- Department of Cardiology, Beijing Anzhen Hospital, Capital Medical University, Beijing Institute of Heart Lung and Blood Vessel Disease, Beijing, China
Perivascular adipose tissue (PVAT) has been identified to have significant endocrine and paracrine functions, such as releasing bioactive adipokines, cytokines, and chemokines, rather than a non-physiological structural tissue. Considering the contiguity with the vascular wall, PVAT could play a crucial role in the pathogenic microenvironment of atherosclerosis. Growing clinical evidence has shown an association between PVAT and atherosclerosis. Moreover, based on computed tomography, the fat attenuation index of PVAT was verified as an indication of vulnerable atherosclerotic plaques. Under pathological conditions, such as obesity and diabetes, PVAT shows a proatherogenic phenotype by increasing the release of factors that induce endothelial dysfunction and inflammatory cell infiltration, thus contributing to atherosclerosis. Growing animal and human studies have investigated the mechanism of the above process, which has yet to be fully elucidated. Furthermore, traditional treatments for atherosclerosis have been proven to act on PVAT, and we found several studies focused on novel drugs that target PVAT for the prevention of atherosclerosis. Emerging as an indication, contributor to, and therapeutic target for atherosclerosis, PVAT warrants further investigation.
Introduction
Atherosclerosis is a process in which the formation and buildup of lipid, cells, as well as matrix, and represents a major stage of atheromatous plaque formation. Atherosclerosis can cause myocardial infarction, stroke, and disabling peripheral artery disease, leading to high morbidity and mortality worldwide (Libby et al., 2019). The pathogenesis of atherosclerosis including endothelial dysfunction, inflammatory cells recruitment, vascular smooth muscle cells (VSMCs) proliferation and migration. During the process, cytokines are released by different types of cells and exert multiple effects, such as crosstalk among endothelial cells, vascular smooth muscle cells, inflammatory cells, and adipocytes (Tousoulis et al., 2016). The dynamic microenvironment plays a fundamental role in the pathogenesis of atherosclerosis.
The perivascular adipose tissue (PVAT) has long been considered a non-physiological structural tissue, but in the last decade, an increasing number of studies have identified it to have significant endocrine and paracrine functions, including the release of bioactive adipokines, cytokines, and chemokines (Szasz and Webb, 2012). Given the anatomical proximity between PVAT and the vascular wall, PVAT could play a crucial role in the pathogenic processes of atherosclerosis, mainly toward endothelial dysfunction (Virdis, 2016) and inflammatory cell recruitment (Omar et al., 2014). However, the pathophysiological characteristics of PVAT seem to be distinct in different anatomical locations (Gil-Ortega et al., 2015) and metabolic statuses (Molica et al., 2015; Ferrara et al., 2019), which remains to be clarified. In this review, we discuss the PVAT imaging features from the latest clinical evidence, the proatherogenic and antiatherogenic phenotypes of PVAT, the underlying molecular mechanisms and pathways, and potential therapeutic measures.
Imaging Features of PVAT
Ultrasound
Carotid intima-media thickness (IMT) is an index measure derived from high-resolution carotid ultrasound to assess the burden of atherosclerosis. As a complement, carotid extramedial thickness (EMT) focuses on the adventitial structure of arteries, which is mainly attributed to PVAT (Falk et al., 2009). Haberka et al. (2019) reported that EMT is an independent and strong predictor of significant internal carotid artery stenosis instead of obesity measurements. In addition, the combination of ultrasound indexes related to PVAT and the vascular wall was associated with more complex atherosclerotic coronary artery disease (CAD) in patients with high risk (Haberka et al., 2018). Carotid EMT is a promising index for early assessment of the clinical risk of atherosclerosis (Skilton, 2019). Similarly, intravascular photoacoustic ultrasound detected increased iliac arteries-PVAT in Ossabaw swine with metabolic syndrome, which was verified by histology as early-stage atherosclerotic changes (Kole et al., 2019).
Computed Tomography
CT-based volumetric quantification of PVAT is feasible and highly reproducible (Schlett et al., 2009). Recent evidence has demonstrated that coronary artery-PVAT transforms from the lipid to aqueous phase during vascular inflammation, resulting in increased CT attenuation around the inflamed coronary artery (Antonopoulos et al., 2017). A novel non-invasive biomarker, the fat attenuation index (FAI), was designed to predict vulnerable atherosclerotic plaques and cardiac mortality (Antonopoulos et al., 2017; Antoniades et al., 2019; Lin et al., 2019). Moreover, the FAI was validated in a clinical cohort to show significant reduction around the culprit lesion; by contrast, there was no change around the stable atherosclerotic plaques (Antonopoulos et al., 2017). In CT imaging, we can identify adipose tissue with voxels between -190 and -30HU, and perform quantitative evaluation by calculating FAI or volume of PVAT, which helps the prediction of atherosclerosis.
Magnetic Resonance Imaging
Due to its high cost and time consumption, studies based on MRI are limited and controversial. Randrianarisoa et al. (2018) showed a correlation between brachial artery PVAT and insulin resistance, while aorta PVAT is associated with carotid IMT, indicating that brachial artery PVAT and aorta PVAT may act differently as possible modulators of insulin resistance and subclinical atherosclerosis. However, Alkhalil et al. (2018) demonstrated the dissociation between the spatial distribution of PVAT and arterial wall thickening in the aorta and carotid arteries, which does not support that PVAT promotes atherosclerotic plaque through the paracrine route. The quantitative evaluations were implemented with different special software. Further research focusing on the assessment of PVAT with MRI is warranted.
PVAT and Vascular Calcification
Vascular calcification was found to be associated with PVAT as a surrogate measure of atherosclerosis. The coronary artery segment covered by a myocardial bridge (MB) was isolated from the influence of PVAT. Verhagen et al. (2013) revealed that segments without an MB have a higher calcium score than segments covered with an MB. The association between calcium scores and MBs was influenced by local PVAT thickness (Verhagen et al., 2013). Moreover, women with systemic lupus erythematosus (SLE) were demonstrated to have greater aortic PVAT, which correlated with the calcification of different vascular beds (Shields et al., 2013). The summary of clinical imaging studies of PVAT and atherosclerosis is presented in Table 1. Based on above studies, it can be concluded that the increased volume or thickness of PVAT is associated with atherosclerosis. The enlargement of PVAT may indicates the transition from anti-atherogenic to pro-atherogenic phenotypes, which mediating the progression of atherosclerosis. The imaging features and pathological characteristics of PVAT needs further investigations.
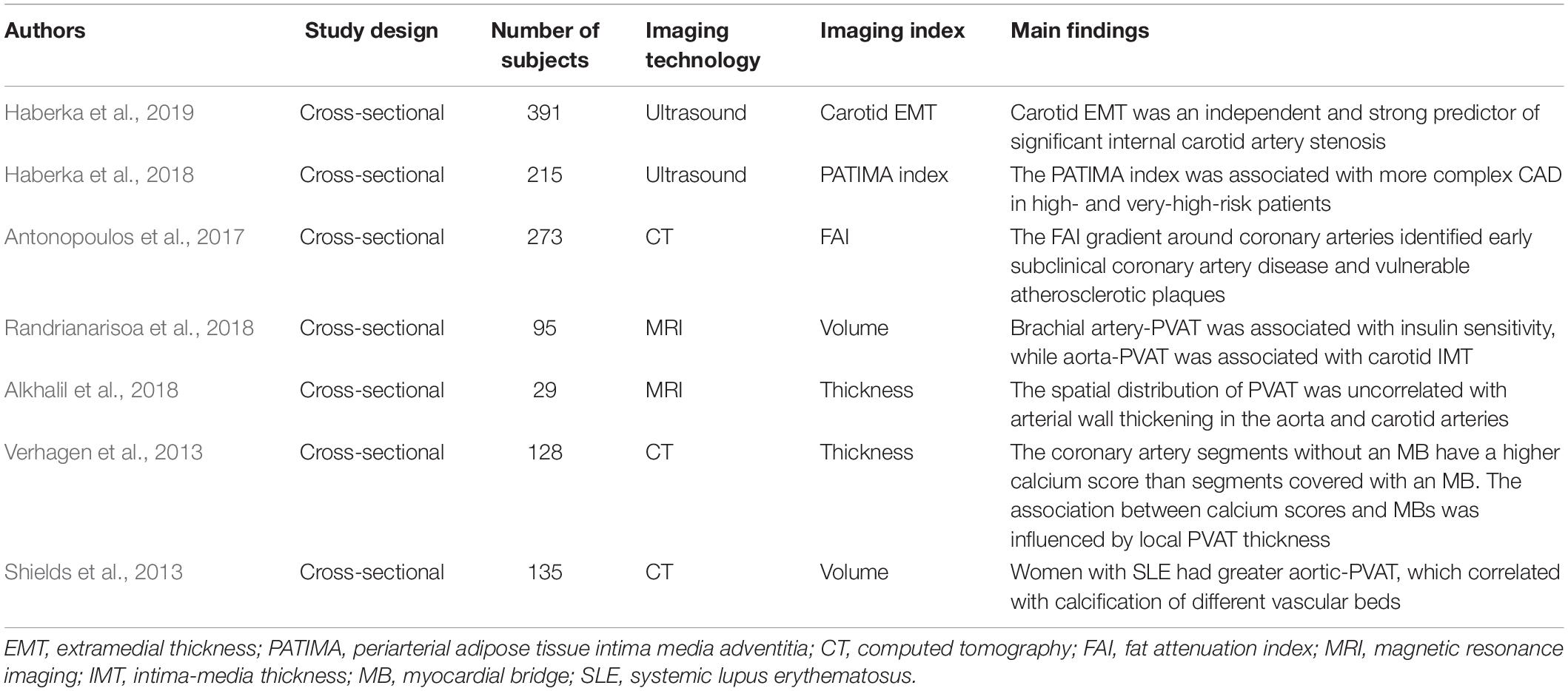
Table 1. Overview of clinical imaging studies of perivascular adipose tissue (PVAT) and atherosclerosis.
Regional and Sex Differences in PVAT
Regional Differences in PVAT
Several rodents and human studies found regional differences in PVAT impacting vascular function. Mouse abdominal aorta-PVAT is more inflamed than thoracic aorta-PVAT, which is independent of aging (Padilla et al., 2013). The phenotypic differences in PVAT between the regions of the aorta may account for the evidence that the abdominal aorta is more vulnerable to atherosclerosis than the thoracic aorta (Padilla et al., 2013; Victorio et al., 2016). A study based on multimodal non-linear optical imaging reported that mouse thoracic PVAT changes differentially from the initial stages to advanced stages of atherosclerosis and undergoes spatial impairment focused on atherosclerotic plaques (Kim et al., 2019). In humans, the phenotype of internal thoracic artery (ITA)-PVAT is closer to that of subcutaneous adipose tissue than that of coronary artery (CA)-PVAT (Numaguchi et al., 2019). ITA-PVAT appears to be protected from inflammation and consecutive adipose tissue remodeling, which may explain the decreased atherosclerotic plaque burden in the ITA (Numaguchi et al., 2019). Similarly, in patients with CAD, the genes related to inflammation, lipid metabolism and myocardial processes are differentially expressed in CA-PVAT and ITA-PVAT, demonstrating that PVAT is the key determinant in the development of atherosclerosis (Lu et al., 2017). In a community-based sample, individuals with high thoracic aorta-PVAT in the absence of high visceral adipose tissue were characterized by adverse cardiometabolic profiles, such as smoking and reduced high-density lipoprotein cholesterol (Britton et al., 2012). Therefore, compared with thoracic aorta and ITA, the vulnerability of abdominal aorta and CA to atherosclerosis may be explained by the regional differences of PVAT.
Sex Differences in PVAT
Abdominal aortic aneurysm is a fatal disease with significant sexual dimorphism. Testosterone was verified to increase aneurysm rupture rates in female, which suggest the aortic vascular biology is regulated by sex chromosome (Alsiraj et al., 2017). Similarly, male is a risk factor of CAD, and estrogen could protect vascular from atherosclerosis. El Khoudary et al. (2015) demonstrated women gain cardiovascular fat after menopause, and the aortic PVAT volume is positively associated with estradiol reduction. Therefore, sex and sex hormones could play an important role in PVAT-mediated vascular atherosclerosis. Thromboxane and PGF2α mediate PVAT-induced vasoconstriction of porcine coronary artery in male and female pigs, respectively (Ahmad et al., 2017b). PVAT from female pigs inhibit contraction of porcine coronary artery, not male pigs (Ahmad et al., 2017a). Sex differences in PVAT have been reviewed in detail recently (Victorio et al., 2020), while the sex differences in PVAT-mediated atherosclerosis remains to be verified.
Inflammation and Immunity of PVAT
Genome-wide expression analyses identified that the genes associated with the regulation of inflammation, angiogenesis, blood clotting, and vascular morphology were differentially expressed in human perivascular adipocytes and subcutaneous adipocytes (Chatterjee et al., 2013). Perivascular adipocytes signal to both inflammatory cells and endothelial cells, thus significantly modulating vascular inflammatory crosstalk in atherogenesis (Chatterjee et al., 2013).
Proinflammatory and Anti-inflammatory Phenotypes of PVAT
Under physiological conditions, PVAT protects the artery against atherosclerosis by counteracting inflammation. Ren et al. (2019) founded that PVAT was formed around the disturbed blood flow-induced carotid atherosclerosis, and transplantation of thoracic PVAT from wild-type mice, which showed lower messenger RNA (mRNA) levels of inflammatory cytokines than thoracic PVAT from ApoE–/– mice, decreased plaque macrophage content. Similarly, wild-type mouse periaortic PVAT transplantation inhibited atherosclerosis development by exerting TGF (transforming growth factor)-β1-mediated anti-inflammatory activity, which might involve M2 macrophages (Terada et al., 2018). Under pathological conditions, PVAT contributes to the formation process of atherosclerosis. Transplantation of abdominal aortic PVAT from high-fat diet (HFD)-fed mice increased aortic atherosclerosis and inhibited endothelium-dependent relaxation (Horimatsu et al., 2018). In the transplanted abdominal aortic PVAT tissue, MCP-1 (monocyte chemoattractant protein-1) and TNF (tumor necrosis factor)-α expression was elevated, while adiponectin expression was reduced (Horimatsu et al., 2018). Moreover, compared with PVAT surrounding a normal vessel (internal mammary artery), human PVAT of atherosclerotic arteries (coronary artery) showed more extensive inflammation, lymphangiogenesis, and fibrosis, probably due to local VEGF (vascular endothelial growth factor)-C, VEGF-D, and angiopoietin-2 overexpression (Drosos et al., 2019).
Similarly, the proinflammatory state of PVAT was identified with cardiovascular risk factors, such as hypertension, diabetes mellitus and peripheral arterial disease. In a hypertensive mouse model, T cells preferentially accumulated in aortic PVAT and could be increased by angiotensin II (Guzik et al., 2007). Accordingly, aortic PVAT-specific renin-angiotensin system activation contributes to accelerating atherosclerotic development in uninephrectomized mice (Kawahito et al., 2013). Angiotensin II type 1 receptor (AT1) plays an important role in the HFD-induced phenotypic alteration of aortic PVAT in ApoE–/– mice, identified as higher expression of proinflammatory cytokines and inflammatory cell infiltration, and modulation of AT1 may exert beneficial effects on atherosclerosis (Irie et al., 2015). In patients with diabetes, carotid PVAT surrounding the atheromatous plaques showed an increase in the mRNA levels of TNF-a, MCP-1, and IL (interleukin)-6 (Hamlat-Khennaf et al., 2017). Patients with peripheral arterial disease showed higher gene expression of TNF-α in renal artery-PVAT (Cejkova et al., 2017). According to Wakana et al. (2015), maternal HFD accelerated atherosclerosis development in offspring, as the increasing thoracic aorta-PVAT specific inflammatory response mediated by higher expression of macrophage colony-stimulating factor. In contrast, an Ossabaw pig experiment exhibited disconnection between left anterior descending coronary artery-PVAT inflammation and HFD-induced cardiometabolic dysfunction (Vieira-Potter et al., 2015).
Inflammatory Cells of PVAT
Human aortic PVAT produces different chemokines, such as IL-8 and MCP-1, which induce the chemotaxis of granulocytes, monocytes, and activated T cells (Henrichot et al., 2005). In patients with CAD, the ratio of macrophages was significantly higher in the coronary arterial wall, and a close relationship was identified between the ratio of macrophages in the arterial wall and PVAT (Verhagen et al., 2012; Kralova Lesna et al., 2015). Coronary PVAT macrophages were associated with stenosis of the adjacent vessel, and M2 macrophages were more abundant than M1 macrophages in PVAT (Verhagen et al., 2014). The inflammatory cells in PVAT seem to play a crucial role in atherogenesis. A postmortem study revealed that the plaque/media ratio increased with the area and macrophages of coronary PVAT (Verhagen et al., 2012). The area of coronary PVAT was related to the presence of a lipid core and the infiltration of macrophages and lymphocytes in atherosclerotic plaques (Verhagen et al., 2012). Similarly, Farias-Itao et al. (2019) identified the correlation between inflammatory cells in coronary PVAT and atherosclerotic plaque features. In periplaque coronary PVAT, the density of CD68+ macrophages and CD20+ B lymphocytes increased with the size of the plaque, and coronary PVAT surrounding unstable atherosclerotic plaques exhibited greater CD68+ macrophages than surrounding stable lesions (Farias-Itao et al., 2019). In addition, in the development of atherogenesis, commensal microbes activate B2 cells in PVAT through lipid metabolism-independent mechanisms (Chen et al., 2016). B1 cells in PVAT were found to produce immunoglobulin type M antibodies to limit atherosclerosis development (Srikakulapu et al., 2017).
PVAT and Immunity
Both rodents and human experiments suggested that PVAT produced complement proteins C3 and C4, which bind to elastin fibers and collagen within the adventitia, leading to increased vascular atherosclerosis and vascular calcification (Shields et al., 2011; Nagaraj et al., 2015). In rats, immunization intracutaneously with native human (nh)-LDL or nh-HDL induced increased PVAT volume and atherosclerosis-like changes in the aortic wall: leukocytes accumulation, intima destruction and media structure disruption (Fomina et al., 2017). Therefore, the immune response toward native lipoproteins might be adipogenic and atherogenic.
Taken together, the inflammation and immunity of PVAT are key determinants in the pathogenesis of atherosclerosis, which deserve further exploration.
Endothelial Function and PVAT-Derived Adipocytokines
Endothelial function is largely based on endothelial nitric oxide synthase (eNOS) function and activity (Daiber et al., 2019). PVAT may regulate arterial tone by releasing diffusible vasorelaxation factors, which, through endothelium-derived NO production, compensate for impaired vasodilatation in metabolic disorders (Kagota et al., 2017; Baltieri et al., 2018). On the other hand, factors released from PVAT may inhibit endothelial NO production and induce vasocontraction by increasing the expression of Cav-1 protein (Lee et al., 2014). AMP-activated protein kinase (AMPK) was revealed to regulate adipocyte metabolism, adipose biology and vascular function (Almabrouk et al., 2014). Activation of AMPK restrains the production of PVAT-released adipokines and prevents endothelial dysfunction by increasing the bioavailability of NO (Gao et al., 2018). Compared with the PVAT of the thoracic aorta, eNOS-derived NO production decreased in the PVAT of the abdominal aorta, suggesting the susceptibility of the abdominal aorta to vascular injury (Victorio et al., 2016). It is now well accepted that adiponectin, as a protective adipocytokine secreted by PVAT, possesses anti-inflammatory, insulin-sensitizing, and vasodilating properties (Milutinovic et al., 2019). In HFD mice, endothelium-mediated vascular relaxation was impaired, and subcutaneously pumped adiponectin was demonstrated to increase eNOS phosphorylation and decrease PVAT inflammation, thereby restoring endothelial cell function (Sena et al., 2017). Consistently, endothelium-dependent relaxation was reduced in obese patients (Virdis, 2016; Cybularz et al., 2017). Endogenous adiponectin expression was increased in PVAT to maintain endothelial function (Cybularz et al., 2017). Moreover, in patients with atherosclerosis, peroxidation products produced in the vascular wall promote adiponectin gene expression in PVAT through a peroxisome proliferator-activated receptor-γ (PPARγ)-dependent mechanism (Margaritis et al., 2013). Then, adiponectin restores eNOS coupling to improve the redox state (Margaritis et al., 2013). In addition, PPARγ deficiency in PVAT enhances atherosclerosis and results in vascular and systemic inflammation (Xiong et al., 2018). Additionally, PVAT-secreted adiponectin promotes macrophage autophagy by suppressing the Akt/FOXO3a signaling pathway, subsequently suppressing plaque formation (Li et al., 2015). Growing studies focus on glucose metabolism of endothelial and VSMCs. Diabetes restrains glycolysis upregulation in endothelial cells under hypoxia conditions (Primer et al., 2020). High concentration of glucose promotes P. gingivalis invasion of human aortic SMCs, then initiates osteogenic phenotype switch, which results in calcification (Chen et al., 2018). Adiponectin also participates in the regulation of related process. High glucose leads to vascular adiponectin resistance, and contributes to diabetic endothelial dysfunction (Liu et al., 2015). High glucose could reduce adiponectin expression of stromal cells in epicardial adipose tissue, which induce an inflammatory paracrine process in endothelial cells (Fernández-Trasancos et al., 2016). Besides, adiponectin could reduce VSMCs proliferation and migration which induced by high glucose (Cersosimo et al., 2020). Leptin was perceived as a risk factor in atherosclerosis progression, which promote endothelial dysfunction, PVAT inflammation and vascular smooth muscle cell phenotypic switching (Dib et al., 2014; Li et al., 2014; Payne et al., 2014). Circulating plasma leptin negatively correlates with endothelial dependent vasodilation (Gonzalez et al., 2013). In Ossabaw with metabolic syndrome, the increased epicardial PVAT leptin was revealed to exacerbate coronary endothelial dysfunction via a protein kinase C-β-dependent pathway (Payne et al., 2010). In female mice, leptin was found to induce endothelial dysfunction through aldosterone-dependent mechanisms (Huby et al., 2016). In addition, patients with coronary artery disease were identified to have more severe hypoxia in local tissue and higher expression of leptin in PVAT, as well as increased inflammation, vascularization, and fibrosis, accounting for the increased atherosclerotic plaque burden within the coronary arteries (Drosos et al., 2016). A study of ewes showed that leptin increased IL-1B and TNF-a gene expression, which may moderate the inflammatory reaction progress in PVAT (Krawczynska et al., 2019).
Perivascular adipose tissue dysfunction is characterized by its inflammatory state, reduced production of vasoprotective adipocyte-derived relaxing factors such as adiponectin and omentin (Van de Voorde et al., 2013) and augmented production of proinflammatory factors such as leptin, resistin, cytokines (TNF-α and IL-6) and chemokines (MCP-1 and RANTES) (Nosalski and Guzik, 2017). These factors promote inflammatory cell infiltration and induce eNOS dysfunction, ultimately leading to the development of atherosclerotic diseases (Van de Voorde et al., 2013; Nosalski and Guzik, 2017; Aroor et al., 2018).
PVAT and Thermoregulation
Perivascular adipose tissue is similar to brown adipose tissue and is essential for intravascular thermoregulation upon cold acclimation, which plays a protective role in the pathogenesis of atherosclerosis (Chang et al., 2012). Proteomics analysis of PVAT from mice identified that cold exposure improves endothelial function and inhibits atherosclerosis (Chang et al., 2012). Moreover, an experiment in ferrets found that cold exposure downregulated gene expression in aortic PVAT, which was associated with the immune response, cell cycle and gene expression regulation (Reynes et al., 2017). Aortic PVAT exhibited an anti-inflammatory response to cold acclimation and demonstrated a protective effect against atherogenesis (Reynes et al., 2017). Different proteins mediated the thermoregulatory response of PVAT. As a mitochondrial outer membrane protein, the CDGSH iron sulfur domain 1 protein (referred to as mitoNEET) could regulate oxidative capacity and the browning of adipose tissue (Xiong et al., 2017). MitoNEET in PVAT contributes to PVAT-dependent thermogenesis, thereby preventing atherosclerosis development (Xiong et al., 2017). In addition, Tang et al. (2018) demonstrated ribosomal protein S3A as a key factor in modulating the brown fat-specific gene uncoupling protein 1 and carbon metabolic enzymes in epicardial adipose tissue to prevent CAD. These findings suggested that the thermogenic capacity of PVAT is beneficial to the protection of atherosclerosis.
Proatherogenesis-Related Molecular Mechanisms and Pathways
With respect to the proatherogenic role of PVAT, different proteins, bioactive factors and pathways have been investigated. Coronary PVAT of Ossabaw swine was identified to attenuate vasodilation through the inhibitions of vascular smooth muscle K + channels (Noblet et al., 2015). Carotid PVAT transplantation leads to endothelial dysfunction and accelerated atherosclerosis in ApoE–/– mice, and it could be blocked by neutralization of Psgl-1 (P-selectin glycoprotein ligand-1) (Ohman et al., 2011). Consistently, Psgl-1 deficiency was revealed to prevent mesenteric PVAT inflammation and endothelial dysfunction in obese mice (Wang et al., 2012). Thus, Psgl-1 may play a crucial role in the formation of atherosclerotic lesions. Moreover, in HFD-fed rabbits, especially in carotid PVAT(+) groups, CRP (C-reactive protein) significantly promoted endothelial dysfunction, which might be mediated by activating the inflammatory response of adipose tissue (Chen et al., 2013). CRP was also found to contribute to vasa vasorum growth by activating the proangiogenic activity of adipose-derived stem cells (Chen et al., 2016), which promote the pathogenesis of atherosclerosis.
Xpr1 and Taf3, a regulator of macrophage differentiation and a core transcription factor, which were detected in mouse PVAT, were significantly upregulated during atherosclerosis, suggesting a role in the pathogenesis of atherosclerosis (Hueso et al., 2016). Interestingly, hepatokine fibroblast growth factor 21 exerts secretome-modulating responses in human perivascular adipocytes, establishing a novel liver-PVAT-blood vessel axis that possibly accounts for vascular inflammation and atherosclerosis (Berti et al., 2016). Moreover, miR-19b in endothelial cell-derived microvesicles promotes atherosclerosis progression by increasing PVAT-specific inflammation by diminishing SOCS3 (suppressor of cytokine signaling 3) expression (Li et al., 2018). In addition, endoplasmic reticulum stress in PVAT destabilizes atherosclerotic plaques by increasing GM-CSF (granulocyte macrophage colony stimulating factor) in a paracrine manner via the transcription factor NF-κB (Ying et al., 2018). In addition, higher levels of inhibiting protein of activated STAT1 (PIAS1) and diminished expression of NF-κB- or STAT1-regulated genes involved in adipocyte inflammation, differentiation, senescence and apoptosis were present in mouse PVAT, while PIAS1 was decreased in the PVAT of patients with atherosclerosis (Schutz et al., 2019), suggesting that STAT1- or NF-κB-regulated genes may mediate the proatherogenesis effects of PVAT. Taken together, PVAT may contribute to atherosclerosis through promoting endothelial dysfunction, accelerating inflammation reaction, and motivating vasa vasorum growth. The underlining molecular mechanism warrants further clarification.
Therapeutic Targets
Smoking Cessation
Nicotine, as the key active component of cigarette smoke, was proven to correlate with PVAT inflammation and contribute to endothelial dysfunction. The PVAT of smokers showed higher expression and activity of the P2 × 7 receptor-inflammasome complex, which contributed to the proinflammatory status (Rossi et al., 2014). Nicotine induced mature adipocyte dysfunction, thus leading to the abnormal secretion of adiponectin and inflammatory adipokines and exacerbating endothelial inflammation (Wang et al., 2016). In addition, adipocytes promote nicotine-induced apoptosis of endothelial cells through the NF-κB pathway (Liu et al., 2017). Smoking cessation could prevent vascular atherosclerosis induced by PVAT dysfunction.
Antidiabetic Drugs
Pioglitazone, an insulin sensitizer, alleviated PVAT oxidative damage induced by fructose treatment and prominently diminished proinflammatory markers in ApoE–/– mice (Quesada et al., 2018). Additionally, pioglitazone dramatically downregulated the expression of vascular cell adhesion molecule-1 (VCAM-1) and matrix metalloprotein-9 (MMP-9), as well as the activity of MMP-9 in the aortic media wall, and markedly decreased the accumulation of macrophages and lipids in atheroma plaques (Quesada et al., 2018). Pioglitazone treatment increased adiponectin serum level (Negrotto et al., 2016), which enhance endothelial mediated vasodilation (Quinn et al., 2010) and increase the number and function of endothelial progenitor cells (Werner et al., 2007), thereby improve vascular dysfunction (Fernandez et al., 2008).
It was reported that glucagon-like peptide-1 (GLP-1)-based therapeutic methods may positively affect autophagy in PVAT, thus improving endothelial dysfunction caused by obesity (Costantino and Paneni, 2019). Both dipeptidyl peptidase-4 (DPP-4) inhibitors and GLP-1 receptor agonists are modulators of this process. In addition, PVAT is a source of DPP-4, and its biology could be influenced by DPP-4 inhibition (Akoumianakis and Antoniades, 2017). DPP-4 inhibition was associated with diminished oxidative stress and local inflammation both in the PVAT and the vascular wall, potentially regulating atherogenesis progression in vivo (Akoumianakis and Antoniades, 2017). For instance, teneligliptin could decrease the expression of a major NADPH oxidase subunit, Nox-4, and a macrophage marker in perivascular adipocytes of normoglycemic ApoE–/– mice (Salim et al., 2017).
Sodium glucose cotransporter 2 (SGLT2) inhibitors were revealed to suppress the inflammation of PVAT and attenuate atherogenesis in an in vivo mouse model. Empagliflozin ameliorated the RNA expression of inflammatory factors in PVAT, attenuated diabetes-induced endothelial dysfunction, and decreased the atherosclerotic lesion area in the aortic arch of diabetic ApoE–/– mice (Ganbaatar et al., 2020). Empagliflozin suppressed the expression of PDGF-B in PVAT macrophages, thereby attenuating neointimal hyperplasia after wire injury in HFD-fed mice (Mori et al., 2019).
Other Drugs
Short-term intensive atorvastatin therapy attenuates PVAT inflammation by inhibiting the 5-lipoxygenase pathway, thus significantly ameliorating endothelial dysfunction in HFD rabbits (Wang et al., 2014). The selective Mas receptor agonist AVE0991 (angiotensin 1-7 mimetic) affected macrophage differentiation and recruitment into the perivascular space, exhibiting anti-inflammatory and antiatherosclerotic actions during the early stages of atherosclerosis in ApoE–/– mice (Skiba et al., 2017).
Morinda citrifolia leaf extract was shown to reduce PVAT deposition while preventing atherosclerosis through lipid elimination and anti-inflammatory reactions in ovariectomized HFD-fed mice (Chong et al., 2018). Similarly, pseudoprotodioscin (PPD), a phytoestrogen isolated from Dioscorea nipponica Makino, alleviated atherosclerotic lesions and exerted estrogenic and anti-inflammatory properties in ovariectomized HFD-fed ApoE–/– mice (Sun et al., 2020).
Polysaccharide peptide from Ganoderma lucidum, as one source of antioxidant, had a significant effect in decreasing H2O2 levels, PVAT thickness, the number of foam cells, and atherosclerotic plaque width in mice (Andri Wihastuti et al., 2015). 10,12 Conjugated linoleic acid (10,12 CLA)-supplemented mice were characterized by enrichment of M2 macrophages in artery lesions and surrounding PVAT, which contributed to the antiatherosclerotic role of 10,12 CLA (Kanter et al., 2018). Dietary ethanolic extract of mangosteen pericarp significantly diminished the expression of VCAM-1 and decreased the thickness of PVAT and IMT in HFD-fed mice (Wihastuti et al., 2019).
Smoking, antidiabetic drugs and other drugs have been verified to act on PVAT, reducing inflammation reaction and endothelial dysfunction, thus preventing atherosclerosis. PVAT is emerging as the therapeutic target of atherosclerosis.
Conclusion
Growing clinical evidence based on imaging technology suggests that the phenotype of PVAT is associated with inflammation and the metabolic profile of the corresponding vasculature as the key determinants for the pathogenesis of atherosclerosis. Under physiological conditions, PVAT acts as an antiatherogenic phenotype by releasing vasodilating factors, exerting an anti-inflammatory response, and thermoregulation. Under pathological conditions, such as obesity and diabetes, PVAT shows the proatherogenic phenotype by increasing the release of adipocytokines and chemokines, which induce endothelial dysfunction, inflammatory cell infiltration, VSMC proliferation and migration, thus contributing to the augmented atherosclerotic plaque burden of the arteries (Figure 1). Improving cardiovascular risk stratification based on PVAT imaging could help identify individuals with unstable lesions. Furthermore, the traditional treatments for atherosclerosis, such as smoking cessation, atorvastatin and antidiabetic drugs, have been proven to act on PVAT. Rapid progress in pharmaceutical research has led to the identification of novel drugs targeting PVAT for the prevention of atherosclerosis. Defining the mechanism of PVAT regulation of vascular homeostasis warrants more and better controlled investigations.
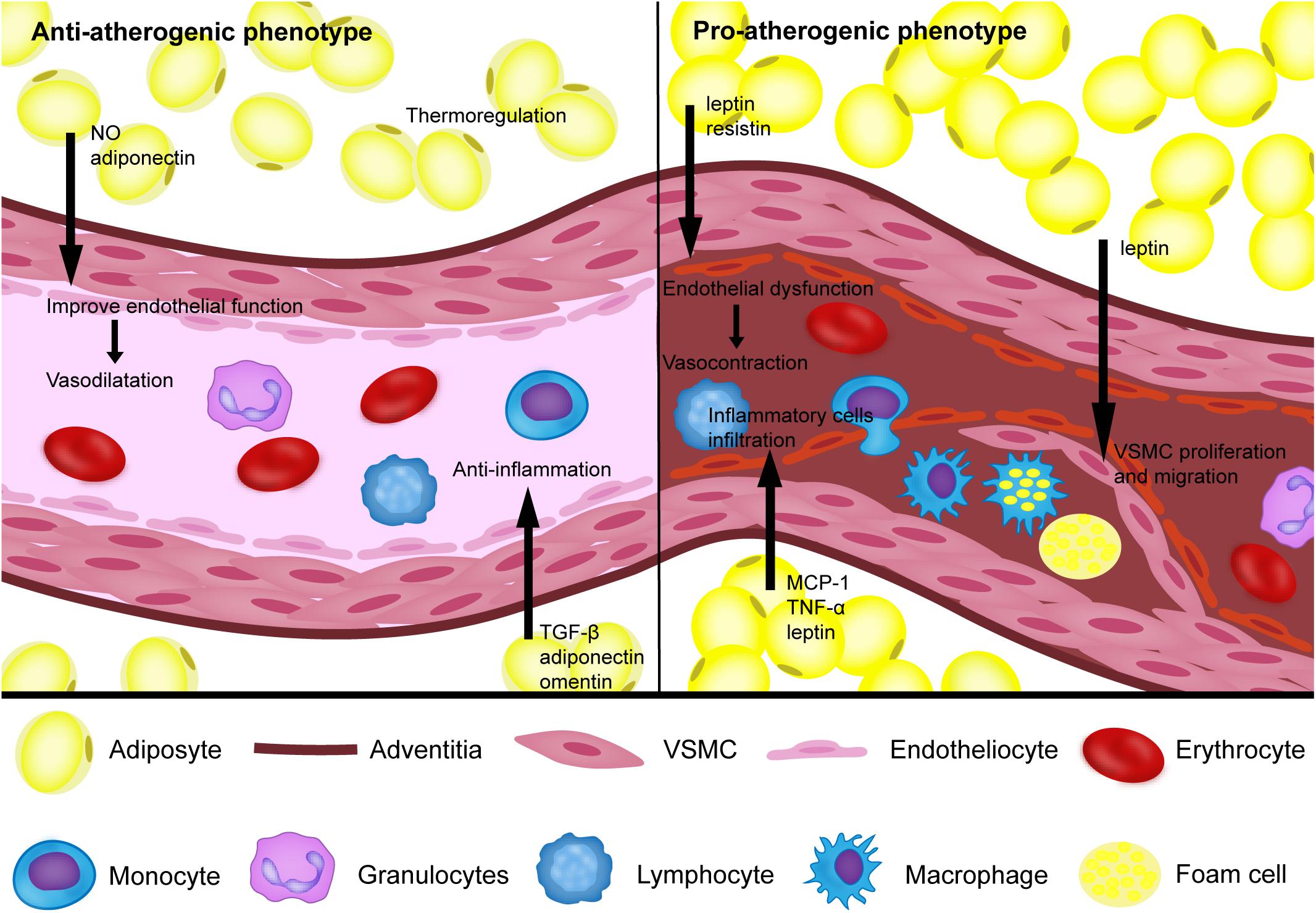
Figure 1. The proatherogenic and antiatherogenic phenotypes of perivascular adipose tissue. Under physiological conditions, perivascular adipose tissue (PVAT) can secrete NO, TGF-β, adiponectin, omentin, and other bioactive factors to reduce the inflammatory response and improve endothelial function, which helps vasodilation. In addition, PVAT also acts as an antiatherogenic phenotype through thermoregulation. Under pathological conditions, such as obesity and diabetes, PVAT shows a proatherogenic phenotype by increasing the release of MCP-1, TNF-a, leptin, resistin and other bioactive factors to induce endothelial dysfunction, inflammatory cell infiltration, vascular smooth cell (VSMC) proliferation and migration.
Author Contributions
YL and YXZ conceived the original scope of this manuscript. YL, YS, CH, JL, and AG wrote specific sections. HH, MC, JZ, YJZ, and YXZ critically reviewed and revised the final manuscript. All authors read and approved the final manuscript.
Funding
This work was supported by grants from the National Key Research and Development Program of China (2017YFC0908800), the Beijing Municipal Administration of Hospitals’ Ascent Plan (DFL20150601) and Mission plan (SML20180601), and the Beijing Municipal Health Commission “Project of Science and Technology Innovation Center” (PXM2019_026272_000006) (PXM2019_026272_000005).
Conflict of Interest
The authors declare that the research was conducted in the absence of any commercial or financial relationships that could be construed as a potential conflict of interest.
Abbreviations
10,12 CLA, 10,12 conjugated linoleic acid; AMPK, AMP-activated protein kinase; AT1, angiotensin II type 1 receptor; CA, coronary artery; CAD, atherosclerotic coronary artery disease; CRP, C-reactive protein; CT, computed tomography; DPP-4, dipeptidyl peptidase-4; EMT, extramedial thickness; eNOS, endothelial nitric oxide synthase; FAI, fat attenuation index; GLP-1, glucagon like peptide-1; GM-CSF, granulocyte macrophage colony stimulating factor; HFD, high-fat diet; IL, interleukin; IMT, intima-media thickness; ITA, internal thoracic artery; MB, myocardial bridge; MCP-1, monocyte chemoattractant protein-1; mitoNEET, CDGSH iron sulfur domain 1 protein; MMP-9, matrix metalloprotein-9; MRI, magnetic resonance imaging; mRNA, messenger RNA; Nh, native human; PIAS1, inhibiting protein of activated STAT1; PPARγ, peroxisome proliferator-activated receptor-γ; PPD, pseudoprotodioscin; Psgl-1, P-selectin glycoprotein ligand-1; PVAT, perivascular adipose tissue; SGLT2, sodium glucose cotransporter 2; SLE, systemic lupus erythematosus; SOCS3, suppressor of cytokine signaling 3; TGF, transforming growth factor; TNF, tumor necrosis factor; VCAM-1, vascular cell adhesion molecule-1; VEGF, vascular endothelial growth factor; VSMCs, vascular smooth muscle cells.
References
Ahmad, A. A., Randall, M. D., and Roberts, R. E. (2017a). Sex differences in the regulation of porcine coronary artery tone by perivascular adipose tissue: a role of adiponectin? Br. J. Pharmacol. 174, 2773–2783. doi: 10.1111/bph.13902
Ahmad, A. A., Randall, M. D., and Roberts, R. E. (2017b). Sex differences in the role of phospholipase A(2) -dependent arachidonic acid pathway in the perivascular adipose tissue function in pigs. J. Physiol. 595, 6623–6634. doi: 10.1113/jp274831
Akoumianakis, I., and Antoniades, C. (2017). Dipeptidyl peptidase IV inhibitors as novel regulators of vascular disease. Vascul. Pharmacol. 9, 1–4. doi: 10.1016/j.vph.2017.07.001
Alkhalil, M., Edmond, E., Edgar, L., Digby, J. E., Omar, O., Robson, M. D., et al. (2018). The relationship of perivascular adipose tissue and atherosclerosis in the aorta and carotid arteries, determined by magnetic resonance imaging. Diab. Vasc. Dis. Res. 15, 286–293. doi: 10.1177/1479164118757923
Almabrouk, T. A., Ewart, M. A., Salt, I. P., and Kennedy, S. (2014). Perivascular fat, AMP-activated protein kinase and vascular diseases. Br. J. Pharmacol. 171, 595–617. doi: 10.1111/bph.12479
Alsiraj, Y., Thatcher, S. E., Charnigo, R., Chen, K., Blalock, E., Daugherty, A., et al. (2017). Female mice with an XY sex chromosome complement develop severe angiotensin II-induced abdominal aortic aneurysms. Circulation 135, 379–391. doi: 10.1161/circulationaha.116.023789
Andri Wihastuti, T., Sargowo, D., Heriansyah, T., Eka Aziza, Y., Puspitarini, D., Nur Iwana, A., et al. (2015). The reduction of aorta histopathological images through inhibition of reactive oxygen species formation in hypercholesterolemia rattus norvegicus treated with polysaccharide peptide of Ganoderma lucidum. Iran. J. Basic Med. Sci. 18, 514–519.
Antoniades, C., Kotanidis, C. P., and Berman, D. S. (2019). State-of-the-art review article. Atherosclerosis affecting fat: what can we learn by imaging perivascular adipose tissue? J. Cardiovasc. Comput. Tomogr. 13, 288–296. doi: 10.1016/j.jcct.2019.03.006
Antonopoulos, A. S., Sanna, F., Sabharwal, N., Thomas, S., and Antoniades, C. (2017). Detecting human coronary inflammation by imaging perivascular fat. Sci. Transl. Med. 9:eaal2658. doi: 10.1126/scitranslmed.aal2658
Aroor, A. R., Jia, G., and Sowers, J. R. (2018). Cellular mechanisms underlying obesity-induced arterial stiffness. Am. J. Physiol. Regul. Integr. Comp. Physiol. 314, R387–R398. doi: 10.1152/ajpregu.00235.2016
Baltieri, N., Guizoni, D. M., Victorio, J. A., and Davel, A. P. (2018). Protective role of perivascular adipose tissue in endothelial dysfunction and insulin-induced vasodilatation of hypercholesterolemic LDL receptor-deficient mice. Front. Physiol. 9:229. doi: 10.3389/fphys.2018.00229
Berti, L., Hartwig, S., Irmler, M., Radle, B., Siegel-Axel, D., Beckers, J., et al. (2016). Impact of fibroblast growth factor 21 on the secretome of human perivascular preadipocytes and adipocytes: a targeted proteomics approach. Arch. Physiol. Biochem. 122, 281–288. doi: 10.1080/13813455.2016.1212898
Britton, K. A., Pedley, A., Massaro, J. M., Corsini, E. M., Murabito, J. M., Hoffmann, U., et al. (2012). Prevalence, distribution, and risk factor correlates of high thoracic periaortic fat in the Framingham Heart Study. J. Am. Heart Assoc. 1:e004200. doi: 10.1161/jaha.112.004200
Cejkova, S., Kralova Lesna, I., Fronek, J., Janousek, L., Kralova, A., Zdychova, J., et al. (2017). Pro-inflammatory gene expression in adipose tissue of patients with atherosclerosis. Physiol. Res. 66, 633–640. doi: 10.33549/physiolres.933352
Cersosimo, E., Xu, X., Terasawa, T., and Dong, L. Q. (2020). Anti-inflammatory and anti-proliferative action of adiponectin mediated by insulin signaling cascade in human vascular smooth muscle cells. Mol. Biol. Rep. 47, 6561–6572. doi: 10.1007/s11033-020-05707-w
Chang, L., Villacorta, L., Li, R., Hamblin, M., Xu, W., Dou, C., et al. (2012). Loss of perivascular adipose tissue on peroxisome proliferator-activated receptor-gamma deletion in smooth muscle cells impairs intravascular thermoregulation and enhances atherosclerosis. Circulation 126, 1067–1078. doi: 10.1161/circulationaha.112.104489
Chatterjee, T. K., Aronow, B. J., Tong, W. S., Manka, D., Tang, Y., Bogdanov, V. Y., et al. (2013). Human coronary artery perivascular adipocytes overexpress genes responsible for regulating vascular morphology, inflammation, and hemostasis. Physiol. Genomics 45, 697–709. doi: 10.1152/physiolgenomics.00042.2013
Chen, J., Gu, Z., Wu, M., Yang, Y., Zhang, J., Ou, J., et al. (2016). C-reactive protein can upregulate VEGF expression to promote ADSC-induced angiogenesis by activating HIF-1alpha via CD64/PI3k/Akt and MAPK/ERK signaling pathways. Stem Cell Res. Ther. 7:114. doi: 10.1186/s13287-016-0377-1
Chen, L., Ishigami, T., Nakashima-Sasaki, R., Kino, T., Doi, H., Minegishi, S., et al. (2016). Commensal microbe-specific activation of B2 cell subsets contributes to atherosclerosis development independently of lipid metabolism. EBioMedicine 13, 237–247. doi: 10.1016/j.ebiom.2016.10.030
Chen, T. C., Lin, C. T., Chien, S. J., Chang, S. F., and Chen, C. N. (2018). Regulation of calcification in human aortic smooth muscle cells infected with high-glucose-treated Porphyromonas gingivalis. J. Cell. Physiol. 233, 4759–4769. doi: 10.1002/jcp.26268
Chen, Y., Wang, X., Mai, J., Zhao, X., Liang, Y., Gu, M., et al. (2013). C-reactive protein promotes vascular endothelial dysfunction partly via activating adipose tissue inflammation in hyperlipidemic rabbits. Int. J. Cardiol. 168, 2397–2403. doi: 10.1016/j.ijcard.2013.01.158
Chong, C. L. G., Othman, F., and Hussan, F. (2018). Vascular protective effects of Morinda citrifolia leaf extract on postmenopausal rats fed with thermoxidized palm oil diet: evidence at microscopic level. Int. J. Vasc. Med. 2018:6317434. doi: 10.1155/2018/6317434
Costantino, S., and Paneni, F. (2019). GLP-1-based therapies to boost autophagy in cardiometabolic patients: from experimental evidence to clinical trials. Vascul. Pharmacol. 115, 64–68. doi: 10.1016/j.vph.2019.03.003
Cybularz, M., Langbein, H., Zatschler, B., Brunssen, C., Deussen, A., Matschke, K., et al. (2017). Endothelial function and gene expression in perivascular adipose tissue from internal mammary arteries of obese patients with coronary artery disease. Atheroscler. Suppl. 30, 149–158. doi: 10.1016/j.atherosclerosissup.2017.05.042
Daiber, A., Xia, N., Steven, S., Oelze, M., Hanf, A., Kroller-Schon, S., et al. (2019). New therapeutic implications of endothelial nitric oxide synthase (eNOS) function/dysfunction in cardiovascular disease. Int. J. Mol. Sci. 20:187. doi: 10.3390/ijms20010187
Dib, L. H., Ortega, M. T., Fleming, S. D., Chapes, S. K., and Melgarejo, T. (2014). Bone marrow leptin signaling mediates obesity-associated adipose tissue inflammation in male mice. Endocrinology 155, 40–46. doi: 10.1210/en.2013-1607
Drosos, I., Chalikias, G., Pavlaki, M., Kareli, D., Epitropou, G., Bougioukas, G., et al. (2016). Differences between perivascular adipose tissue surrounding the heart and the internal mammary artery: possible role for the leptin-inflammation-fibrosis-hypoxia axis. Clin. Res. Cardiol. 105, 887–900. doi: 10.1007/s00392-016-0996-7
Drosos, I., Pavlaki, M., Ortega Carrillo, M. D. P., Kourkouli, A., Buschmann, K., Konstantinou, F., et al. (2019). Increased lymphangiogenesis and lymphangiogenic growth factor expression in perivascular adipose tissue of patients with coronary artery disease. J. Clin. Med. 8:1000. doi: 10.3390/jcm8071000
El Khoudary, S. R., Shields, K. J., Janssen, I., Hanley, C., Budoff, M. J., Barinas-Mitchell, E., et al. (2015). Cardiovascular fat, menopause, and sex hormones in women: the SWAN cardiovascular fat ancillary study. J. Clin. Endocrinol. Metab. 100, 3304–3312. doi: 10.1210/jc.2015-2110
Falk, E., Thim, T., and Kristensen, I. B. (2009). Atherosclerotic plaque, adventitia, perivascular fat, and carotid imaging. JACC Cardiovasc. Imaging 2, 183–186. doi: 10.1016/j.jcmg.2008.11.005
Farias-Itao, D. S., Pasqualucci, C. A., Nishizawa, A., da Silva, L. F. F., Campos, F. M., Bittencourt, M. S., et al. (2019). B lymphocytes and macrophages in the perivascular adipose tissue are associated with coronary atherosclerosis: an autopsy study. J. Am. Heart Assoc. 8:e013793. doi: 10.1161/jaha.119.013793
Fernandez, M., Triplitt, C., Wajcberg, E., Sriwijilkamol, A. A., Musi, N., Cusi, K., et al. (2008). Addition of pioglitazone and ramipril to intensive insulin therapy in type 2 diabetic patients improves vascular dysfunction by different mechanisms. Diabetes Care 31, 121–127. doi: 10.2337/dc07-0711
Fernández-Trasancos, Á, Guerola-Segura, R., Paradela-Dobarro, B., Álvarez, E., García-Acuña, J. M., Fernández, ÁL., et al. (2016). Glucose and inflammatory cells decrease adiponectin in epicardial adipose tissue cells: paracrine consequences on vascular endothelium. J. Cell. Physiol. 231, 1015–1023. doi: 10.1002/jcp.25189
Ferrara, D., Montecucco, F., Dallegri, F., and Carbone, F. (2019). Impact of different ectopic fat depots on cardiovascular and metabolic diseases. J. Cell. Physiol. 234, 21630–21641. doi: 10.1002/jcp.28821
Fomina, K., Beduleva, L., Menshikov, I., Anikaeva, M. M., Suntsova, D., Sidorov, A., et al. (2017). Immune response to native lipoproteins induces visceral obesity and aortic wall injury in rats: the role of testosterone. Endocr. Metab. Immune Disord. Drug Targets 17, 125–133. doi: 10.2174/1871530317666170711154825
Ganbaatar, B., Fukuda, D., Shinohara, M., Yagi, S., Kusunose, K., Yamada, H., et al. (2020). Empagliflozin ameliorates endothelial dysfunction and suppresses atherogenesis in diabetic apolipoprotein E-deficient mice. Eur. J. Pharmacol. 875:173040. doi: 10.1016/j.ejphar.2020.173040
Gao, F., Chen, J., and Zhu, H. (2018). A potential strategy for treating atherosclerosis: improving endothelial function via AMP-activated protein kinase. Sci. China Life Sci. 61, 1024–1029. doi: 10.1007/s11427-017-9285-1
Gil-Ortega, M., Somoza, B., Huang, Y., Gollasch, M., and Fernandez-Alfonso, M. S. (2015). Regional differences in perivascular adipose tissue impacting vascular homeostasis. Trends Endocrinol. Metab. 26, 367–375. doi: 10.1016/j.tem.2015.04.003
Gonzalez, M., Lind, L., and Söderberg, S. (2013). Leptin and endothelial function in the elderly: the prospective investigation of the vasculature in uppsala seniors (PIVUS) study. Atherosclerosis 228, 485–490. doi: 10.1016/j.atherosclerosis.2013.03.018
Guzik, T. J., Hoch, N. E., Brown, K. A., McCann, L. A., Rahman, A., Dikalov, S., et al. (2007). Role of the T cell in the genesis of angiotensin II induced hypertension and vascular dysfunction. J. Exp. Med. 204, 2449–2460. doi: 10.1084/jem.20070657
Haberka, M., Lelek, M., Bochenek, T., Kowalowka, A., Mlynarski, R., Mizia-Stec, K., et al. (2018). Novel combined index of cardiometabolic risk related to periarterial fat improves the clinical prediction for coronary artery disease complexity. Atherosclerosis 268, 76–83. doi: 10.1016/j.atherosclerosis.2017.09.015
Haberka, M., Skilton, M., Biedron, M., Szostak-Janiak, K., Partyka, M., Matla, M., et al. (2019). Obesity, visceral adiposity and carotid atherosclerosis. J. Diabetes Complications 33, 302–306. doi: 10.1016/j.jdiacomp.2019.01.002
Hamlat-Khennaf, N., Neggazi, S., Ayari, H., Feugier, P., Bricca, G., Aouichat-Bouguerra, S., et al. (2017). Inflammation in the perivascular adipose tissue and atherosclerosis. C. R. Biol. 340, 156–163. doi: 10.1016/j.crvi.2017.01.001
Henrichot, E., Juge-Aubry, C. E., Pernin, A., Pache, J. C., Velebit, V., Dayer, J. M., et al. (2005). Production of chemokines by perivascular adipose tissue: a role in the pathogenesis of atherosclerosis? Arterioscler. Thromb. Vasc. Biol. 25, 2594–2599. doi: 10.1161/01.atv.0000188508.40052.35
Horimatsu, T., Patel, A. S., Prasad, R., Reid, L. E., Benson, T. W., Zarzour, A., et al. (2018). Remote effects of transplanted perivascular adipose tissue on endothelial function and atherosclerosis. Cardiovasc. Drugs Ther. 32, 503–510. doi: 10.1007/s10557-018-6821-y
Huby, A. C., Otvos, L. Jr., and Belin de Chantemèle, E. J. (2016). Leptin induces hypertension and endothelial dysfunction via aldosterone-dependent mechanisms in obese female mice. Hypertension 67, 1020–1028. doi: 10.1161/hypertensionaha.115.06642
Hueso, M., De Ramon, L., Navarro, E., Ripoll, E., Cruzado, J. M., Grinyo, J. M., et al. (2016). Silencing of CD40 in vivo reduces progression of experimental atherogenesis through an NF-kappaB/miR-125b axis and reveals new potential mediators in the pathogenesis of atherosclerosis. Atherosclerosis 255, 80–89. doi: 10.1016/j.atherosclerosis.2016.11.002
Irie, D., Kawahito, H., Wakana, N., Kato, T., Kishida, S., Kikai, M., et al. (2015). Transplantation of periaortic adipose tissue from angiotensin receptor blocker-treated mice markedly ameliorates atherosclerosis development in apoE-/- mice. J. Renin Angiotensin Aldosterone Syst. 16, 67–78. doi: 10.1177/1470320314552434
Kagota, S., Iwata, S., Maruyama, K., McGuire, J. J., and Shinozuka, K. (2017). Time-dependent differences in the influence of perivascular adipose tissue on vasomotor functions in metabolic syndrome. Metab. Syndr. Relat. Disord. 15, 233–239. doi: 10.1089/met.2016.0146
Kanter, J. E., Goodspeed, L., Wang, S., Kramer, F., Wietecha, T., Gomes-Kjerulf, D., et al. (2018). 10,12 conjugated linoleic acid-driven weight loss is protective against atherosclerosis in mice and is associated with alternative macrophage enrichment in perivascular adipose tissue. Nutrients 10:1416. doi: 10.3390/nu10101416
Kawahito, H., Yamada, H., Irie, D., Kato, T., Akakabe, Y., Kishida, S., et al. (2013). Periaortic adipose tissue-specific activation of the renin-angiotensin system contributes to atherosclerosis development in uninephrectomized apoE-/- mice. Am. J. Physiol. Heart Circ. Physiol. 305, H667–H675. doi: 10.1152/ajpheart.00053.2013
Kim, S., Lee, E. S., Lee, S. W., Kim, Y. H., Lee, C. H., Jo, D. G., et al. (2019). Site-specific impairment of perivascular adipose tissue on advanced atherosclerotic plaques using multimodal nonlinear optical imaging. Proc. Natl. Acad. Sci. U.S.A. 116, 17765–17774. doi: 10.1073/pnas.1902007116
Kole, A., Cao, Y., Hui, J., Bolad, I. A., Alloosh, M., Cheng, J. X., et al. (2019). Comparative quantification of arterial lipid by intravascular photoacoustic-ultrasound imaging and near-infrared spectroscopy-intravascular ultrasound. J. Cardiovasc. Transl. Res. 12, 211–220. doi: 10.1007/s12265-018-9849-2
Kralova Lesna, I., Tonar, Z., Malek, I., Maluskova, J., Nedorost, L., Pirk, J., et al. (2015). Is the amount of coronary perivascular fat related to atherosclerosis? Physiol. Res. 64(Suppl. 3), S435–S443.
Krawczynska, A., Herman, A. P., Antushevich, H., Bochenek, J., Wojtulewicz, K., and Zieba, D. A. (2019). The influence of photoperiod on the action of exogenous leptin on gene expression of proinflammatory cytokines and their receptors in the thoracic perivascular adipose tissue (PVAT) in ewes. Mediators Inflamm. 2019:7129476. doi: 10.1155/2019/7129476
Lee, M. H., Chen, S. J., Tsao, C. M., and Wu, C. C. (2014). Perivascular adipose tissue inhibits endothelial function of rat aortas via caveolin-1. PLoS One 9:e99947. doi: 10.1371/journal.pone.0099947
Li, C., Li, S., Zhang, F., Wu, M., Liang, H., Song, J., et al. (2018). Endothelial microparticles-mediated transfer of microRNA-19b promotes atherosclerosis via activating perivascular adipose tissue inflammation in apoE(-/-) mice. Biochem. Biophys. Res. Commun. 495, 1922–1929. doi: 10.1016/j.bbrc.2017.11.195
Li, C., Wang, Z., Wang, C., Ma, Q., and Zhao, Y. (2015). Perivascular adipose tissue-derived adiponectin inhibits collar-induced carotid atherosclerosis by promoting macrophage autophagy. PLoS One 10:e0124031. doi: 10.1371/journal.pone.0124031
Li, H., Wang, Y. P., Zhang, L. N., and Tian, G. (2014). Perivascular adipose tissue-derived leptin promotes vascular smooth muscle cell phenotypic switching via p38 mitogen-activated protein kinase in metabolic syndrome rats. Exp. Biol. Med. (Maywood) 239, 954–965. doi: 10.1177/1535370214527903
Libby, P., Buring, J. E., Badimon, L., Hansson, G. K., Deanfield, J., Bittencourt, M. S., et al. (2019). Atherosclerosis. Nat. Rev. Dis. Primers 5:56. doi: 10.1038/s41572-019-0106-z
Lin, A., Dey, D., Wong, D. T. L., and Nerlekar, N. (2019). Perivascular adipose tissue and coronary atherosclerosis: from biology to Imaging phenotyping. Curr. Atheroscler. Rep. 21:47. doi: 10.1007/s11883-019-0817-3
Liu, G. Z., Liang, B., Lau, W. B., Wang, Y., Zhao, J., Li, R., et al. (2015). High glucose/high lipids impair vascular adiponectin function via inhibition of caveolin-1/AdipoR1 signalsome formation. Free Radic. Biol. Med. 89, 473–485. doi: 10.1016/j.freeradbiomed.2015.09.005
Liu, X., Wang, C. N., Qiu, C. Y., Song, W., Wang, L. F., and Liu, B. (2017). Adipocytes promote nicotine-induced injury of endothelial cells via the NF-kappaB pathway. Exp. Cell. Res. 359, 251–256. doi: 10.1016/j.yexcr.2017.07.022
Lu, D., Wang, W., Xia, L., Xia, P., and Yan, Y. (2017). Gene expression profiling reveals heterogeneity of perivascular adipose tissues surrounding coronary and internal thoracic arteries. Acta Biochim. Biophys. Sin. (Shanghai) 49, 1075–1082. doi: 10.1093/abbs/gmx113
Margaritis, M., Antonopoulos, A. S., Digby, J., Lee, R., Reilly, S., Coutinho, P., et al. (2013). Interactions between vascular wall and perivascular adipose tissue reveal novel roles for adiponectin in the regulation of endothelial nitric oxide synthase function in human vessels. Circulation 127, 2209–2221. doi: 10.1161/circulationaha.112.001133
Milutinovic, A., Suput, D., and Zorc-Pleskovic, R. (2019). Pathogenesis of atherosclerosis in the tunica intima, media, and adventitia of coronary arteries: an updated review. Bosn. J. Basic Med. Sci. 20, 21–30. doi: 10.17305/bjbms.2019.4320
Molica, F., Morel, S., Kwak, B. R., Rohner-Jeanrenaud, F., and Steffens, S. (2015). Adipokines at the crossroad between obesity and cardiovascular disease. Thromb. Haemost. 113, 553–566. doi: 10.1160/th14-06-0513
Mori, Y., Terasaki, M., Hiromura, M., Saito, T., Kushima, H., Koshibu, M., et al. (2019). Luseogliflozin attenuates neointimal hyperplasia after wire injury in high-fat diet-fed mice via inhibition of perivascular adipose tissue remodeling. Cardiovasc. Diabetol. 18:143. doi: 10.1186/s12933-019-0947-5
Nagaraj, N., Matthews, K. A., Shields, K. J., Barinas-Mitchell, E., Budoff, M. J., and El Khoudary, S. R. (2015). Complement proteins and arterial calcification in middle aged women: cross-sectional effect of cardiovascular fat. The SWAN cardiovascular fat ancillary study. Atherosclerosis 243, 533–539. doi: 10.1016/j.atherosclerosis.2015.10.095
Negrotto, L., Farez, M. F., and Correale, J. (2016). Immunologic effects of metformin and pioglitazone treatment on metabolic syndrome and multiple sclerosis. JAMA Neurol. 73, 520–528. doi: 10.1001/jamaneurol.2015.4807
Noblet, J. N., Owen, M. K., Goodwill, A. G., Sassoon, D. J., and Tune, J. D. (2015). Lean and obese coronary perivascular adipose tissue impairs vasodilation via differential inhibition of vascular smooth muscle K+ channels. Arterioscler. Thromb. Vasc. Biol. 35, 1393–1400. doi: 10.1161/atvbaha.115.305500
Nosalski, R., and Guzik, T. J. (2017). Perivascular adipose tissue inflammation in vascular disease. Br. J. Pharmacol. 174, 3496–3513. doi: 10.1111/bph.13705
Numaguchi, R., Furuhashi, M., Matsumoto, M., Sato, H., Yanase, Y., Kuroda, Y., et al. (2019). Differential phenotypes in perivascular adipose tissue surrounding the internal thoracic artery and diseased coronary artery. J. Am. Heart Assoc. 8:e011147. doi: 10.1161/jaha.118.011147
Ohman, M. K., Luo, W., Wang, H., Guo, C., Abdallah, W., Russo, H. M., et al. (2011). Perivascular visceral adipose tissue induces atherosclerosis in apolipoprotein E deficient mice. Atherosclerosis 219, 33–39. doi: 10.1016/j.atherosclerosis.2011.07.012
Omar, A., Chatterjee, T. K., Tang, Y., Hui, D. Y., and Weintraub, N. L. (2014). Proinflammatory phenotype of perivascular adipocytes. Arterioscler. Thromb. Vasc. Biol. 34, 1631–1636. doi: 10.1161/atvbaha.114.303030
Padilla, J., Jenkins, N. T., Vieira-Potter, V. J., and Laughlin, M. H. (2013). Divergent phenotype of rat thoracic and abdominal perivascular adipose tissues. Am. J. Physiol. Regul. Integr. Comp. Physiol. 304, R543–R552. doi: 10.1152/ajpregu.00567.2012
Payne, G. A., Borbouse, L., Kumar, S., Neeb, Z., Alloosh, M., Sturek, M., et al. (2010). Epicardial perivascular adipose-derived leptin exacerbates coronary endothelial dysfunction in metabolic syndrome via a protein kinase C-beta pathway. Arterioscler. Thromb. Vasc. Biol. 30, 1711–1717. doi: 10.1161/atvbaha.110.210070
Payne, G. A., Tune, J. D., and Knudson, J. D. (2014). Leptin-induced endothelial dysfunction: a target for therapeutic interventions. Curr. Pharm. Des. 20, 603–608. doi: 10.2174/13816128113199990017
Primer, K. R., Psaltis, P. J., Tan, J. T. M., and Bursill, C. A. (2020). The role of high-density lipoproteins in endothelial cell metabolism and diabetes-impaired angiogenesis. Int. J. Mol. Sci. 21:3633. doi: 10.3390/ijms21103633
Quesada, I., Cejas, J., Garcia, R., Cannizzo, B., Redondo, A., and Castro, C. (2018). Vascular dysfunction elicited by a cross talk between periaortic adipose tissue and the vascular wall is reversed by pioglitazone. Cardiovasc. Ther. 36:e12322. doi: 10.1111/1755-5922.12322
Quinn, C. E., Lockhart, C. J., Hamilton, P. K., Loughrey, C. M., and McVeigh, G. E. (2010). Effect of pioglitazone on endothelial function in impaired glucose tolerance. Diabetes Obes. Metab. 12, 709–715. doi: 10.1111/j.1463-1326.2010.01224.x
Randrianarisoa, E., Stefan, N., Fritsche, A., Reis-Damaschk, N., Hieronimus, A., Balletshofer, B., et al. (2018). Periaortic adipose tissue compared with peribrachial adipose tissue mass as markers and possible modulators of cardiometabolic risk. Angiology 69, 854–860. doi: 10.1177/0003319718755581
Ren, L., Wang, L., You, T., Liu, Y., Wu, F., Zhu, L., et al. (2019). Perivascular adipose tissue modulates carotid plaque formation induced by disturbed flow in mice. J. Vasc. Surg. 70, 927–936.e924. doi: 10.1016/j.jvs.2018.09.064
Reynes, B., van Schothorst, E. M., Garcia-Ruiz, E., Keijer, J., Palou, A., and Oliver, P. (2017). Cold exposure down-regulates immune response pathways in ferret aortic perivascular adipose tissue. Thromb. Haemost. 117, 981–991. doi: 10.1160/th16-12-0931
Rossi, C., Santini, E., Chiarugi, M., Salvati, A., Comassi, M., Vitolo, E., et al. (2014). The complex P2X7 receptor/inflammasome in perivascular fat tissue of heavy smokers. Eur. J. Clin. Invest. 44, 295–302. doi: 10.1111/eci.12232
Salim, H. M., Fukuda, D., Higashikuni, Y., Tanaka, K., Hirata, Y., Yagi, S., et al. (2017). Teneligliptin, a dipeptidyl peptidase-4 inhibitor, attenuated pro-inflammatory phenotype of perivascular adipose tissue and inhibited atherogenesis in normoglycemic apolipoprotein-E-deficient mice. Vascul. Pharmacol. 9, 19–25. doi: 10.1016/j.vph.2017.03.003
Schlett, C. L., Massaro, J. M., Lehman, S. J., Bamberg, F., O’Donnell, C. J., Fox, C. S., et al. (2009). Novel measurements of periaortic adipose tissue in comparison to anthropometric measures of obesity, and abdominal adipose tissue. Int. J. Obes. (Lond.) 33, 226–232. doi: 10.1038/ijo.2008.267
Schutz, E., Gogiraju, R., Pavlaki, M., Drosos, I., Georgiadis, G. S., Argyriou, C., et al. (2019). Age-dependent and -independent effects of perivascular adipose tissue and its paracrine activities during neointima formation. Int. J. Mol. Sci. 21:282. doi: 10.3390/ijms21010282
Sena, C. M., Pereira, A., Fernandes, R., Letra, L., and Seica, R. M. (2017). Adiponectin improves endothelial function in mesenteric arteries of rats fed a high-fat diet: role of perivascular adipose tissue. Br. J. Pharmacol. 174, 3514–3526. doi: 10.1111/bph.13756
Shields, K. J., Barinas-Mitchell, E., Gingo, M. R., Tepper, P., Goodpaster, B. H., Kao, A. H., et al. (2013). Perivascular adipose tissue of the descending thoracic aorta is associated with systemic lupus erythematosus and vascular calcification in women. Atherosclerosis 231, 129–135. doi: 10.1016/j.atherosclerosis.2013.09.004
Shields, K. J., Stolz, D., Watkins, S. C., and Ahearn, J. M. (2011). Complement proteins C3 and C4 bind to collagen and elastin in the vascular wall: a potential role in vascular stiffness and atherosclerosis. Clin. Transl. Sci. 4, 146–152. doi: 10.1111/j.1752-8062.2011.00304.x
Skiba, D. S., Nosalski, R., Mikolajczyk, T. P., Siedlinski, M., Rios, F. J., Montezano, A. C., et al. (2017). Anti-atherosclerotic effect of the angiotensin 1-7 mimetic AVE0991 is mediated by inhibition of perivascular and plaque inflammation in early atherosclerosis. Br. J. Pharmacol. 174, 4055–4069. doi: 10.1111/bph.13685
Skilton, M. R. (2019). Revisiting carotid imaging: integrating atherosclerosis, the adventitia, and perivascular adipose tissue. Kardiol. Pol. 77, 1005–1006. doi: 10.33963/kp.15066
Srikakulapu, P., Upadhye, A., Rosenfeld, S. M., Marshall, M. A., McSkimming, C., Hickman, A. W., et al. (2017). Perivascular adipose tissue harbors atheroprotective IgM-producing B cells. Front. Physiol. 8:719. doi: 10.3389/fphys.2017.00719
Sun, B., Yang, D., Yin, Y. Z., and Xiao, J. (2020). Estrogenic and anti-inflammatory effects of pseudoprotodioscin in atherosclerosis-prone mice: insights into endothelial cells and perivascular adipose tissues. Eur. J. Pharmacol. 869:172887. doi: 10.1016/j.ejphar.2019.172887
Szasz, T., and Webb, R. C. (2012). Perivascular adipose tissue: more than just structural support. Clin. Sci. (Lond.) 122, 1–12. doi: 10.1042/cs20110151
Tang, Y., He, Y., Li, C., Mu, W., Zou, Y., Liu, C., et al. (2018). RPS3A positively regulates the mitochondrial function of human periaortic adipose tissue and is associated with coronary artery diseases. Cell Discov. 4:52. doi: 10.1038/s41421-018-0041-2
Terada, K., Yamada, H., Kikai, M., Wakana, N., Yamamoto, K., Wada, N., et al. (2018). Transplantation of periaortic adipose tissue inhibits atherosclerosis in apoE(-/-) mice by evoking TGF-beta1-mediated anti-inflammatory response in transplanted graft. Biochem. Biophys. Res. Commun. 501, 145–151. doi: 10.1016/j.bbrc.2018.04.196
Tousoulis, D., Oikonomou, E., Economou, E. K., Crea, F., and Kaski, J. C. (2016). Inflammatory cytokines in atherosclerosis: current therapeutic approaches. Eur. Heart J. 37, 1723–1732. doi: 10.1093/eurheartj/ehv759
Van de Voorde, J., Pauwels, B., Boydens, C., and Decaluwe, K. (2013). Adipocytokines in relation to cardiovascular disease. Metabolism 62, 1513–1521. doi: 10.1016/j.metabol.2013.06.004
Verhagen, S. N., Buijsrogge, M. P., Vink, A., van Herwerden, L. A., van der Graaf, Y., and Visseren, F. L. (2014). Secretion of adipocytokines by perivascular adipose tissue near stenotic and non-stenotic coronary artery segments in patients undergoing CABG. Atherosclerosis 233, 242–247. doi: 10.1016/j.atherosclerosis.2013.12.005
Verhagen, S. N., Rutten, A., Meijs, M. F., Isgum, I., Cramer, M. J., van der Graaf, Y., et al. (2013). Relationship between myocardial bridges and reduced coronary atherosclerosis in patients with angina pectoris. Int. J. Cardiol. 167, 883–888. doi: 10.1016/j.ijcard.2012.01.091
Verhagen, S. N., Vink, A., van der Graaf, Y., and Visseren, F. L. (2012). Coronary perivascular adipose tissue characteristics are related to atherosclerotic plaque size and composition. A post-mortem study. Atherosclerosis 225, 99–104. doi: 10.1016/j.atherosclerosis.2012.08.031
Victorio, J. A., da Costa, R. M., Tostes, R. C., and Davel, A. P. (2020). Modulation of vascular function by perivascular adipose tissue: sex differences. Curr. Pharm. Des. 26, 3768–3777. doi: 10.2174/1381612826666200701211912
Victorio, J. A., Fontes, M. T., Rossoni, L. V., and Davel, A. P. (2016). Different anti-contractile function and nitric oxide production of thoracic and abdominal perivascular adipose tissues. Front. Physiol. 7:295. doi: 10.3389/fphys.2016.00295
Vieira-Potter, V. J., Lee, S., Bayless, D. S., Scroggins, R. J., Welly, R. J., Fleming, N. J., et al. (2015). Disconnect between adipose tissue inflammation and cardiometabolic dysfunction in Ossabaw pigs. Obesity (Silver Spring) 23, 2421–2429. doi: 10.1002/oby.21252
Virdis, A. (2016). Endothelial dysfunction in obesity: role of inflammation. High Blood Press. Cardiovasc. Prev. 23, 83–85. doi: 10.1007/s40292-016-0133-8
Wakana, N., Irie, D., Kikai, M., Terada, K., Yamamoto, K., Kawahito, H., et al. (2015). Maternal high-fat diet exaggerates atherosclerosis in adult offspring by augmenting periaortic adipose tissue-specific proinflammatory response. Arterioscler. Thromb. Vasc. Biol. 35, 558–569. doi: 10.1161/atvbaha.114.305122
Wang, C. N., Yang, G. H., Wang, Z. Q., Liu, C. W., Li, T. J., Lai, Z. C., et al. (2016). Role of perivascular adipose tissue in nicotineinduced endothelial cell inflammatory responses. Mol. Med. Rep. 14, 5713–5718. doi: 10.3892/mmr.2016.5934
Wang, H., Luo, W., Wang, J., Guo, C., Wang, X., Wolffe, S. L., et al. (2012). Obesity-induced endothelial dysfunction is prevented by deficiency of P-selectin glycoprotein ligand-1. Diabetes 61, 3219–3227. doi: 10.2337/db12-0162
Wang, X., Lin, Y., Luo, N., Chen, Z., Gu, M., Wang, J., et al. (2014). Short-term intensive atorvastatin therapy improves endothelial function partly via attenuating perivascular adipose tissue inflammation through 5-lipoxygenase pathway in hyperlipidemic rabbits. Chin. Med. J. (Engl.) 127, 2953–2959.
Werner, C., Kamani, C. H., Gensch, C., Böhm, M., and Laufs, U. (2007). The peroxisome proliferator-activated receptor-gamma agonist pioglitazone increases number and function of endothelial progenitor cells in patients with coronary artery disease and normal glucose tolerance. Diabetes 56, 2609–2615. doi: 10.2337/db07-0069
Wihastuti, T. A., Aini, F. N., Tjahjono, C. T., and Heriansyah, T. (2019). Dietary ethanolic extract of Mangosteen pericarp reduces VCAM-1, perivascular adipose tissue and aortic intimal medial thickness in hypercholesterolemic rat model. Open Access Maced. J. Med. Sci. 7, 3158–3163. doi: 10.3889/oamjms.2019.717
Xiong, W., Zhao, X., Garcia-Barrio, M. T., Zhang, J., Lin, J., Chen, Y. E., et al. (2017). MitoNEET in perivascular adipose tissue blunts atherosclerosis under mild cold condition in mice. Front. Physiol. 8:1032. doi: 10.3389/fphys.2017.01032
Xiong, W., Zhao, X., Villacorta, L., Rom, O., Garcia-Barrio, M. T., Guo, Y., et al. (2018). Brown adipocyte-specific PPARgamma (peroxisome proliferator-activated receptor gamma) deletion impairs perivascular adipose tissue development and enhances atherosclerosis in mice. Arterioscler. Thromb. Vasc. Biol. 38, 1738–1747. doi: 10.1161/atvbaha.118.311367
Keywords: perivascular adipose tissue, atherosclerosis, inflammation, imaging, adipocytokines
Citation: Liu Y, Sun Y, Hu C, Liu J, Gao A, Han H, Chai M, Zhang J, Zhou Y and Zhao Y (2020) Perivascular Adipose Tissue as an Indication, Contributor to, and Therapeutic Target for Atherosclerosis. Front. Physiol. 11:615503. doi: 10.3389/fphys.2020.615503
Received: 09 October 2020; Accepted: 30 November 2020;
Published: 18 December 2020.
Edited by:
Joshua Thomas Butcher, Oklahoma State University, United StatesReviewed by:
Jessica Faulkner, Augusta University, United StatesEliana Hiromi Akamine, University of São Paulo, Brazil
Ibra Fancher, University of Delaware, United States
Copyright © 2020 Liu, Sun, Hu, Liu, Gao, Han, Chai, Zhang, Zhou and Zhao. This is an open-access article distributed under the terms of the Creative Commons Attribution License (CC BY). The use, distribution or reproduction in other forums is permitted, provided the original author(s) and the copyright owner(s) are credited and that the original publication in this journal is cited, in accordance with accepted academic practice. No use, distribution or reproduction is permitted which does not comply with these terms.
*Correspondence: Yingxin Zhao, enlpbmd4aW5taUAxNjMuY29t