- Division of Cell Biology, Institute of Physiology and Cell Biology, University of Veterinary Medicine Hannover, Hannover, Germany
Regeneration after injury is accompanied by transient and lasting changes in the neuroarchitecture of the nervous system and, thus, a form of structural plasticity. In this review, we introduce the olfactory pathway of a particular insect as a convenient model to visualize neural regeneration at an anatomical level and study functional recovery at an electrophysiological level. The olfactory pathway of the locust (Locusta migratoria) is characterized by a multiglomerular innervation of the antennal lobe by olfactory receptor neurons. These olfactory afferents were axotomized by crushing the base of the antenna. The resulting degeneration and regeneration in the antennal lobe could be quantified by size measurements, dye labeling, and immunofluorescence staining of cell surface proteins implicated in axonal guidance during development. Within 3 days post lesion, the antennal lobe volume was reduced by 30% and from then onward regained size back to normal by 2 weeks post injury. The majority of regenerating olfactory receptor axons reinnervated the glomeruli of the antennal lobe. A few regenerating axons project erroneously into the mushroom body on a pathway that is normally chosen by second-order projection neurons. Based on intracellular responses of antennal lobe output neurons to odor stimulation, regenerated fibers establish functional synapses again. Following complete absence after nerve crush, responses to odor stimuli return to control level within 10–14 days. On average, regeneration of afferents, and re-established synaptic connections appear faster in younger fifth instar nymphs than in adults. The initial degeneration of olfactory receptor axons has a trans-synaptic effect on a second order brain center, leading to a transient size reduction of the mushroom body calyx. Odor-evoked oscillating field potentials, absent after nerve crush, were restored in the calyx, indicative of regenerative processes in the network architecture. We conclude that axonal regeneration in the locust olfactory system appears to be possible, precise, and fast, opening an avenue for future mechanistic studies. As a perspective of biomedical importance, the current evidence for nitric oxide/cGMP signaling as positive regulator of axon regeneration in connectives of the ventral nerve cord is considered in light of particular regeneration studies in vertebrate central nervous systems.
Introduction
Central nervous systems are capable to reorganize their anatomical connectivity as a consequence of disruption or manipulation of sensory input. Insects are excellent models for studying such structural plasticity of the nervous system. Insect brains retain plastic capacities during adulthood, such as changes in neuropil volume and alterations in synaptic circuitry (Groh and Meinertzhagen, 2010; Kremer et al., 2010; Sugie et al., 2018). These characteristics support age-dependent, experience-dependent, and behavioral-coupled reorganization of the nervous system (Withers et al., 1993; Heisenberg et al., 1995; Gronenberg et al., 1996; Cayre et al., 2005; Ott and Rogers, 2010; Eriksson et al., 2019). A biomedical aspect relates to the plastic changes occurring in the nervous system after injury. Whereas the regenerative capacity of the central nervous system (CNS) in higher vertebrates is rather low (Ramon y Cajal, 1928; Rossignol et al., 2007), some insects are quite capable of neuronal regeneration both in the peripheral and the CNS (Spira et al., 1987; Stern et al., 1997; Jacobs and Lakes-Harlan, 1999; Pätschke et al., 2004; Ayaz et al., 2008; Stern and Bicker, 2008). Thus, core requirements for regeneration can be analyzed in insect nervous systems without the need to take myelin-associated inhibitory factors such as NOGO-A or MAG (Schwab and Strittmatter, 2014) into consideration, that are characteristics of the mature vertebrate neural tissue.
In the last decade, research into the mechanistics of peripheral nervous system (PNS) regeneration has made some remarkable progress in the genetic model insect, Drosophila melanogaster (reviewed by Brace and DiAntonio, 2017). Since the D. melanogaster larva is translucent, peripheral nerve injury can be delivered by pinching the cuticle and nerve with a sharp pair of forceps, severing both sensory and motor axons. Several studies deal with the ensuing degeneration of the separated distal segment, and molecules interfering with such degeneration such as the ubiquitin ligase, highwire (Xiong et al., 2012) or the NAD+ synthesizing axonal survival factor NMNAT2 (Walker et al., 2017). Some of these factors, e.g., the dual leucine zipper-containing kinase (DLK, Wallenda in D. melanogaster), interact both with distal segment degeneration (Brace et al., 2014) and initiation of regenerative mechanisms, like sprouting, in the proximal segment when activated by experimental elimination of the spectraplakin short stop (Valakh et al., 2013). Nevertheless, motor axons do not successfully navigate across the injury site using the crush paradigm in the D. melanogaster larva (Xiong et al., 2012). A second larval regeneration model in Drosophila melanogaster employs laser transection of dendrites and axons of genetically GFP-labeled sensory dendritic arborization (da) neurons (Song et al., 2012). Using this injury paradigm, the involvement of several cell intrinsic signaling pathways in enhancing regenerative responses to axon injury have been demonstrated: the Pten/Akt pathway (Song et al., 2012), the RNA processing enzyme dRtca (Song et al., 2015), or the CamKII/NOS/PKG pathway activated via the mechanosensitive Ca2+ channel piezo (Song et al., 2019). In summary, interference with those neuron-intrinsic mechanisms led to moderate improvements of incomplete axonal regeneration into the CNS. However, genetic reprogramming of glial metabolism to increased glycolysis could promote both significant da neuron regeneration after laser injury in the ventral nerve cord and restoration of the specific avoidance behavior mediated by these neurons (Li et al., 2020). Less has, so far, been achieved regarding regeneration in the adult Drosophila melanogaster. In the PNS, regeneration of wing vein sensory axons can be studied after laser axotomy. Surprisingly, regeneration is inhibited in this paradigm by the c-Jun N-terminal kinase (JNK) pathway (Soares et al., 2014), which is usually necessary for axon extension during nervous system development in D. melanogaster and elsewhere (Xiong et al., 2012). There is also an example for regeneration in the adult D. melanogaster CNS. In explanted adult Drosophila melanogaster brains, Ayaz et al. (2008) have studied regeneration of a group of identified neurons after microsurgical axotomy. The limited regenerative capacity of these axons was promoted by constitutive activation of protein kinase A, and further enhanced by activation of the JNK pathway in this system. Taken together, promising approaches to elucidate mechanisms of regeneration processes are in progress, but a powerful model for functional regeneration in the adult D. melanogaster brain has yet to be developed.
The Insect Olfactory System
Mature insect olfactory neuropils exhibit a remarkably high degree of structural plasticity associated with specific behaviors (Withers et al., 1993; Heisenberg et al., 1995; Ott and Rogers, 2010; Kraft et al., 2019).
The locust is an accessible animal for the analysis of circuit mechanisms of olfactory coding strategies. Each locust antenna is equipped with 50,000 olfactory receptor neurons (ORNs) whose axons project into the antennal lobe (AL), the primary olfactory center (Ernst et al., 1977; Figure 1). The wiring of the insect AL shares common features with the vertebrate olfactory bulb (Strausfeld and Hildebrand, 1999). As in the olfactory bulb, in the insect AL ORNs synapse with local interneurons and olfactory projection neurons (vertebrates: mitral- and tufted cells) in spherical neuropil compartments termed glomeruli. The axons of the olfactory projection neurons (PNs) exit from the AL and project toward the mushroom body and the lateral horn (Laurent and Naraghi, 1994; Anton and Hansson, 1996; Hansson and Stensmyr, 2011). In the calycal compartment, PNs provide excitatory input to subsets of ca. 50,000 densely packed Kenyon cells, the intrinsic neurons of the mushroom body. Similar to mammals, in most insects each olfactory receptor neuron expresses only a single olfactory receptor gene. The receptor neurons expressing the same olfactory receptor gene converge their axons to the same glomerulus in the AL. Consequently, the number of receptor genes roughly corresponds to the number of glomeruli.
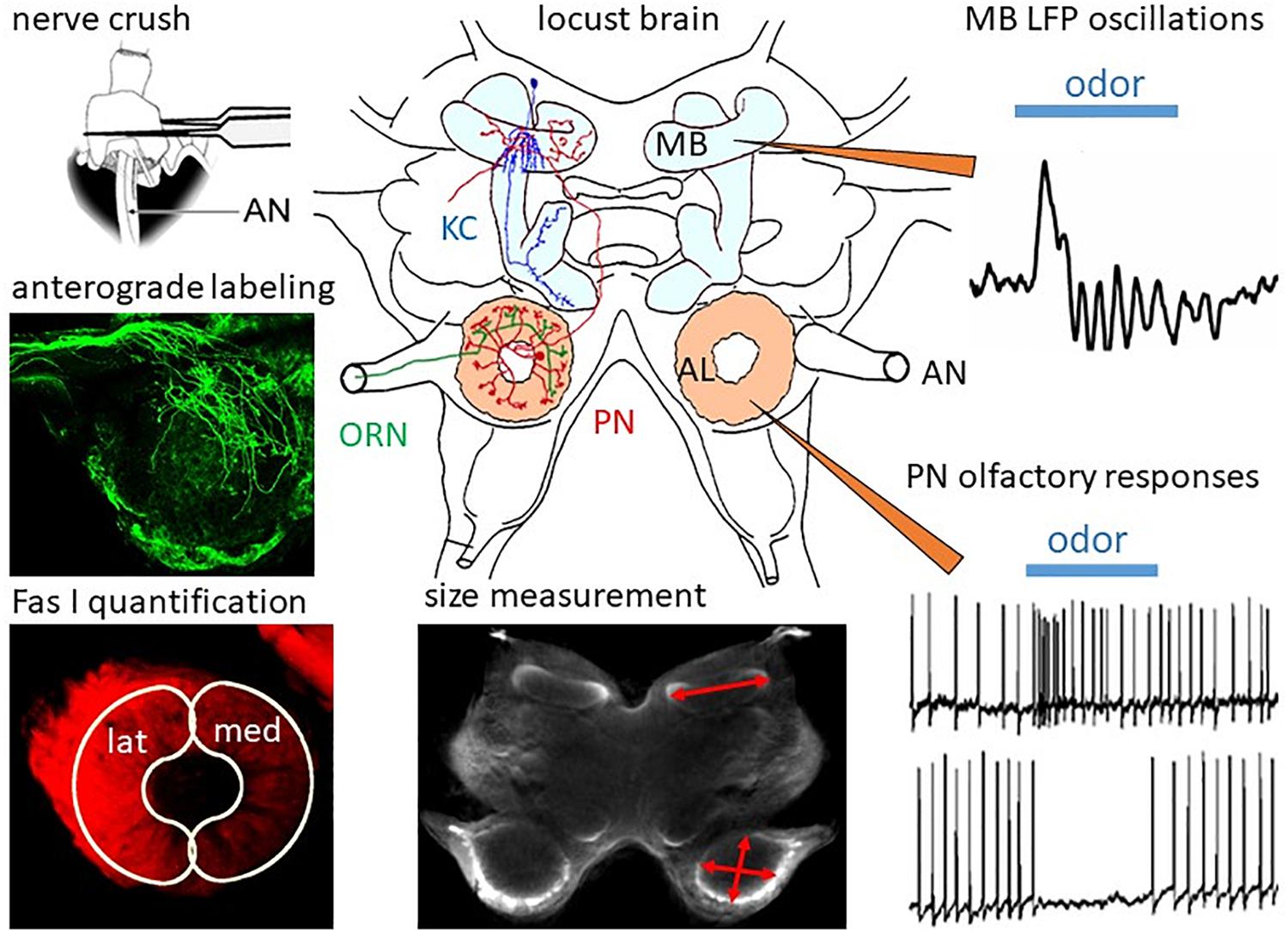
Figure 1. Regeneration in the olfactory system of the locust. In the locust brain, olfactory information from the ORNs projecting through the antennal nerves (AN) is processed in the ALs by local interneurons and PNs, which convey information to the mushroom bodies (MB) containing ca. 50,000 Kenyon cells (KC). After ORN axotomy by crushing the AN, degeneration and subsequent regeneration can be studied by measurement of MB and AL size, by anterograde labeling of ORNs, and by quantification of ORN projections into the lateral (lat) and medial (med) part of the AL by measuring immunofluorescence of their cell surface molecule, Fas I. Functional regeneration can be measured by intracellular recording odor-evoked responses from PNs and by recording of extracellular field potentials in the calyx of the MB. Adapted from Wasser et al. (2017) and Wasser and Stern (2017).
The locust uses a different wiring strategy in its olfactory system. Although the locust genome contains about 174 olfactory receptor genes (142 odorant receptors plus 32 ionotropic receptors, Wang et al., 2015), the AL comprises roughly 1,000 glomeruli (Ernst et al., 1977; Anton and Hansson, 1996). Each ORN axon branches in the AL, innervating multiple glomeruli (Ernst et al., 1977; Anton and Hansson, 1996; Hansson and Stensmyr, 2011). Each of the 830 PNs (Leitch and Laurent, 1996) receives its input from multiple glomeruli and electrophysiological estimates show that each Kenyon cell is contacted by half of the population of PNs (Jortner et al., 2007). This connectivity scheme of the locust olfactory system generates very selective responses by coincidence detection in the Kenyon cells, leading to sparse odor representations in the mushroom body (Jortner et al., 2007).
Since both the antennal sensory neurons and the PNs show a remarkable degree of convergence and also divergence to their postsynaptic partner neurons, one can assume that this type of connectivity pattern is not too rigidly specified. Indeed, in Drosophila melanogaster the synaptic connectivity from PNs to the Kenyon cells appears to be probabilistic (Murthy et al., 2008; Caron et al., 2013). At each larval molt during the hemimetabolous development of the locust, additional segments (annuli) are added to the flagellum of the antenna (Chapman, 2002; Boyan and Ehrhardt, 2020) which, in combination with growth of individual annuli, leads to an increase in the number of sensory neurons. The increasing number of ORN axons has to be incorporated into the already functioning circuitry. This requires some flexible synaptic reorganization, as observed, for instance, in the insect terminal ganglion, where a growing number of wind sensitive afferent connects to a fixed set of giant interneurons during postembryonic development (Chiba et al., 1988; Thompson et al., 1992). In the locust, the postembryonic remodeling of synaptic connectivity of prosternal filiform hair receptors has also been found (Pflüger et al., 1994). It is possible that a similar type of flexibility in the neuroachitecture of the AL, together with a somewhat loosely specified synaptic connectivity of the PNs to the mushroom body might be a helpful prerequisite for regeneration studies in the olfactory system.
Regeneration in the Locust Olfactory System
In the following paragraphs, we will summarize some of the work in our own lab on regeneration in the olfactory system of adult and last larval instar locusts, which has been published in a series of papers (Eickhoff et al., 2012; Stern et al., 2012; Wasser et al., 2017; Wasser and Stern, 2017). The methodology is summarized in Figure 1.
In the locust antenna, ORN axons can be easily axotomized by crushing the scapus at the base of the antenna (Figure 1). The resulting changes in the brain can be quantified by size measurements on sections or, by using scanning laser optical tomography (SLOT, Lorbeer et al., 2011; Bode et al., 2020) in whole brains. The progression of degeneration and regeneration of ORN axons is traced by anterograde dye labeling through the antennal nerve and by quantitative immunofluorescence of the cell surface marker, Fasciclin 1 (Fas I). Functional regeneration is monitored by intracellular recording from PNs postsynaptic to regenerated ORNs and by extracellularly recording oscillating local field potentials from the mushroom body calyx (Figure 1). After AN crushing, the size of the AL is reduced by 30% within 3 days (Stern et al., 2012). Anterograde labeling of the antennal afferents resolves the initial axotomy of the receptor cell axons. By scraping-off tips of olfactory sensilla on single flagellar annuli, only a few receptor cell axons can be dye-labeled (Wasser et al., 2017). This method reveals in finer detail that, similar to Wallerian degeneration in vertebrates (Waller, 1850), the axons distal to the crush degenerate. After 3 days post crushing, single fibers can be resolved which begin to regrow beyond the crush site in the antennal nerve. Reinnervation of the AL commences on 4 days post crush. After 1 week, large numbers of regenerating ORN axons start to grow around the borders of the glomerular neuropil. This leads to an enlargement of the AL, which returns to normal size within 2 weeks (Stern et al., 2012).
Expression of Cell Surface Molecules During Development and Regeneration
Details of the regeneration process in the olfactory neuropils (Stern et al., 2012) were resolved by using monoclonal antibodies against cell surface proteins that were initially discovered as axonal guidance molecules in the ventral nerve cord of the locust embryo. Here, we will briefly describe their distribution pattern during brain development before monitoring their expression during regeneration. Fasciclin I (Fas I) is a neural cell adhesion molecule (Bastiani et al., 1987). After completing half of embryogenesis, antennal pioneer neurons and clusters of mushroom body neuroblasts are labeled by the Fas I antibody (Eickhoff and Bicker, 2012). During further development, the Fas I-immunoreactivity increases in the antennal nerve, AL neuropil, and a growing number of Kenyon cells. Fas I expression persists in ALs and mushroom bodies of larval and adult brains. An ortholog of the guidance molecule semaphorin 1a (Sema 1a), originally discovered by its function in pioneer axon navigation (Kolodkin et al., 1992; Isbister et al., 1999), contributes to the sorting of ORNs to different glomeruli in the developing AL of Drosophila melanogaster (Komiyama et al., 2007). During embryonic development of the locust, this transmembrane glycoprotein is also transiently expressed in ORNs and all olfactory neuropils (Eickhoff and Bicker, 2012). Another useful developmental marker is lachesin, a GPI-linked protein of the immunoglobulin family, that is expressed on the surface of developing locust neurons (Karlstrom et al., 1993; Boyan and Ehrhardt, 2020), including ingrowing larval ORN axons (Eickhoff and Bicker, 2012).
After experimental injury of the antennal nerve, Fas I expression fades in the AL neuropil until 4 days post crush, but remains unaltered in the mushroom bodies (Stern et al., 2012). Subsequently, Fas I-positive ORN axons begin to reinnervate the glomeruli of the AL. Twenty-one days post operation, the immunofluorescence intensity of glomerular staining becomes almost identical on treated and control sides. Regenerating fibers transiently express lachesin, indicative of a recapitulation of developmental programs. By day 21 post crush, lachesin labeling in the antennal nerve and lobe has completely vanished. Sema 1a expression shows that the neuropil of the AL retains its basic glomerular structure during deafferentation and regeneration. The immunofluorescence of this marker is rather uniformly distributed over the glomeruli of the AL and persists after the operation (Stern et al., 2012). Thus, the neuroanatomical branching pattern of PNs and local interneurons of the AL appears rather undamaged. Due to the conserved glomerular architecture during degeneration, the AL size reduction seems to be mainly caused by disintegration of the distal segments of ORNs.
Structural Brain Plasticity After Sensory Deprivation
Does deafferentation of antennal input only affect the volume of ALs or also the target area of the PNs in the mushroom body? The consequences of nerve crush and complete antennal ablation on AL and mushroom body size were analyzed on days 7, 14, and 21 post operation (Eickhoff et al., 2012). Whereas after crush, ALs shrink within the first week, and grow back to normal size after that (Figure 2), they continue shrinking after antennal ablation, losing as much as 60% of their initial volume within 21 days. Measuring the calyx diameter revealed shrinkage to 86% of the original size within 21 days after antennal ablation. After nerve crush, a slight but significant decline in calyx diameter (95% of the control) is detectable after 7 days, but no significant size differences to controls remain after 14 and 21 days (Eickhoff et al., 2012; Figure 2). This result suggests the occurrence of regenerative processes within the olfactory pathway at later stages. To summarize, sensory deprivation of the primary olfactory center causes shrinkage of the mushroom body calyx, a transsynaptically induced structural change in a second order information processing neuropil.
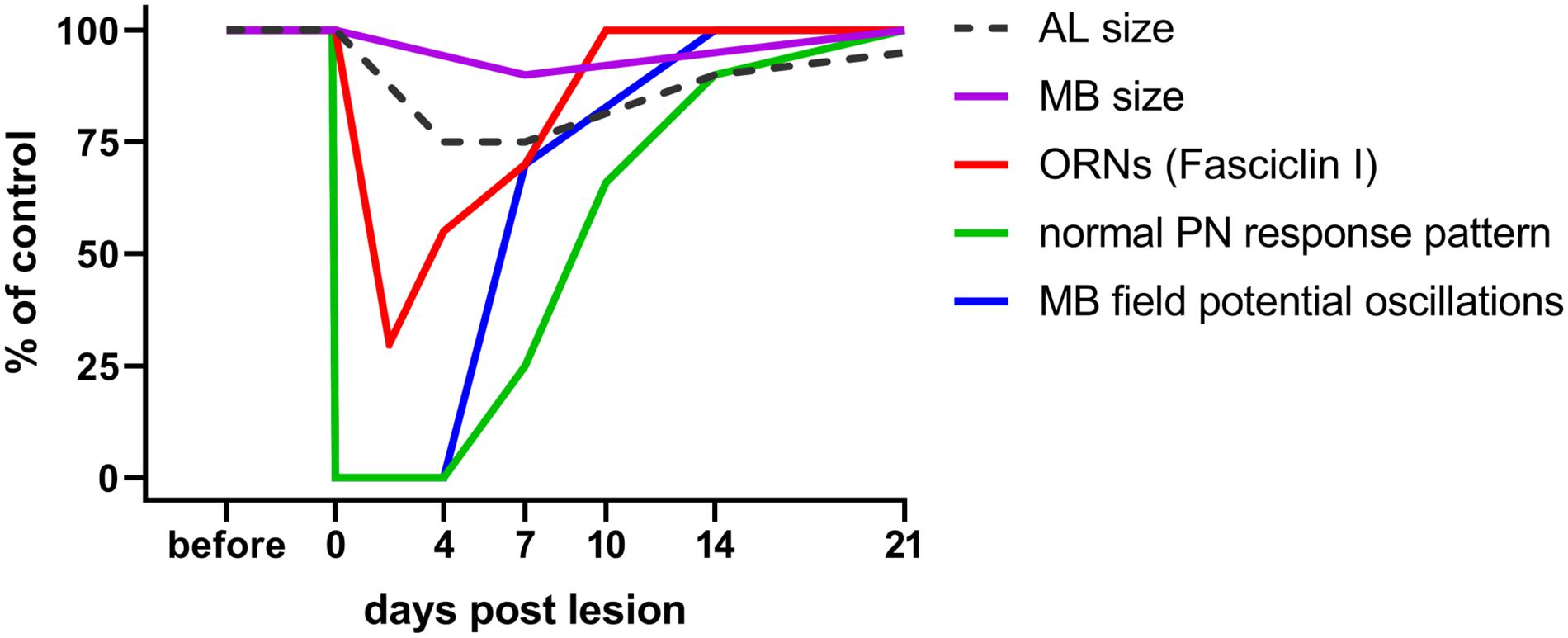
Figure 2. Time course of plastic changes in the adult locust olfactory system after lesion. Values are expressed as percentage of the unlesioned situation (before). On the neuroanatomical level, neuropil volumes and the cell surface protein fasciclin I indicative for ORN terminals in the AL decrease within the first 4 days post lesion, and increase back to normal within 21 days. On the electrophysiological level, responses are completely absent directly after the lesion, begin to return after 4 days, and return back to normal within 21 days as well. For technical reasons in a session of intracellular recordings from AL projection neurons, the chance to hit one of the very few (out of 830) cells with regenerated input is rather low. The mushroom body, however, integrates over all PNs. Thus, the chance to detect a regenerated input is much higher, explaining earlier re-appearance of mushroom body signals. Data from Eickhoff et al. (2012); Wasser et al. (2017), and Wasser and Stern (2017).
Precision and Misrouting of Regenerating Fibers
The olfactory pathway of the locust is capable of fast regeneration on a structural level, both at the first order neuropil of the AL and the second order neuropil of mushroom body. How precise is the process of AL re-innervation? Anterograde labeling of a small number of afferents on a single antennal annulus reveals a weak topographic match between ORN origin on the antenna and the position of innervated glomeruli (Wasser et al., 2017; Figure 3). Fibers originating distally on the antenna tend to innervate more peripherally located glomeruli, whereas projections from proximal parts of the antenna are more or less evenly distributed over the AL (Wasser et al., 2017). This pattern is most likely due to the sequence of postembryonic antennal development and growth. The first instar locust hatches with 11 antennal annuli, whereas the adult bears 21 of them. Not only the number of annuli but also their size increases between molts and the number of olfactory sensilla increases alongside (Ochieng et al., 1998). Between larval molts, proximal annuli divide, annuli in the middle of the antenna grow in size, and most distal annuli do not change much (Chapman and Greenwood, 1986; Chapman, 2002). Thus, many new ORNs are generated on proximal, some on medial, and hardly any on the most distal annuli. At the same time, number and size of AL glomeruli increase, with many new glomeruli arising in the center of the AL, and fewer intercalating between existing microglomeruli (Anton et al., 2002). Therefore, most of the oldest glomeruli innervated mostly by ORNs originating distally on the antenna end up in the periphery of the AL, whereas younger glomeruli, innervated by ORNs from the proximal part are rather evenly scattered over the AL neuropil (Figure 3). This antennotopic pattern is, however, less clearly defined than e.g., the topographic representation of mechanosensory afferents in the locust thoracic ganglion (Newland, 1991), and whether it has any functional significance is not clear. Anton and Hansson (1996) reported no correlation between PN response characteristics and the distance of the arborizations from the central fiber core. In preparations with lesioned nerves, the antennotopic arborization patterns of ORNs did not reappear (Wasser et al., 2017; Figure 3). Nevertheless, the vast majority of ORNs terminated in the AL, which is quite unusual. Generally, pathfinding errors are very common for regenerating axons (Chiba and Murphey, 1991; Lakes and Kalmring, 1991; Lakes-Harlan and Pfahlert, 1995; Stern et al., 1997; Stern and Bicker, 2008; Krüger and Lakes-Harlan, 2010). For instance, in the regenerating auditory pathway of locusts, even as many as 70% of the regenerated fibers are misrouted (Jacobs and Lakes-Harlan, 2000). Thus, the precise termination of most regenerating ORN axons in the AL is an exception. A few axons (up to 15) fail to terminate in the AL, however, they leave the AL via the antennal lobe tract (ALT), arborizing in the mushroom body calyx and the lateral horn, areas that are normally innervated by the PN axons running in this tract (Figure 3). Rössler et al. (1999) have described such a misrouting of antennal afferents during pupal development of the hawk moth Manduca with experimentally damaged sorting zone glia. However, fibers had also grown incorrectly into other brain regions under their experimental circumstances, whereas misrouted regenerating locust ORN axon were confined to components of the olfactory pathway (Stern et al., 2012). One explanation could be that the ALT is the only exit from the AL, which is, like the ALT itself, enveloped by glial processes (Hähnlein et al., 1996). Possibly, the rather unique multiglomerular architecture of the acridid Locusta AL as compared to other, even closely related insects (Ignell et al., 2001), enhanced the chance for successful functional regeneration.
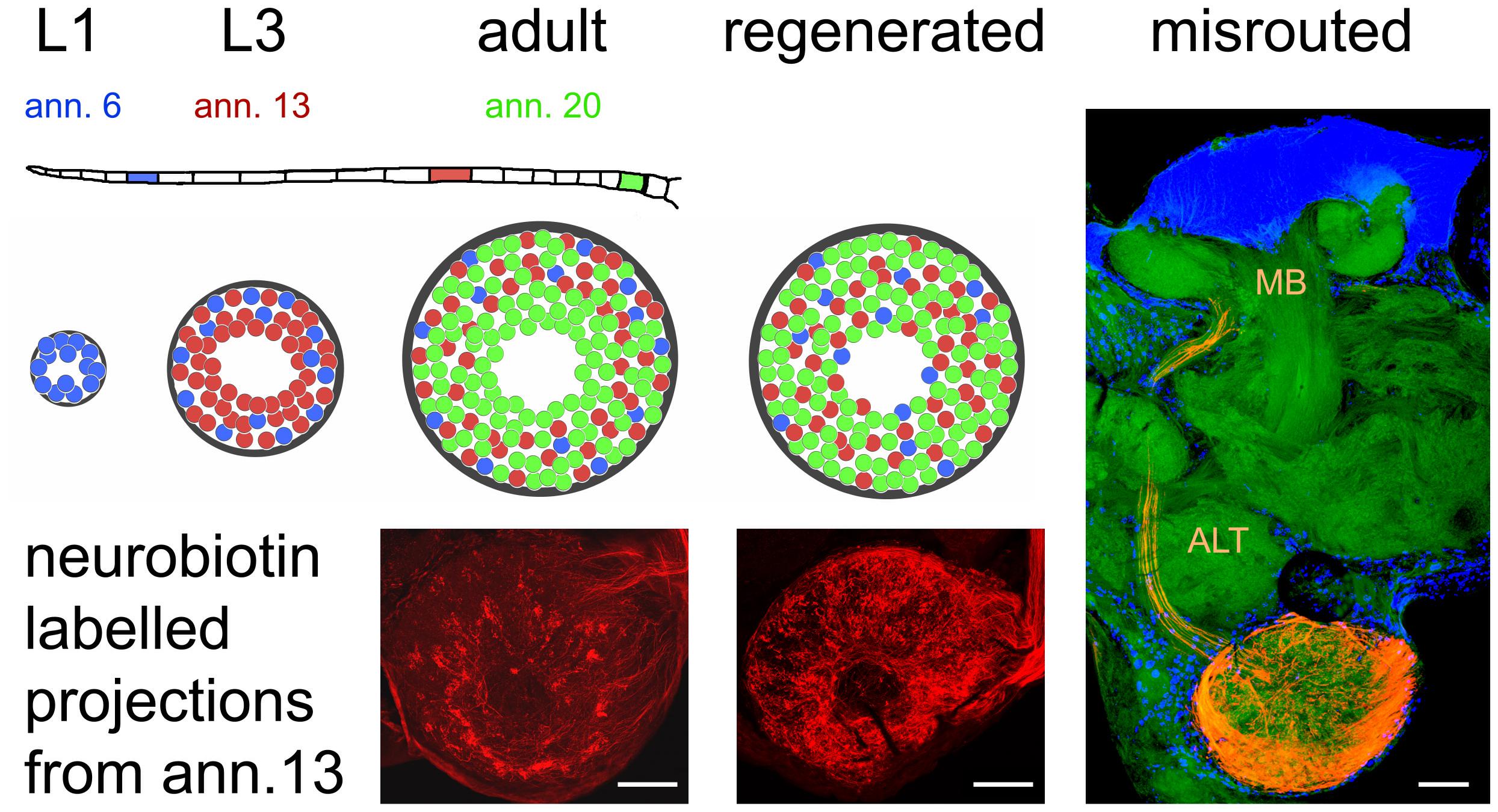
Figure 3. Organization of olfactory receptor neuron projections into the antennal lobe during development and regeneration. The flagellum of the locust antenna consists of 21 rings or annuli (ann.), which increase in number and size during postembryonic development through five larval stages (L1, L3 as examples) till adulthood. Receptor neurons terminate in a distributed fashion in the available glomeruli of the antennal lobe, which also gains size during development. This leads to a weak topographic pattern where older neurons born in younger stages (L1, blue) tend to terminate in the periphery, younger neurons born later (L3, red) terminate peripherally and medially, and terminals of younger neurons born just before the final molt (adult, green) are evenly distributed all over the antennal lobe. After regeneration, this pattern is absent. The photomicrographs of the neurobiotin-labeled sections correspond to the red glomeruli in the schematic. Only a small fraction of receptor neurons (∼15 axons when labeling the complete antennal nerve) fail to terminate in the antennal lobe and are misrouted through the antennal lobe tract (ALT) to the mushroom body (MB). Photomicrographs from Wasser et al. (2017). Scale bars 100 μm.
Physiological Proof of Regenerating Synapses
Successful regeneration requires not only the re-innervation of the correct target but also recovery of physiological function. The olfactory system of the locust is quite amenable to studies of functional recovery by electrophysiological recording of field potentials and intracellular recordings at the level of antennal receptor cells, AL neurons, and mushroom body neurons (Laurent and Naraghi, 1994; Anton and Hansson, 1996). When recording from PNs in the ALs of axotomized locusts, the first few odor stimulus evoked postsynaptic responses from regenerated ORNs occurred from days 4 to 7 post crush on, matching well to the observations of regeneration on the neuroanatomical level (Figure 2). The proportions of response categories (excitatory vs. inhibitory) changed during regeneration, but were back to normal within 21 days (Wasser and Stern, 2017; Figure 2). Odor-evoked oscillating extracellular local field potentials (LFP) were recorded in the mushroom body (Figure 1). These responses, absent after antennal nerve crush, reappeared, in a few animals as soon as 4 days post crush. Odor-induced oscillation patterns were restored within 7 days post crush (Figure 2). Both intra- and extracellular recordings indicate the capability of the locust olfactory system to re-establish synaptic contacts in the AL after antennal nerve lesion. Thus, electrophysiological analysis at the systems level shows plastic recovery of synaptic circuitry.
Behavioral studies of odor discrimination after regeneration are still lacking. Whether the animals could reacquire the lost sense of smell, and discriminate odors again, could only be tested by behavioral choice experiments as recently developed by Simoes et al. (2011).
The Influence of Age on Regeneration
In the vertebrate CNS, age is an important factor that determines the extent and speed of regeneration after injury. Of course, we wondered also about the precision and age-dependence of the regeneration process in the locust olfactory system. Both ORN distal segment degeneration in the AL and regeneration of ORN axons measured by FAS I-immunofluorescence were significantly faster in 5th instar nymphs than in adults (Wasser et al., 2017). Accordingly, re-establishment of ORN-PN synapses was significantly quicker in nymphs than in adults. Finally, the restoration of the AL network, judged by correct ratios of inhibitory to excitatory inputs to the PN population, was also faster in 5th instars than in adults (Wasser and Stern, 2017). Faster or more effective regeneration has also been demonstrated in other sensory pathways in insect preparations, e.g., the auditory nerve of locusts (Lakes et al., 1990) and bush crickets (Krüger et al., 2011a,b), as well as the peripheral nerve of cockroach (Guthrie, 1962). The shorter distance in the slightly smaller 5th instar can only partly explain faster regeneration. An explanation could be that cell intrinsic factors limit the regeneration capabilities of older neurons as observed in the mammalian spinal cord (Blackmore and Letourneau, 2006). However, we found no evidence for limited regeneration of the older ORNs originating on the peripheral antennal annuli as compared to younger, proximal ORNs. A different explanation could be that the subadult antennal system is still in a state of growth and rearrangement that may be more permissive for ORN regeneration and synapse formation than the adult AL. In the silk moth and in Drosophila melanogaster, molting hormones (20-HE) in the hemolymph have been shown to enhance neurite outgrowth both in vitro (Kraft et al., 1998) and in vivo (Zwart et al., 2013).
Mechanistic Approaches to Regeneration
We have introduced the olfactory pathway of the locust Locusta migratoria as a model system for neural regeneration demonstrating that axonal regeneration in this system is possible and beyond that, fast and precise (Stern et al., 2012). Differences between experimental groups based on age can be quantified on the neuroanatomical and physiological level (Wasser et al., 2017; Wasser and Stern, 2017). Is it possible to manipulate the regeneration process in a way that function of the neural circuitry can be more efficiently restored? Currently it is too early to provide a conclusive answer, but some results from other labs and our own are outlined. A convenient way to manipulate regeneration in the locust are gain-of-function experiments in which drugs or natural ligands are applied during regeneration. After injection into the animals or bath application to cell cultures, neuronal responses can be quantified. For example, addition of 9-cis retinoic acid to dissociated cell cultures of embryonic locusts causes the extension of more neurites per neuron (Sukiban et al., 2014). Application of recombinant human erythropoetin facilitates the regeneration of neurites in dissociated locust brain neurons and, using a behavioral assay with the grasshopper Chorthippus biguttulus, accelerates reestablishment of sound source localization after unilateral crush injury of the peripheral tympanic nerve (Ostrowski et al., 2011).
During embryonic development, the nitric oxide/cGMP system facilitates axon extension in antennal pioneer neurons (Seidel and Bicker, 2000) and the migration of enteric neurons on the midgut (Haase and Bicker, 2003; Stern et al., 2007; Stern and Bicker, 2010). In both cases, nitric oxide induces specific neurons of the PNS to raise the intracellular cGMP level, which in turn causes a reorganization of the cytoskeleton leading to increased cell motility. Based on these findings, axonal regeneration in the CNS of locust embryos was also addressed in a culture system for an embryo preparation in which the CNS is exposed to chemical compounds targeting the NO/cGMP system and the connectives between abdominal ganglia were crushed (Stern and Bicker, 2008). To analyze the axonal response to injury, changes in the anatomy of serotonergic multisegmental interneurons by immunocytochemistry after crushing ventral nerve cord connectives were followed. After initial retraction, serotonergic axons grow back into the adjacent ganglion within a few days. Regeneration rate is improved by NO donors and reduced by inhibitors of the NO-cGMP pathway. Since the regenerating serotonergic neurons express NO-induced cGMP-immunoreactivity, they are presumably the targets of NO signaling (Stern and Bicker, 2008). Most likely, axons of nitrergic neurons damaged by the crush procedure are a source of internal NO. After crushing of connectives, the accumulation of citrulline, a by-product of NO synthesis, is visualized in nitrergic neurons by immunocytochemistry (Stern et al., 2010). In the presence of NO-scavengers, we find reduced regeneration of injured axons, suggesting that NO from internal sources enhances the regeneration process (Stern and Bicker, 2008). In the nervous system of the locust, the AL contains the highest amount of Ca2+/calmodulin-dependent NO synthase activity (Müller and Bicker, 1994; Elphick et al., 1995). The source of NO is a group of nitrergic interneurons which innervate all glomeruli. It might be rewarding to find out whether the functional regeneration of the insect olfactory pathway could also be improved by NO donors or other external stimulators of the NO/cGMP cascade.
Outlook
Does our research on an invertebrate animal have implications for neuroregenerative therapies in human patients? A particular challenge provides spinal cord injury, in which the non-permissive tissue environment and neuron intrinsic factors restrict the regeneration of injured axons. In the mammalian nervous system, the limited regenerative response to spinal cord injury is usually considered to involve a different cyclic nucleotide pathway, the cAMP/protein kinase A signaling cascade (Hannila and Filbin, 2008). Along similar lines, an investigation of human model neurons in cell culture confirmed that neurite outgrowth is indeed enhanced by activating cAMP/Protein kinase A signal transduction (Tegenge et al., 2011). These results argue against excessive generalization of concepts derived from phylogenetically very distant animals. However, it is well known that even in the vertebrate CNS, growth responses of neurites depend on the species, specific part of the brain, and neuron type. In lower vertebrates, such as fish and amphibians, the regeneration capacity of the CNS is certainly higher than in mammals, which cannot regenerate injured axons (Ramon y Cajal, 1928; Rossignol et al., 2007).
For example, the olfactory system of the African clawed frog Xenopus provides ample opportunities for studying mechanisms of regeneration (Yoshino and Tochinai, 2006). Here, neurogenesis during development and a lifelong neuronal turnover in the olfactory epithelium are essential contributions to the regenerative capabilities of the olfactory circuitry. Transsection of the fully functional olfactory nerve of Xenopus larvae causes degeneration of ORN in the sensory epithelium which is followed by cell death in the postsynaptic olfactory bulb and reduction of its volume (Hawkins et al., 2017). The neural injury induces an increase in epithelial stem cell proliferation and newly formed ORN start to reinnervate the olfactory bulb within 1 week. Olfactory network reconstruction, as assayed by cellular calcium imaging in the olfactory bulb, appears to recover within 7 weeks (Hawkins et al., 2017).
Another striking example is the regeneration of the amphibian optic nerve after injury, which led to intriguing experimental studies culminating in the chemoaffinity hypothesis of Sperry (1963). This hypothesis proposed that developing neurons systematically acquire chemical identification tags, by which they selectively recognize their correct targets. Optic nerve injury in the goldfish causes induction of the neuronal NO synthase enzyme (Koriyama et al., 2009) and the resulting NO release promotes neurite outgrowth similar to locust ventral nerve cord regeneration. Again, axonal regeneration of the fish retinal ganglion cell axons is significantly enhanced by pharmacological activators of NO/cGMP signaling (Koriyama et al., 2009). Downstream targets of NO/cGMP signaling during neuroregeneration have to be identified, but there are likely candidates. The second messenger cyclic GMP can activate protein kinase G (PKG) which is also implicated in the regeneration of retinal ganglion cell axons (Koriyama et al., 2009). During mouse development, NO and cGMP positively regulate neurite length and migration of immature neurons derived from the medial ganglionic eminence (Mandal et al., 2013). An increase in cellular motility is mediated by PKG which, in turn, downregulates the RhoA signaling pathway and eventually is thought to affect the cytoskeleton via myosin light chain phosphatase (Mandal et al., 2013). The RhoA pathway is one of the key elements responsible for the restricted regeneration capacity of the mammalian CNS (Mueller et al., 2005).
In the mammalian CNS, extracellular growth inhibiting factors bind to several specific receptors on the axonal growth cone and converge on the small GTPase RhoA signaling pathway (Tönges et al., 2011). In the active state, RhoA interacts with downstream effector proteins such as Rho-associated coiled coil forming protein serine/threonine kinase (ROCK), thereby leading to the phosphorylation of various target proteins which regulate mainly actin cytoskeletal dynamics (Mueller et al., 2005). Experimental studies in rodents show that inhibition of RhoA-ROCK overcomes the inhibitory effects of myelin and chondroitin sulfate proteoglycans, resulting in increased axonal regeneration in cell culture and improved fiber sprouting across the lesion site (Dergham et al., 2002; Fournier et al., 2003; Lingor et al., 2007). High concentrations of the analgesic, Ibuprofen decrease RhoA activation and promote neurite growth in cell cultures of human model neurons (Roloff et al., 2015). Inhibition of the downstream effector ROCK by the drugs Y-27632 and Fasudil enhances the neurite growth capacity (Roloff et al., 2015). In line with these encouraging results, the inhibitory effects of the pesticide rotenone on axon extension of locust pioneer neurons can be rescued by co-application of the ROCK inhibitor Y-27632 (Bergmann et al., 2019). Since neurite extension is one of the essential components of regeneration, experimental results from mammals and the locust suggest that molecules of the RhoA/ROCK signaling cascade are useful targets for identification of pharmaceutical compounds with therapeutic benefit for the treatment of spinal cord injury.
Author Contributions
GB and MS wrote the manuscript. Both authors agreed to be accountable for the content of the work.
Funding
Our research has been supported by grants from the German Research Council (Deutsche Forschungsgemeinschaft) and the German Federal Ministry of Education and Research (BMBF).
Conflict of Interest
The authors declare that the research was conducted in the absence of any commercial or financial relationships that could be construed as a potential conflict of interest.
Acknowledgments
We like to thank members of our lab for their contributions to the cited publications.
References
Anton, S., and Hansson, B. S. (1996). Antennal lobe interneurons in the desert locust Schistocerca gregaria (Forskal): processing of aggregation pheromones in adult males and females. J. Comp. Neurol. 370, 85–96.
Anton, S., Ignell, R., and Hansson, B. S. (2002). Developmental changes in the structure and function of the central olfactory system in gregarious and solitary desert locusts. Mic. Res. Tech. 56, 281–291. doi: 10.1159/000047222
Ayaz, D., Leyssen, M., Koch, M., Yan, J., Srahna, M., Sheeba, V., et al. (2008). Axonal injury and regeneration in the adult brain of Drosophila. J. Neurosci. 28, 6010–6021. doi: 10.1523/JNEUROSCI.0101-08.2008
Bastiani, M. J., Harrelson, A. L., Snow, P. M., and Goodman, C. S. (1987). Expression of fasciclin I and II glycoproteins on subsets of axon pathways during neuronal development in the grasshopper. Cell 48, 745–755. doi: 10.1016/0092-8674(87)90072-9
Bergmann, G. A., Frömbling, S., Joseph, N., Bode, K., Bicker, G., and Stern, M. (2019). An intact insect embryo for developmental neurotoxicity testing of directed axonal elongation. ALTEX 36, 643–649. doi: 10.14573/altex.1901292
Blackmore, M., and Letourneau, P. C. (2006). Changes within maturing neurons limit axonal regeneration in the developing spinal cord. J. Neurobiol. 66, 348–360. doi: 10.1002/neu.20224
Bode, K., Nolte, L., Kamin, H., Desens, M., Ulmann, A., Bergmann, G. A., et al. (2020). Scanning laser optical tomography resolves developmental neurotoxic effects on pioneer neurons. Sci. Rep. 10:2641. doi: 10.1038/s41598-020-59562-7
Boyan, G., and Ehrhardt, E. (2020). Epithelial domains and the primordial antennal nervous system of the embryonic grasshopper Schistocerca gregaria. Invert. Neurosci. 20:6. doi: 10.1007/s10158-020-0240-z
Brace, E. J., and DiAntonio, A. (2017). Models of axon regeneration in Drosophila. Exp. Neurol. 287, 310–317. doi: 10.1016/j.expneurol.2016.03.014
Brace, E. J., Wu, C., Valakh, V., and DiAntonio, A. (2014). SkpA restrains synaptic terminal growth during development and promotes axonal degeneration following injury. J. Neurosci. 34, 8398–8410. doi: 10.1523/JNEUROSCI.4715-13.2014
Caron, S. J. C., Ruta, V., Abbott, L. F., and Axel, R. (2013). Random convergence of olfactory inputs in the Drosophila mushroom body. Nature 497, 113–117. doi: 10.1038/nature12063
Cayre, M., Malaterre, J., Scotto-Lomassese, S., Holstein, G. R., Martinelli, G. P., Forni, C., et al. (2005). A role for nitric oxide in sensory-induced neurogenesis in an adult insect brain. Eur. J. Neurosci. 21, 2893–2902. doi: 10.1111/j.1460-9568.2005.04153.x
Chapman, R. F. (2002). Development of phenotypic differences in sensillum populations on the antennae of a grasshopper, Schistocerca americana. J. Morphol. 254, 186–194. doi: 10.1002/jmor.10026
Chapman, R. F., and Greenwood, M. (1986). Changes in distribution and abundance of antennal sensilla during growth of Locusta migratoria L. (Orthoptera: Acrididae). Int. J. Insect Morphol. Embryol. 15, 83–96.
Chiba, A., and Murphey, R. K. (1991). Connectivity of identified central synapses in the cricket is normal following regeneration and blockade of presynaptic activity. J. Neurobiol. 22, 130–142. doi: 10.1002/neu.480220204
Chiba, A., Shepherd, D., and Murphey, R. K. (1988). Synaptic rearrangement during postembryonic development in the cricket. Science 240, 901–905. doi: 10.1126/science.3363372
Dergham, P., Ellezam, B., Essagian, C., Avedissian, H., Lubell, W. D., and McKerracher, L. (2002). Rho signaling pathway targeted to promote spinal cord repair. J. Neurosci. 22, 6570–6577.
Eickhoff, R., and Bicker, G. (2012). Developmental expression of cell recognition molecules in the mushroom body and antennal lobe of the locust Locusta migratoria. J. Comp. Neurol. 520, 2021–2040. doi: 10.1002/cne.23026
Eickhoff, R., Lorbeer, R. A., Scheiblich, H., Heisterkamp, A., Meyer, H., Stern, M., et al. (2012). Scanning laser optical tomography resolves structural plasticity during regeneration in an insect brain. PLoS One 7:e41236. doi: 10.1371/journal.pone.0041236
Elphick, M. R., Rayne, R. C., Riveros-Moreno, V., Moncada, S., and O’Shea, M. (1995). Nitric oxide synthesis in locust olfactory interneurons. J. Exp. Biol. 198, 821–829.
Eriksson, M., Nylin, S., and Carlsson, M. A. (2019). Insect brain plasticity: effects of olfactory input on neuropil size. R. Soc. Open Sci. 6:190875. doi: 10.1098/rsos.190875
Ernst, K. D., Boeckh, J., and Boeckh, V. (1977). A neuroanatomical study on the organization of the central antennal pathways in insects. Z. Zellforsch. 176, 285–306.
Fournier, A. E., Takizawa, B. T., and Strittmatter, S. M. (2003). Rho kinase inhibition enhances axonal regeneration in the injured CNS. J. Neurosci. 23, 1416–1423.
Groh, C., and Meinertzhagen, I. A. (2010). Brain plasticity in Diptera and Hymenoptera. Front. Biosci. 2, 268–288.
Gronenberg, W., Heeren, S., and Hölldobler, B. (1996). Age-dependent and task-related morphological changes in the brain and mushroom bodies of the ant Camponotus floridanus. J. Exp. Biol. 199, 2011–2019.
Haase, A., and Bicker, G. (2003). Nitric oxide and cyclic nucleotides are regulators of neuronal migration in an insect embryo. Development 130, 3977–3987. doi: 10.1242/dev.00612
Hähnlein, I., Härtig, W., and Bicker, G. (1996). Datura stramonium lectin staining of glial associated extracellular material in insect brains. J. Comp. Neurol. 376, 175–187.
Hannila, S. S., and Filbin, M. T. (2008). The role of cyclic AMP signaling in promoting axonal regeneration after spinal cord injury. Exp. Neurol. 209, 321–332. doi: 10.1016/j.expneurol.2007.06.020
Hansson, B. S., and Stensmyr, M. C. (2011). Evolution of insect olfaction. Neuron 72, 698–711. doi: 10.1016/j.neuron.2011.11.003
Hawkins, S. J., Weiss, L., Offner, T., Dittrich, K., Hassenklöver, T., and Manzini, I. (2017). Functional reintegration of sensory neurons and transitional dendritic reduction of Mitral/tufted cells during injury-induced recovery of the larval xenopus olfactory circuit. Front. Cell. Neurosci. 11:380. doi: 10.3389/fncel.2017.00380
Heisenberg, M., Heusipp, M., and Wanke, C. (1995). Structural plasticity in the Drosophila brain. J. Neurosci. 15, 1951–1960. doi: 10.1523/JNEUROSCI.15-03-01951.1995
Ignell, R., Anton, S., and Hansson, B. S. (2001). The antennal lobe of orthoptera - anatomy and evolution. Brain Behav. Evol. 57, 1–17. doi: 10.1159/000047222
Isbister, C. M., Tsai, A., Wong, S. T., Kolodkin, A. L., and O’Connor, T. P. (1999). Discrete roles for secreted and transmembrane semaphorins in neuronal growth cone guidance in vivo. Development 126, 2007–2019.
Jacobs, K., and Lakes-Harlan, R. (1999). Axonal degeneration within the tympanal nerve of Schistocerca gregaria. Cell Tissue Res. 298, 167–178. doi: 10.1007/s004419900056
Jacobs, K., and Lakes-Harlan, R. (2000). Pathfinding, target recognition, and synapse formation of single regenerating fibers in the adult grasshopper Schistocerca gregaria. J. Neurobiol. 42, 394–409.
Jortner, R. A., Farivar, S. S., and Laurent, G. (2007). A simple connectivity scheme for sparse coding in an olfactory system. J. Neurosci. 27, 1659–1669. doi: 10.1523/JNEUROSCI.4171-06.2007
Karlstrom, R. O., Wilder, L. P., and Bastiani, M. J. (1993). Lachesin: an immunoglobulin superfamily protein whose expression correlates with neurogenesis in grasshopper embryos. Development 118, 509–522.
Kolodkin, A. L., Matthes, D. J., O’Connor, T. P., Patel, N. H., Admon, A., Bentley, D., et al. (1992). Fasciclin IV: sequence, expression, and function during growth cone guidance in the grasshopper embryo. Neuron 5, 831–845. doi: 10.1016/0896-6273(92)90237-8
Komiyama, T., Sweeney, L. B., Schuldiner, O., Garcia, K. C., and Luo, L. (2007). Graded expression of semaphorin-1a cell-autonomously directs dendritic targeting of olfactory projection neurons. Cell 128, 399–410. doi: 10.1016/j.cell.2006.12.028
Koriyama, Y., Yasuda, R., Homma, K., Mawatari, K., Nagashima, M., Sugitani, K., et al. (2009). Nitric oxide-cGMP signaling regulates axonal elongation during optic nerve regeneration in the goldfish in vitro and in vivo. J. Neurochem. 110, 890–901. doi: 10.1111/j.1471-4159.2009.06182.x
Kraft, N., Spaethe, J., Rössler, W., and Groh, C. (2019). Neuronal plasticity in the mushroom-body calyx of bumble bee workers during early adult development. Dev. Neurobiol. 79, 287–302. doi: 10.1002/dneu.22678
Kraft, R., Levine, R. B., and Restifo, L. L. (1998). The steroid hormone 20-hydroxyecdysone enhances neurite growth of drosophila mushroom body neurons isolated during metamorphosis. J. Neurosci. 18, 8886–8899. doi: 10.1523/JNEUROSCI.18-21-08886.1998
Kremer, M. C., Christiansen, F., Leiss, F., Paehler, M., Knapek, S., Andlauer, T. F. M., et al. (2010). Structural long-term changes at mushroom body input synapses. Curr. Biol. 20, 1938–1944. doi: 10.1016/j.cub.2010.09.060
Krüger, S., Butler, C. S., and Lakes-Harlan, R. (2011a). Morphological and physiological regeneration in the auditory system of adult Mecopoda elongata (Orthoptera: Tettigoniidae). J. Comp. Physiol. A 197, 181–192. doi: 10.1007/s00359-010-0598-1
Krüger, S., Haller, B., and Lakes-Harlan, R. (2011b). Regeneration in the auditory system of nymphal and adult bush crickets Tettigonia viridissima. Physiol. Entomol. 36, 235–246. doi: 10.1111/j.1365-3032.2011.00789.x
Krüger, S., and Lakes-Harlan, R. (2010). Changes in the auditory neuropil after deafferentation in adult grasshoppers (Schistocerca gregaria). Arthropod Struct. Dev. 39, 26–32. doi: 10.1016/j.asd.2009.10.006
Lakes, R., and Kalmring, K. (1991). Regeneration of the projection and synaptic connections of tympanic receptor fibers of Locusta migratoria (Orthoptera) after Axotomy. J. Neurobiol. 22, 169–181. doi: 10.1002/neu.480220207
Lakes, R., Kalmring, K., and Engelhardt, K. H. (1990). Changes in the auditory system of locusts (Locusta migratoria and Schistocerca gregaria) after deafferentation. J. Comp. Physiol. A 166, 553–563. doi: 10.1007/BF00192026
Lakes-Harlan, R., and Pfahlert, C. (1995). Regeneration of axotomized tympanal nerve fibers in the adult grasshopper Chorthippus biguttulus (L.) (Orthoptera: Acrididae) correlates with regaining the localization ability. J. Comp. Physiol. A 176, 797–807. doi: 10.1007/BF00192627
Laurent, G., and Naraghi, M. (1994). Odorant-induced oscillations in the mushroom bodies of the locust. J. Neurosci. 14, 2993–3004. doi: 10.1523/JNEUROSCI.14-05-02993.1994
Leitch, B., and Laurent, G. (1996). GABAergic synapses in the antenna1 lobe and mushroom body of the locust olfactory system. J. Comp. Neurol. 372, 487–514.
Li, F., Sami, A., Noristani, H. N., Slattery, K., Qiu, J., Groves, T., et al. (2020). Glial metabolic rewiring promotes axon regeneration and functional recovery in the central nervous system. Cell Metab. 32, 767–785.e7. doi: 10.1016/j.cmet.2020.08.015
Lingor, P., Teusch, N., Schwarz, K., Mueller, R., Mack, H., Bähr, M., et al. (2007). Inhibition of Rho kinase (ROCK) increases neurite outgrowth on chondroitin sulphate proteoglycan in vitro and axonal regeneration in the adult optic nerve in vivo. J. Neurochem. 103, 181–189. doi: 10.1111/j.1471-4159.2007.04756.x
Lorbeer, R. A., Heidrich, M., Lorbeer, C., Ramirez Ojeda, D. F., Bicker, G., Meyer, H., et al. (2011). Highly efficient 3D fluorescence microscopy with a scanning laser optical tomograph. Opt. Express 19, 5419–5430. doi: 10.1364/OE.19.005419
Mandal, S., Stanco, A., Buys, E. S., Enikolopov, G., and Rubenstein, J. L. R. (2013). Soluble guanylate cyclase generation of cGMP regulates migration of MGE neurons. J. Neurosci. 33, 16897–16914. doi: 10.1523/JNEUROSCI.1871-13.2013
Mueller, B. K., Mack, H., and Teusch, N. (2005). Rho kinase, a promising drug target for neurological disorders. Nat. Rev. Drug Discov. 4, 387–398. doi: 10.1038/nrd1719
Müller, U., and Bicker, G. (1994). Calcium-activated release of nitric oxide and cellular distribution of nitric oxide-synthesizing neurons in the nervous system of the locust. J. Neurosci. 74, 7521–7528. doi: 10.1523/JNEUROSCI.14-12-07521.1994
Murthy, M., Fiete, I., and Laurent, G. (2008). Testing odor response stereotypy in the Drosophila mushroom body. Neuron 59, 1009–1023. doi: 10.1016/j.neuron.2008.07.040
Newland, P. L. (1991). Morphology and somatotopic organisation of the central projections of afferents from tactile hairs on the hind leg of the locust. J. Comp. Neurol. 312, 493–508. doi: 10.1002/cne.903120402
Ochieng, S. A., Hallberg, E., and Hansson, B. S. (1998). Fine structure and distribution of antennal sensilla of the desert locust, Schistocerca gregaria (Orthoptera: Acrididae). Cell Tissue Res. 291, 525–536. doi: 10.1007/s004410051022
Ostrowski, D., Ehrenreich, H., and Heinrich, R. (2011). Erythropoietin promotes survival and regeneration of insect neurons in vivo and in vitro. Neuroscience 188, 95–108. doi: 10.1016/j.neuroscience.2011.05.018
Ott, S. R., and Rogers, S. M. (2010). Gregarious desert locusts have substantially larger brains with altered proportions compared with the solitarious phase. Proc. Biol. Sci. 22, 3087–3096. doi: 10.1098/rspb.2010.0694
Pätschke, A., Bicker, G., and Stern, M. (2004). Regeneration of proctolinergic neurons in the ventral nerve cord of the locust. Dev. Brain Res. 150, 73–76. doi: 10.1016/j.devbrainres.2004.02.006
Pflüger, H. J., Hurdelbrink, S., Czjzek, A., and Burrows, M. J. (1994). Activity-dependent structural dynamics of insect sensory fibers. J. Neurosci. 14, 6946–6955. doi: 10.1523/JNEUROSCI.14-11-06946.1994
Ramon y Cajal, S. (1928). Degeneration and Regeneration of the Nervous System. New York, NY: Hafner.
Roloff, F., Scheiblich, H., Dewitz, C., Dempewolf, S., Stern, M., and Bicker, G. (2015). Enhanced neurite outgrowth of human model (NT2) neurons by small-molecule inhibitors of Rho/ROCK signaling. PLoS One 10:e0118536. doi: 10.1371/journal.pone.0118536
Rossignol, S., Schwab, M., Schwartz, M., and Fehlings, M. G. (2007). Spinal cord injury: Time to move? J. Neurosci. 27, 11782–11792. doi: 10.1523/JNEUROSCI.3444-07.2007
Rössler, W., Oland, L. A., Higgins, M. R., Hildebrand, J. G., and Tolbert, L. P. (1999). Development of a glia-rich axon-sorting zone in the olfactory pathway of the moth Manduca sexta. J. Neurosci. 19, 9865–9877. doi: 10.1523/JNEUROSCI.19-22-09865.1999
Schwab, M. E., and Strittmatter, S. M. (2014). Nogo limits neural plasticity and recovery from injury. Curr. Opin. Neurobiol. 27, 53–60. doi: 10.1016/j.conb.2014.02.011
Seidel, C., and Bicker, G. (2000). Nitric oxide and cGMP influence axonogenesis of antennal pioneer neurons. Development 127, 4541–4549.
Simoes, P. M., Ott, S. R., and Niven, J. E. (2011). Associative olfactory learning in the desert locust, Schistocerca gregaria. J. Exp. Biol. 214, 2495–2503. doi: 10.1242/jeb.055806
Soares, L., Parisi, M., and Bonini, N. M. (2014). Axon injury and regeneration in the adult Drosophila. Sci. Rep. 4:6199. doi: 10.1038/srep06199
Song, Y., Li, D., Farrelly, O., Miles, L., Li, F., Kim, S. E., et al. (2019). The mechanosensitive ion channel Piezo inhibits axon regeneration. Neuron 102, 373–389.e6. doi: 10.1016/j.neuron.2019.01.050
Song, Y., Ori-McKenney, K. M., Zheng, Y., Han, C., Jan, L. Y., and Jan, Y. N. (2012). Regeneration of Drosophila sensory neuron axons and dendrites is regulated by the Akt pathway involving Pten and microRNA bantam. Genes Dev. 26, 1612–1625. doi: 10.1101/gad.193243.112
Song, Y., Sretavan, D., Salegio, E. A., Berg, J., Huang, X., Cheng, T., et al. (2015). Regulation of axon regeneration by the RNA repair/splicing pathway. Nat. Neurosci. 18, 817–825. doi: 10.1038/nn.4019
Sperry, R. W. (1963). Chemoaffinity in the orderly growth of nerve fiber patterns and connections. Proc. Natl. Acad. Sci. U.S.A. 50, 703–710. doi: 10.1073/pnas.50.4.703
Spira, M. E., Zeldes, D., Hochner, B., and Dormann, A. (1987). The effects of microenvironment on the redifferentiation of regenerating neurones: neurite architecture, acetylcholine receptors and Ca2+ channel distribution. J. Exp. Biol. 132, 111–131.
Stern, M., and Bicker, G. (2008). Nitric oxide regulates axonal regeneration in an insect embryonic CNS. Dev. Neurobiol. 68, 295–308. doi: 10.1002/dneu.20585
Stern, M., and Bicker, G. (2010). NO as a regulator of neuronal motility and regeneration in the locust nervous system. J. Insect Physiol. 56, 958–965. doi: 10.1016/j.jinsphys.2010.03.031
Stern, M., Böger, N., Eickhoff, R., Kerßen, U., Lorbeer, C., Ziegler, M., et al. (2010). Development of nitrergic neurons in the nervous system of the locust embryo. J. Comp. Neurol. 518, 1157–1175. doi: 10.1002/cne.22303
Stern, M., Ediger, V. L., Gibbon, C. R., Blagburn, J. M., and Bacon, J. P. (1997). Regeneration of cercal filiform hair sensory neurons in the first instar cockroach restores escape behaviour. J. Neurobiol. 33, 439–458.
Stern, M., Knipp, S., and Bicker, G. (2007). Embryonic differentiation of serotonin-containing neurons in the enteric nervous system of the locust (Locusta migratoria). J. Comp. Neurol. 501, 38–51. doi: 10.1002/cne.21235
Stern, M., Scheiblich, H., Eickhoff, R., Didwischus, N., and Bicker, G. (2012). Regeneration of olfactory afferent axons in the locust brain. J. Comp. Neurol. 520, 679–693. doi: 10.1002/cne.22770
Strausfeld, N. J., and Hildebrand, J. G. (1999). Olfactory systems: Common design, uncommon origins? Curr. Opin. Neurobiol. 9, 634–639. doi: 10.1016/S0959-4388(99)00019-7
Sugie, A., Marchetti, G., and Tavosanis, G. (2018). Structural aspects of plasticity in the nervous system of Drosophila. Neural Dev. 13:14. doi: 10.1186/s13064-018-0111-z
Sukiban, J., Bräunig, P., Mey, J., and Bui-Göbbels, K. (2014). Retinoic acid as a survival factor in neuronal development of the grasshopper, Locusta migratoria. Cell Tissue Res. 358, 303–312. doi: 10.1007/s00441-014-1957-y
Tegenge, M. A., Roloff, F., and Bicker, G. (2011). Rapid differentiation of human embryonal carcinoma stem cells (NT2) into neurons for neurite outgrowth analysis. Cell. Mol. Neurobiol. 31, 635–643.
Thompson, K. S., Blagburn, J. M., Gibbon, C. R., and Bacon, J. P. (1992). Correlation of filiform hair position with sensory afferent morphology and synaptic connections in the second instar cockroach. J. Comp. Neurol. 320, 213–227. doi: 10.1002/cne.903200206
Tönges, L., Koch, J. C., Bähr, M., and Lingor, P. (2011). ROCKing regeneration: Rho kinase inhibition as molecular target for neurorestoration. Front. Mol. Neurosci. 4:39. doi: 10.3389/fnmol.2011.00039
Valakh, V., Walker, L. J., Skeath, J. B., and DiAntonio, A. (2013). Loss of the spectraplakin short stop activates the DLK injury response pathway in Drosophila. J. Neurosci. 33, 17863–17873. doi: 10.1523/JNEUROSCI.2196-13.2013
Walker, L. J., Summers, D. W., Sasaki, Y., Brace, E. J., Milbrandt, J., and DiAntonio, A. (2017). MAPK signaling promotes axonal degeneration by speeding the turnover of the axonal maintenance factor NMNAT2. eLife 6:e22540. doi: 10.7554/eLife.22540
Waller, A. (1850). Experiments on the section of glossopharyngeal and hypoglossal nerves of the frog and observations of the alternatives produced thereby in the structure of their primitive fibres. Philos. Trans. R. Soc. Lond. B 140, 423–429.
Wang, Z., Yang, P., Chen, D., Jiang, F., Li, Y., Wang, X., et al. (2015). Identification and functional analysis of olfactory receptor family reveal unusual characteristics of the olfactory system in the migratory locust. Cell. Mol. Life Sci. 72, 4429–4443. doi: 10.1007/s00018-015-2009-9
Wasser, H., Biller, A., Antonopoulos, G., Meyer, H., Bicker, G., and Stern, M. (2017). Regeneration of axotomized olfactory neurons in young and adult locusts quantified by fasciclin I immunofluorescence. Cell Tissue Res. 386, 1–12. doi: 10.1007/s00441-016-2560-1
Wasser, H., and Stern, M. (2017). Regeneration of synapses in the olfactory pathway of locusts after antennal deafferentation. J. Comp. Physiol. A 203, 867–877. doi: 10.1007/s00359-017-1199-z
Withers, G. S., Fahrbach, S. E., and Robinson, G. E. (1993). Selective neuroanatomical plasticity and division of labour in the honeybee. Nature 364, 238–240. doi: 10.1038/364238a0
Xiong, X., Hao, Y., Sun, K., Li, J., Li, X., Mishra, B., et al. (2012). The Highwire ubiquitin ligase promotes axonal degeneration by tuning levels of Nmnat protein. PLoS Biol. 10:e1001440. doi: 10.1371/journal.pbio.1001440
Yoshino, J., and Tochinai, S. (2006). Functional regeneration of the olfactory bulb requires reconnection to the olfactory nerve in Xenopus larvae. Dev. Growth Differ. 48, 15–24. doi: 10.1111/j.1440-169X.2006.00840.x
Keywords: antennal lobe, mushroom body, fasciclin I, semaphorin 1a, field potential oscillations
Citation: Bicker G and Stern M (2020) Structural and Functional Plasticity in the Regenerating Olfactory System of the Migratory Locust. Front. Physiol. 11:608661. doi: 10.3389/fphys.2020.608661
Received: 21 September 2020; Accepted: 12 November 2020;
Published: 03 December 2020.
Edited by:
Wolfgang Rössler, Julius Maximilian University of Würzburg, GermanyReviewed by:
Hong Lei, Arizona State University, United StatesReinhard Lakes-Harlan, University of Giessen, Germany
Copyright © 2020 Bicker and Stern. This is an open-access article distributed under the terms of the Creative Commons Attribution License (CC BY). The use, distribution or reproduction in other forums is permitted, provided the original author(s) and the copyright owner(s) are credited and that the original publication in this journal is cited, in accordance with accepted academic practice. No use, distribution or reproduction is permitted which does not comply with these terms.
*Correspondence: Gerd Bicker, Z2VyZC5iaWNrZXJAdGloby1oYW5ub3Zlci5kZQ==; orcid.org/0000-0003-3158-067X; Michael Stern, TWljaGFlbC5zdGVybkB0aWhvLWhhbm5vdmVyLmRl; orcid.org/0000-0002-5088-5439
†These authors have contributed equally to this work