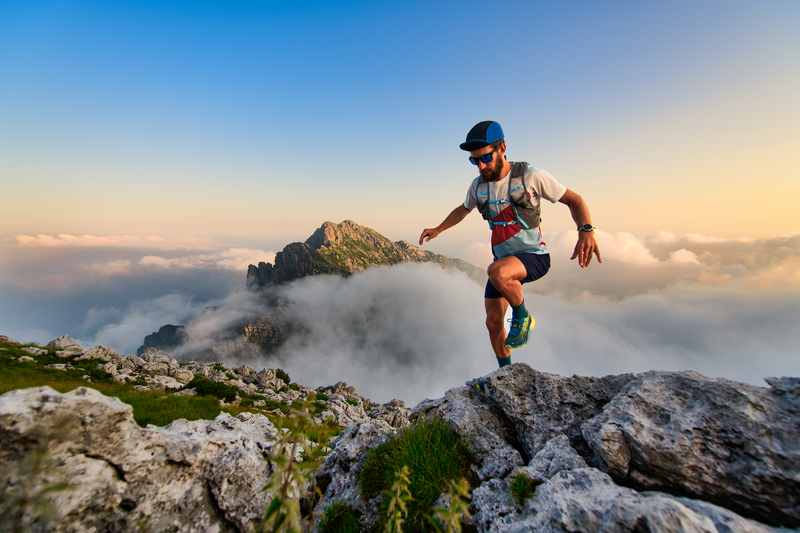
95% of researchers rate our articles as excellent or good
Learn more about the work of our research integrity team to safeguard the quality of each article we publish.
Find out more
PERSPECTIVE article
Front. Physiol. , 20 January 2021
Sec. Vascular Physiology
Volume 11 - 2020 | https://doi.org/10.3389/fphys.2020.616189
This article is part of the Research Topic Mechanisms of Vessel Development: From a Primitive Draft to a Mature Vasculature View all 14 articles
Cardiovascular diseases are the leading cause of mortality worldwide. Such a widespread diffusion makes the conditions affecting the heart and blood vessels a primary medical and economic burden. It, therefore, becomes mandatory to identify effective treatments that can alleviate this global problem. Among the different solutions brought to the attention of the medical-scientific community, therapeutic angiogenesis is one of the most promising. However, this approach, which aims to treat cardiovascular diseases by generating new blood vessels in ischemic tissues, has so far led to inadequate results due to several issues. In this perspective, we will discuss cutting-edge approaches and future perspectives to alleviate the potentially lethal impact of cardiovascular diseases. We will focus on the consolidated role of resident endothelial progenitor cells, particularly endothelial colony forming cells, as suitable candidates for cell-based therapy demonstrating the importance of targeting intracellular Ca2+ signaling to boost their regenerative outcome. Moreover, we will elucidate the advantages of physical stimuli over traditional approaches. In particular, we will critically discuss recent results obtained by using optical stimulation, as a novel strategy to drive endothelial colony forming cells fate and its potential in the treatment of cardiovascular diseases.
The vascular network is indispensable for all organisms to distribute oxygen (O2) and nutrients to the tissues and to remove carbon dioxide and other metabolic waste products (Heinke et al., 2012). Additionally, the circulatory system serves to maintain homeostasis by stabilizing body temperature and avoiding pH unbalance, to facilitate inter-organ humoral communication, and finally, to guide immune cells towards sites of inflammation or infection (Heinke et al., 2012; Udan et al., 2013). Insufficient vascularization or impairment of regional blood flow due to local vessel obstruction results in ischemia, thereby promoting coronary artery disease, acute myocardial infarction, peripheral artery disease, stroke, pre-eclampsia, and obesity- or neurodegenerative associated disorders (Draoui et al., 2017; Potente and Mäkinen, 2017). Cardiovascular disease (CVD) induced by disruption of the vascular network in heart, limbs and brain is, therefore, regarded as a global medical and economic issue with high prevalence and mortality rates (Benjamin et al., 2019). The World Health Organization and Global Burden Disease have listed CVD as the first cause of death worldwide (Mensah et al., 2019). Therapeutic angiogenesis (TA) represents a promising strategy that aims at reconstructing the damaged vascular network by stimulating the regrowth of the endothelial cell layer that lines the inner lumen of blood vessels and plays a crucial role in adjusting blood supply according to local energy demand (Qadura et al., 2018; Prasad et al., 2020). Endothelial colony forming cells (ECFCs), which represent the only known truly endothelial precursor (Medina et al., 2017), are mobilized in peripheral circulation to maintain endothelial homeostasis throughout postnatal life and to rescue local blood flow upon an ischemic insult (D’Alessio et al., 2015; Tasev et al., 2016; O’Neill et al., 2018). A wealth of in vitro and in vivo studies has been recently carried out to design an effective strategy to stimulate endogenous ECFCs’ regenerative potential for therapeutic purposes, thereby alleviating the life-threatening impact of CVD (Tasev et al., 2016; Moccia et al., 2018a; O’Neill et al., 2018; Paschalaki and Randi, 2018).
In this perspective, we will briefly describe how endothelial precursors generate the primitive vascular plexus and can, therefore, be exploited for TA. Then, we will explain the rationale for targeting the Ca2+ handling machinery, which delivers a crucial pro-angiogenic signaling input. Finally, we will review recent approaches, based on the use of physical stimuli in place of chemical cues. Specifically, we will report on the use of visible light pulses to stimulate ECFCs’ proliferation and bidimensional tube formation. Optical modulation could provide an effective strategy to rescue ECFCs’ vasoreparative potential in patients affected by CVD and to circumvent the main hurdles associated to autologous stem cell therapy.
The circulatory system is the first functional organ to develop (already during gastrulation) with the purpose to supply growing tissues with O2 and nutrients and thereby sustain organism growth (Udan et al., 2013; Potente and Mäkinen, 2017). Embryonic blood vessels arise from endothelial progenitor cells (EPCs), also known as angioblasts, which differentiate from multipotent mesodermal precursors. EPCs coalesce and assembly into a primitive capillary plexus, according to a process known as vasculogenesis. This is followed by further expansion of the vascular network via angiogenesis, which may occur through either sprouting or splitting of pre-existing vessels (Udan et al., 2013; Potente and Mäkinen, 2017). The endothelial monolayer retains a state of proliferative quiescence for years, but it may undergo sprouting angiogenesis to meet local metabolic demand under hypoxia, i.e., during skeletal muscle exercise, or in the cycling ovary and in the placenta during pregnancy (Potente and Mäkinen, 2017). Furthermore, EPCs may be released on demand by cytokines released from hypoxic/injured tissues to support local angiogenesis and rescue local blood flow (Moccia et al., 2012; O’Neill et al., 2018). Since the landmark discovery of a population of endothelial precursors circulating in peripheral blood (Asahara et al., 1997), multiple EPC subtypes were isolated, characterized and probed for their therapeutic potential (Asahara et al., 2011; Keighron et al., 2018). Nevertheless, ECFCs were recently presented as the most suitable cellular substrate for regenerative therapy of CVD (Moccia et al., 2015; Tasev et al., 2016; Medina et al., 2017; Paschalaki and Randi, 2018; O’Leary et al., 2019). Unlike other myeloid EPC subtypes, which stimulate neovessel growth in a paracrine manner, ECFCs display the following properties: (1) they are truly endothelial progenitors, able to assembly into capillary-networks in vitro and to form patent vessels in vivo; (2) display high clonogenic potential and may be replated into secondary and tertiary colonies; (3) rescue injured vascular networks by physically engrafting within neovessels and by releasing pro-angiogenic signals; (4) interact with perimural cells, which ensures neovessel stability; and (5) are more amenable for pharmacological and genetic manipulation aiming at improving their vasoreparative phenotype (Moccia et al., 2015, 2018a,b; Tasev et al., 2016; Medina et al., 2017; Paschalaki and Randi, 2018; O’Leary et al., 2019).
A finely tuned spatio-temporal increase in intracellular Ca2+ concentration [(Ca2+)i] in vascular endothelial cells has long been known to stimulate angiogenesis (Fiorio Pla and Munaron, 2014; Moccia et al., 2014, 2019; Negri et al., 2020a). Endothelial Ca2+ signals may indeed mediate the pro-angiogenic effect of multiple growth factors, including vascular endothelial growth factor (VEGF; Potenza et al., 2014; Yokota et al., 2015; Savage et al., 2019), and epidermal growth factor (Moccia et al., 2003), inflammatory mediators, such as ATP (Moccia et al., 2001), and pleiotropic hormones, such as erythropoietin (Yu et al., 2017). Likewise, a recent series of reports documented that intracellular Ca2+ signals stimulate ECFCs to undergo angiogenesis both in vitro (Zuccolo et al., 2016; Lodola et al., 2017a; Wu et al., 2017) and in vivo (Zuccolo et al., 2018; Balbi et al., 2019). For instance, VEGF-induced intracellular Ca2+ oscillations stimulated ECFC proliferation and tube formation by promoting the nuclear translocation of the Ca2+-sensitive transcription factor, nuclear factor-κB (NF-κB; Dragoni et al., 2015b; Lodola et al., 2017a), whereas biphasic Ca2+ signals favored stromal derived factor-1α (SDF-1α)-induced ECFC homing to injured tissues by recruiting the extracellular signal-regulated kinase (ERK) and phosphoinositide 3-kinases (PI3K)/Akt (Zuccolo et al., 2018). The Ca2+ response to these pro-angiogenic cues was initiated by endogenous Ca2+ release from the endoplasmic reticulum (ER) through inositol-1,4,5-trisphosphate (InsP3) receptors (InsP3Rs), followed by store-operated Ca2+ entry (SOCE) activation (Lodola et al., 2017a; Zuccolo et al., 2018; Figure 1). SOCE is activated upon InsP3-induced ER Ca2+ depletion to refill the ER with Ca2+ and is mediated by the interplay among STIM1, Orai1, and Transient Receptor Potential (TRP) Canonical 1 in ECFCs (Lodola et al., 2012; Figure 1). TRP channels provide an alternative pathway for extracellular Ca2+ entry in both vascular endothelial cells (Negri et al., 2020a) and ECFCs (Inoue and Xiong, 2009; Hofmann et al., 2014; Dragoni et al., 2015a; Figure 1). Endothelial cells use TRP channels to sense the local microenvironment in which they reside, thereby adapting to subtle changes in the chemical composition of the extracellular milieu and/or in the mechanical forces acting on the vascular wall (Genova et al., 2020; Negri et al., 2020a). For instance, the endothelial TRPV1 is sensitive to an increase in local temperature above 43°C (Negri et al., 2020b) and/or in local hydrogen peroxide (H2O2; DelloStritto et al., 2016), whereas TRPV4 is sensitive to physical stimuli, such as shear stress (Schierling et al., 2011) and pulsatile stretch (Thodeti et al., 2009), and to arachidonic acid (AA) production (Fiorio Pla et al., 2008). Recent studies suggested that TRP channels may also stimulate ECFCs’ angiogenic activity. For instance, TRPV1-mediated uptake of anandamide stimulates ECFC migration (Hofmann et al., 2014), whereas TRPV4-mediated nitric oxide release promotes the pro-angiogenic effects of AA (Zuccolo et al., 2016). It has, therefore, been suggested that targeting TRP channels could represent an efficient strategy to boost ECFCs’ regenerative potential (Moccia et al., 2015, 2018a). Indeed, TRP channels are physically coupled to specific Ca2+-dependent effectors which translate extracellular Ca2+ entry through specific pathways into precise biological outputs which differentially affect endothelial cell fate (Smani et al., 2018; Genova et al., 2020; Negri et al., 2020a).
Figure 1. Endothelial colony forming cells (ECFCs) Ca2+ machinery and pro-angiogenic Ca2+ signals. Growth factors (like VEGF or IGF2) and chemokines (like SDF-1) bind to Receptor tyrosine kinases (RTKs) and G protein-coupled receptors (GPCRs) respectively, thus activating specific PLC isoforms, which in turn leads to production of inositol 1,4,5-trisphosphate (InsP3). InsP3 binds to InsP3 receptors (InsP3R) bearing the release of Ca2+ from the endoplasmic reticulum (ER) pool. The Ca2+ store depletion, detected by Ca2+ sensor Stromal interaction molecule 1 (STIM1), is the signal for store-operated calcium entry (SOCE) activation. SOCE, the major Ca2+ entry pathway in ECFCs, is mediated by the interaction among STIM1 and the proteins Orai1 and Transient receptor potential canonical 1 (TRPC1). These plasma membrane pore channels allow Ca2+ entry from the extracellular space that will be subsequently transported from the cytosol within the SR by the Sarco-Endoplasmic Reticulum Calcium ATPase (SERCA), while Plasma membrane Calcium ATPase (PMCA) contributes to clear cytosolic Ca2+ levels. Transient receptor potential vanilloid (TRPV) channels (TRPV1 and TRPV4) also represent an alternative pathway for extracellular Ca2+ entry in ECFCs. These intracellular Ca2+ signals evoke pathways (NF-κB, ERK1/2, PI3K/Akt) that lead to nuclear transcription of pro-angiogenic factors.
ECFCs hold great promise for TA. Conversely, clinical trials clearly showed that cell therapy based upon transplantation of myeloid EPCs fail to induce a remarkable improvement in capillary density and local blood flow in patients affected by CVD (Moccia et al., 2012; Prasad et al., 2020). Indeed, an array of hurdles hampered the enthusiasm towards ECFC introduction in therapy. Firstly, the frequency of circulating ECFCs is rather low, ranging from 0.28 to 15 ECFCs/107 mononuclear cells, which is insufficient to achieve a therapeutically relevant outcome (Moccia et al., 2017, 2018a). Secondly, ECFCs’ angiogenic activity is severely compromised by CVD (Sung et al., 2013; Mauge et al., 2014; Su et al., 2017; Komici et al., 2020) and by cardiovascular risk factors (Shelley et al., 2012; Jarajapu et al., 2014; Mena et al., 2018), which may ultimately lead to ischemia-related disorders. Thirdly, ECFCs’ angiogenic activity could be further reduced once they reach the harsh microenvironment of ischemic tissues. For instance, ECFC proliferation and tube formation are affected in the presence of elevated pro-inflammatory signaling (Mena et al., 2018), oxidative stress (Gremmels et al., 2017), and hypoxia (He et al., 2018; Tasev et al., 2018). As recently reviewed (Faris et al., 2020), the therapeutic use of umbilical cord blood-derived ECFCs, which display a greater pro-angiogenic potential as compared to circulating ECFCs, is currently not feasible for the high cost of their processing and banking and potential immune complications. It has, therefore, been proposed that the therapeutic outcome of ECFCs-based treatment of CVD could be remarkably improved by boosting the specific pro-angiogenic signaling pathways of circulating ECFCs (Tasev et al., 2016; Moccia et al., 2018a,b; Paschalaki and Randi, 2018).
The evidence that ECFC harvested from CVD patients often present a dysfunctional phenotype with low proliferative potential and reduced vasculogenic and angiogenic capability boosted numerous efforts to improve ECFC therapeutic efficacy (Paschalaki and Randi, 2018). The large majority of these trials relies on a chemical approach, and include epigenetic activation through stimulation of proangiogenic signaling pathways by specific drugs, as well as administration of bioactive compounds (i.e. fucoidan, genistein, globular adiponectin; Tasev et al., 2016). Very recently, acidic preconditioning has been also reported to have positive effects on ECFC adhesion, vascular density and inflammation reduction (Mena et al., 2018). Chemically controlled methods proved to be successful in many cases. Unfortunately, they are mostly considered to be still insufficient to modulate ECFCs’ activity and to promote TA in a fully satisfactory way. In more detail, their critical limitations consist in limited spatial and temporal resolution of administration, as well as lack of reversibility. Thus, the opportunity to employ physical stimuli has been emerging in the latest years as an alternative, innovative tool to control ECFC fate. Several possibilities are being explored in this direction. First, the effects of micropatterning and nano-patterning and, more generally, of mechanical cues, is under intensive investigation. The hypothesis that the direct micropatterning of ECFCs induces morphological elongation, cytoskeletal alignment, and changes in immunogenic and thrombogenic–related gene expression, is being tested. It was recently reported that ECFCs cultured on top of micropatterned polyurethane substrates show sizable alignment to the underlying substrate geometry, accompanied by the alignment of actin fibers and microtubules. However, this did not correspond to significant cellular elongation in the case of ECFCs, nor to sizable changes in the expression of the transcription factor Krüppel-like Factor 2 (KLF-2) or its downstream targets (Hagen and Hinds, 2020). Conversely, in another work, cells patterned on 25 μm-wide lanes, created by alternating collagen-I and a blocking polymer, clearly displayed elongation, and actin alignment. Micropatterning increased their packing densities, without affecting the apoptotic rate, and KLF-2 gene expression was increased in micropatterned relative to non-patterned ECFCs after 50 h. No significant differences were seen in the other genes tested (Hagen and Hinds, 2019). Lower, sub-micrometric scale was also addressed; patterning of ECFCs in this case lead to a decrease in the ECFC area and perimeter, as well as to an increase in their filopodial outgrowth, associated with a modulation of the focal adhesions and overexpression of the ROCK gene (Cui et al., 2018). Overall, however, the number of studies addressing the use of mechanical stimuli on ECFCs is still very limited and does not allow for sketching a complete picture of their effects.
A second possible approach, still in the early stages, is the use of electromagnetic stimulation. It was reported that electrical stimulation, provided by a wearable solar cell, favored the secretion of angiogenic growth factors and EPC migration (Jeong et al., 2017). Moreover, electrical stimulation promoted the formation of capillaries and arterioles in a mouse model of ischemia, while attenuating muscle necrosis and fibrosis and eventually preventing loss of the injured limb. Interestingly, it was also reported that electrical stimulation significantly increases, among other effects, the number of EPCs in the peripheral blood of rats subjected to fluid percussion injury (Zheng et al., 2017). Magnetic field-guided transplantation of silica-coated magnetic iron oxide nanoparticle-labeled EPCs was associated with their enhanced aggregation in the infarcted border zone (Zhang et al., 2019). These initial, promising results are expected to boost the investigation of electromagnetic stimulation in the field of TA, and in more detail the investigation of the effects of a localized electromagnetic field on ECFC activity.
Thirdly, the use of light stimuli may be perfectly suited for TA. In the last decade, the scientific community has exploited the use of light to control the activity of different cell types genetically modified to express light-sensitive ion channels, thus gaining an unprecedented control in terms of selectivity and reversibility (Knollmann, 2010; Deisseroth, 2011). An alternative strategy, that obviates the need of viral gene transfer is based on the use of hybrid interfaces between living cells and organic semiconductors (OS), used as artificial light transducers (Rivnay et al., 2017; Di Maria et al., 2018; Fang et al., 2020; Ohayon and Inal, 2020). OS, and thiophene-based materials in particular, have emerged as promising tools for biological application, thanks to a series of key-enabling characteristics: they are soft materials with a high degree of mechanical conformability; they are highly biocompatible and very well tolerated within in vivo conditions; they support both electronic and ionic charge conduction; they are sensitive to visible and near-infrared light; they are easily processed from solution. Among other materials, it has been demonstrated that optical excitation of regioregular Poly (3-hexyl-thiophene), P3HT, reliably and efficiently modulates the activity of living cells, tissues and systems, including non-excitable (Benfenati et al., 2014; Martino et al., 2015) and excitable cells (Ghezzi et al., 2011; Feyen et al., 2016; Lodola et al., 2019b), retinal explants (Ghezzi et al., 2013), as well as invertebrate (Tortiglione et al., 2017) and mammal animal models (Maya-Vetencourt et al., 2017). It has been also reported that illumination of thiophene thin films leads to a functional interplay with cytochrome C protein, opening the path to selective targeting of sub-cellular organelles (Abdel Aziz et al., 2020).
Interestingly, it was demonstrated that optical excitation of P3HT leads to sizable modulation of TRPV1 channels, in TRPV1 Stable Cell Line-HEK-293 (Lodola et al., 2017b). Moreover, we unambiguously proved that optical excitation of thiophene-based materials leads to non-toxic activation of photoelectrochemical phenomena (Tullii et al., 2017; Abdel Aziz et al., 2020), i.e., reactive oxygen species (ROS) generation and subsequent modulation of Ca2+ dynamics (Bossio et al., 2018; Moros et al., 2018). Indeed, reduction of the oxygen present in the extracellular medium in consequence to the polymer photoexcitation leads to the formation of superoxide (O2−), intermediate ROS and ends up with spatially and temporally controlled generation of H2O2, which, in turn, can permeate the plasma membrane, thereby causing an increase in the cytosolic H2O2 levels, which can activate TRPV1 and induced extracellular Ca2+ entry (DelloStritto et al., 2016; Lodola et al., 2019a). A local reduction in extracellular pH because of polymer photoexcitation could also gate TRPV1 (Negri et al., 2020b), but its role in P3HT-mediated TRPV1 activation remains to be investigated.
This experimental evidence prompted us to investigate whether a similar optically-triggered approach could have a beneficial effect on the modulation of ECFC’s angiogenic activity. To this purpose, circulating ECFCs were seeded on top of P3HT and subjected to light stimulation in the green visible region (Figure 2A). We observed that P3HT excitation leads to spatiotemporally resolved modulation of the Ca2+ permeable TRPV1 channel, as well as increased ECFC proliferation and tubulogenesis (Lodola et al., 2019a). The interplay among these experimental evidences was clarified by means of a detailed pharmacological analysis: TRPV1 inhibition and manipulation of intracellular free Ca2+ levels by selective drugs impaired the pro-angiogenic effect of P3HT excitation thus highlighting the pivotal role of TRPV1-mediated Ca2+ influx in ECFC proliferation and tube formation. Moreover, we experimentally identified the phototransduction effect as due to a temporally and spatially localized activation of photoelectrochemical reactions at the interface between the conjugated polymer surface and the cell membrane. Finally, we depicted the molecular scenario observing that polymer photoexcitation led to a significant nuclear translocation of the Ca2+-sensitive transcription factor NF-kB and subsequent up-regulated the mRNA levels of specific pro-angiogenic genes (Figure 2B). Overall, these results start paving the way towards the use of conjugated polymers as reliable and efficient functional materials for precise and reversible optically-driven modulation of ECFC physiological activity.
Figure 2. Conjugated polymers optically drive the fate of Endothelial Colony Forming Cells. (A) Sketch of the polymer device used for cell optical activation. ECFCs are cultured on top of P3HT thin films, deposited on glass substrates. Optical excitation is provided by a green LED (λMAXEM 525 nm). (B) Photo-thermal and photo-electrochemical reactions occur at the interface between material and ECFC membrane. The latter is the predominant mechanism triggering TRPV1 activation. A subsequent increase in [Ca2+]i results in the degradation of IκB, the inhibitory sub-unit of the transcriptional factor NF-κB. As a consequence, the p65 NF-κB subunit is released from IκB inhibition and translocates into the nucleus leading to a robust up-regulation of angiogenic genes, which are under NF-κB-dependent transcriptional control.
In this perspective, we have summarized the most recent outcomes in the field of TA. ECFCs are emerging as suitable candidates for cell-based therapy, but to achieve clinically relevant results it is pivotal to ameliorate current treatment limitations (i.e., insufficient circulating ECFCs frequency, impaired angiogenic activity in CVD, low engraftment, survival and integration within the inhospitable environment of damaged myocardium). The use of physical stimuli, a still less beaten path that should ideally receive increasing attention in the forthcoming years, may allow to overcome these drawbacks. The development of novel biohybrid interfaces between ECFC and materials endowed with electrical, photoacoustic, piezoelectric, magnetic, and/or optical properties may reveal a successful route for selective stimulation of pro-angiogenic signaling pathways. The portfolio of different possibilities is still fully open and among them, the use of optical stimuli represents a minimally invasive strategy, able to trigger the desired biophysical pathways with unprecedented selectivity and spatial resolution. In particular, the promising results shown by ECFC optical stimulation using light-sensitive conjugated polymers (Lodola et al., 2019a) may be further exploited in multiple directions. Optical stimulation could be harnessed to stimulate also capillary endothelial cells nearby the injury site, thereby promoting local angiogenesis. Besides circulating ECFCs, TRPV1 is largely expressed and drives proliferation and tube formation in vascular endothelial cells (Negri et al., 2020a,b). Light active materials can be easily patterned with micro- and sub-micrometer resolution, and processed in three-dimensional architectures (Tullii et al., 2020). Another possible action consists in the development of optically active beads, eventually functionalized with specific moieties, for the selective targeting of ECFCs. Polymer nanoparticles can be easily internalized within cells, can target subcellular organelles, show excellent photocatalytic properties, and are able to modulate intracellular Ca2+ dynamics and display optimal in vivo biocompatibility properties (Bossio et al., 2018; Maya-Vetencourt et al., 2020). Thus, they may serve as sub-micrometer active sites for local triggering of ECFC pathways relevant for TA. Moreover, conjugated polymers are prone to chemical functionalization with specific drugs, opening the possibility to couple optical excitation with on-demand pharmacological treatment. Many crucial issues should be carefully addressed in detail before any preclinical test can be envisaged: (i) understand the complex interplay among materials, physical stimuli and ECFCs biophysical pathways, e.g., the investigation of additional ROS-sensitive pro-angiogenic channels, such as TRP Melastatin 2 (Mittal et al., 2015); (ii) critically evaluate the dose-response efficiency and reliability of the different approaches and stimulation devices; (iii) assess any possible biocompatibility issue and adverse side effects; (iv) develop suitable tools for implantation and in vivo chronic use (i.e., engineering of proper waveguides as well as implementation of microscopic, minimally invasive light sources already optimized for optogenetics). Experimental studies in this direction, though highly promising, are currently at a very embryonal stage, and in our opinion would deserve supra-disciplinary efforts from the bioengineering, materials science, and physics communities. We believe the effort will be worth taking and will pay off in time.
The original contributions presented in the study are included in the article/supplementary material, further inquiries can be directed to the corresponding author.
All authors listed have made a substantial, direct and intellectual contribution to the work and approved it for publication.
The authors gratefully acknowledge financial support from: Italian Ministry of Education, University and Research (MIUR): Dipartimenti di Eccellenza Program (2018–2022) - Dept. of Biology and Biotechnology “L. Spallanzani”, University of Pavia (FM); Fondo Ricerca Giovani from the University of Pavia (FM); European Research Council (ERC) under the European Union’s Horizon 2020 research and innovation program ‘LINCE’, grant agreement n. 803621 (MA); EU Horizon 2020 FETOPEN-2018-2020 Programme ‘LION-HEARTED’, grant agreement n. 828984 (FM, MA, and FL).
The authors declare that the research was conducted in the absence of any commercial or financial relationships that could be construed as a potential conflict of interest.
Figures created with BioRender.com.
Abdel Aziz, I., Malferrari, M., Roggiani, F., Tullii, G., Rapino, S., and Antognazza, M. R. (2020). Light-triggered electron transfer between a conjugated polymer and cytochrome C for optical modulation of redox signaling. iScience 23:101091. doi: 10.1016/j.isci.2020.101091
Asahara, T., Kawamoto, A., and Masuda, H. (2011). Concise review: circulating endothelial progenitor cells for vascular medicine. Stem Cells 29, 1650–1655. doi: 10.1002/stem.745
Asahara, T., Murohara, T., Sullivan, A., Silver, M., van der Zee, R., Li, T., et al. (1997). Isolation of putative progenitor endothelial cells for angiogenesis. Science 275, 964–966. doi: 10.1126/science.275.5302.964
Balbi, C., Lodder, K., Costa, A., Moimas, S., Moccia, F., van Herwaarden, T., et al. (2019). Supporting data on in vitro cardioprotective and proliferative paracrine effects by the human amniotic fluid stem cell secretome. Data Brief 25:104324. doi: 10.1016/j.dib.2019.104324
Benfenati, V., Martino, N., Antognazza, M. R., Pistone, A., Toffanin, S., Ferroni, S., et al. (2014). Organic polymers: photostimulation of whole-cell conductance in primary rat neocortical astrocytes mediated by organic semiconducting thin films (Adv. Healthcare mater. 3/2014). Adv. Healthc. Mater. 3:306. doi: 10.1002/adhm.201470013
Benjamin, E. J., Muntner, P., Alonso, A., Bittencourt, M. S., Callaway, C. W., Carson, A. P., et al. (2019). Heart disease and stroke statistics—2019 update: a report from the American Heart Association. Circulation 139, e56–e528. doi: 10.1161/CIR.0000000000000659
Bossio, C., Abdel Aziz, I., Tullii, G., Zucchetti, E., Debellis, D., Zangoli, M., et al. (2018). Photocatalytic activity of polymer nanoparticles modulates intracellular calcium dynamics and reactive oxygen species in HEK-293 cells. Front. Bioeng. Biotechnol. 6:114. doi: 10.3389/fbioe.2018.00114
Cui, L. -H., Joo, H. J., Kim, D. H., Seo, H. -R., Kim, J. S., Choi, S. -C., et al. (2018). Manipulation of the response of human endothelial colony-forming cells by focal adhesion assembly using gradient nanopattern plates. Acta Biomater. 65, 272–282. doi: 10.1016/j.actbio.2017.10.026
D’Alessio, A., Moccia, F., Li, J. -H., Micera, A., and Kyriakides, T. R. (2015). Angiogenesis and vasculogenesis in health and disease. Bio. Med. Res. Int. 2015, 1–2. doi: 10.1155/2015/126582
DelloStritto, D. J., Connell, P. J., Dick, G. M., Fancher, I. S., Klarich, B., Fahmy, J. N., et al. (2016). Differential regulation of TRPV1 channels by H2O2: implications for diabetic microvascular dysfunction. Basic Res. Cardiol. 111:21. doi: 10.1007/s00395-016-0539-4
Di Maria, F., Lodola, F., Zucchetti, E., Benfenati, F., and Lanzani, G. (2018). The evolution of artificial light actuators in living systems: from planar to nanostructured interfaces. Chem. Soc. Rev. 47, 4757–4780. doi: 10.1039/C7CS00860K
Dragoni, S., Guerra, G., Pla, A. F., Bertoni, G., Rappa, A., Poletto, V., et al. (2015a). A functional transient receptor potential Vanilloid 4 (TRPV4) channel is expressed in human endothelial progenitor cells: TRPV4 EXPRESSION IN EPCs. J. Cell. Physiol. 230, 95–104. doi: 10.1002/jcp.24686
Dragoni, S., Reforgiato, M., Zuccolo, E., Poletto, V., Lodola, F., Ruffinatti, F. A., et al. (2015b). Dysregulation of VEGF-induced proangiogenic Ca2+ oscillations in primary myelofibrosis-derived endothelial colony-forming cells. Exp. Hematol. 43, 1019–1030.e3. doi: 10.1016/j.exphem.2015.09.002
Draoui, N., de Zeeuw, P., and Carmeliet, P. (2017). Angiogenesis revisited from a metabolic perspective: role and therapeutic implications of endothelial cell metabolism. Open Biol. 7:170219. doi: 10.1098/rsob.170219
Fang, Y., Meng, L., Prominski, A., Schaumann, E. N., Seebald, M., and Tian, B. (2020). Recent advances in bioelectronics chemistry. Chem. Soc. Rev. 49, 7978–8035. doi: 10.1039/D0CS00333F
Faris, P., Negri, S., Perna, A., Rosti, V., Guerra, G., and Moccia, F. (2020). Therapeutic potential of endothelial colony-forming cells in ischemic disease: strategies to improve their regenerative efficacy. Int. J. Mol. Sci. 21:7406. doi: 10.3390/ijms21197406
Feyen, P., Colombo, E., Endeman, D., Nova, M., Laudato, L., Martino, N., et al. (2016). Light-evoked hyperpolarization and silencing of neurons by conjugated polymers. Sci. Rep. 6:22718. doi: 10.1038/srep22718
Fiorio Pla, A., Grange, C., Antoniotti, S., Tomatis, C., Merlino, A., Bussolati, B., et al. (2008). Arachidonic acid-induced Ca2+ entry is involved in early steps of tumor angiogenesis. Mol. Cancer Res. 6, 535–545. doi: 10.1158/1541-7786.MCR-07-0271
Fiorio Pla, A., and Munaron, L. (2014). Functional properties of ion channels and transporters in tumour vascularization. Philos. Trans. R. Soc. B Biol. Sci. 369:20130103. doi: 10.1098/rstb.2013.0103
Genova, T., Gaglioti, D., and Munaron, L. (2020). Regulation of vessel permeability by TRP channels. Front. Physiol. 11:421. doi: 10.3389/fphys.2020.00421
Ghezzi, D., Antognazza, M. R., Dal Maschio, M., Lanzarini, E., Benfenati, F., and Lanzani, G. (2011). A hybrid bioorganic interface for neuronal photoactivation. Nat. Commun. 2:166. doi: 10.1038/ncomms1164
Ghezzi, D., Antognazza, M. R., Maccarone, R., Bellani, S., Lanzarini, E., Martino, N., et al. (2013). A polymer optoelectronic interface restores light sensitivity in blind rat retinas. Nat. Photonics 7, 400–406. doi: 10.1038/nphoton.2013.34
Gremmels, H., de Jong, O. G., Hazenbrink, D. H., Fledderus, J. O., and Verhaar, M. C. (2017). The transcription factor Nrf2 protects angiogenic capacity of endothelial colony-forming cells in high-oxygen radical stress conditions. Stem Cells Int. 2017, 1–11. doi: 10.1155/2017/4680612
Hagen, M. W., and Hinds, M. T. (2019). Static spatial growth restriction micropatterning of endothelial colony forming cells influences their morphology and gene expression. PLoS One 14:e0218197. doi: 10.1371/journal.pone.0218197
Hagen, M., and Hinds, M. T. (2020). The effects of topographic micropatterning on endothelial colony forming cells. Tissue Eng. Part A. doi: 10.1089/ten.TEA.2020.0066 [Epub ahead of print]
He, M., Ma, S., Cai, Q., Wu, Y., Shao, C., Kong, H., et al. (2018). Hypoxia induces the dysfunction of human endothelial colony-forming cells via HIF-1α signaling. Respir. Physiol. Neurobiol. 247, 87–95. doi: 10.1016/j.resp.2017.09.013
Heinke, J., Patterson, C., and Moser, M. (2012). Life is a pattern: vascular assembly within the embryo. Front. Biosci. 30, 2269–2288. doi: 10.2741/541
Hofmann, N. A., Barth, S., Waldeck-Weiermair, M., Klec, C., Strunk, D., Malli, R., et al. (2014). TRPV1 mediates cellular uptake of anandamide and thus promotes endothelial cell proliferation and network-formation. Biol. Open 3, 1164–1172. doi: 10.1242/bio.20149571
Inoue, K., and Xiong, Z. -G. (2009). Silencing TRPM7 promotes growth/proliferation and nitric oxide production of vascular endothelial cells via the ERK pathway. Cardiovasc. Res. 83, 547–557. doi: 10.1093/cvr/cvp153
Jarajapu, Y. P. R., Hazra, S., Segal, M., LiCalzi, S., Jhadao, C., Qian, K., et al. (2014). Vasoreparative dysfunction of CD34+ cells in diabetic individuals involves hypoxic desensitization and impaired autocrine/paracrine mechanisms. PLoS One 9:e93965. doi: 10.1371/journal.pone.0093965
Jeong, G. -J., Oh, J. Y., Kim, Y. -J., Bhang, S. H., Jang, H. -K., Han, J., et al. (2017). Therapeutic angiogenesis via solar cell-facilitated electrical stimulation. ACS Appl. Mater. Interfaces 9, 38344–38355. doi: 10.1021/acsami.7b13322
Keighron, C., Lyons, C. J., Creane, M., O’Brien, T., and Liew, A. (2018). Recent advances in endothelial progenitor cells toward their use in clinical translation. Front. Med. 5:354. doi: 10.3389/fmed.2018.00354
Knollmann, B. C. (2010). Pacing lightly: optogenetics gets to the heart. Nat. Methods 7, 889–891. doi: 10.1038/nmeth1110-889
Komici, K., Faris, P., Negri, S., Rosti, V., García-Carrasco, M., Mendoza-Pinto, C., et al. (2020). Systemic lupus erythematosus, endothelial progenitor cells and intracellular Ca2+ signaling: a novel approach for an old disease. J. Autoimmun. 112:102486. doi: 10.1016/j.jaut.2020.102486
Lodola, F., Laforenza, U., Bonetti, E., Lim, D., Dragoni, S., Bottino, C., et al. (2012). Store-operated Ca2+ entry is remodelled and controls in vitro angiogenesis in endothelial progenitor cells isolated from tumoral patients. PLoS One 7:e42541. doi: 10.1371/journal.pone.0042541
Lodola, F., Laforenza, U., Cattaneo, F., Ruffinatti, F. A., Poletto, V., Massa, M., et al. (2017a). VEGF-induced intracellular Ca2+ oscillations are down-regulated and do not stimulate angiogenesis in breast cancer-derived endothelial colony forming cells. Oncotarget 8, 95223–95246. doi: 10.18632/oncotarget.20255
Lodola, F., Martino, N., Tullii, G., Lanzani, G., and Antognazza, M. R. (2017b). Conjugated polymers mediate effective activation of the mammalian ion channel transient receptor potential Vanilloid 1. Sci. Rep. 7:8477. doi: 10.1038/s41598-017-08541-6
Lodola, F., Rosti, V., Tullii, G., Desii, A., Tapella, L., Catarsi, P., et al. (2019a). Conjugated polymers optically regulate the fate of endothelial colony-forming cells. Sci. Adv. 12:eaav4620. doi: 10.1126/sciadv.aav4620
Lodola, F., Vurro, V., Crasto, S., Di Pasquale, E., and Lanzani, G. (2019b). Optical pacing of human-induced pluripotent stem cell-derived cardiomyocytes mediated by a conjugated polymer Interface. Adv. Healthc. Mater. 8:1900198. doi: 10.1002/adhm.201900198
Martino, N., Feyen, P., Porro, M., Bossio, C., Zucchetti, E., Ghezzi, D., et al. (2015). Photothermal cellular stimulation in functional bio-polymer interfaces. Sci. Rep. 5:8911. doi: 10.1038/srep08911
Mauge, L., Sabatier, F., Boutouyrie, P., D’Audigier, C., Peyrard, S., Bozec, E., et al. (2014). Forearm ischemia decreases endothelial colony-forming cell angiogenic potential. Cytotherapy 16, 213–224. doi: 10.1016/j.jcyt.2013.09.007
Maya-Vetencourt, J. F., Ghezzi, D., Antognazza, M. R., Colombo, E., Mete, M., Feyen, P., et al. (2017). A fully organic retinal prosthesis restores vision in a rat model of degenerative blindness. Nat. Mater. 16, 681–689. doi: 10.1038/nmat4874
Maya-Vetencourt, J. F., Manfredi, G., Mete, M., Colombo, E., Bramini, M., Di Marco, S., et al. (2020). Subretinally injected semiconducting polymer nanoparticles rescue vision in a rat model of retinal dystrophy. Nat. Nanotechnol. 15, 698–708. doi: 10.1038/s41565-020-0696-3
Medina, R. J., Barber, C. L., Sabatier, F., Dignat-George, F., Melero-Martin, J. M., Khosrotehrani, K., et al. (2017). Endothelial progenitors: a consensus statement on nomenclature: endothelial progenitors nomenclature. Stem Cells Transl. Med. 6, 1316–1320. doi: 10.1002/sctm.16-0360
Mena, H. A., Zubiry, P. R., Dizier, B., Schattner, M., Boisson-Vidal, C., and Negrotto, S. (2018). Acidic preconditioning of endothelial colony-forming cells (ECFC) promote vasculogenesis under proinflammatory and high glucose conditions in vitro and in vivo. Stem Cell Res Ther 9:120. doi: 10.1186/s13287-018-0872-7
Mensah, G. A., Roth, G. A., and Fuster, V. (2019). The global burden of cardiovascular diseases and risk factors. J. Am. Coll. Cardiol. 74, 2529–2532. doi: 10.1016/j.jacc.2019.10.009
Mittal, M., Urao, N., Hecquet, C. M., Zhang, M., Sudhahar, V., Gao, X., et al. (2015). Novel role of reactive oxygen species–activated trp melastatin channel-2 in mediating angiogenesis and postischemic neovascularization. Arterioscler. Thromb. Vasc. Biol. 35, 877–887. doi: 10.1161/ATVBAHA.114.304802
Moccia, F., Baruffi, S., Spaggiari, S., Coltrini, D., Berra-Romani, R., Signorelli, S., et al. (2001). P2Y1 and P2Y2 receptor-operated Ca2+ signals in primary cultures of cardiac microvascular endothelial cells. Microvasc. Res. 61, 240–252. doi: 10.1006/mvre.2001.2306
Moccia, F., Berra-Romani, R., and Rosti, V. (2018a). Manipulating intracellular Ca2+ signals to stimulate therapeutic angiogenesis in cardiovascular disorders. Curr. Pharm. Biotechnol. 19, 686–699. doi: 10.2174/1389201019666180808165309
Moccia, F., Berra-Romani, R., Tritto, S., Signorelli, S., Taglietti, V., and Tanzi, F. (2003). Epidermal growth factor induces intracellular Ca2+ oscillations in microvascular endothelial cells. J. Cell. Physiol. 194, 139–150. doi: 10.1002/jcp.10198
Moccia, F., Bonetti, E., Dragoni, S., Fontana, J., Lodola, F., Berra Romani, R., et al. (2012). Hematopoietic progenitor and stem cells circulate by surfing on intracellular Ca2+ waves: a novel target for cell-based therapy and anti-cancer treatment? Curr. Signal Transduct. Ther. 7, 161–176. doi: 10.2174/157436212800376672
Moccia, F., Fotia, V., Tancredi, R., Porta, M. G. D., Rosti, V., Bonetti, E., et al. (2017). Breast and renal cancer-derived endothelial colony forming cells share a common gene signature. Eur. J. Cancer 77, 155–164. doi: 10.1016/j.ejca.2017.01.025
Moccia, F., Lucariello, A., and Guerra, G. (2018b). TRPC3-mediated Ca2+ signals as a promising strategy to boost therapeutic angiogenesis in failing hearts: the role of autologous endothelial colony forming cells. J. Cell. Physiol. 233, 3901–3917. doi: 10.1002/jcp.26152
Moccia, F., Ruffinatti, F. A., and Zuccolo, E. (2015). Intracellular Ca2+ signals to reconstruct a broken heart: still a theoretical approach? Curr. Drug Targets 16, 793–815. doi: 10.2174/1389450116666141219121723
Moccia, N., Shekha, F., and Guerra, (2019). Endothelial Ca2+ signaling, angiogenesis and vasculogenesis: just what it takes to make a blood vessel. Int. J. Mol. Sci. 20:3962. doi: 10.3390/ijms20163962
Moccia, F., Tanzi, F., and Munaron, L. (2014). Endothelial remodelling and intracellular calcium machinery. Curr. Mol. Med. 14, 457–480. doi: 10.2174/1566524013666131118113410
Moros, M., Lewinska, A., Onorato, G., Antognazza, M. R., Di Maria, F., Blasio, M., et al. (2018). Light-triggered modulation of cell antioxidant defense by polymer semiconducting nanoparticles in a model organism. MRS Commun. 8, 918–925. doi: 10.1557/mrc.2018.104
Negri, S., Faris, P., Berra-Romani, R., Guerra, G., and Moccia, F. (2020a). Endothelial transient receptor potential channels and vascular remodeling: extracellular Ca2 + entry for angiogenesis, arteriogenesis and vasculogenesis. Front. Physiol. 10:1618. doi: 10.3389/fphys.2019.01618
Negri, S., Faris, P., Rosti, V., Antognazza, M. R., Lodola, F., and Moccia, F. (2020b). Endothelial TRPV1 as an emerging molecular target to promote therapeutic angiogenesis. 30.
O’Leary, O. E., Canning, P., Reid, E., Bertelli, P. M., McKeown, S., Brines, M., et al. (2019). The vasoreparative potential of endothelial colony-forming cells in the ischemic retina is enhanced by cibinetide, a non-hematopoietic erythropoietin mimetic. Exp. Eye Res. 182, 144–155. doi: 10.1016/j.exer.2019.03.001
O’Neill, C. L., McLoughlin, K. J., Chambers, S. E. J., Guduric-Fuchs, J., Stitt, A. W., and Medina, R. J. (2018). The vasoreparative potential of endothelial colony forming cells: a journey through pre-clinical studies. Front. Med. 5:273. doi: 10.3389/fmed.2018.00273
Ohayon, D., and Inal, S. (2020). Organic bioelectronics: from functional materials to next-generation devices and power sources. Adv. Mater. 32:2001439. doi: 10.1002/adma.202001439
Paschalaki, K. E., and Randi, A. M. (2018). Recent advances in endothelial colony forming cells toward their use in clinical translation. Front. Med. 5:295. doi: 10.3389/fmed.2018.00295
Potente, M., and Mäkinen, T. (2017). Vascular heterogeneity and specialization in development and disease. Nat. Rev. Mol. Cell Biol. 18, 477–494. doi: 10.1038/nrm.2017.36
Potenza, D. M., Guerra, G., Avanzato, D., Poletto, V., Pareek, S., Guido, D., et al. (2014). Hydrogen sulphide triggers VEGF-induced intracellular Ca2+ signals in human endothelial cells but not in their immature progenitors. Cell Calcium 56, 225–234. doi: 10.1016/j.ceca.2014.07.010
Prasad, M., Corban, M. T., Henry, T. D., Dietz, A. B., Lerman, L. O., and Lerman, A. (2020). Promise of autologous CD34+ stem/progenitor cell therapy for treatment of cardiovascular disease. Cardiovasc. Res. 116, 1424–1433. doi: 10.1093/cvr/cvaa027
Qadura, M., Terenzi, D. C., Verma, S., Al-Omran, M., and Hess, D. A. (2018). Concise review: cell therapy for critical limb ischemia: an integrated review of preclinical and clinical studies: stem cell therapy for critical limb ischemia. Stem Cells 36, 161–171. doi: 10.1002/stem.2751
Rivnay, J., Wang, H., Fenno, L., Deisseroth, K., and Malliaras, G. G. (2017). Next-generation probes, particles, and proteins for neural interfacing. Sci. Adv. 3:e1601649. doi: 10.1126/sciadv.1601649
Savage, A. M., Kurusamy, S., Chen, Y., Jiang, Z., Chhabria, K., MacDonald, R. B., et al. (2019). tmem33 is essential for VEGF-mediated endothelial calcium oscillations and angiogenesis. Nat. Commun. 10:732. doi: 10.1038/s41467-019-08590-7
Schierling, W., Troidl, K., Apfelbeck, H., Troidl, C., Kasprzak, P. M., Schaper, W., et al. (2011). Cerebral arteriogenesis is enhanced by pharmacological as well as fluid-shear-stress activation of the Trpv4 calcium channel. Eur. J. Vasc. Endovasc. Surg. 41, 589–596. doi: 10.1016/j.ejvs.2010.11.034
Shelley, W. C., Leapley, A. C., Huang, L., Critser, P. J., Zeng, P., Prater, D., et al. (2012). Changes in the frequency and in vivo vessel-forming ability of rhesus monkey circulating endothelial colony–forming cells across the lifespan (birth to aged). Pediatr. Res. 71, 156–161. doi: 10.1038/pr.2011.22
Smani, T., Gómez, L. J., Regodon, S., Woodard, G. E., Siegfried, G., Khatib, A. -M., et al. (2018). TRP channels in angiogenesis and other endothelial functions. Front. Physiol. 9:1731. doi: 10.3389/fphys.2018.01731
Su, S. -H., Wu, C. -H., Chiu, Y. -L., Chang, S. -J., Lo, H. -H., Liao, K. -H., et al. (2017). Dysregulation of vascular endothelial growth factor receptor-2 by multiple miRNAs in endothelial colony-forming cells of coronary artery disease. J. Vasc. Res. 54, 22–32. doi: 10.1159/000449202
Sung, S. -H., Wu, T. -C., Chen, J. -S., Chen, Y. -H., Huang, P. -H., Lin, S. -J., et al. (2013). Reduced number and impaired function of circulating endothelial progenitor cells in patients with abdominal aortic aneurysm. Int. J. Cardiol. 168, 1070–1077. doi: 10.1016/j.ijcard.2012.11.002
Tasev, D., Dekker-Vroling, L., van Wijhe, M., Broxterman, H. J., Koolwijk, P., and van Hinsbergh, V. W. M. (2018). Hypoxia impairs initial outgrowth of endothelial colony forming cells and reduces their proliferative and sprouting potential. Front. Med. 5:356. doi: 10.3389/fmed.2018.00356
Tasev, D., Koolwijk, P., and van Hinsbergh, V. W. M. (2016). Therapeutic potential of human-derived endothelial colony-forming cells in animal models. Tissue Eng. Part B Rev. 22, 371–382. doi: 10.1089/ten.teb.2016.0050
Thodeti, C. K., Matthews, B., Ravi, A., Mammoto, A., Ghosh, K., Bracha, A. L., et al. (2009). TRPV4 channels mediate cyclic strain–induced endothelial cell reorientation through integrin-to-integrin signaling. Circ. Res. 104, 1123–1130. doi: 10.1161/CIRCRESAHA.108.192930
Tortiglione, C., Antognazza, M. R., Tino, A., Bossio, C., Marchesano, V., Bauduin, A., et al. (2017). Semiconducting polymers are light nanotransducers in eyeless animals. Sci. Adv. 3:e1601699. doi: 10.1126/sciadv.1601699
Tullii, G., Desii, A., Bossio, C., Bellani, S., Colombo, M., Martino, N., et al. (2017). Bimodal functioning of a mesoporous, light sensitive polymer/electrolyte interface. Org. Electron. 46, 88–98. doi: 10.1016/j.orgel.2017.04.007
Tullii, G., Donini, S., Bossio, C., Lodola, F., Pasini, M., Parisini, E., et al. (2020). Micro- and nanopatterned silk substrates for antifouling applications. ACS Appl. Mater. Interfaces 12, 5437–5446. doi: 10.1021/acsami.9b18187
Udan, R. S., Culver, J. C., and Dickinson, M. E. (2013). Understanding vascular development: understanding vascular development. Wiley Interdiscip. Rev. Dev. Biol. 2, 327–346. doi: 10.1002/wdev.91
Wu, Y., He, M. -Y., Ye, J. -K., Ma, S. -Y., Huang, W., Wei, Y. -Y., et al. (2017). Activation of ATP-sensitive potassium channels facilitates the function of human endothelial colony-forming cells via Ca 2+/Akt/eNOS pathway. J. Cell. Mol. Med. 21, 609–620. doi: 10.1111/jcmm.13006
Yokota, Y., Nakajima, H., Wakayama, Y., Muto, A., Kawakami, K., Fukuhara, S., et al. (2015). Endothelial Ca2+ oscillations reflect VEGFR signaling-regulated angiogenic capacity in vivo. eLife 4:e08817. doi: 10.7554/eLife.08817
Yu, Y. -B., Su, K. -H., Kou, Y. R., Guo, B. -C., Lee, K. -I., Wei, J., et al. (2017). Role of transient receptor potential vanilloid 1 in regulating erythropoietin-induced activation of endothelial nitric oxide synthase. Acta Physiol. 219, 465–477. doi: 10.1111/apha.12723
Zhang, B., Jiang, H., Chen, J., Hu, Q., Yang, S., and Liu, X. (2019). Silica-coated magnetic nanoparticles labeled endothelial progenitor cells alleviate ischemic myocardial injury and improve long-term cardiac function with magnetic field guidance in rats with myocardial infarction. J. Cell. Physiol. 234, 18544–18559. doi: 10.1002/jcp.28492
Zheng, Z., Dong, X., Li, Y., Gao, W., Zhou, Y., Jiang, R., et al. (2017). Electrical stimulation improved cognitive deficits associated with traumatic brain injury in rats. Brain Behav. 7:e00667. doi: 10.1002/brb3.667
Zuccolo, E., Di Buduo, C., Lodola, F., Orecchioni, S., Scarpellino, G., Kheder, D. A., et al. (2018). Stromal cell-derived factor-1α promotes endothelial colony-forming cell migration through the Ca 2+-dependent activation of the extracellular signal-regulated kinase 1/2 and phosphoinositide 3-kinase/AKT pathways. Stem Cells Dev. 27, 23–34. doi: 10.1089/scd.2017.0114
Keywords: cardiovascular disease, therapeutic angiogenesis, endothelial colony forming cells, intracellular Ca2+ signaling, transient receptor potential vanilloid 1, cell fate, optical stimulation, conjugated polymers
Citation: Moccia F, Antognazza MR and Lodola F (2021) Towards Novel Geneless Approaches for Therapeutic Angiogenesis. Front. Physiol. 11:616189. doi: 10.3389/fphys.2020.616189
Received: 11 October 2020; Accepted: 08 December 2020;
Published: 20 January 2021.
Edited by:
Sara Petrillo, University of Turin, ItalyReviewed by:
Andrea Gerbino, National Research Council, ItalyCopyright © 2021 Moccia, Antognazza and Lodola. This is an open-access article distributed under the terms of the Creative Commons Attribution License (CC BY). The use, distribution or reproduction in other forums is permitted, provided the original author(s) and the copyright owner(s) are credited and that the original publication in this journal is cited, in accordance with accepted academic practice. No use, distribution or reproduction is permitted which does not comply with these terms.
*Correspondence: Francesco Lodola, ZnJhbmNlc2NvLmxvZG9sYUB1bmltaWIuaXQ=
†These authors have contributed equally to this work
Disclaimer: All claims expressed in this article are solely those of the authors and do not necessarily represent those of their affiliated organizations, or those of the publisher, the editors and the reviewers. Any product that may be evaluated in this article or claim that may be made by its manufacturer is not guaranteed or endorsed by the publisher.
Research integrity at Frontiers
Learn more about the work of our research integrity team to safeguard the quality of each article we publish.