Rusnati et al., 2009; Cagno et al., 2019). The negative electrostatic proteoglycans charges interact with glycoproteins basic residues on the viral surface (Rusnati et al., 2009). The SARS-CoV2 spike protein (S-protein) interact with HS (Liu et al., 2020) and the binding affinity increases if HS is added to the S-protein proteolytic cleavage site (Liu et al., 2020). The HSPGs could increase the HCov-NL63 expression and could promote virus entry (Milewska et al., 2014; Kim et al., 2020).
Scientific Literature on COVID-19 highlighted the central role of the SAAR in the mechanisms of SARS-CoV2 cellular internalization, particularly for the occurrence of virus binding to ACE2 receptor expressed on the cell membrane of the tissues targeted by SARS-CoV2 infection (Hoffmann et al., 2020; Ingraham et al., 2020; Liu et al., 2020).
Midkine is a relevant component of heparin releasable endothelial proteins (HREPs) that are bound to the endothelial surface through proteoglycans and exert several specific functions in the vascular homeostasis (Novotny et al., 1993). Midkine strongly binds the hypersulfated structures of HS (Kaneda et al., 1996). Two Cardin and Weintraub (CW) motifs form a binding site based on heparan HS at the Midkine dimerization occurrence (Gallagher, 2015). The interaction with all three Midkine sulfate groups (6-O, 2-O, and n-sulfates) is crucial for the heparin-binding (Muramatsu et al., 1994; Kaneda et al., 1996; Asai et al., 1997; Maeda et al., 1999).
Midkine expression on cell surface strongly needs HS (Gallagher, 2015) and the tri-sulfate unit of HS is the binding site for Midkine itself (Kaneda et al., 1996). Midkine role as neuronal growth factor is impaired when cells are deprived of HS and activity is suppressed by heparin saccharides, which may block the site of interaction between HS and Midkine (Gallagher, 2015). The main receptor complex of Midkine includes Syndecan-1, glycosaminoglycans (GAGs), low density lipoprotein receptor-related protein 1 (LRP-1), Notch-2, integrins, protein tyrosine phosphatase ζ (PTP ζ), and anaplastic lymphoma kinase (ALK; Maeda et al., 1999). Other potential interplay between Midkine and some other extracellular ligands that bind Syndecans and/or interact with the LRP-1, as the tissue factor pathway inhibitor (TFPI), lipoprotein lypase, and several others, could have a relevant role in fostering Midkine activity and in determining other relevant biological functions (Kojima et al., 1996; Tinholt et al., 2015).
We hypothesize that Midkine could be involved in the early stages of viral attack during COVID-19 (Figure 2). The S-protein fosters the entry of virus into cells (Hoffmann et al., 2020). The SARS CoV2 S-protein is composed by the S1 and S2 domains that are respectively correlated with the binding and fusion of virus to target cells (Hoffmann et al., 2020). The S1 expresses the receptor-binding domain (RBD) responsible for ACE2 receptor binding (He et al., 2004). S1 subunit of RBD exists in two different conformations, closed and open: the open RBD is able to bind the virus more than closed conformation (Hao et al., 2020). Enzymatic cleavage of protein S at the level of S1/S2 domains supports fusion of viruses to cell membranes via the S2 subunit (Liu et al., 2020). SARS-CoV2 S-protein interacts with both the cellular HS and ACE2 through its RBD and can simultaneously engage heparin and ACE2 (Clausen et al., 2020). Positively charged amino acids in a subdomain of RBD are responsible for the binding of heparin/HS complex via an interaction site that appears independent on the site involved in ACE2 binding (Clausen et al., 2020). SARS CoV2 protein S appears to bind HS cooperatively with ACE2 receptor on the cell surface (Clausen et al., 2020).
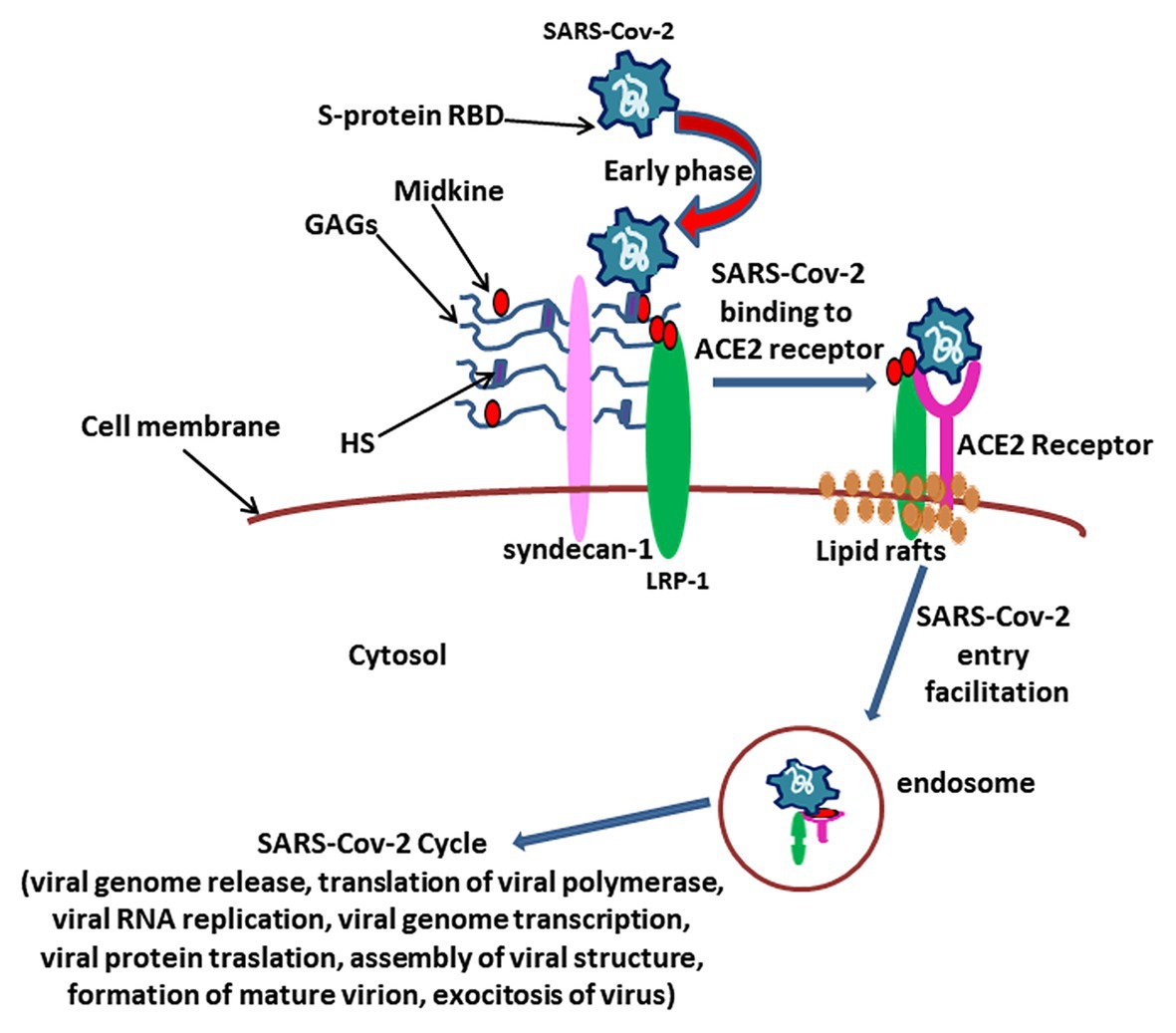
Figure 2. The hypothesis over the role for Midkine in SARS CoV2 viral attack. The complex between Midkine, Syndecan-1, glycosaminoglycans (GAGs), and Heparan sulfate (HS) could play a pivotal role in the early phase of virus attack by amplifying receptor-binding domain (RBD) sulfatation sites of Spike (S)-protein, in such way enhancing the Angiotensin I converting enzyme 2 (ACE2) receptor binding affinity and determining virus localization on the extracellular membrane. After SARS-CoV2/ACE2 receptor binding, Midkine could facilitate virus entry into the cell through LRP-1-mediated endocytosis, allowing the virus cycle as described (V’kovski et al., 2020).
SARS-CoV2 may employ several different promoting factors to infect ACE2 receptor-expressing cells in the upper respiratory tract with greater efficiency than SARS-CoV, and this occurrence may explain the greater transmissibility of SARS-CoV2 compared to SARS-CoV (Hoffmann et al., 2020).
It is reasonable to assume that Midkine could amplify RBD sulfatation sites of S-protein, increasing the binding affinity with ACE2 receptor, and that Midkine would facilitate the open conformation of S1, in such way promoting the subsequent viral attack (Clausen et al., 2020).
Midkine and Lipid Rafts
Scientific literature suggests the overall role of lipids in viral infection of target cells (Cervin and Anderson, 1991; Lajoie and Nabi, 2007; Li et al., 2007; Lu et al., 2008; Baglivo et al., 2020). Lipid rafts result in microdomains rich in cholesterol, glycosphingolipids, and phospholipids on the plasma membrane, potentially involved in the fusion, internalization, transport, and assembly of viral proteins of numerous viruses, including coronaviruses (Guo et al., 2017; Fecchi et al., 2020). Cholesterol represents the structural glue of lipid rafts (Fecchi et al., 2020). The ACE2 receptor is precisely located in the lipid rafts and is responsible for the initial phase of the viral infection of SARS-Cov2 (Fecchi et al., 2020). LRP-1 promotes endocytosis, is localized on lipid rafts, promotes the accumulation of cholesterol esters and the lipoproteins absorption (Actis Dato et al., 2020). Midkine is translocated into the nucleus by LRP-1 via nucleolin (Muramatsu et al., 2000).
In our hypothesis, the supposed interplay between the virus, Midkine, and HS and the presence of LRP-1 on lipid rafts might reveal new potential features of SARS-CoV2 infection mechanisms (Figure 2).
Midkine and Immune Regulation: A Potential Role in Covid-19?
Immunological tolerance and immune homeostasis involve regulatory T cells (Tregs; Terrazzano et al., 2020). Tolerogenic dendritic cells (DCregs) influence the inducible Tregs development (Takeuchi, 2014). mTOR (mammalian target of rapamycin) is a protein kinase, involved in apoptosis, cell cycle, metabolic disorders and autoimmunity, carcinogenesis, inflammation and autophagy, immunoregulation, and tolerance (Terrazzano et al., 2020). mTOR forms two complexes: mTORC1 induces the T helper (Th) 1 and Th17 differentiation upon viral antigen presentation by dendritic cells (DC; Omarjee et al., 2020). mTORC2 mediates Th2 differentiation (Omarjee et al., 2020), while both complexes restrict Tregs differentiation. The two mTOR complexes are involved in the regulation of Tregs homeostasis (Omarjee et al., 2020). mTOR-dependent pathways may uncover molecular targets useful for controlling the cellular damage, oxidative stress, and hyperinflammation that occur in COVID-19. Recently, mTOR inhibition therapy has been hypothesized to mitigate the cytokine storm and to reduce hyperactivation of immune responses in COVID-19 (Terrazzano et al., 2020).
COVID-19 patients who undergo ARDS are characterized by highly enhanced pro-inflammatory cytokine production (the cytokine storm) and lung repair dysfunction, which is partially due to reduced or defective Tregs involvement (Gladstone et al., 2020). Midkine suppresses the generation DCregs, which drive the development of inducible Treg (Misa et al., 2017; Figure 1), and reduces phosphorylated STAT3 levels in DCregs (Misa et al., 2017). The specific inhibition of Midkine by RNA-based aptamer increased the DCregs and Tregs and decreased the autoreactive Th1 and Th17 cells, and it has been associated with the amelioration of the clinical symptoms in experimental autoimmune encephalomyelitis model (Takeuchi, 2014).
A dysregulation in the signaling pathways of mTOR, hypoxia-inducible factor 1 (HIF-1) alpha, tumor necrosis factor (TNF) has been identified during SARS-CoV2 infection (Appelberg et al., 2020). An increased expression of Midkine in the lung appears to be mediated by HIF-1 alpha (Reynolds et al., 2004). The respiratory epithelium responds to hypoxia through Midkine dependent HIF-1 alpha regulation (Reynolds et al., 2004). Midkine expression in human polymorphonuclear neutrophils (PMNs), monocytes, and endothelium increased by hypoxia (Weckbach et al., 2019).
Anaplastic lymphoma kinase (ALK) phosphorylates the insulin receptor substrate-1 and activates mitogen-activated protein (MAP) kinase and phosphoinositide 3 (PI3)-kinase leading to transcriptional activation of nuclear factor kappa-light-chain-enhancer of activated B cells (NF-κB; Filippou et al., 2020). Filippou et al. (2020) recently reported that Midkine modulates the activity of the protein kinase B (Akt)/mTOR axis, via the ALK receptor, to prevent cell death mediated by cannabinoid-induced autophagy. Autophagy is a useful mechanism against viral infection. Autophagy plays a role in innate immunity, in the degradation of viruses or intracellular pathogens, and in the presentation of pathogens to the immune system (Fecchi et al., 2020). Viruses evolved mechanisms to escape the autophagic process (Carmona-Gutierrez et al., 2020).
SARS-CoV2, similarly to MERS-CoV, is able to reduce autophagy in infected cell lines by reducing the mTORC1-pathway, autophagy-related signaling, and the fusion between autophagosome and lysosome (Fecchi et al., 2020). SARS-CoV2 could benefit from reducing autophagy, preventing viral degradation, and improving the availability of double membrane vesicles (DMVs) needed for viral replication (Fecchi et al., 2020).
Midkine a Key Factor For Neutrophil Activation in Covid-19?
The activation of neutrophils is very relevant during COVID-19 occurrence (Leppkes et al., 2020). In the course of inflammatory diseases, neutrophils excrete chromatin, histones and the contents of their own granules in a cellular process described as neutrophil extracellular trap (NET) formation (Leppkes et al., 2020). NET has been correlated to lung disease (Leppkes et al., 2020), neutrophils from pneumonia-associated ARDS undergo NET formation (Leppkes et al., 2020), extracellular histones are elevated in ARDS (Lv et al., 2017), and NET process is described in COVID-19 (Zuo et al., 2020). Furthermore, exacerbated aggregation of NET (NETs) could alter vascular districts and damage tissues (Leppkes et al., 2020). In the vascular system, NETs determine platelet activation and thrombosis, probably due to the release of histones that can be recognized through toll-like receptors (TLRs) on platelets and immune cells (Becker, 2020).
A recent report described that NET formation increases in COVID-19 patients undergoing mechanical ventilation (Zuo et al., 2020).
Patients with severe forms of COVID-19 show a marked increase in neutrophils compared to less severe subjects (Huang C. et al., 2020).
Midkine promotes the trafficking of neutrophils in myocardium and the NET formation in myocarditis (Weckbach et al., 2019). We suggest the occurrence of an important interplay between Midkine, PMN, NETs, and COVID-19. In this regard, we hypothesize that Midkine could promote neutrophil infiltration and NET formation in the myocardium via LRP1.
Moreover, it is likely that the Midkine-dependent promotion of neutrophil activation and NETs formation strongly degenerates the complex homeostatic mechanism of coagulation and plays a relevant role in the determinism of thrombotic events correlated to neutrophil hyperactivation (Iba and Levy, 2018). In this regard, neutrophil hyperactivation and NETs formation have been associated with ARDS in influenza pneumonitis (Narasaraju et al., 2011) and with thromboinflammatory response and intravascular thrombosis during sepsis (Iba and Levy, 2018). Finally, the molecules involved in hemostasis, as procoagulant or anticoagulant, should be deeply investigated for their potential relationship with Midkine, such as thrombin and thrombomodulin that are described to interplay each other to determine different effects on hemostasis (Rezaie, 2010) and have associated with NETs occurrence (Toh et al., 2016): Midkine could alter the balance between procoagulant and anticoagulant and could foster thromboinflammatory response and intravascular thrombosis during COVID-19 occurrence.
Conclusion
Since December 2019, SARS-Cov2 infection has manifested broad pandemic connotations and several pathophysiological conditions that do not limit COVID-19 to abnormal pneumonia (Cevik et al., 2020; Chen et al., 2020). In this regard, severe phases of COVID-19 present a poor prognosis in those patients underlying clinical conditions such as hypertension, chronic obstructive pulmonary disease, diabetes, and/or cardiovascular disease (Harapan et al., 2020; Nikolich-Zugich et al., 2020). Indeed, such compromised patients incur a greater risk of rapid progression to ARDS, septic-type systemic shock, coagulation dysfunction, arrhythmia and heart failure, renal and/or heart failure, hepatic dysfunction, and the occurrence of secondary infection (Cevik et al., 2020; Chen et al., 2020; Harapan et al., 2020; Nikolich-Zugich et al., 2020).
In this mini review, we suggest the potential and intriguing scenario concerning the interaction between SARS-CoV2 and Midkine, in order to understand the pathophysiological mechanisms occurring in COVID-19.
We highlight a possible involvement of Midkine in the in SARS-CoV2 infection mechanisms. Indeed, Midkine could amplify S-protein RBD sulfatation sites, increasing the binding affinity of SARS-CoV2 with ACE2 receptor. In addition, the interplay between coronavirus, Midkine, HS, LRP-1, and lipid rafts could foster SARS-CoV2 internalization.
The main feature of the immune-mediated involvement in COVID-19 is characterized by neutrophil hyperactivation. In this regard, Midkine signaling could enhance neutrophil proliferation and migration. Several studies have showed that Midkine is involved in neutrophil infiltration and chemokine expression as well as in the Netosis occurrence (Figure 1). Moreover, a crucial interplay between Midkine, neutrophils, NET, and COVID-19 might occur. Severe COVID-19 correlates with exacerbated neutrophil hyperactivation and NET occurrence. Midkine could promote neutrophil infiltration and NET formation in the myocardium via LRP-1. In addition, Midkine could be involved in the pulmonary remodeling and fibrosis, through the collagen deposition and the Nox1, MK, Notch2, and ACE signaling pathway (Figure 1). We overviewed literature concerning Midkine-related pathway and its receptors, highlighting a common pathway with mTOR and autophagy that SARS-Cov2 could employ to elude in order to foster virus replication.
Taken in all, we hypothesize a key role of Midkine, particularly in organ dysfunction at the basis of COVID-19 pathogenesis and also propose such protein as a potential biomarker (Table 1) of pathophysiological conditions and as a key target for new potential COVID-19 therapeutical strategies by employing anti-Midkine monoclonal antibodies to be specifically prepared for clinical use in humans.
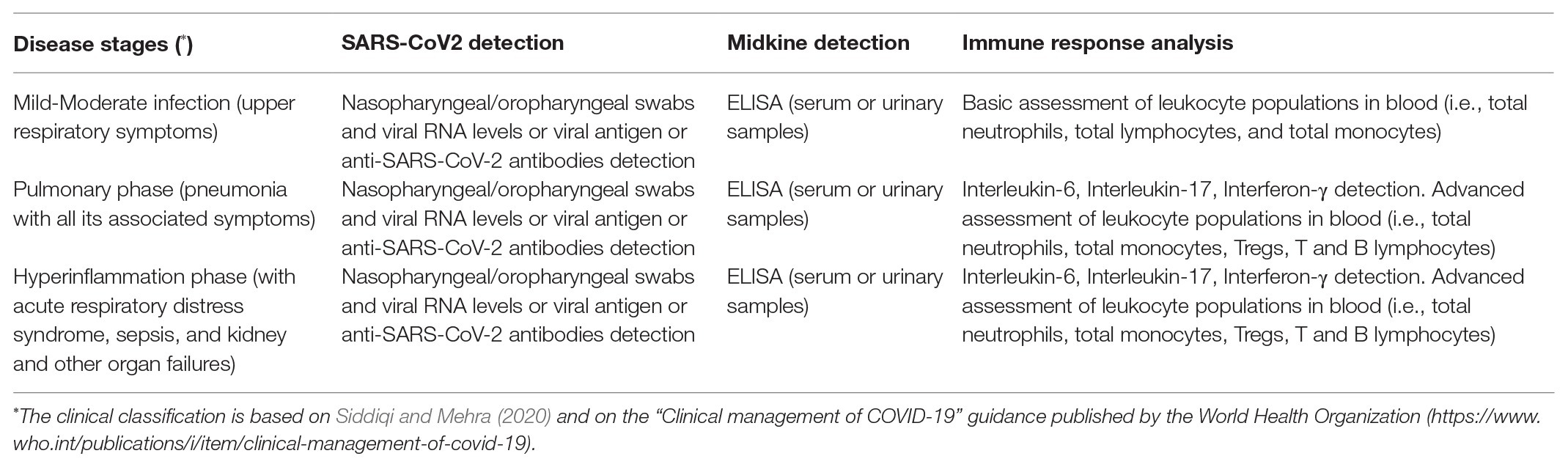
Table 1. Brief suggestions for studying the implication as a biomarker of Midkine in SARS-CoV2 infection and in COVID-19 patients.
Author Contributions
GS and GT equally contributed, conceptualized, and wrote the manuscript. MB contributed to the manuscript reading and editing. All authors contributed to the article and approved the submitted version.
Conflict of Interest
The authors declare that the research was conducted in the absence of any commercial or financial relationships that could be construed as a potential conflict of interest.
References
Ackermann, M., Verleden, S. E., Kuehnel, M., Haverich, A., Welte, T., Laenger, F., et al. (2020). Pulmonary vascular endothelialitis, thrombosis, and angiogenesis in COVID-19. N. Engl. J. Med. 383, 120–128. doi: 10.1056/NEJMoa2015432
Actis Dato, V., Benitez-Amaro, A., de Gonzalo-Calvo, D., Vazquez, M., Bonacci, G., Llorente-Cortés, V., et al. (2020). LRP1-mediated AggLDL endocytosis promotes cholesteryl ester accumulation and impairs insulin response in HL-1 cells. Cell 9:182. doi: 10.3390/cells9010182
Afratis, N., Gialeli, C., Nikitovic, D., Tsegenidis, T., Karousou, E., Theocharis, A. D., et al. (2012). Glycosaminoglycans: key players in cancer cell biology and treatment. FEBS J. 279, 1177–1197. doi: 10.1111/j.1742-4658.2012.08529.x
Appelberg, S., Gupta, S., Svensson, A. S., Ambikan, A. T., Mikaeloff, F., and Saccon, E. (2020). Dysregulation in Akt/mTOR/HIF-1 signaling identified by proteo-transcriptomics of SARS-CoV2 infected cells. Emerg. Microbes. Infect. 9, 1–36. doi: 10.1080/22221751.2020.1799723
Asai, T., Watanabe, K., Ichihara-Tanaka, K., Kaneda, N., Kojima, S., and Iguchi, A. (1997). Identification of heparin-binding sites in midkine and their role in neurite-promotion. Biochem. Biophys. Res. Commun. 236, 66–70. doi: 10.1006/bbrc.1997.6905
Azkur, A. K., Akdis, M., Azkur, D., Sokolowska, M., van de Veen, W., Brüggen, M. C., et al. (2020). Immune response to SARS-CoV2 and mechanisms of immunopathological changes in COVID-19. Allergy 75, 1564–1581. doi: 10.1111/all.14364
Baglivo, M., Baronio, M., Natalini, G., Beccari, T., Chiurazzi, P., Fulcheri, E., et al. (2020). Natural small molecules as inhibitors of coronavirus lipid-dependent attachment to host cells: a possible strategy for reducing SARS-COV2 infectivity? Acta Biomed 91, 161–164. doi: 10.23750/abm.v91i1.9402
Beauvais, D. M., and Rapraeger, A. C. (2003). Syndecan-1-mediated cell spreading requires signaling by alphavbeta3 integrins in human breast carcinoma cells. Exp. Cell Res. 286, 219–232. doi: 10.1016/s0014-4827(03)00126-5
Becker, R. C. (2020). COVID-19 update: Covid-19-associated coagulopathy. J. Thromb. Thrombolysis 50, 54–67. doi: 10.1007/s11239-020-02134-3
Cagno, V., Tseligka, E. D., Jones, S. T., and Tapparel, C. (2019). Heparan sulfate proteoglycans and viral attachment: true receptors or adaptation bias? Viruses 11:596. doi: 10.3390/v11070596
Carmona-Gutierrez, D., Bauer, M. A., Zimmermann, A., Kainz, K., Hofer, S. J., Kroemer, G., et al. (2020). Digesting the crisis: autophagy and coronaviruses. Microb. Cell 7, 119–128. doi: 10.15698/mic2020.05.715
Cervin, M., and Anderson, R. (1991). Modulation of coronavirus-mediated cell fusion by homeostatic control of cholesterol and fatty acid metabolism. J. Med.Vir. 35, 142–149. doi: 10.1002/jmv.1890350213
Cevik, M., Bamford, C., and Ho, A. (2020). COVID-19 pandemic—a focused review for clinicians. Clin. Microbiol. Infect. 26, 842–847. doi: 10.1016/j.cmi.2020.04.023
Changyaleket, B., Deliu, Z., Chignalia, A. Z., and Feinstein, D. L. (2017). Heparanase: potential roles in multiple sclerosis. J. Neuroimmunol. 310, 72–81. doi: 10.1016/j.jneuroim.2017.07.001
Chen, Y., Liu, Q., and Guo, D. (2020). Emerging coronaviruses: genome structure, replication, and pathogenesis. J. Med. Virol. 92, 418–423. doi: 10.1002/jmv.25681
Christianson, H. C., and Belting, M. (2014). Heparan sulfate proteoglycan as a cell-surface endocytosis receptor. Matrix Biol. 35, 51–55. doi: 10.1016/j.matbio.2013.10.004
Clausen, T. M., Sandoval, D. R., Spliid, C. B., Pihl, J., Painter, C. D., Thacker, B. E., et al. (2020). SARS-CoV2 infection depends on cellular heparan sulfate and ACE2. Cell 183, 1–15. doi: 10.1016/j.cell.2020.09.033
Ezquerra, L., Herradon, G., Nguyen, T., Silos-Santiago, I., and Deuel, T. F. (2005). Midkine, a newly discovered regulator of the renin-angiotensin pathway in mouse aorta: significance of the pleiotrophin/midkine developmental gene family in angiotensin II signaling. Biochem. Biophys. Res. Commun. 333, 636–643. doi: 10.1016/j.bbrc.2005.05.113
Fecchi, K., Anticoli, S., Peruzzu, D., Iessi, E., Gagliardi, M. C., Matarrese, P., et al. (2020). Coronavirus interplay with lipid rafts and autophagy unveils promising therapeutic targets. Front. Microbiol. 11:1821. doi: 10.3389/fmicb.2020.01821
Filippou, P. S., Karagiannis, G. S., and Constantinidou, A. (2020). Midkine (MDK) growth factor: a key player in cancer progression and a promising therapeutic target. Oncogene 39, 2040–2054. doi: 10.1038/s41388-019-1124-8
Frantz, C., Stewart, K. M., and Weaver, V. M. (2010). The extracellular matrix at a glance. J. Cell Sci. 123, 4195–4200. doi: 10.1242/jcs.023820
Gallagher, J. (2015). Fell-Muir Lecture: heparan sulphate and the art of cell regulation: a polymer chain conducts the protein orchestra. Int. J. Exp. Pathol. 96, 203–231. doi: 10.1111/iep.12135
Gladstone, D. E., Kim, B. S., Mooney, K., Karaba, A. H., and D'Alessio, F. R. (2020). Regulatory T cells for treating patients with COVID-19 and acute respiratory distress syndrome: two case reports. Ann. Intern. Med. 173, 852–853. doi: 10.7326/L20-0681
Guo, H., Huang, M., Yuan, Q., Wei, Y., Gao, Y., Mao, L., et al. (2017). The important role of lipid raft-mediated attachment in the infection of cultured cells by coronavirus infectious bronchitis virus beaudette strain. PLoS One 12:e0170123. doi: 10.1371/journal.pone.0170123
Hao, W., Ma, B., Li, Z., Wang, X., Gao, X., Li, Y., et al. (2020). Binding of the SARS-CoV2 spike protein to glycans. bioRxiv [Preprint]. doi: 10.1101/2020.05.17.100537
Harapan, H., Itoh, N., Yufika, A., Winardi, W., Keam, S., Te, H., et al. (2020). Coronavirus disease 2019 (COVID-19): a literature review. J. Infect. Public Health 13, 667–673. doi: 10.1016/j.jiph.2020.03.019
He, Y., Zhou, Y., Liu, S., Kou, K., Li, W., Farzan, M., et al. (2004). Receptor-binding domain of SARS-CoV spike protein induces highly potent neutralizing antibodies: implication for developing subunit vaccine. Biochem. Biophys. Res. Commun. 324, 773–781. doi: 10.1016/j.bbrc.2004.09.106
Hoffmann, M., Kleine-Weber, H., Schroeder, S., Krüger, N., Herrler, T., Erichsen, S., et al. (2020). SARS-CoV2 cell entry depends on ACE2 and TMPRSS2 and is blocked by a clinically proven protease inhibitor. Cell 181, 271–280. doi: 10.1016/j.cell.2020.02.052
Huang, S., Kaipainen, A., Strasser, M., and Baranzini, S. (2020). Mechanical ventilation stimulates expression of the SARS-CoV2 receptor ACE2 in the lung and may trigger a vicious cycle [Preprint]. doi: 10.20944/preprints202005.0429.v1
Huang, C., Wang, Y., Li, X., Ren, L., Zhao, J., Hu, Y., et al. (2020). Clinical features of patients infected with 2019 novel coronavirus in Wuhan. Lancet 395, 497–506. doi: 10.1016/S0140-6736(20)30183-5
Iba, T., and Levy, J. H. (2018). Inflammation and thrombosis: roles of neutrophils, platelets and endothelial cells and their interactions in thrombus formation during sepsis. J. Thromb. Haemost. 16, 231–241. doi: 10.1111/jth.13911
Ikematsu, S., Yano, A., Aridome, K., Kikuchi, M., Kumai, H., Nagano, H., et al. (2000). Serum midkine levels are increased in patients with various types of carcinomas. Br. J. Cancer 83, 701–706. doi: 10.1054/bjoc.2000.1339
Ingraham, N. E., Barakat, A. G., Reilkoff, R., Bezdicek, T., Schacker, T., Chipman, J. G., et al. (2020). Understanding the renin-angiotensin-aldosterone-SARS-CoV axis: a comprehensive review. Eur. Respir. J. 56:2000912. doi: 10.1183/13993003.00912-2020
Kadomatsu, K. (2005). The midkine family in cancer, inflammation and neural development. Nagoya J. Med. Sci. 67, 71–82.
Kadomatsu, K. (2010). Midkine regulation of the renin-angiotensin system. Curr. Hypertens. Rep. 12, 74–79. doi: 10.1007/s11906-010-0092-8
Kadomatsu, K., Kishida, S., and Tsubota, S. (2013). The heparin-binding growth factor midkine: the biological activities and candidate receptors. J. Biochem. 153, 511–521. doi: 10.1093/jb/mvt035
Kadomatsu, K., Tomomura, M., and Muramatsu, T. (1988). cDNA cloning and sequencing of a new gene intensely expressed in early differentiation stages of embryonal carcinoma cells and in mid-gestation period of mouse embryogenesis. Biochem. Biophys. Res. Commun. 3, 1312–1318. doi: 10.1016/s0006-291x(88)80505-9
Kaneda, N., Talukder, A., Ishihara, M., Hara, S., Yoshida, K., and Muramatsu, T. (1996). Structural characteristics of heparin-like domain required for interaction of midkine with embryonic neurons. Biochem. Biophys. Res. Commun. 220, 108–112. doi: 10.1006/bbrc.1996.0365
Kim, S. Y., Jin, W., Sood, A., Montgomery, D. W., Grant, O. C., Fuster, M. M., et al. (2020). Characterization of heparin and severe acute respiratory syndrome-related coronavirus 2 (SARS-CoV2) spike glycoprotein binding interactions. Antivir. Res. 181:104873. doi: 10.1016/j.antiviral.2020.104873
Kim, H. M., Kang, Y. H., Byun, J. H., Jang, S. J., Rho, G. J., Lee, J. S., et al. (2017). Midkine and NANOG have similar immunohistochemical expression patterns and contribute equally to an adverse prognosis of oral squamous cell carcinoma. Int. J. Mol. Sci. 18, 2339–2354. doi: 10.3390/ijms18112339
Kojima, T., Katsumi, A., Yamazaki, T., Muramatsu, T., Nagasaka, T., Ohsumi, K., et al. (1996). Human ryudocan from endothelium-like cells binds basic fibroblast growth factor, midkine, and tissue factor pathway inhibitor. J. Biol. Chem. 271, 5914–5920. doi: 10.1074/jbc.271.10.5914
Lajoie, P., and Nabi, I. R. (2007). Regulation of raft-dependent endocytosis. J. Cell. Mol. Med. 11, 644–653. doi: 10.1111/j.1582-4934.2007.00083.x
Leppkes, M., Knopf, J., Naschberger, E., Lindemann, A., Singh, J., Herrmann, I., et al. (2020). Vascular occlusion by neutrophil extracellular traps in COVID-19. EBioMedicine 58:102925. doi: 10.1016/j.ebiom.2020.102925
Li, G. M., Li, Y. G., Yamate, M., Li, S. M., and Ikutaa, K. (2007). Lipid rafts play an important role in the early stage of severe acute respiratory syndrome-coronavirus life cycle. Microbes Infect. 9, 96–102. doi: 10.1016/j.micinf.2006.10.015
Liu, L., Chopra, P., Li, X., Wolfert, M. A., Tompkins, S. M., and Boons, G. J. (2020). SARS-CoV2 spike protein binds heparan sulfate in a length‐ and sequence-dependent manner. bioRxiv [Preprint]. doi: 10.1101/2020.05.10.087288
Lu, Y., Liu, D. X., and Tam, J. P. (2008). Lipid rafts are involved in SARS-CoV entry into Vero E6 cells. Biochem. Biophys. Res. Commun. 369, 344–349. doi: 10.1016/j.bbrc.2008.02.023
Lu, P., Takai, K., Weaver, V. M., and Werb, Z. (2011). Extracellular matrix degradation and remodeling in development and disease. Cold Spring Harb. Perspect. Biol. 3:a005058. doi: 10.1101/cshperspect.a005058
Lv, X., Wen, T., Song, J., Xie, D., Wu, L., Jiang, X., et al. (2017). Extracellular histones are clinically relevant mediators in the pathogenesis of acute respiratory distress syndrome. Respir. Res. 18:165. doi: 10.1186/s12931-017-0651-5
Maeda, N., Ichihara-Tanaka, K., Kimura, T., Kadomatsu, K., Muramatsu, T., and Noda, M. (1999). A receptor-like protein-tyrosine phosphatase PTPzeta/RPTPbeta binds a heparin-binding growth factor midkine: involvement of arginine 78 of midkine in the high affinity binding to PTPzeta. J. Biol. Chem. 274, 12474–12479. doi: 10.1074/jbc.274.18.12474
Maeda, S., Shinchi, H., Kurahara, H., Mataki, Y., Noma, H., Maemura, K., et al. (2007). Clinical significance of midkine expression in pancreatic head carcinoma. Br. J. Cancer 97, 405–411. doi: 10.1038/sj.bjc.6603879
Milewska, A., Zarebski, M., Nowak, P., Stozek, K., Potempa, J., and Perlman, K. P. (2014). Human coronavirus NL63 utilizes heparan sulfate proteoglycans for attachment to target cells. J. Virol. 88, 13221–13230. doi: 10.1128/JVI.02078-14
Misa, K., Tanino, Y., Wang, X., Nikaido, T., Kikuchi, M., Sato, Y., et al. (2017). Involvement of midkine in the development of pulmonary fibrosis. Physiol. Rep. 5:e13383. doi: 10.14814/phy2.13383
Muramatsu, H., Inui, T., Kimura, T., Sakakibara, S., Song, X., Murata, H., et al. (1994). Localization of heparin-binding, neurite outgrowth and antigenic regions in midkine molecule. Biochem. Biophys. Res. Commun. 203, 1131–1139. doi: 10.1006/bbrc.1994.2300
Muramatsu, H., Zou, K., Sakaguchi, N., Ikematsu, S., Sakuma, S., and Muramatsu, T. (2000). LDL receptor-related protein as a component of the midkine receptor. Biochem. Biophys. Res. Commun. 270, 936–941. doi: 10.1006/bbrc.2000.2549
Mycroft-West, C., Su, D., Elli, S., Guimond, S., Miller, G., Turnbull, J., et al. (2020). The 2019 coronavirus (SARS-CoV2) surface protein (Spike) S1Receptor Binding Domain undergoes conformational change upon heparin binding. bioRxiv [Preprint]. doi: 10.1101/2020.02.29.971093
Narasaraju, T., Yang, E., Samy, R. P., Ng, H. H., Poh, W. P., Liew, A. A., et al. (2011). Excessive neutrophils and neutrophil extracellular traps contribute to acute lung injury of influenza pneumonitis. Am. J. Pathol. 179, 199–210. doi: 10.1016/j.ajpath.2011.03.013
Nikolich-Zugich, J., Knox, K. S., Rios, C. T., Natt, B., Bhattacharya, D., and Fain, M. J. (2020). SARS-CoV-2 and COVID-19 in older adults: what we may expect regarding pathogenesis, immune responses, and outcomes. GeroScience 42, 505–514. doi: 10.1007/s11357-020-00186-0
Novotny, W. F., Maffi, T., Mehta, R. L., and Milner, P. G. (1993). Identification of novel heparin-releasable proteins, as well as the cytokines midkine and pleiotrophin, in human postheparin plasma. Arterioscler. Thromb. 13, 1798–1805. doi: 10.1161/01.ATV.13.12.1798
O’Brien, T., Cranston, D., Fuggle, S., Bicknell, R., and Harris, A. L. (1996). The angiogenic factor midkine is expressed in bladder cancer, and overexpression correlates with a poor outcome in patients with invasive cancers. Cancer Res. 56, 2515–2518.
Omarjee, L., Janin, A., Perrot, F., Laviolle, B., Meilhac, O., Mahe, G., et al. (2020). Targeting T-cell senescence and cytokine storm with rapamycin to prevent severe progression in COVID-19. Clin. Immunol. 216:108464. doi: 10.1016/j.clim.2020.108464
Reynolds, P. R., Mucenski, M. L., Le Cras, T. D., Nichols, W. C., and Whitsett, J. A. (2004). Midkine is regulated by hypoxia and causes pulmonary vascular remodeling. J. Biol. Chem. 279, 37124–37132. doi: 10.1074/jbc.M405254200
Rezaie, A. R. (2010). Regulation of the protein C anticoagulant and antiinflammatory pathways. Curr. Med. Chem. 17, 2059–2069. doi: 10.2174/092986710791233706
Rusnati, M., Vicenzi, E., Donalisio, M., Oreste, P., Landolfo, S., and Lembo, D. (2009). Sulfated K5 Escherichia coli polysaccharide derivatives: a novel class of candidate antiviral microbicides. Pharmacol. Ther. 123, 310–322. doi: 10.1016/j.pharmthera.2009.05.001
Salvati, F., Santoro, A., and Pedone, C. (2011). BPCO e nefropatie croniche. Ital. J. Med. 5S, 67–74. doi: 10.1016/j.itjm.2011.01.010
Sato, W., Kadomatsu, K., Yuzawa, Y., Muramatsu, H., Hotta, N., Matsuo, S., et al. (2001). Midkine is involved in neutrophil infiltration into the tubulointerstitium in ischemic renal injury. J. Immunol. 167, 3463–3469. doi: 10.4049/jimmunol.167.6.3463
Siddiqi, H. K., and Mehra, M. R. (2020). COVID-19 illness in native and immunosuppressed states: a clinical-therapeutic staging proposal. J. Heart Lung Transplant. 39, 405–407. doi: 10.1016/j.healun.2020.03.012
Stephen-Victor, E., Das, M., Karnam, A., Pitard, B., Gautier, J. F., and Bayry, J. (2020). Potential of regulatory T cell-based therapies in the management of severe COVID-19. Eur. Respir. J. 56:2002182. doi: 10.1183/13993003.02182-2020
Takada, T., Toriyama, K., Muramatsu, H., Song, X. J., Torii, S., and Muramatsu, T. (1997). Midkine, a retinoic acid-inducible heparin-binding cytokine in inflammatory responses: chemotactic activity to neutrophils and association with inflammatory synovitis. J. Biochem. 122, 453–458. doi: 10.1093/oxfordjournals.jbchem.a021773
Takeuchi, H. (2014). Midkine and multiple sclerosis. Br. J. Pharmacol. 171, 931–935. doi: 10.1111/bph.12499
Terrazzano, G., Rubino, V., Palatucci, A. T., Giovazzino, A., Carriero, F., and Ruggiero, G. (2020). An open question: is it rational to inhibit the mTor-dependent pathway as COVID-19 therapy? Front. Pharmacol. 11:856. doi: 10.3389/fphar.2020.00856
Tinholt, M., Stavik, B., Louch, W., Rein Carlson, C., Sletten, M., Ruf, W., et al. (2015). Syndecan-3 and TFPI Colocalize on the surface of endothelial-, smooth muscle-, and cancer cells. PLoS One 10:e0117404. doi: 10.1371/journal.pone.0117404
Toh, C. H., Alhamdi, Y., and Abrams, S. T. (2016). Current pathological and laboratory considerations in the diagnosis of disseminated intravascular coagulation. Ann. Lab. Med. 36, 505–512. doi: 10.3343/alm.2016.36.6.505
V’kovski, P., Kratzel, A., Steiner, S., Stalder, H., and Thiel, V. (2020). Coronavirus biology and replication: implications for SARS-CoV-2. Nat. Rev. Microbiol. 1–16. doi: 10.1038/s41579-020-00468-6
Vallamkondu, J., John, A., Wani, W. Y., Ramadev, S. P., Jella, K. K., Reddy, P. H., et al. (2020). SARS-CoV2 pathophysiology and assessment of coronaviruses in CNS diseases with a focus on therapeutic targets. Biochim. Biophys. Acta Mol. basis Dis. 1866:165889. doi: 10.1016/j.bbadis.2020.165889
Verdecchia, P., Cavallini, P., Spanevello, A., and Angeli, F. (2020). The pivotal link between ACE2 deficiency and SARS-CoV2 infection. Eur. J. Intern. Med. 76, 14–20. doi: 10.1016/j.ejim.2020.04.037
Weckbach, L. T., Grabmaier, U., Uhl, A., Gess, S., Boehm, F., Zehrer, A., et al. (2019). Midkine drives cardiac inflammation by promoting neutrophil trafficking and NETosis in myocarditis. J. Exp. Med. 216, 350–368. doi: 10.1084/jem.20181102
Weckbach, L. T., Groesser, L., Borgolte, J., Pagel, J. I., Pogoda, F., Schymeinsky, J., et al. (2012). Midkine acts as proangiogenic cytokine in hypoxia-induced angiogenesis. Am. J. Physio. Heart. Circ. Physiol. 303, 429–438. doi: 10.1152/ajpheart.00934.2011
Weckbach, L. T., Muramatsu, T., and Walzog, B. (2011). Midkine in inflammation. ScientificWorldJournal 11, 2491–2505. doi: 10.1100/2011/517152
Xia, X., Lu, J. J., Zhang, S. S., Su, C. H., and Luo, H. H. (2016). Midkine is a serum and urinary biomarker for the detection and prognosis of non-small cell lung cancer. Oncotarget 7, 87462–87472. doi: 10.18632/oncotarget.13865
Zhang, H., and Baker, A. (2017). Recombinant human ACE2: acing out angiotensin II in ARDS therapy. Crit. Care 21:305. doi: 10.1186/s13054-017-1882-z
Zhang, R., Pan, Y., Fanelli, V., Wu, S., Luo, A. A., Islam, D., et al. (2015). Mechanical stress and the induction of lung fibrosis via the midkine signaling pathway. Am. J. Respir. Crit. Care Med. 192, 315–323. doi: 10.1164/rccm.201412-2326OC
Keywords: midkine, SARS-CoV2, COVID-19, neutrophil infiltration, NETs, autophagy, immune responses
Citation: Sanino G, Bosco M and Terrazzano G (2020) Physiology of Midkine and Its Potential Pathophysiological Role in COVID-19. Front. Physiol. 11:616552. doi: 10.3389/fphys.2020.616552
Edited by:
Deborah Schechtman, University of São Paulo, BrazilReviewed by:
Jawed Fareed, Loyola University Medical Center, United StatesJanos Paloczi, National Institutes of Health (NIH), United States
Copyright © 2020 Sanino, Bosco and Terrazzano. This is an open-access article distributed under the terms of the Creative Commons Attribution License (CC BY). The use, distribution or reproduction in other forums is permitted, provided the original author(s) and the copyright owner(s) are credited and that the original publication in this journal is cited, in accordance with accepted academic practice. No use, distribution or reproduction is permitted which does not comply with these terms.
*Correspondence: Giulia Sanino, giuliasanino84@gmail.com; Giuseppe Terrazzano, giuseppe.terrazzano@unibas.it