Corrigendum: Lactate Metabolism and Satellite Cell Fate
- 1Department of Clinical Application, Center for iPS Cell Research and Application (CiRA), Kyoto University, Kyoto, Japan
- 2Research Center of Molecular Exercise Science, University of Physical Education, Budapest, Hungary
- 3Graduate School of Sports and Health Science, Doshisha University, Kyoto, Japan
Lactate is one of the metabolic products of glycolysis. It is widely accepted as an important energy source for many cell types and more recently has been proposed to actively participate in cell-cell communication. Satellite cells (SCs), which are adult skeletal muscle stem cells, are the main players of the skeletal muscle regeneration process. Recent studies have proposed a metabolic switch to increase glycolysis in activated SCs. Moreover, lactate has been shown to affect SCs and myoblasts in vivo and in vitro. In this short review, we describe how metabolic variations relate with SC fate (quiescence, activation, proliferation, migration, differentiation, fusion, and self-renewal), as well as discuss possible relationships between lactate as a metabolite and as a signaling molecule affecting SC fate.
Introduction
Satellite cells (SCs) are the main players responsible for the regeneration and maintenance of human adult skeletal muscle (Chargé and Rudnicki, 2004). During homeostasis, SCs are in a quiescent state with relatively low metabolic demands (Rodgers et al., 2014; Pala et al., 2018). Under stress, however, caused by injury, exercise, or other variables, SCs become activated to proliferate, differentiate, and fuse to regenerate the tissue. The transcriptional cascade that leads to these events has been deeply studied (Baghdadi and Tajbakhsh, 2018), but the mechanism that leads to these transcriptomic activations is not fully understood. Recent research has suggested that external factors such as metabolites or factors released by surrounding cells cause the activation of SCs (Tsuchiya et al., 2020; Zhang et al., 2020).
Interestingly, glycolysis has been related to many mechanisms that regulate epigenetic modifications in SCs (Ryall et al., 2015; Yucel et al., 2019). Lactate, which is produced by glycolysis, is easily transported between cells, making it a candidate mediator for these glycolysis effects. Furthermore, one recent study reported a new epigenetic modification process called lactylation, which is regulated by lactate (Zhang et al., 2019). However, little research has given attention to the possibility that lactate may regulate the epigenetic modifications that determine the SC state. In this short review, we summarize recent findings regarding SC metabolism and its effect on SC fate, with special consideration on lactate.
Role of Satellite Cells in Skeletal Muscle
Healthy skeletal muscle is characterized by a high regenerative capacity (Chargé and Rudnicki, 2004). This regenerative capacity is attributed to the presence of SCs (Mauro, 1961). SCs are located beneath the basal lamina and close to the capillaries (Christov et al., 2007; Nederveen et al., 2016; Verma et al., 2018). Proper SC function is critical for not only the regeneration of adult skeletal muscle, but also its maintenance including muscle mass (Bentzinger et al., 2012).
During homeostasis, SCs exist in a quiescent state. Upon injury, SCs enter the cell cycle to become activated and undergo several rounds of proliferation, a process that happens very quickly from the 1st days after injury (Baghdadi et al., 2018). During proliferation, asymmetric and symmetric divisions happen. Symmetrically divided cells (mostly planar divisions) can give rise to two activated SCs or two SCs committed to myogenesis, while asymmetrically divided cells (mostly apical-basal division) will give rise to one activated SC and one quiescent SC, which contributes to the self-renewal of SCs (Cossu and Tajbakhsh, 2007; Kuang et al., 2007; Motohashi and Asakura, 2014). Activated SCs possess increased migratory capacity, which allows them to migrate to the injured sites (Baghdadi et al., 2018; Evano and Tajbakhsh, 2018). Once they reach there, the majority of activated SCs will start to differentiate into myoblasts and fuse to each other and to existing fibers to regenerate the injured site. A smaller population of active SCs will re-enter the quiescent state to maintain the SC pool (Rocheteau et al., 2012).
Satellite cells have been deeply studied, and the transcriptional program that regulates their fate has been established (Baghdadi and Tajbakhsh, 2018). Quiescent SCs are characterized by the expression of PAX7 (Seale et al., 2000), a paired-type homeobox transcription factor that is recognized as the major marker of adult SCs. After activation, PAX7 expression is decreased, and myogenic regulatory factors (MRFs) are expressed. MRFs are a family of basic helix-loop-helix (bHLH) transcription factors composed of MYF5, MYOD, MRF4, and MYOGENIN that promote the expressions of skeletal muscle-specific genes, determining which cells commit to myogenic differentiation (Pownall et al., 2002; Zammit, 2017; Asfour et al., 2018). When SCs become activated, MYF5 and MYOD expression is increased, while PAX7 expression decreases such that its protein level is undetectable in proliferating SCs. Later, cells that undergo differentiation will start expressing MYOG and MRF4 to exit the cell cycle and fully commit into myogenic differentiation (Baghdadi and Tajbakhsh, 2018).
Besides the transcription factors that determine SC fate, epigenetic changes in SCs have been shown to regulate myogenic differentiation (Liu et al., 2013; Wang et al., 2019; Shcherbina et al., 2020). Histones are proteins that provide structural support for packing DNA. Many histone modifications are known to be important for regulating transcriptional activity (Strahl and Allis, 2000). Consistently, when transitioning from the quiescent to activated state, SCs undergo several histone modifications associated with transcriptional changes that lead to myogenic differentiation (Liu et al., 2013). Furthermore, growing evidence has linked histone modifications with metabolism (Sabari et al., 2017).
Metabolic Regulation of Satellite Cells During Regeneration
Different studies have shown that the metabolic profiles differ between quiescent, activated, and differentiated SCs, indicating that metabolic changes may affect SC fate (Ryall, 2013; Tang and Rando, 2014; Ryall et al., 2015; Pala et al., 2018; Yucel et al., 2019).
The majority of adult stem cells exist in a quiescent state and have low metabolic demand (Ito and Suda, 2014). SCs have no exception to this rule. It has been reported that after injury, SCs exit quiescence and increase their energy expenditure (Rodgers et al., 2014). Quiescent SCs have a low metabolism characterized by an increase in the expression of genes related to fatty acids oxidation (Fukada et al., 2007; Ryall et al., 2015), suggesting that lipids are the main source of energy for these cells. An RNA-sequencing (RNA-seq) analysis of freshly isolated mouse SCs (quiescent state) and cultured SCs (proliferating state) revealed that the expression of lipid metabolism-related genes is higher in the quiescent SCs than proliferating SCs (Fukada et al., 2007). Similarly, two recent studies (Ryall et al., 2015; Yucel et al., 2019) found that SCs when activated undergo a metabolic shift from lipids to glucose oxidation. When SCs exit the quiescent state, their energetic demands increase (Rocheteau et al., 2012; Rodgers et al., 2014; Dell’Orso et al., 2019; Purohit and Dhawan, 2019). Furthermore, it has been shown that quiescent SCs possess lower levels of mitochondrial activity than activated SCs (Rocheteau et al., 2012; Rodgers et al., 2014). Importantly, one single cell RNA-seq study of SCs in a skeletal muscle mouse injury model confirmed the increased expression of genes related to glycolysis and the tricarboxylic acid (TCA) cycle in activated SCs (Dell’Orso et al., 2019), suggesting that the higher energetic need of the activated SCs is fulfilled by aerobic and anaerobic pathways.
These metabolic changes have been proposed to play important roles in SCs fate. For instance, one study (Theret et al., 2017) suggested that AMP-activated protein kinase (AMPK), which is a master regulator of metabolic homeostasis (Hardie et al., 2012), regulates SC self-renewal by controlling metabolic homeostasis. In that study, AMPK knockout mouse SCs showed a high self-renewal rate and a switch of their metabolism to higher glycolysis, which impaired skeletal muscle regeneration. These findings provided evidence that metabolism and SC fate are connected. At the same time, AMPK has been shown to be regulated by nutritional strategies such as caloric restriction (Canto and Auwerx, 2011). In fact, caloric restriction has been shown to increase SC activity and to enhance skeletal muscle regeneration in mice (Cerletti et al., 2012), strengthening the hypothesis that metabolism regulates SCs fate.
Metabolic changes associated with different SC states have been also correlated with chromatin modifications (Purohit and Dhawan, 2019). Histone acetylation, which is a chromatin modification associated with open chromatin and gene expressions, is regulated in part by the availability of acetyl-CoA (Wellen et al., 2009; Moussaieff et al., 2015). Furthermore, glucose is one of the main precursors for acetyl-CoA; during glycolysis, glucose produces pyruvate, which can be later converted into acetyl-CoA in mitochondria in a process mediated by pyruvate dehydrogenase (PDH). Thereafter, acetyl-CoA is converted to citrate, which can enter the TCA cycle and can be used to produce energy. Moreover, citrate is also transported out of mitochondria and diffuses to nuclei, where it can be used as a substrate to produce acetyl-CoA for histone acetylation. Interestingly, in proliferating SCs, an increase in glucose-derived citrate was found (Yucel et al., 2019). The same study found that this excess of citrate led to an increase in histone acetylation, further leading to a more accessible chromatin that ultimately modulated myogenic differentiation (Figure 1A).
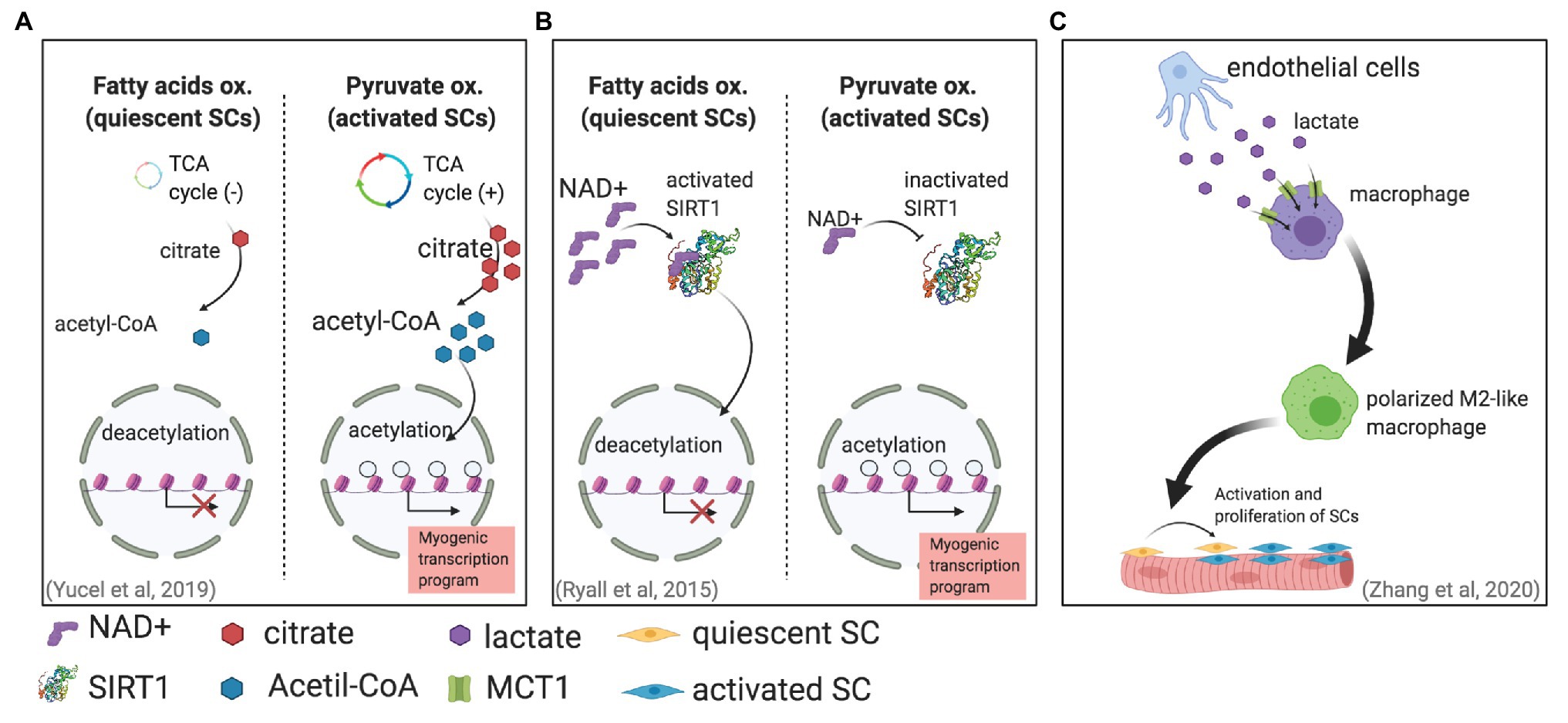
Figure 1. Different mechanisms in which metabolism can affect satellite cell (SC) fate. (A) SC activation is associated with an increase in pyruvate oxidation. This increase generates more citrate production, leading to more acetyl-CoA for histone acetylation that will enable gene transcription (Yucel et al., 2019). (B) Activated SCs increase pyruvate oxidation, reducing NAD+ and therefore SIRT1 activity. The result is higher histone acetylation and an activated myogenic transcriptional program. (C) Endothelial cells can secrete lactate that enters and induces the polarization of macrophages, supporting muscle regeneration by promoting SC proliferation and fusion (Zhang et al., 2020).
Another mechanism has been proposed for the metabolically induced chromatin modifications in activated SCs (Ryall et al., 2015). Metabolic changes can regulate the NAD+-SIRT1 pathway; SIRT1 is a histone deacetylase that uses NAD+ as an enzymatic cofactor to accomplish histone deacetylation (Guarente, 2011; Jing and Lin, 2015). This mechanism, which links metabolic and stress changes with epigenetic modifications of the DNA, is of special relevance to maintaining genomic integrity (Bosch-Presegue and Vaquero, 2015). This mechanism has been suggested to be fundamental for regulating epigenetic processes in stem cells (Fang et al., 2019). Ryall et al. (2015) showed in vitro by using cultured mouse SCs and in vivo by using SIRT1 deacetylase domain ablation mouse models that the shift from fatty acid oxidation to glycolytic metabolism in activated SCs produces less intracellular NAD+ and consequently less SIRT1 activity, which ultimately leads to histone acetylation and the activation of muscle-specific gene transcription that promotes myogenesis (Figure 1B).
Given that lactate is a precursor of pyruvate and its role in reactions that involve NAD+/NADH conversion (see Figure 2), and also considering that the NAD+/NADH balance is critical for SIRT1 activation, it is reasonable to think that lactate may have some role in the metabolic control of SC fate with SIRT1 inactivation, favoring SCs activation. Indeed, some studies have suggested that lactate may affect SC commitment by inducing myogenic differentiation (Willkomm et al., 2014; Oishi et al., 2015; Ohno et al., 2019), but no study has reported a direct mechanism yet.
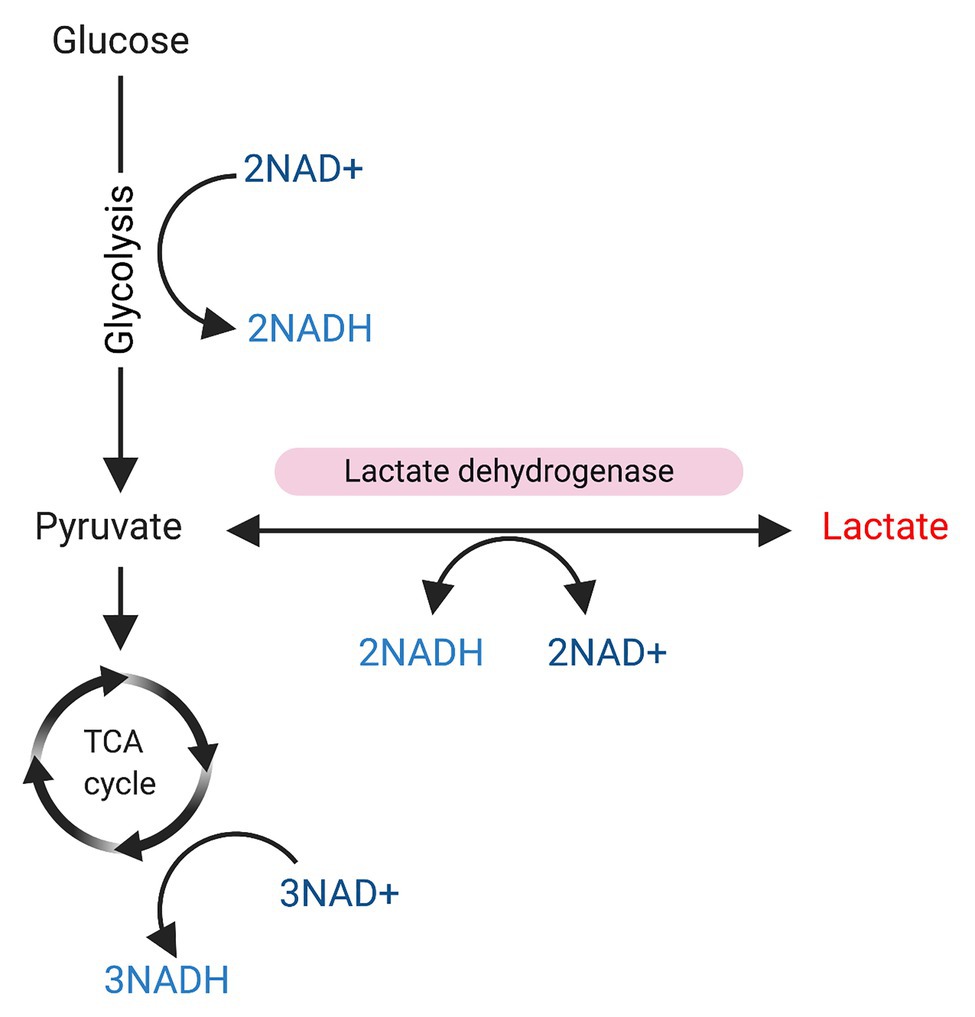
Figure 2. Glycolysis and sources of NAD/NADH+. Glucose is used as a substrate for glycolysis. During glycolysis 2 NAD+ will be converted to NADH, and pyruvate will be produced. Pyruvate can be used as substrate for the tricarboxylic acid (TCA) cycle, resulting in the conversion of 3NAD+ into NADH. On the other hand, pyruvate can be also converted into lactate in a reaction mediated by lactate dehydrogenase, which converts 2NADH to NAD+.
Lactate as a Key Metabolite in the Control of Cell Signaling
Lactate is a metabolite produced from pyruvate by lactate dehydrogenase (LDH), with the LDH isoform A (LDHA) facilitating the pyruvate-to-lactate conversion in cells with high glycolytic rates, and the LDH isoform B (LDHB) facilitating the lactateto-pyruvate conversion in highly oxidative cells. When the cytoplasmic lactate concentration is elevated, lactate can be co-transported with one H+ ion outside the cell by facilitated diffusion via monocarboxylate transporters (MCTs; Halestrap and Wilson, 2012; Kitaoka et al., 2012; Halestrap, 2013; Perez-Escuredo et al., 2016). MCT1 and MCT4 are MCT isoforms expressed in skeletal muscle (Bonen, 2001). MCT1, which has a relatively low Km (3.5–10mM; Halestrap, 2012), is the predominant isoform in oxidative skeletal muscle fibers and considered responsible for lactate uptake (McCullagh et al., 1997; Juel and Halestrap, 1999; Pilegaard et al., 1999; Halestrap, 2012; Chatel et al., 2017). On the other hand, MCT4, which has a much higher Km (22–28mM; Halestrap, 2012), is the isoform predominantly expressed in glycolytic skeletal muscle fibers and considered responsible for lactate release (Dimmer et al., 2000; Fox et al., 2000; Bisetto et al., 2019). Extracellular lactate can travel through the blood stream to many cells, serving as an important energy source for several tissues and organs such as the brain (van Hall et al., 2009; Mosienko et al., 2015), liver, and skeletal muscle (Hui et al., 2017; Brooks, 2020). Given lactate’s ability to travel between cells, tissues, and organs, recently it was proposed to be a signaling molecule (Nalbandian and Takeda, 2016; Brooks, 2020).
The idea of lactate being a signaling molecule has been tested in several models. In differentiated C2C12 cells (mouse immortalized myoblasts), culture with L-lactate increased the expression of PGC1-alpha (peroxisome proliferator-activated receptor gamma coactivator 1-alpha), which is a transcription factor responsible for mitochondrial biogenesis (Nalbandian et al., 2019). Similarly, in differentiated L6 cells (immortalized rat myoblasts), culture with lactate increased the expression of PGC1-alpha as well as the expression of several genes related to metabolism like MCT1 (Hashimoto et al., 2007). Moreover, these results were confirmed in vivo by the intraperitoneal injection of lactate in mice (Kitaoka et al., 2016), where lactate could increase the skeletal muscle PGC1-alpha expression after 3 h. Additionally, in cancer cells (De Saedeleer et al., 2012; Colegio et al., 2014) and endothelial cells (Sonveaux et al., 2012), lactate induces the activation of hypoxia inducible factor-1 (HIF-1), which is a transcription factor recognized to have an important role in hypoxia and metabolism. Interestingly, HIF-1 has been shown to facilitate lactate transport in cancer cells by upregulating the expression of MCT4 (Ullah et al., 2006).
Together, the above studies indicate that on top of being an energy source, lactate can trigger several tissue-specific mechanisms that lead to the activation of diverse signaling pathways. Because of their location near blood vessels and capillaries (Christov et al., 2007; Verma et al., 2018), SCs may be exposed to high concentrations of lactate. Therefore, in the next section, we will discuss current evidence showing lactate affects SC fate.
Possible Lactate Metabolism Effects on Satellite Cells
The effect of lactate on myogenesis has been studied in vitro and in vivo. In undifferentiated C2C12 myoblasts, culture with lactate and caffeine increased the number of proliferating cells and the number of nuclei per fiber in differentiated myotubes (Oishi et al., 2015). The same study showed that the lactate effect in myoblast differentiation was accompanied by an upregulation of mTOR and P70S6K phosphorylation, suggesting that lactate may work by activating anabolic signals in SCs. Similarly, a more recent study (Ohno et al., 2019) showed that differentiated C2C12 myoblasts cultured in differentiation medium containing lactate increased the size of myotube diameters and the number of nuclei per fiber. Moreover, the study showed that in mouse, oral administration of lactate stimulated muscle hypertrophy and increased the number of Pax7-positive cells, indicating the positive effect of lactate on skeletal muscle regeneration. In contrast, another study reported that lactate promoted the early stages of C2C12 differentiation in a reactive oxygen-dependent manner, but delayed the late stages, as indicated by the number of MHC-positive nuclei (Willkomm et al., 2014).
Another lactate-dependent mechanism that indirectly directs SC fate was reported recently. By using pfkfb3-deficient mice (pfkfb3 codes 6-phosphofructo-2-kinase/fructose-2,6-bisphosphatase 3 protein, which regulates glycolysis), Zhang et al. (2020) found that lactate secreted by endothelial cells was uptaken by and regulates the polarization of macrophages, which improved muscle reperfusion in hindlimb ischemia, and therefore promoted muscle revascularization and regeneration upon SC activation (Figure 1C).
Another mechanism by which lactate has been shown to affect macrophage polarity (which has not been related to muscle regeneration yet) is histone lactylation in which lactate mediates histone modifications (Zhang et al., 2019). That study correlated histone lactylation in macrophages during M1 polarization with the increased expression of genes that are involved in wound healing (i.e., genes that characterize M2 macrophages), suggesting a role in tissue homeostasis. It is unclear whether histone lactylation plays a role in skeletal muscle regeneration or if this histone modification is relevant for SC fate. Future studies should address these biological questions.
Conclusion
Metabolism has been shown to be closely related with SC fate. A switch from fatty acids oxidation to glycolytic metabolism accompanies the transition from quiescent to activated SCs. This metabolic switch is also related to the redox balance produced by epigenetic changes via the NAD+-SIRT1 pathway and ultimately directs the fate of SCs to commit to myogenic differentiation. Lactate affects myoblast differentiation. Considering this, lactate’s close relationship with the redox balance and NAD+/NADH, and lactate’s mediation of histone modifications, the role of lactate in the myogenic differentiation of SCs should be considered in future investigations.
Author Contributions
MN conceptualized the manuscript and wrote the manuscript. MT and ZR proofread the manuscript. All authors contributed to the article and approved the submitted version.
Conflict of Interest
The authors declare that the research was conducted in the absence of any commercial or financial relationships that could be construed as a potential conflict of interest.
Acknowledgments
We would like to thank Peter Karagiannis for proofreading the manuscript. Figures were created with BioRender.com.
References
Asfour, H. A., Allouh, M. Z., and Said, R. S. (2018). Myogenic regulatory factors: the orchestrators of myogenesis after 30 years of discovery. Exp. Biol. Med. 243, 118–128. doi: 10.1177/1535370217749494
Baghdadi, M. B., Firmino, J., Soni, K., Evano, B., Di Girolamo, D., Mourikis, P., et al. (2018). Notch-induced miR-708 antagonizes satellite cell migration and maintains quiescence. Cell Stem Cell 23, 859–868. doi: 10.1016/j.stem.2018.09.017
Baghdadi, M. B., and Tajbakhsh, S. (2018). Regulation and phylogeny of skeletal muscle regeneration. Dev. Biol. 433, 200–209. doi: 10.1016/j.ydbio.2017.07.026
Bentzinger, C. F., Wang, Y. X., and Rudnicki, M. A. (2012). Building muscle: molecular regulation of myogenesis. Cold Spring Harb. Perspect. Biol. 4:a008342. doi: 10.1101/cshperspect.a008342
Bisetto, S., Wright, M. C., Nowak, R. A., Lepore, A. C., Khurana, T. S., Loro, E., et al. (2019). New insights into the lactate shuttle: role of MCT4 in the modulation of the exercise capacity. iScience 22, 507–518. doi: 10.1016/j.isci.2019.11.041
Bonen, A. (2001). The expression of lactate transporters (MCT1 and MCT4) in heart and muscle. Eur. J. Appl. Physiol. 86, 6–11. doi: 10.1007/s004210100516
Bosch-Presegue, L., and Vaquero, A. (2015). Sirtuin-dependent epigenetic regulation in the maintenance of genome integrity. FEBS J. 282, 1745–1767. doi: 10.1111/febs.13053
Brooks, G. A. (2020). Lactate as a fulcrum of metabolism. Redox Biol. 35:101454. doi: 10.1016/j.redox.2020.101454
Canto, C., and Auwerx, J. (2011). Calorie restriction: is AMPK a key sensor and effector? Physiology 26, 214–224. doi: 10.1152/physiol.00010.2011
Cerletti, M., Jang, Y. C., Finley, L. W., Haigis, M. C., and Wagers, A. J. (2012). Short-term calorie restriction enhances skeletal muscle stem cell function. Cell Stem Cell 10, 515–519. doi: 10.1016/j.stem.2012.04.002
Chargé, S. B. P., and Rudnicki, M. A. (2004). Cellular and molecular regulation of muscle regeneration. Physiol. Rev. 84, 209–238. doi: 10.1152/physrev.00019.2003
Chatel, B., Bendahan, D., Hourde, C., Pellerin, L., Lengacher, S., Magistretti, P., et al. (2017). Role of MCT1 and CAII in skeletal muscle pH homeostasis, energetics, and function: in vivo insights from MCT1 haploinsufficient mice. FASEB J. 31, 2562–2575. doi: 10.1096/fj.201601259R
Christov, C., Chrétien, F., Khalil, R. A., Bassez, G., Vallet, G., Authier, F. J., et al. (2007). Muscle satellite cells and endothelial cells: close neighbors and privileged partners. Mol. Biol. Cell 18, 1397–1409. doi: 10.1091/mbc.e06-08-0693
Colegio, O. R., Chu, N. Q., Szabo, A. L., Chu, T., Rhebergen, A. M., Jairam, V., et al. (2014). Functional polarization of tumour-associated macrophages by tumour-derived lactic acid. Nature 513, 559–563. doi: 10.1038/nature13490
Cossu, G., and Tajbakhsh, S. (2007). Oriented cell divisions and muscle satellite cell heterogeneity. Cell 129, 859–861. doi: 10.1016/j.cell.2007.05.029
De Saedeleer, C. J., Copetti, T., Porporato, P. E., Verrax, J., Feron, O., and Sonveaux, P. (2012). Lactate activates HIF-1 in oxidative but not in Warburg-phenotype human tumor cells. PLoS One 7:e46571. doi: 10.1371/journal.pone.0046571
Dell’orso, S., Juan, A. H., Ko, K. D., Naz, F., Perovanovic, J., Gutierrez-Cruz, G., et al. (2019). Single cell analysis of adult mouse skeletal muscle stem cells in homeostatic and regenerative conditions. Development 146:dev174177. doi: 10.1242/dev.174177
Dimmer, K. -S., Friedrich, B., Lang, F., Deitmer, J. W., and Bröer, S. (2000). The low-affinity monocarboxylate transporter MCT4 is adapted to the export of lactate in highly glycolytic cells. Biochem. J. 350, 219–227. doi: 10.1042/bj3500219
Evano, B., and Tajbakhsh, S. (2018). Skeletal muscle stem cells in comfort and stress. NPJ Regen. Med. 3:24. doi: 10.1038/s41536-018-0062-3
Fang, Y., Tang, S., and Li, X. (2019). Sirtuins in metabolic and epigenetic regulation of stem cells. Trends Endocrinol. Metab. 30, 177–188. doi: 10.1016/j.tem.2018.12.002
Fox, J. E. M., Meredith, D., and Halestrap, A. P. (2000). Characterisation of human monocarboxylate transporter 4 substantiates its role in lactic acid efflux from skeletal muscle. J. Physiol. 529, 285–293. doi: 10.1111/j.1469-7793.2000.00285.x
Fukada, S., Uezumi, A., Ikemoto, M., Masuda, S., Segawa, M., Tanimura, N., et al. (2007). Molecular signature of quiescent satellite cells in adult skeletal muscle. Stem Cells 25, 2448–2459. doi: 10.1634/stemcells.2007-0019
Guarente, L. (2011). The logic linking protein acetylation and metabolism. Cell Metab. 14, 151–153. doi: 10.1016/j.cmet.2011.07.007
Halestrap, A. P. (2012). The monocarboxylate transporter family—structure and functional characterization. IUBMB Life 64, 1–9. doi: 10.1002/iub.573
Halestrap, A. P. (2013). The SLC16 gene family - structure, role and regulation in health and disease. Mol. Asp. Med. 34, 337–349. doi: 10.1016/j.mam.2012.05.003
Halestrap, A. P., and Wilson, M. C. (2012). The monocarboxylate transporter family—role and regulation. IUBMB Life 64, 109–119. doi: 10.1002/iub.572
Hardie, D. G., Ross, F. A., and Hawley, S. A. (2012). AMPK: a nutrient and energy sensor that maintains energy homeostasis. Nat. Rev. Mol. Cell Biol. 13, 251–262. doi: 10.1038/nrm3311
Hashimoto, T., Hussien, R., Oommen, S., Gohil, K., and Brooks, G. A. (2007). Lactate sensitive transcription factor network in L6 cells: activation of MCT1 and mitochondrial biogenesis. FASEB J. 21, 2602–2612. doi: 10.1096/fj.07-8174com
Hui, S., Ghergurovich, J. M., Morscher, R. J., Jang, C., Teng, X., Lu, W., et al. (2017). Glucose feeds the TCA cycle via circulating lactate. Nature 551, 115–118. doi: 10.1038/nature24057
Ito, K., and Suda, T. (2014). Metabolic requirements for the maintenance of self-renewing stem cells. Nat. Rev. Mol. Cell Biol. 15, 243–256. doi: 10.1038/nrm3772
Jing, H., and Lin, H. (2015). Sirtuins in epigenetic regulation. Chem. Rev. 115, 2350–2375. doi: 10.1021/cr500457h
Juel, C., and Halestrap, A. P. (1999). Lactate transport in skeletal muscle role and regulation of the monocarboxylate transporter. J. Physiol. 517, 633–642.
Kitaoka, Y., Hoshino, D., and Hatta, H. (2012). Monocarboxylate transporter and lactate metabolism. J. Phys. Fit. Sports Med. 1, 247–252. doi: 10.7600/jpfsm.1.247
Kitaoka, Y., Takeda, K., Tamura, Y., and Hatta, H. (2016). Lactate administration increases mRNA expression of PGC-1alpha and UCP3 in mouse skeletal muscle. Appl. Physiol. Nutr. Metab. 41, 695–698. doi: 10.1139/apnm-2016-0016
Kuang, S., Kuroda, K., Le Grand, F., and Rudnicki, M. A. (2007). Asymmetric self-renewal and commitment of satellite stem cells in muscle. Cell 129, 999–1010. doi: 10.1016/j.cell.2007.03.044
Liu, L., Cheung, T. H., Charville, G. W., Hurgo, B. M., Leavitt, T., Shih, J., et al. (2013). Chromatin modifications as determinants of muscle stem cell quiescence and chronological aging. Cell Rep. 4, 189–204. doi: 10.1016/j.celrep.2013.05.043
Mauro, A. (1961). Satellite cell of skeletal muscle fibers. J. Biophys. Biochem. Cytol. 9, 493–495. doi: 10.1083/jcb.9.2.493
Mccullagh, K. J., Poole, R. C., Halestrap, A. P., Tipton, K. F., O’brien, M., and Bonen, A. (1997). Chronic electrical stimulation increases MCT1 and lactate uptake in red and white skeletal muscle. Am. J. Physiol. Endocrinol. Metab. 273, E239–E246. doi: 10.1152/ajpendo.1997.273.2.E239
Mosienko, V., Teschemacher, A. G., and Kasparov, S. (2015). Is L-lactate a novel signaling molecule in the brain? J. Cereb. Blood Flow Metab. 35, 1069–1075. doi: 10.1038/jcbfm.2015.77
Motohashi, N., and Asakura, A. (2014). Muscle satellite cell heterogeneity and self-renewal. Front. Cell Dev. Biol. 2:1. doi: 10.3389/fcell.2014.00001
Moussaieff, A., Rouleau, M., Kitsberg, D., Cohen, M., Levy, G., Barasch, D., et al. (2015). Glycolysis-mediated changes in acetyl-CoA and histone acetylation control the early differentiation of embryonic stem cells. Cell Metab. 21, 392–402. doi: 10.1016/j.cmet.2015.02.002
Nalbandian, M., Radak, Z., and Takeda, M. (2019). N-acetyl-L-cysteine prevents lactate-mediated PGC1-alpha expression in C2C12 myotubes. Biology 8:44. doi: 10.3390/biology8020044
Nalbandian, M., and Takeda, M. (2016). Lactate as a signaling molecule that regulates exercise-induced adaptations. Biology 5:38. doi: 10.3390/biology5040038
Nederveen, J. P., Joanisse, S., Snijders, T., Ivankovic, V., Baker, S. K., Phillips, S. M., et al. (2016). Skeletal muscle satellite cells are located at a closer proximity to capillaries in healthy young compared with older men. J. Cachexia Sarcopenia Muscle 7, 547–554. doi: 10.1002/jcsm.12105
Ohno, Y., Ando, K., Ito, T., Suda, Y., Matsui, Y., Oyama, A., et al. (2019). Lactate stimulates a potential for hypertrophy and regeneration of mouse skeletal muscle. Nutrients 11:869. doi: 10.3390/nu11040869
Oishi, Y., Tsukamoto, H., Yokokawa, T., Hirotsu, K., Shimazu, M., Uchida, K., et al. (2015). Mixed lactate and caffeine compound increases satellite cell activity and anabolic signals for muscle hypertrophy. J. Appl. Physiol. 118, 742–749. doi: 10.1152/japplphysiol.00054.2014
Pala, F., Di Girolamo, D., Mella, S., Yennek, S., Chatre, L., Ricchetti, M., et al. (2018). Distinct metabolic states govern skeletal muscle stem cell fates during prenatal and postnatal myogenesis. J. Cell Sci. 131:jcs212977. doi: 10.1242/jcs.212977
Perez-Escuredo, J., Van Hee, V. F., Sboarina, M., Falces, J., Payen, V. L., Pellerin, L., et al. (2016). Monocarboxylate transporters in the brain and in cancer. Biochim. Biophys. Acta 1863, 2481–2497. doi: 10.1016/j.bbamcr.2016.03.013
Pilegaard, H., Terzis, G., Halestrap, A., and Juel, C. (1999). Distribution of the lactate/H+ transporter isoforms MCT1 and MCT4 in human skeletal muscle. Am. J. Physiol. Endocrinol. Metab. 276, E843–E848. doi: 10.1152/ajpendo.1999.276.5.E843
Pownall, M. E., Gustafsson, M. K., and Emerson, C. P. Jr. (2002). Myogenic regulatory factors and the specification of muscle progenitors in vertebrate embryos. Annu. Rev. Cell Dev. Biol. 18, 747–783. doi: 10.1146/annurev.cellbio.18.012502.105758
Purohit, G., and Dhawan, J. (2019). Adult muscle stem cells: exploring the links between systemic and cellular metabolism. Front. Cell Dev. Biol. 7:312. doi: 10.3389/fcell.2019.00312
Rocheteau, P., Gayraud-Morel, B., Siegl-Cachedenier, I., Blasco, M. A., and Tajbakhsh, S. (2012). A subpopulation of adult skeletal muscle stem cells retains all template DNA strands after cell division. Cell 148, 112–125. doi: 10.1016/j.cell.2011.11.049
Rodgers, J. T., King, K. Y., Brett, J. O., Cromie, M. J., Charville, G. W., Maguire, K. K., et al. (2014). mTORC1 controls the adaptive transition of quiescent stem cells from G0 to G(Alert). Nature 510, 393–396. doi: 10.1038/nature13255
Ryall, J. G. (2013). Metabolic reprogramming as a novel regulator of skeletal muscle development and regeneration. FEBS J. 280, 4004–4013. doi: 10.1111/febs.12189
Ryall, J. G., Dell’orso, S., Derfoul, A., Juan, A., Zare, H., Feng, X., et al. (2015). The NAD+-dependent SIRT1 deacetylase translates a metabolic switch into regulatory epigenetics in skeletal muscle stem cells. Cell Stem Cell 16, 171–183. doi: 10.1016/j.stem.2014.12.004
Sabari, B. R., Zhang, D., Allis, C. D., and Zhao, Y. (2017). Metabolic regulation of gene expression through histone acylations. Nat. Rev. Mol. Cell Biol. 18, 90–101. doi: 10.1038/nrm.2016.140
Seale, P., Sabourin, L. A., Girgis-Gabardo, A., Mansouri, A., Gruss, P., and Rudnicki, M. A. (2000). Pax7 is required for the specification of myogenic satellite cells. Cell 102, 777–786. doi: 10.1016/S0092-8674(00)00066-0
Shcherbina, A., Larouche, J., Fraczek, P., Yang, B. A., Brown, L. A., Markworth, J. F., et al. (2020). Dissecting murine muscle stem cell aging through regeneration using integrative genomic analysis. Cell Rep. 32:107964. doi: 10.1016/j.celrep.2020.107964
Sonveaux, P., Copetti, T., De Saedeleer, C. J., Vegran, F., Verrax, J., Kennedy, K. M., et al. (2012). Targeting the lactate transporter MCT1 in endothelial cells inhibits lactate-induced HIF-1 activation and tumor angiogenesis. PLoS One 7:e33418. doi: 10.1371/journal.pone.0033418
Strahl, B. D., and Allis, C. D. (2000). The language of covalent histone modifcations. Nature 403, 41–45. doi: 10.1038/47412
Tang, A. H., and Rando, T. A. (2014). Induction of autophagy supports the bioenergetic demands of quiescent muscle stem cell activation. EMBO J. 33, 2782–2797. doi: 10.15252/embj.201488278
Theret, M., Gsaier, L., Schaffer, B., Juban, G., Ben Larbi, S., Weiss-Gayet, M., et al. (2017). AMPKalpha1-LDH pathway regulates muscle stem cell self-renewal by controlling metabolic homeostasis. EMBO J. 36, 1946–1962. doi: 10.15252/embj.201695273
Tsuchiya, Y., Kitajima, Y., Masumoto, H., and Ono, Y. (2020). Damaged myofiber-derived metabolic enzymes act as activators of muscle satellite cells. Stem Cell Rep. 15, 926–940. doi: 10.1016/j.stemcr.2020.08.002
Ullah, M. S., Davies, A. J., and Halestrap, A. P. (2006). The plasma membrane lactate transporter MCT4, but not MCT1, is up-regulated by hypoxia through a HIF-1alpha-dependent mechanism. J. Biol. Chem. 281, 9030–9037. doi: 10.1074/jbc.M511397200
van Hall, G., Stromstad, M., Rasmussen, P., Jans, O., Zaar, M., Gam, C., et al. (2009). Blood lactate is an important energy source for the human brain. J. Cereb. Blood Flow Metab. 29, 1121–1129. doi: 10.1038/jcbfm.2009.35
Verma, M., Asakura, Y., Murakonda, B. S. R., Pengo, T., Latroche, C., Chazaud, B., et al. (2018). Muscle satellite cell cross-talk with a vascular niche maintains quiescence via VEGF and notch signaling. Cell Stem Cell 23, 530–543. doi: 10.1016/j.stem.2018.09.007
Wang, S., Sun, Y., Ren, R., Xie, J., Tian, X., Zhao, S., et al. (2019). H3K27me3 depletion during differentiation promotes myogenic transcription in porcine satellite cells. Gen. Dent. 10:231. doi: 10.3390/genes10030231
Wellen, K. E., Hatzivassiliou, G., Sachdeva, U. M., Bui, T. V., Cross, J. R., and Thompson, C. B. (2009). ATP-citrate lyase links cellular metabolism to histone acetylation. Science 324, 1076–1080. doi: 10.1126/science.1164097
Willkomm, L., Schubert, S., Jung, R., Elsen, M., Borde, J., Gehlert, S., et al. (2014). Lactate regulates myogenesis in C2C12 myoblasts in vitro. Stem Cell Res. 12, 742–753. doi: 10.1016/j.scr.2014.03.004
Yucel, N., Wang, Y. X., Mai, T., Porpiglia, E., Lund, P. J., Markov, G., et al. (2019). Glucose metabolism drives histone acetylation landscape transitions that dictate muscle stem cell function. Cell Rep. 27, 3939–3955. doi: 10.1016/j.celrep.2019.05.092
Zammit, P. S. (2017). Function of the myogenic regulatory factors Myf5, MyoD, Myogenin and MRF4 in skeletal muscle, satellite cells and regenerative myogenesis. Semin. Cell Dev. Biol. 72, 19–32. doi: 10.1016/j.semcdb.2017.11.011
Zhang, D., Tang, Z., Huang, H., Zhou, G., Cui, C., Weng, Y., et al. (2019). Metabolic regulation of gene expression by histone lactylation. Nature 574, 575–580. doi: 10.1038/s41586-019-1678-1
Keywords: lactate, muscle stem cell, metabolism, skeletal muscle, muscle regeneration
Citation: Nalbandian M, Radak Z and Takeda M (2020) Lactate Metabolism and Satellite Cell Fate. Front. Physiol. 11:610983. doi: 10.3389/fphys.2020.610983
Edited by:
Luc Pellerin, University of Poitiers, FranceReviewed by:
Laurent André Messonnier, Université Savoie Mont Blanc, FranceKatrien De Bock, ETH Zürich, Switzerland
Copyright © 2020 Nalbandian, Radak and Takeda. This is an open-access article distributed under the terms of the Creative Commons Attribution License (CC BY). The use, distribution or reproduction in other forums is permitted, provided the original author(s) and the copyright owner(s) are credited and that the original publication in this journal is cited, in accordance with accepted academic practice. No use, distribution or reproduction is permitted which does not comply with these terms.
*Correspondence: Minas Nalbandian, aG0ubmFsYmFuZGlhbkBjaXJhLmt5b3RvLXUuYWMuanA=; Masaki Takeda, bXRha2VkYUBtYWlsLmRvc2hpc2hhLmFjLmpw