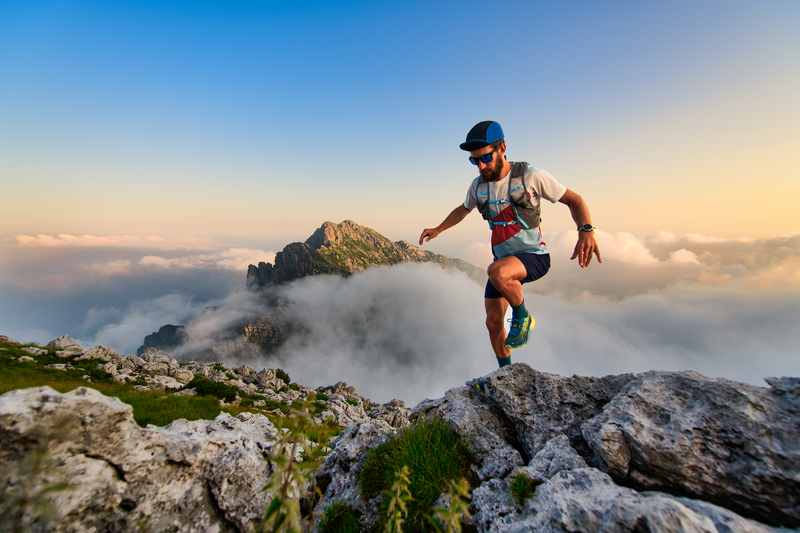
95% of researchers rate our articles as excellent or good
Learn more about the work of our research integrity team to safeguard the quality of each article we publish.
Find out more
BRIEF RESEARCH REPORT article
Front. Physiol. , 10 December 2020
Sec. Vascular Physiology
Volume 11 - 2020 | https://doi.org/10.3389/fphys.2020.611884
This article is part of the Research Topic Involvement of Blood Brain Barrier Efficacy, Neurovascular Coupling and Angiogenesis in the Healthy and Diseased Brain View all 19 articles
Astrocytic Ca2+ fluctuations associated with functional hyperemia have typically been measured from large cellular compartments such as the soma, the whole arbor and the endfoot. The most prominent Ca2+ event is a large magnitude, delayed signal that follows vasodilation. However, previous work has provided little information about the spatio-temporal properties of such Ca2+ transients or their heterogeneity. Here, using an awake, in vivo two-photon fluorescence-imaging model, we performed detailed profiling of delayed astrocytic Ca2+ signals across astrocytes or within individual astrocyte compartments using small regions of interest next to penetrating arterioles and capillaries along with vasomotor responses to vibrissae stimulation. We demonstrated that while a 5-s air puff that stimulates all whiskers predominantly generated reproducible functional hyperemia in the presence or absence of astrocytic Ca2+ changes, whisker stimulation inconsistently produced astrocytic Ca2+ responses. More importantly, these Ca2+ responses were heterogeneous among subcellular structures of the astrocyte and across different astrocytes that resided within the same field of view. Furthermore, we found that whisker stimulation induced discrete Ca2+ “hot spots” that spread regionally within the endfoot. These data reveal that astrocytic Ca2+ dynamics associated with the microvasculature are more complex than previously thought, and highlight the importance of considering the heterogeneity of astrocytic Ca2+ activity to fully understanding neurovascular coupling.
Functional hyperemia is a fundamental control mechanism that provides a rapid local increase in blood flow in response to increased neuronal activity. It is well established that neurotransmission can directly affect the vasculature through innervation (Hamel, 2006; Schummers et al., 2008; Nimmerjahn et al., 2009) or through neuromodulators (Bekar et al., 2008; Takata et al., 2011; Ding et al., 2013; Paukert et al., 2014). These processes are critical in cerebral blood flow (CBF) regulation and serve to ensure that the blood supply matches temporally and spatially changing metabolic demands of neurons. It has been proposed that astrocytes are mediators that relay neuronal information to the vasculature – perhaps on slower timescales – helping to control vessel diameter in addition to neurons and, in turn, regulate blood flow (Zonta et al., 2003; Mulligan and MacVicar, 2004; Straub and Nelson, 2007; Gordon et al., 2008). Our previous study has reported that the delayed astrocytic endfoot Ca2+ signal is mediated by both neurons and vasculature, suggesting a complex interplay between multiple mechanisms that must temporally and spatially coincide to cause a large activation of endfeet. This intriguing finding necessitates further detailed analysis of endfoot Ca2+ dynamics to gain insights into their contributions to functional hyperemia (Tran et al., 2018).
The work performed on ex vivo brain slice preparations has shown that increases in cytosolic Ca2+ concentration [(Ca2+)], produced by uncaging caged Ca2+ compounds (Mulligan and MacVicar, 2004; Straub et al., 2006) or through neuronal stimulation (Simard et al., 2003; Zonta et al., 2003; Gordon et al., 2008), are critical mediators of functional hyperemia, suggesting that activity-dependent vascular changes are facilitated by an astrocyte-mediated Ca2+-dependent process. Some in vivo two-photon imaging studies in anesthetized animals have provided support for this notion, demonstrating rapid astrocytic Ca2+ transients followed by vasodilation (Winship et al., 2007; Lind et al., 2013, 2018) or an increase in red blood cell (RBC) velocity (Otsu et al., 2015) in various regions of the cerebral cortex in response to sensory stimuli. However, other in vivo studies in anesthetized or slightly sedated animals have provided evidence that functional hyperemia can be achieved in the absence of astrocytic Ca2+ increases (Schulz et al., 2012; Takata et al., 2013; Bonder and McCarthy, 2014), or precedes the occasional astrocyte Ca2+ transients (Nizar et al., 2013). In awake, resting animals using astrocyte AAV lck-GCaMP6f tools, whisker stimulation triggered both a fast and slow Ca2+ signals during functional hyperemia (Stobart et al., 2018a). Our own work in awake and active animals using astrocyte Rhod-2 AM, GCaMP3, or GCaMP6s, revealed that whisker stimulation elicited large astrocytic Ca2+ signals that followed rather than preceded vasodilation (Tran et al., 2018). Remarkably, these astrocytic endfoot Ca2+ events were mediated by both glutamatergic transmission and vascular-derived nitric oxide. These data signify that astrocytic Ca2+ dynamics and its contributions to functional hyperemia maybe more complex than previously thought. It has been shown that astrocytic Ca2+ activity is dynamic and heterogeneous (Bindocci et al., 2017; Stobart et al., 2018b). Characterizations of Ca2+ activity in astrocytes have commonly focused on the soma, processes, microdomains or macrodomains (Shigetomi et al., 2013; Srinivasan et al., 2015), but rarely on the astrocytic endfoot. This critical subcellular structure of the neurovascular unit has functional relevance to the astro-vascular relationship. Here, we characterized the cortical astrocyte Ca2+ dynamics, in particular astrocytic endfoot Ca2+, and examined its relationship with functional hyperemia in completely awake mice in vivo using two-photon imaging.
The Animal Care and Use Committee of the University of Calgary approved all the animal procedures. All studies were either performed on male GLAST-Cre ERTx LSL-GCaMP3 mice (Jax#014538) between postnatal day 30 (P30) and P60. Animals were injected on three consecutive days with tamoxifen (100 mg/kg, Sigma), prepared as a 10 mg/ml stock in corn oil. Injections started between P19 and P35. Animals were kept on a normal 12-h light/12-h dark cycle and had ad libitum access to food and water.
All surgical procedures and isoflurane anesthesia were performed as previously described (Tran and Gordon, 2015). Briefly, 1 week before the imaging session, a head bar was surgically installed on the animal, after which the animal was returned to its home cage to recover. Mice were initially trained on a passive air-supported Styrofoam ball treadmill under head restraint for 30 min and habituated to whisker stimulation with an air puff on contralateral vibrissae once every minute for 5 s using a Picospritzer III (General Valve Corp.) for 2 consecutive days. After training, the animal was returned to its home cage. On imaging day, bone and dura over the primary somatosensory cortex were removed and a ∼3 × 3mm cover glass (thickness #0) was installed over the cranial window.
Rhodamine B isothiocyanate (RhodB)-dextran (MW 70,000; Sigma) was injected via the tail vein (100–200 μl of a 2.3% (w/v) solution in saline) to visualize the blood plasma. The animal was allowed to recover on the treadmill, with its head immobilized, for 30 min prior to imaging.
Fluorescence images were obtained using a custom-built in vivo two-photon microscope (Rosenegger et al., 2014) illuminated with a tunable Ti:sapphire laser (Coherent Chameleon, Ultra II), equipped with GaAsP PMTs (Hamamatsu) and controlled by an open-source ScanImage software. A Nikon 16X objective lens (0.8NA, 3 mm WD) or a Zeiss 40X objective lens (1.0NA, 2.5 mm WD) was used. GCaMP3 and Rhodamine B dextran were excited at 920 nm. Green fluorescence signals were obtained using a 525/50 nm band-pass filter, and an orange/red light was obtained using a 605/70 nm band-pass filter (Chroma Technology). Bidirectional xy raster scanning was used at a frame rate of 3.91 Hz. Animal behaviors were captured using a near-infrared LED (780 nm) and a camera at 14 Hz. A 5-s air puff that deflected all whiskers on the contralateral side without impacting the face was applied using a Picospritzer while vasodilation and astrocytic Ca2+ responses were monitored in the barrel cortex (layers 1–3).
All data were processed using ImageJ. Movement artifacts in the xy plane were corrected for using the align_slices plugin. ROIs corresponding to astrocyte endfeet, soma, and arbor were analyzed separately. Small ROIs (2.5×2.5 μm) placed next to one and another around the endfoot was analyzed to obtain temporal sequence of Ca2+ signals around the endfoot. Ca2+ responses were calculated as ΔF/F = (Ft−Frest)/Frest, where Ft is the measured fluorescence at any given time and Frest is the average fluorescence obtained over 2 s prior to whisker stimulation. Ca2+ signals with an intensity that crossed a 3-standard deviation (SD) threshold (average 3SD: ΔF/Fef = 5.9; ΔF/Fsoma = 3.8; ΔF/Farbor = 2.6 and the associated coefficient of variation: 56.5, 65.6, and 63.5% respectively) relative to signal fluctuations during a 2-s prestimulus baseline and remained above the threshold for at least 0.5 s were detected as astrocyte Ca2+ increases. Penetrating arteriole cross-sectional area was analyzed by using the threshold feature in imageJ, after which particle analysis was used to measure the area of the lumen filled with RhodB-dextran. Cross-sectional area changes were calculated as Δd/d = (dt−drest)/drest where dt is the area obtained at any given time and drest is the average baseline area obtained over 2 s prior to whisker stimulation. Area change with an intensity that crossed a 3-SD threshold (average SD for Δd/d = 2.8; coefficient of variation: 50.8%); relative to signal fluctuations during a 2-s prestimulus baseline, and remained above the threshold for at least 0.5 s were detected as vasodilation. Onset corresponds to the first time point at which the signal reached the threshold and remained over it for at least 0.5 s. Duration was calculated as the difference between response onset and response offset. Statistical analyses used a paired or unpaired t-test or one-way analysis of variance (ANOVA) followed by Tukey’s multiple comparisons test as appropriate. Statistical “n” constituted a single experimental trial or an experimental animal, as indicated. Data are expressed as means ± SEM. values of p <0.05 were considered statistically significant. All statistical analyses were done using GraphPad. A 95% confidence interval (CI) was calculated using modified Wald method.
We previously showed that sensory stimulation induces rapid functional hyperemic response that is followed by delayed astrocytic Ca2+ (Tran et al., 2018). In this previous study, we primarily focused on a single penetrating arteriole enwrapped by an endfoot and associated soma and arbor. Since other astrocytes, and in particular endfeet, that enwrapped nearby capillaries within the same cortical layer as that of penetrating arterioles were not examined, it remained unclear whether sensory stimulation induced a global effect that elicited homogeneous Ca2+ changes in all astrocytes. In the present study, we extended these analyses, examining Ca2+ dynamics in as many astrocytes within the field of view as possible and monitoring endfoot Ca2+ changes associated with penetrating arterioles and capillaries in the same cortical layer and focal plane. We used a genetically engineered Ca2+ indicator, a cytosolic form of GCaMP3, driven by the tamoxifen-inducible astrocyte-specific promoter, Slc1a3-Cre/ERT (GLAST-ERT), to assess local intracellular astrocyte Ca2+ dynamics, with concurrent tail vein injection of RhodB-dextran to visualize the vasculature and monitor vascular responses (Figures 1A,B,D). A 5-s whisker stimulation of contralateral side vibrissae predominantly induced a rapid functional hyperemic response that was followed by a rise in astrocytic Ca2+ (Figures 1C,E,G, n = 9 mice; number of trials: vessels, 25; endfoot at penetrating arteriole, 33; endfoot at capillaries, 68; soma, 28; arbor, 37). Even though stimulation of vibrissae did not elicit arteriole dilation in all cases, it induced more vasodilatory responses (74%, CI: 0.55–0.87) than Ca2+ rises (48%, CI: 0.33–0.65) in endfeet, enveloping the penetrating arterioles (Figure 1F). Sensory-induced increases in Ca2+ rises were observed in some, but not all, soma (68%, CI: 0.49–0.82), endfeet (at cap: 60%, CI: 0.48–0.71) and arbors (62%, CI: 0.46–0.76; Figure 1F). Interestingly, there were more cases where sensory-associated Ca2+ increases were observed at endfeet wrapping around the capillaries than at those enwrapping the penetrating arterioles (Figure 1F). More importantly, the spatial and temporal profiles of Ca2+ signals in a given subcellular structure were not always similar from one astrocyte to another when they were imaged simultaneously within the same field of view (Figures 1G–K). While whisker stimulation elicited a rise in astrocytic Ca2+ in all regions of interest (ROIs) from the subcellular compartments in some trials (endfoot at penetrating arterioles: three trials, CI: 0.18–0.81; endfoot at capillaries: 10 trials, CI: 0.23–0.59; soma: four trials, CI: 0.24–0.84; arbor: three trials, CI: 0.12–0.65), it did not trigger Ca2+ changes in any ROIs in other trials. There were also trials in which at least one ROI did not exhibit a rise in Ca2+ (Figure 1G). An increase in endfoot Ca2+ associated with penetrating arterioles was not always accompanied by an increase in endfoot Ca2+ at the capillaries and similarly, a lack of endfoot Ca2+ increases at the penetrating arterioles did not always correspond to a lack in endfoot Ca2+ changes at the capillaries when they were imaged simultaneously within the same field of view (an increase in endfoot Ca2+ at both penetrating arterioles and capillaries: 12 trials, CI: 0.65– >0.99; no increase in endfoot Ca2+ at penetrating arterioles with an increase in endfoot Ca2+ at capillaries: 10 trials, CI: 0.45–0.89; Figure 1H). Functional hyperemic responses were not only more frequently observed than Ca2+ rises, but were also initiated before endfoot Ca2+ increased (onset: 2.2 ± 1.1 s and 3.4 ± 2.3 s for diameter and endfoot Ca2+ response respectively; p = 0.02; Figure 1I), a finding that is consistent with our previous observation (Tran et al., 2018). Although the onset of functional hyperemia was faster than that of endfoot, soma or arbor Ca2+ increases, the durations and time to peak of all responses were comparable (Data not shown). The distribution of onset time for sensory-induced astrocytic Ca2+ changes was more widespread than that of functional hyperemic responses (Figure 1J). While the majority of trials displayed an onset time between 1 and 3 s for functional hyperemia, the onset time of astrocytic Ca2+ rise could be varied from less than 1 s to more than 8 s (Figure 1J).
Figure 1. Contralateral whisker stimulation predominantly elicits reliable arteriole dilation but generates heterogeneous astrocytic Ca2+ responses. (A) Image of an awake mouse and schematics showing the in vivo experimental setup. (B) Representative images from a cranial window over the barrel cortex of a GLAST-Cre ERT x GCaMP3 mouse showing Ca2+ fluctuations (green) from endfoot (ef), soma (s), process (p) and vessel response in penetrating arterioles (pa), pre-capillaries (pre-cap) and capillaries (cap) [labeled with Rhod-B dextran (red)] before (top images) and during (bottom images) a 5 s whisker stimulation. Regions of interest (ROIs) (bottom left) correspond to representative traces in (C). (C) Representative traces from a GLAST-Cre ERT x GCaMP3 mouse (from ROIs as indicated in B) showing the percent change in peak diameter of a penetrating arteriole (navy) and Ca2+ activity from an endfoot at the penetrating arteriole (red), endfoot at the capillaries (magenta), soma (purple), and arbor (green) in response to a 5-s whisker stimulation. Vertical shaded areas indicate periods of whisker stimulation. (D) Higher-magnification images of a penetrating arteriole and nearby capillary enveloped by an astrocytic endfoot from a different mouse showing responses immediately prior to (0 s) and during (5 s) whisker stimulation. ROIs (top right) correspond to representative traces in (E). (E) Representative traces showing changes in penetrating arteriole diameter change and astrocytic Ca2+ fluctuations (from ROIs as indicated in D (top right)) in different subcellular compartments in response to whisker stimulation. (F) Plot showing the percentage of trials generating arteriole dilation and Ca2+ increases in response to a 5-s whisker stimulation. (G) Summary showing the number of trials that displayed Ca2+ changes in all, all but one (at least one) or none from a given subcellular structure among several astrocytes observed within a single field of view. (H) Summary showing heterogeneity of Ca2+ increases observed at endfeet wrapping around the penetrating arterioles and those enwrapping capillaries when they were imaged simultaneously. (I) Summary showing arteriole dilation onset time and that of Ca2+ changes in all subcellular compartments of the astrocyte in response to a 5 s whisker stimulation. (J) Heat map showing the distribution of onset time of arteriole dilation and Ca2+ changes in all subcellular compartments of the astrocyte.
Although some in vivo studies have reported that arteriole dilation is associated with a rapid rise in endfoot Ca2+ (Lind et al., 2013, 2018; Stobart et al., 2018a), others have shown that a majority of vasodilatory responses to sensory stimulation lack an associated astrocytic Ca2+ response (Nizar et al., 2013; Bonder and McCarthy, 2014). In the present work, vasodilation in response to a 5-s whisker stimulation was observed in both the presence and absence of a rise in endfoot Ca2+ (Figures 2A,B). Of the total 175 trials in 22 mice, 95 exhibited an endfoot Ca2+ increase and 80 lacked it. Trials with a rise in endfoot Ca2+ were more frequently associated with vasodilation (42%) than those without an endfoot Ca+ increase (29%; Figure 2C), but we found no significant differences in peak percentage increase in arteriole cross-sectional area, onset time or duration of dilation, or time to peak dilation between these two trial groups (Figure 2D). These data suggest that changes in local endfoot Ca2+ are not directly linked to arteriole dilation during experimentally evoked sensory stimulation in completely awake behaving mice. On the other hand, arteriole dilation significantly enhanced endfoot Ca2+ (ΔF/F = 58.9 ± 7.8% with vasodilation vs. 31.6 ± 7.7% without vasodilation; p = 0.02, n = 22 mice, 175 trials; Figure 2E). Furthermore, the onset of endfoot Ca2+ elevations was significantly faster in cases when there was an associated vasodilation (5.2 ± 0.5 s vs. 7.4 ± 1.1 s, p = 0.04, n = 22 mice, 175 trials). These observations further support our previous findings that changes in arteriole diameter evoked endfoot Ca2+ transients (Tran et al., 2018).
Figure 2. Whisker stimulation-induced arteriole dilation occurs in the absence of astrocytic endfoot Ca2+ but vasodilation augmented endfoot Ca2+. (A) Representative images from two different trials showing a penetrating arteriole enwrapped by an endfoot of a GLAST-Cre ERT x GCaMP3 mouse immediately prior to (0 s) and in response to (6 s) whisker stimulation. (B) Representative traces showing penetrating arteriole diameter and changes in endfoot Ca2+ (from A) from the same mouse but different trials in response to a 5-s whisker stimulation. (C) Percentage of trials with and without dilation in the presence and absence of endfoot Ca2+. (D) Summary of percent peak change (i), onset time (ii), duration (iii), and time to peak (iv) of penetrating arteriole diameter in response to whisker stimulation in the presence or absence of endfoot Ca2+. (E) Summary of percent peak change (i), onset time (ii), duration (iii) and time to peak (iv) of endfoot Ca2+ in response to whisker stimulation in the presence or absence of vasodilation.
We next performed a detailed analysis of endfoot Ca2+ dynamics in response to a 5-s whisker stimulation. Previous studies have shown that astrocytic Ca2+ activity is heterogeneous across different subcellular structures within an astrocyte (Bindocci et al., 2017). Under basal conditions, gliapil, the peripheral region of an astrocyte composed of fine processes, exhibit the highest activity, whereas, soma exhibit the lowest activity. Given their close proximity to the vessel wall, endfeet could conceivably directly modulate, or be modulated by, the vasculature. A detailed analysis of Ca2+ activity within a cross-section of an endfoot enwrapping a penetrating arteriole that dilated in response to whisker stimulation revealed heterogeneous Ca2+ signals (Figure 3). The discrete nature of these Ca2+ signals are revealed in 3-D surface plots that also display, once initiated, how these Ca2+ signals spread (Figures 3Ai,Bi,Ci). Subsequently, we analyzed Ca2+ responses in small regions of interest (ROIs) positioned next to one and another around the endfoot (Figures 3A,B,Cii-iv). Interestingly, sensory stimulation did not initiate a global rise in endfoot Ca2+; instead, it triggered Ca2+ “hot spots” at various discrete regions of the endfoot, from which Ca2+ signals then spread either bidirectionally or unidirectionally (Figure 3E). While some endfeet had several Ca2+ “hot spots” with different onsets (Figures 3A,F), others had only a single hot spot (Figures 3B,F). Occasionally, endfoot Ca2+ increases were observed without any discernable “hot spots” (Figure 3D; n = 10 animals). In some instances, a soma appeared to be physically part of the endfoot; in this particular scenario, Ca2+ signals increased within the soma region either remained localized or did not spread far (Figure 3Ai). This behavior is similar to that observed by Bindocci and colleagues under basal conditions where Ca2+ signals were relatively confined to the boundary of the soma (Bindocci et al., 2017). In another instance in which we could clearly see the endfoot, its associated processes and a soma, 3-D surface plots revealed the spread of Ca2+ signals from different hot spots within each subcellular structure of the astrocyte. However, signals from these astrocytic compartments appeared to be independent of each other (Figure 3C).
Figure 3. Whisker stimulation elicits discrete endfoot Ca2+ signals that subsequently spread. (A) Detailed analysis of Ca2+ dynamics in response to a 5 s whisker stimulation. (i). 3-D surface plot of astrocyte Ca2+ fluctuations in an astrocyte at different time points immediately prior to (0 s), during (1.75 s, 2.75 s), or after (5.25 s, 7.75 s, 11.5 s, 12.5 s, and 15.25 s) a 5-s whisker stimulation. Insets showed the differential images between the two time points. (ii) Higher magnification image of an endfoot and consecutive ROIs from which Ca2+ measurements were obtained. Double-headed arrow indicates the bidirectional spread of the Ca2+ “hot spot” spread. Single-headed arrow indicates the uni-directional spread of the Ca2+ “hot spot”. A dot indicates Ca2+ signals remain localized. (iii) Onset times from different ROIs showing regional Ca2+ activity and vasodilation. (iv) Penetrating arteriole cross-sectional area trace (black) and Ca2+ traces (colors) from different ROIs. Vertical shaded areas indicate periods of whisker stimulation. (B) Detailed analysis similar to that in A, but from a different animal displaying only the endfoot enwrapping a penetrating arteriole. (i) 3-D surface plot of astrocyte Ca2+ fluctuations at different time points immediately prior to (0 s) and after (6.25 s, 7 s, 8 s, 10s, 11.75 s, 13.5 s, and 15.5 s) a 5 s whisker stimulation. Insets showed the differential images between the two time points. (ii) Higher-magnification image of the endfoot and consecutive ROIs (right) from which Ca2+ measurements were obtained. Double-headed arrow indicates bidirectional spread of the Ca2+ “hot spot”. (iii) Onset times from different ROIs showing endfoot Ca2+ activity and vasodilation. (iv) Penetrating arteriole cross-sectional area trace (black) and Ca2+ traces (colors) from different ROIs. Vertical shaded areas indicate periods of 5 s whisker stimulation periods. (C) Detailed analysis similar to that in (A) and (B), but from a different animal showing all subcellular compartments of an astrocyte. (i) 3-D surface plot showing astrocytic Ca2+ fluctuations at different time points immediately prior to (0 s), during (2.5 s, 3 s, and 4 s) and after (5.25 s, 8.75 s, 10.5 s, and 12.5 s) a 5 s whisker stimulation. Insets showed the differential images between the two time points. (ii) Higher-magnification image of an endfoot and consecutive ROIs (top left) from which Ca2+ measurements were obtained. Labels of all the ROIs (bottom) indicated previously. Double-headed arrow indicates bidirectional spread of the Ca2+ “hot spot”. Lower magnification image of the entire astrocyte (top right). (iii) Onset times from different ROIs showing regional Ca2+ activity and vasodilation. (iv) Penetrating arteriole cross-sectional area trace (black) and Ca2+ traces (colors) from different subcellular compartments. Vertical shaded areas indicate periods of whisker stimulation. (D) Summary showing the number of “hot spots” detected associated with the area of the endfoot. (E) Pie chart showing the percentage of how those “hot spots” spread. (F) Scatter plot of onset time for each “hot spot” from individual mouse.
In this study, we revealed that (1) sensory stimulation did not generate a global effect that elicited homogeneous Ca2+ changes in all astrocytes or subcellular compartments of an astrocyte; (2) although the absence of endfoot Ca2+ around the penetrating arteriole did not preclude arteriole dilation, stronger endfoot Ca2+ rises were observed in the presence of vasodilation; (3) sensory stimulation did not elicit a global rise in endfoot Ca2+, but instead triggered discrete Ca2+ “hot spots” that typically spread around the endfoot and occasionally remained localized. These findings indicate that astrocytic Ca2+ dynamics are heterogeneous across different astrocytes as well as between astrocytic subcellular compartments, and suggest that these Ca2+ signals may be compartmentalized during sensory-induced functional hyperemia. They further suggest that the close proximity between the endfoot and the vessel wall of a penetrating arteriole does not necessarily translate to direct effects of local endfoot Ca2+ on arteriole dilation.
In the past decade, studies performed in vivo have presented polarized views on the involvement of astrocytic Ca2+ in functional hyperemia. Some of these studies in anesthetized or slightly sedated animals have shown that fast astrocytic Ca2+ transients precede functional hyperemic responses to sensory stimulation (Takano et al., 2006; Winship et al., 2007; Petzold et al., 2008; Lind et al., 2013, 2018; Stobart et al., 2018a), whereas others have shown that such signals are absent (Schummers et al., 2008; Schulz et al., 2012; Nizar et al., 2013; Bonder and McCarthy, 2014). Our earlier in vivo studies in awake active mice in which we focused on dynamic interactions between a small region of a single penetrating arteriole and its associated endfoot using cross-section imaging showed that a 5-s whisker stimulation induced a rapid functional hyperemic response followed by a delayed increase in endfoot Ca2+ (Tran et al., 2018). The current study in awake animals not only showed that vibrissae stimulation produced fast vasodilation followed by less reliable astrocytic Ca2+ increases but it also revealed the heterogeneous characteristic of astrocytic Ca2+ signals across different astrocytes within a single field of view (Figure 1). These whisker stimulation-induced Ca2+ signals appeared to be asynchronous and regional. Our findings are in agreement with previous studies (Bindocci et al., 2017; Stobart et al., 2018b) that demonstrated some fundamental differences in Ca2+ dynamics between individual structures of an astrocyte. These observations implicate a compartmentalizing effect in astrocyte Ca2+ dynamics. They also call attention to the fact that the majority of prior in vivo studies monitored Ca2+ changes selectively at small regions of astrocytic subcellular structures or assessed bulk changes in Ca2+ in the entire astrocyte, but nonetheless concluded that these observations were representatives of the whole-cell activity or the activity of the entire network. In the current study, simultaneous measurements of vascular reactivity and astrocytic Ca2+ changes revealed that continuous vibrissae stimulation for 5 s predominantly induced arteriole dilation that was accompanied by a rise in endfoot Ca2+. However, a significant number of trials showed vasodilation in the absence of endfoot Ca2+ change, a finding in agreement with previous studies (Schulz et al., 2012; Nizar et al., 2013; Bonder and McCarthy, 2014). Nevertheless, our data showed the likelihood of observing vasodilation with endfoot Ca2+ vs. that without endfoot Ca2+ was ~42% vs. 29% instead of 10% vs. 90%, as reported from Nizar and colleagues (Nizar et al., 2013). They are also clearly distinct from a previous report that endfoot Ca2+ responses were completely absent in all trials (Bonder and McCarthy, 2014). These discrepancies could be attributable partly to the use of anesthesia (Thrane et al., 2011). These findings do not necessarily refute the role of endfoot Ca2+ in regulating functional hyperemia on a whole. In fact, they highlight the heterogeneity of astrocytic Ca2+ dynamics and suggest that the close proximity of the endfoot and vessel wall does not universally translate to a direct effect of endfoot Ca2+ changes on local vasodilation. The dilation of penetrating arterioles observed in layers I–III of the cortex reported here could be due to the retrograde vascular conduction that was initiated deep in the cortex (Uhlirova et al., 2016; Longden et al., 2017), and the endfoot Ca2+ increases subsequently observed in deeper cortical layers could exhibit different dynamics from those observed in the relatively superficial layer of the cortex. Interestingly, we found here that sensory-induced endfoot Ca2+ increases were significantly stronger when they were accompanied by vasodilation of the penetrating arterioles (Figure 2), further supporting our previous observations that vasodilation obtained independent of neural activity modulates endfoot Ca2+ (Tran et al., 2018). The changes in astrocytic Ca2+ in response to changes in vasomotor tone suggest a potential arteriole-to-astrocyte communication as previously suggested by our work and others (Kim et al., 2016; Tran et al., 2018).
Situated as they are between neurons and the vasculature, forming a tripartite architecture, astrocytes are ideally positioned to facilitate neuron-vascular communication and have been increasingly viewed as a hub of integrated activity that modulates neuronal and vascular responses. Activity-dependent astrocytic Ca2+ responses are typically reported as global events (Zonta et al., 2003; Lind et al., 2013), in which increased neuronal activity triggers a global rise in Ca2+ throughout the whole astrocyte or individual compartment. A recent work described more localized events that spread within the confined boundaries of the subcellular structures (Bindocci et al., 2017). Similar to these latter studies, our work reported here using cross-section imaging explored Ca2+ dynamics in some of the endfeet that almost completely ensheathed the vessel (Figure 3). Although an initial analysis appeared to show a global rise in endfoot Ca2+ in response to a 5-s whisker stimulation; a detailed analysis of these Ca2+ responses revealed discrete Ca2+ “hot spots” that typically spread. The majority of these “hot spots” spread uni-directionally, while others spread bidirectionally around the endfoot. Yet, in some instances, the “hot spot” remained as a single discrete Ca2+ signal. There were also cases where no discernible “hot spot” was observed. In agreement with a previous work (Bindocci et al., 2017), we found that astrocytic Ca2+ signals were compartmentalized. For example, in cases where the soma was a part of an endfoot, the somatic Ca2+ signal seemed to remain confined to the region defined as a soma. Similar observations were noted in other soma that were not physically a part of the endfoot. These findings suggest that astrocyte can assemble as multiple local units that function heterogeneously, implying that astrocytes can locally sense features of their surrounding environment, whether it is a nearby neuron or a vessel wall, and respond accordingly. Studies have further shown that resting Ca2+ differs in different astrocytic regions (Zheng et al., 2015), suggesting that there might be some regional control. These observations suggest that there are specific regions within the soma, endfoot and process where plasma membrane channels (e.g., transient receptor potential vanilloid 4, TRPV4) and/or intracellular organelles possessing the machinery, such as the mitochondria (Agarwal et al., 2017), to initiate Ca2+ changes reside, and that these are responsible for inducing the spread of Ca2+ signals, perhaps via Ca2+-induced Ca2+ release. Similarly, these subcellular structures may have organelles, such as endoplasmic reticulum (ER) or mitochondria that buffer Ca2+ and prevent the signals from spreading beyond a boundary. Other studies have described global events observed in all subcellular compartments (Ding et al., 2013; Paukert et al., 2014; Srinivasan et al., 2015). However, these events appeared to be associated with specific physiological conditions, in this case, a startle response (Ding et al., 2013; Bonder and McCarthy, 2014; Paukert et al., 2014; Srinivasan et al., 2015). This implies that an astrocyte needs to reach a certain threshold before it can trigger the coordination between different compartments and, potentially, between different astrocytes. In the quiescent state, astrocytes have a high threshold for activation such that other systems, such as noradrenergic (Paukert et al., 2014) and/or cholinergic (Takata et al., 2011) circuits, must be recruited to enhance local signals.
Our work has unveiled the complexity of astrocytic Ca2+ dynamics and addressed the relationship between such Ca2+ signals and NVC. We do not dispute the role of astrocytic Ca2+ in regulating vascular response; however, for several reasons, we recommend caution in universally interpreting NVC based on data from an isolated subpopulation of astrocytes imaged at a certain layer of the cortex. First, since astrocytic Ca2+ activity is heterogeneous, it would be inaccurate to take observations from a single compartment or a single astrocyte as being representative of the whole cell or the whole network. Second, somatosensory cortical activation is significantly different between layers of the cortex (Krupa et al., 2004). Finally, different levels of the vascular network are structurally and functionally reflected in differences in architecture (Shih et al., 2015), anastomoses, level of neuronal innervation (Hamel, 2006), and expression of receptors and ion channels (Sercombe et al., 1990). Collectively, this vascular architecture serves to provide a supply of blood to every single neural cell sufficient to match the metabolic needs of the cell. In fact, recent studies have demonstrated temporal differences in sensory-induced vasodilation across different layers of the cortex, with the fastest onset of dilation observed below layer IV (Uhlirova et al., 2016). It has been proposed that upstream penetrating arterioles or pial arterioles dilate in response to signals initiated few 100 μm away achieved via a retrograde vascular conduction mechanism (Longden et al., 2017). Nevertheless, this does not imply that conduction is the only process that mediates upstream dilation nor does it rule out the possible involvement of NVC at all cortical depths. There are some important caveats that we need to acknowledge: (1) all two-photon fluorescence imaging were conducted at a frame rate of 3.91 Hz. This might have prevented us from detecting faster discrete Ca2+ signals; (2) we acknowledge that the fact that small ROIs used to detect discrete Ca2+ were done manually could contribute to the under-detection of the signals. In conclusions, our findings further emphasize that examining NVC in 3-Ds and at different layers of the cortex in awake animals is necessary to obtain accurate assessments of astrocytic Ca2+. Furthermore, they highlight the need to have an automation of Ca2+ analysis designed to better detect all signals from all subcellular compartments of the astrocyte.
The original contributions presented in the study are included in the article/supplementary materials, further inquiries can be directed to the corresponding author.
The animal study was reviewed and approved by The Animal Care and Use Committee of the University of Calgary.
CT and GG: conceptualization, methodology, writing – review and editing. CT: investigation. CT and KS: formal analysis, writing – original draft. All authors contributed to the article and approved the submitted version.
This work was supported by operating grants from the Canadian Institute of Health Research to GG (130233) and the Centers of Biomedical Research Excellence (COBRE) to CT (1P20GM130459).
The authors declare that the research was conducted in the absence of any commercial or financial relationships that could be construed as a potential conflict of interest.
We thank the developers and distributers of ScanImage open-source control and acquisition software for two-photon laser-scanning microscopy as well as ImageJ for data analysis. We also want to thank Eslam M F Mehina for her contributions.
Agarwal, A., Wu, P. -H., Hughes, E. G., Fukaya, M., Tischfield, M. A., Langseth, A. J., et al. (2017). Transient opening of the mitochondrial permeability transition pore induces microdomain calcium transients in astrocyte processes. Neuron 93, 587–605.e7. doi: 10.1016/j.neuron.2016.12.034
Bekar, L. K., He, W., and Nedergaard, M. (2008). Locus coeruleus alpha-adrenergic-mediated activation of cortical astrocytes in vivo. Cereb. Cortex 18, 2789–2795. doi: 10.1093/cercor/bhn040
Bindocci, E., Savtchouk, I., Liaudet, N., Becker, D., Carriero, G., and Volterra, A. (2017). Three-dimensional Ca2+ imaging advances understanding of astrocyte biology. Science 356:eaai8185. doi: 10.1126/science.aai8185
Bonder, D. E., and McCarthy, K. D. (2014). Astrocytic Gq-GPCR-linked IP3R-dependent Ca2+ signaling does not mediate neurovascular coupling in mouse visual cortex in vivo. J. Neurosci. 34, 13139–13150. doi: 10.1523/JNEUROSCI.2591-14.2014
Ding, F., O’Donnell, J., Thrane, A. S., Zeppenfeld, D., Kang, H., Xie, L., et al. (2013). α1-adrenergic receptors mediate coordinated Ca2+ signaling of cortical astrocytes in awake, behaving mice. Cell Calcium 54, 387–394. doi: 10.1016/j.ceca.2013.09.001
Gordon, G. R. J., Choi, H. B., Rungta, R. L., Ellis-Davies, G. C. R., and MacVicar, B. A. (2008). Brain metabolism dictates the polarity of astrocyte control over arterioles. Nature 456, 745–749. doi: 10.1038/nature07525
Hamel, E. (2006). Perivascular nerves and the regulation of cerebrovascular tone. J. Appl. Physiol. 100, 1059–1064. doi: 10.1152/japplphysiol.00954.2005
Kim, K. J., Ramiro Diaz, J., Iddings, J. A., and Filosa, J. A. (2016). Vasculo-neuronal coupling: retrograde vascular communication to brain neurons. J. Neurosci. 36, 12624–12639. doi: 10.1523/JNEUROSCI.1300-16.2016
Krupa, D. J., Wiest, M. C., Shuler, M. G., Laubach, M., and Nicolelis, M. A. L. (2004). Layer-specific somatosensory cortical activation during active tactile discrimination. Science 304, 1989–1992. doi: 10.1126/science.1093318
Lind, B. L., Brazhe, A. R., Jessen, S. B., Tan, F. C. C., and Lauritzen, M. J. (2013). Rapid stimulus-evoked astrocyte Ca2+ elevations and hemodynamic responses in mouse somatosensory cortex in vivo. Proc. Natl. Acad. Sci. U. S. A. 110, E4678–E4687. doi: 10.1073/pnas.1310065110
Lind, B. L., Jessen, S. B., Lønstrup, M., Joséphine, C., Bonvento, G., and Lauritzen, M. (2018). Fast Ca2+ responses in astrocyte end-feet and neurovascular coupling in mice. Glia 66, 348–358. doi: 10.1002/glia.23246
Longden, T. A., Dabertrand, F., Koide, M., Gonzales, A. L., Tykocki, N. R., Brayden, J. E., et al. (2017). Capillary K+-sensing initiates retrograde hyperpolarization to increase local cerebral blood flow. Nat. Neurosci. 20, 717–726. doi: 10.1038/nn.4533
Mulligan, S. J., and MacVicar, B. A. (2004). Calcium transients in astrocyte endfeet cause cerebrovascular constrictions. Nature 431, 195–199. doi: 10.1038/nature02827
Nimmerjahn, A., Mukamel, E. A., and Schnitzer, M. J. (2009). Motor behavior activates Bergmann glial networks. Neuron 62, 400–412. doi: 10.1016/j.neuron.2009.03.019
Nizar, K., Uhlirova, H., Tian, P., Saisan, P. A., Cheng, Q., Reznichenko, L., et al. (2013). In vivo stimulus-induced vasodilation occurs without IP3 receptor activation and may precede astrocytic calcium increase. J. Neurosci. 33, 8411–8422. doi: 10.1523/JNEUROSCI.3285-12.2013
Otsu, Y., Couchman, K., Lyons, D. G., Collot, M., Agarwal, A., Mallet, J. -M., et al. (2015). Calcium dynamics in astrocyte processes during neurovascular coupling. Nat. Neurosci. 18, 210–218. doi: 10.1038/nn.3906
Paukert, M., Agarwal, A., Cha, J., Doze, V. A., Kang, J. U., and Bergles, D. E. (2014). Norepinephrine controls astroglial responsiveness to local circuit activity. Neuron 82, 1263–1270. doi: 10.1016/j.neuron.2014.04.038
Petzold, G. C., Albeanu, D. F., Sato, T. F., and Murthy, V. N. (2008). Coupling of neural activity to blood flow in olfactory glomeruli is mediated by astrocytic pathways. Neuron 58, 897–910. doi: 10.1016/j.neuron.2008.04.029
Rosenegger, D. G., Tran, C. H. T., LeDue, J., Zhou, N., and Gordon, G. R. (2014). A high performance, cost-effective, open-source microscope for scanning two-photon microscopy that is modular and readily adaptable. PLoS One 9:e110475. doi: 10.1371/journal.pone.0110475.s005
Schulz, K., Sydekum, E., Krueppel, R., Engelbrecht, C. J., Schlegel, F., Schröter, A., et al. (2012). Simultaneous BOLD fMRI and fiber-optic calcium recording in rat neocortex. Nat. Methods 9, 597–602. doi: 10.1038/nmeth.2013
Schummers, J., Yu, H., and Sur, M. (2008). Tuned responses of astrocytes and their influence on hemodynamic signals in the visual cortex. Science 320, 1638–1643. doi: 10.1126/science.1156120
Sercombe, R., Hardebo, J. E., Kåhrström, J., and Seylaz, J. (1990). Amine-induced responses of pial and penetrating cerebral arteries: evidence for heterogeneous responses. J. Cereb. Blood Flow Metab. 10, 808–818. doi: 10.1038/jcbfm.1990.137
Shigetomi, E., Bushong, E. A., Haustein, M. D., Tong, X., Jackson-Weaver, O., Kracun, S., et al. (2013). Imaging calcium microdomains within entire astrocyte territories and endfeet with GCaMPs expressed using adeno-associated viruses. J. Gen. Physiol. 141, 633–647. doi: 10.1085/jgp.201210949
Shih, A. Y., Rühlmann, C., Blinder, P., Devor, A., Drew, P. J., Friedman, B., et al. (2015). Robust and fragile aspects of cortical blood flow in relation to the underlying angioarchitecture. Microcirculation 22, 204–218. doi: 10.1111/micc.12195
Simard, M., Arcuino, G., Takano, T., Liu, Q. -S., and Nedergaard, M. (2003). Signaling at the gliovascular interface. J. Neurosci. 23, 9254–9262. doi: 10.1523/JNEUROSCI.23-27-09254.2003
Srinivasan, R., Huang, B. S., Venugopal, S., Johnston, A. D., Chai, H., Zeng, H., et al. (2015). Ca2+ signaling in astrocytes from Ip3r2(−/−) mice in brain slices and during startle responses in vivo. Nat. Neurosci. 18, 708–717. doi: 10.1038/nn.4001
Stobart, J. L., Ferrari, K. D., Barrett, M. J. P., Glück, C., Stobart, M. J., Zuend, M., et al. (2018a). Cortical circuit activity evokes rapid astrocyte calcium signals on a similar timescale to neurons. Neuron 98, 726–735.e4. doi: 10.1016/j.neuron.2018.03.050
Stobart, J. L., Ferrari, K. D., Barrett, M. J. P., Stobart, M. J., Looser, Z. J., Saab, A. S., et al. (2018b). Long-term in vivo calcium imaging of astrocytes reveals distinct cellular compartment responses to sensory stimulation. Cereb. Cortex 28, 184–198. doi: 10.1093/cercor/bhw366
Straub, S. V., Bonev, A. D., Wilkerson, M. K., and Nelson, M. T. (2006). Dynamic inositol trisphosphate-mediated calcium signals within astrocytic endfeet underlie vasodilation of cerebral arterioles. J. Gen. Physiol. 128, 659–669. doi: 10.1085/jgp.200609650
Straub, S. V., and Nelson, M. T. (2007). Astrocytic calcium signaling: the information currency coupling neuronal activity to the cerebral microcirculation. Trends Cardiovasc. Med. 17, 183–190. doi: 10.1016/j.tcm.2007.05.001
Takano, T., Tian, G. -F., Peng, W., Lou, N., Libionka, W., Han, X., et al. (2006). Astrocyte-mediated control of cerebral blood flow. Nat. Neurosci. 9, 260–267. doi: 10.1038/nn1623
Takata, N., Mishima, T., Hisatsune, C., Nagai, T., Ebisui, E., Mikoshiba, K., et al. (2011). Astrocyte calcium signaling transforms cholinergic modulation to cortical plasticity in vivo. J. Neurosci. 31, 18155–18165. doi: 10.1523/JNEUROSCI.5289-11.2011
Takata, N., Nagai, T., Ozawa, K., Oe, Y., Mikoshiba, K., and Hirase, H. (2013). Cerebral blood flow modulation by basal forebrain or whisker stimulation can occur independently of large cytosolic Ca2+ signaling in astrocytes. PLoS One 8:e66525. doi: 10.1371/journal.pone.0066525
Thrane, A. S., Rappold, P. M., Fujita, T., Torres, A., Bekar, L. K., Takano, T., et al. (2011). Critical role of aquaporin-4 (AQP4) in astrocytic Ca2+ signaling events elicited by cerebral edema. Proc. Natl. Acad. Sci. U. S. A. 108, 846–851. doi: 10.1073/pnas.1015217108
Tran, C. H. T., and Gordon, G. R. (2015). Acute two-photon imaging of the neurovascular unit in the cortex of active mice. Front. Cell. Neurosci. 9:11. doi: 10.3389/fncel.2015.00011/abstract
Tran, C. H. T., Peringod, G., and Gordon, G. R. (2018). Astrocytes integrate behavioral state and vascular signals during functional hyperemia. Neuron 100, 1133–1148.e3. doi: 10.1016/j.neuron.2018.09.045
Uhlirova, H., Kılıç, K., Tian, P., Thunemann, M., Desjardins, M., Saisan, P. A., et al. (2016). Cell type specificity of neurovascular coupling in cerebral cortex. elife 5:e14315. doi: 10.7554/eLife.14315
Winship, I. R., Plaa, N., and Murphy, T. H. (2007). Rapid astrocyte calcium signals correlate with neuronal activity and onset of the hemodynamic response in vivo. J. Neurosci. 27, 6268–6272. doi: 10.1523/JNEUROSCI.4801-06.2007
Zheng, K., Bard, L., Reynolds, J. P., King, C., Jensen, T. P., Gourine, A. V., et al. (2015). Time-resolved imaging reveals heterogeneous landscapes of Nanomolar Ca2+ in neurons and astroglia. Neuron 88, 277–288. doi: 10.1016/j.neuron.2015.09.043
Keywords: two-photon imaging, cerebral blood flow, calcium, awake in vivo, functional hyperemia, astrocyte
Citation: Sharma K, Gordon GRJ and Tran CHT (2020) Heterogeneity of Sensory-Induced Astrocytic Ca2+ Dynamics During Functional Hyperemia. Front. Physiol. 11:611884. doi: 10.3389/fphys.2020.611884
Received: 29 September 2020; Accepted: 24 November 2020;
Published: 10 December 2020.
Edited by:
Fabrice Dabertrand, University of Colorado School of Medicine, United StatesReviewed by:
Andy Shih, Seattle Children’s Research Institute, United StatesCopyright © 2020 Sharma, Gordon and Tran. This is an open-access article distributed under the terms of the Creative Commons Attribution License (CC BY). The use, distribution or reproduction in other forums is permitted, provided the original author(s) and the copyright owner(s) are credited and that the original publication in this journal is cited, in accordance with accepted academic practice. No use, distribution or reproduction is permitted which does not comply with these terms.
*Correspondence: Cam Ha T. Tran, Y2FtdEB1bnIuZWR1
Disclaimer: All claims expressed in this article are solely those of the authors and do not necessarily represent those of their affiliated organizations, or those of the publisher, the editors and the reviewers. Any product that may be evaluated in this article or claim that may be made by its manufacturer is not guaranteed or endorsed by the publisher.
Research integrity at Frontiers
Learn more about the work of our research integrity team to safeguard the quality of each article we publish.