- 1Key Laboratory for Genetic Breeding of Aquatic Animals and Aquaculture Biology, Freshwater Fisheries Research Center, Chinese Academy of Fishery Sciences, Wuxi, China
- 2Wuxi Fisheries College, Nanjing Agricultural University, Wuxi, China
- 3Tongwei Co., Ltd., Chengdu, China
- 4Healthy Aquaculture Key Laboratory of Sichuan Province, Sichuan, China
Facilitative glucose transporter 4 (GLUT4) plays a central role in mediating insulin function to increase glucose uptake in glucose metabolism homeostasis. In this study, the function and localization of GLUT4 in blunt snout bream (Megalobrama amblycephala) were first investigated, and then, the response measured as carbohydrate level, was analyzed. The results showed that the cDNA sequence of GLUT4 in blunt snout bream (MaGLUT4, GenBank accession no: MT447093) was 2868 bp in length, and the corresponding mRNA contained a 5′-UTR region of 513 bp and a 3′-UTR region of 837 bp. MaGLUT4 had an open reading frame of 1518 bp and was encoded by 505 amino acids. Its theoretical isoelectric point and molecular weight was 6.41 and 55.47 kDa, respectively. A comparison of these characteristics with BLASTP results from the NCBI database showed that MaGLUT4 had the highest homology with Cypriniformes fish, with MaGLUT4 and GLUT4 of other Cypriniformes clustered in the phylogenetic tree with other GLUT1–4 amino acid sequences. Compared with the results from the homo_sapiens and mus_musculus data sets, some mutations were observed in the GLUT4 amino acid sequence of these aquatic animals, including an FQQI mutation to FQQL, LL mutation to MM, and TELEY mutation to TELDY. MaGLUT4 was constitutively expressed in the muscle, intestine, and liver, with the highest mRNA level observed in muscle. Furthermore, the predicted tertiary structure and results of immunohistochemical staining showed that MaGLUT4 was a transmembrane protein primarily located in the plasma membrane, where it accounts for 60.9% of the total expressed, according to an analysis of subcellular localization. Blood glucose level peaked within 1 h, and the insulin level peaked at 6 h, while the mRNA and protein levels of GLUT4 showed an upward trend with an increase in feeding time and decreased sharply after 12 h. These results confirmed that MaGLUT4 was mainly distributed in muscles and crosses the cell membrane. The changes in the insulin, mRNA, and protein levels of MaGUT4 lagged far behind changes in blood glucose levels. This delay in insulin level changes and GLUT4 activation might be the important reasons for glucose intolerance of this fish species.
Introduction
In aquatic animals, carbohydrates are widely used in feed as energy sources. On the one hand, carbohydrates are more readily available and much less expensive than protein and can substitute for protein to prevent the catabolism of expensive protein nutrients to meet energy needs as well as reducing ammonia nitrogen emissions (Honorato et al., 2010; Kamalam et al., 2017). On the other hand, carbohydrates are also effective binding agents during the pelletization process (Arnesen and Krogdahl, 1993). As reported, some fish species, such as Atlantic salmon (Salmo salar) (Hemre and Hansen, 1998), grass carp (Ctenopharyngodon idella) (Gao et al., 2010), rainbow trout (Oncorhynchus mykiss) (Jin et al., 2014), and large yellow croaker (Larimichthys crocea) (Zhou et al., 2016), are capable of utilizing digestible carbohydrates to some extent. However, compared to mammals, high levels of carbohydrates in feed often lead to a reduction in fish growth and prolonged hyperglycemia, similar to type 2 diabetes (Wilson and Poe, 1987; Shi, 1997). The proportion of carbohydrates added to feed is usually low which has restricted the development of aquaculture. To address this problem, researchers have focused on the regulatory mechanism of glycometabolism in aquatic animals (Dalziel et al., 2018; Lu et al., 2018). Unfortunately, the regulatory mechanism of fish is not yet understood. Glucose is a polar molecule that relies on a transporter for transmembrane transport from the outer membrane to the inner membrane (Brant et al., 1993), and studies have shown that the family of specific facilitative glucose transporters (GLUTs) can mediate the absorption and distribution of glucose (Duehlmeier et al., 2007). The first rate-limiting step of these transporters is based on the diffusion gradient of glucose across cell membranes (Rose and Richer, 2005; Lu et al., 2018). Hence, the functional study of GLUTs continues to receive a great deal of attention (Barron et al., 2016).
In 1988, insulin-sensitive glucose transporter 4 (GLUT4) was identified for the first time in the study of insulin regulation of glucose metabolism (James et al., 1988). Thereafter, GLUT4 has been cloned in humans (Fukatmoto et al., 1989), rats (Charon et al., 1989), and mice (Kaestner et al., 1989). Initially, Wright et al. (1998) reported that GLUT4 was absent in teleosts fish, but this observation was due to lack of specific antibodies used for mammalian GLUT4 protein. Later, the presence of GLUT4 was demonstrated in brown trout (Salmo trutta) in 2000 (Planas et al., 2000), which proved that the presence of GLUT4 and paved the new way that glucose homeostasis is possibly regulated by the regulation of GLUT4 in fish. Specifically, it has been proven that GLUT4 is the most abundant glucose transporter in the skeletal muscle of mammals accounting for approximately 70% of the basal glucose transport, compared to the level reported in other tissues (Slot et al., 1991; Becker et al., 2001; Zorzano et al., 2005). In mammals, GLUT4 plays a central role in mediating insulin function to increase glucose uptake in the system of glucose metabolism homeostasis (Díaz et al., 2007; Marín-Juez et al., 2014). However, only a few studies have reported the role of GLUT4 in fish (Planas et al., 2000; Capilla et al., 2004a; Hall et al., 2006), besides, the mechanism is unclear and needs to be further investigated.
Blunt snout bream (Megalobrama amblycephala), a herbivorous freshwater fish widely found in China, Africa, North America, and Eurasia, has many advantages such as good meat quality and high economic value (Zhou et al., 2008). In China, the annual production output of this species reached nearly 800,000 tons in 2018 (Ministry of Agriculture, and Rural Affairs of the People’s Republic of China, Chinese Fisheries Yearbook, 2019). Like other fish species, blunt snout bream has limited ability to utilize carbohydrates, and adding high levels of carbohydrates in the feed may lower their growth performance and disturb glucose metabolism outcomes accompanied by persistent hyperglycemia (Ren et al., 2015). Unfortunately, to date, the reason for the carbohydrate intolerance in fish is not well understood. Moreover, information regarding the functional analysis and localization of GLUT4, as well as the mechanisms through which glucose levels are maintained in plasma remains unclear. Therefore, this study aimed to clone, identify the function of GLUT4, and to investigate its expression in response to carbohydrate fed to blunt snout bream.
Materials and Methods
Ethics Statement
The handling of the juveniles was in accordance with the Ministry of Agriculture, China, and international animal welfare laws, guidelines, and policies (Food and Agriculture Organization [FAO], 2004) and was approved by the Institutional Animal Care and Ethics Committee of Nanjing Agricultural University, Nanjing, China [Permit number: SYXK (Su) 2011-0036].
Experimental Diets
Table 1 showed the various raw ingredients of feed used in the present study, and the diets were formulated to contain 30% glucose level. All the ingredients shown in Table 1 were ground into powder and sieved using a 40 mesh sieve. About 10% amount of water and 6% amount of oil were added to produce a stiff dough which was then forced through a granulator [F-26 (II), South China University of Technology, China] to make pellets. The pelleted diets were dried in a drying oven at 65°C. Finally, the pellets were stored at −20°C in sealed and well labeled plastic bags until required for use.
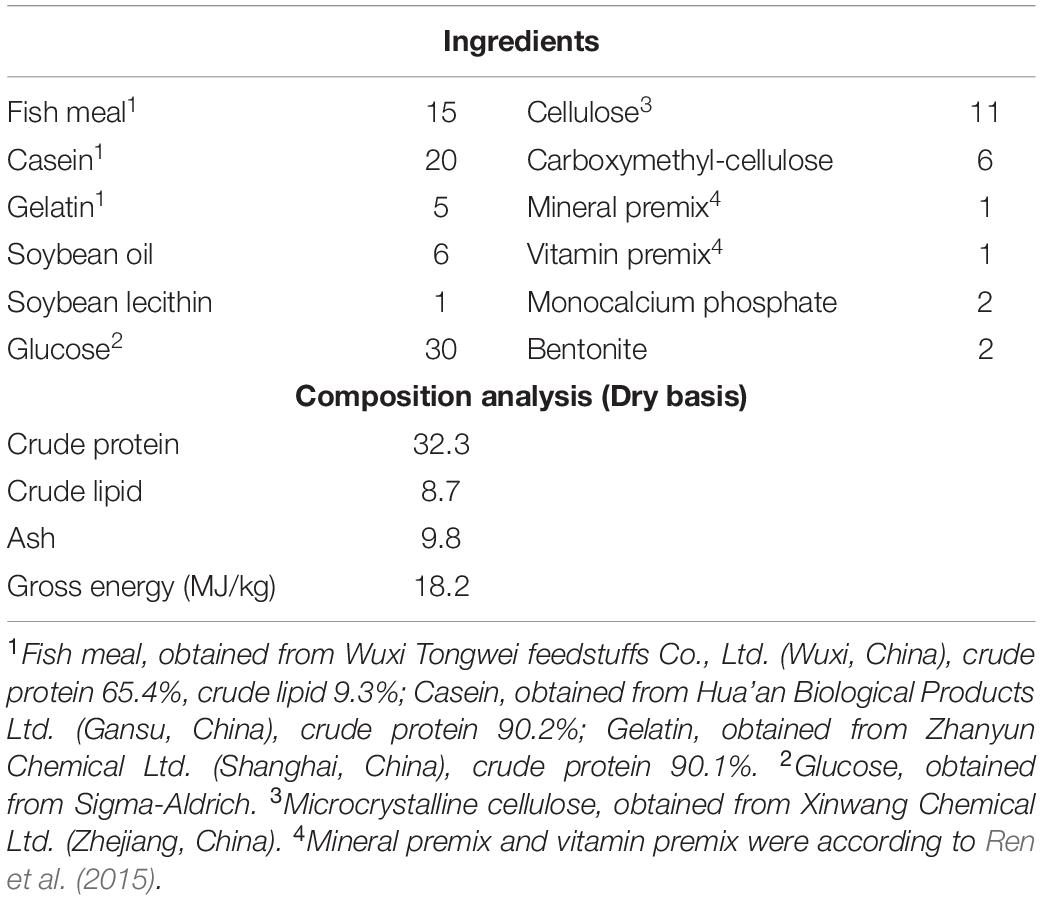
Table 1. Formulation and proximate composition of experimental diet for glucose feeding trial (% dry basis).
Experimental Procedure
Healthy juveniles were selected from the Freshwater Fisheries Research Center (FFRC) of the Chinese Academy of Fishery Sciences breeding center. Before the experiment, the fish were acclimatized to experimental conditions in a water recirculatory system in cylindrical fiberglass tanks (300 L) for 15 days and fed with commercial diets containing 30.8% crude protein, 8.7% crude lipid, and 18.9% carbohydrate levels (Wuxi Tongwei feedstuffs Co., Ltd., China) to apparent satiation three times per day. At the end of the acclimatization period, 20 apparently healthy juvenile fish (initial weight 29.13 ± 0.21 g) were randomly distributed into every tank in triplicates, after which the feeding trial started, three times (08:00, 12:00, and 16:00) to apparent satiation based on the visual observation of fish feeding behavior. Throughout the experimental period, the water quality was tested once a week, the water temperature was maintained 28.13 ± 0.21°C, the pH at 7.22 ± 0.14, ammonia nitrogen values at 0.026 ± 0.002 mg/L, dissolved oxygen values at 6.43 ± 0.30 mg/L. A controlled photoperiod 12:12 h (light:dark) was maintained during the entire experiment.
Sample Collection
After 63 days feeding trial, the fish (three fish per fiberglass tank) were randomly selected from each tank at different time points (1, 6, 12, and 24 h after feeding), and anesthetized using 100 mg/L MS-222. Immediately, blood samples were drawn from the caudal vein using disposable medical syringes a, and centrifuged at 3500 × g at 4°C for 10 min to allow for the collection of plasma. After this, the spleen, intestine, liver, muscle, gill, kidney, heart, and red blood cell samples were also collected into sterile tubes, and muscle were collected at 1, 6, 12, and 24 h after feeding. The collected samples were stored at −80°C in readiness for analysis.
Cloning of GLUT4 cDNA
According to the previous description (Liang et al., 2018), RNAiso Plus reagent (TaKaRa, China) was used to extract the total RNA of mixed tissues. A reverse transcriptase M-MLV kit (TaKaRa, China) was used to synthesize the first-strand cDNA. Besides, 3′-full RACE Core Set Ver.2.0 kit and 5′-full RACE kit (TaKaRa, China) were used to perform 3′-rapid amplification of cDNA ends (RACE) and 5′-RACE. A gel extraction kit (Sangon, China) was used to purify the PCR products and sequenced on an ABI3730 DNA analyzer (ABI, United States) after insertion into a PMD-18T vector (TaKaRa, China). Table 2 showed all the primers used for cloning.
Plasma Parameters
Plasma total glucose (GLU) was measured using an automatic chemical analyzer, Mindray BS-400 (Shenzhen Mindray Biomedical Electronics Co., Ltd., China) following the description by Ren et al. (2017). In addition, an automatic chemiluminescence analyzer, Maglumi 1000, was used to determine plasma insulin levels (Snibe Diagnostic Ltd., China) according to the description of Liang et al. (2019).
Immunohistochemical Analysis
In brief, muscle tissue was encased in resin through several processing steps, including paraformaldehyde fixation, alcohol dehydration, and paraffin embedding. Then, the normal paraffin sections were obtained using a LEICA slicer. Finally, immunohistochemical staining, including dewaxing and rehydration; antigen repair; primary antibody incubation; secondary antibody detection; alkaline phosphatase catalyzed color development; and other processes were performed.
Quantitative Real-Time PCR Analysis of GLUT4 Expression
RNA was first extracted from samples using the RNAiso Plus kit (TaKaRa, China). The quality and quantity of RNA were assessed. After this, GLUT4 mRNA levels were determined using a 7500 real-time PCR machine (Applied Biosystems, United States). Initially, cDNA (2.0 μL) was reacted with 10.0 μL SYBR® Premix Ex Taq II (2×), 0.8 μL forward and reverse primers (10 μM each), 0.4 μL ROX reference dye II (50×), and 6.0 μL RNase-free distilled water in a 20 μl final reaction volume. The specific primers of the target genes used were shown in Table 2. β-actin was used as a reference, non-regulated gene, and no obvious change was observed on its gene expression (Habte-Tsion et al., 2015). Relative genes expression was performed using Pfaffl’s mathematical model for CT calculation (Pfaffl, 2001).
Western Blot Analysis
The method of western blot analysis was performed following the description by Liang et al. (2019). In brief, first, the lysate from the sample was prepared, and then western blot analysis was performed through the following main steps: (1) equal amounts of protein were loaded into the wells of the SDS-PAGE gel, (2) the proteins from the gel were transferred to a PVDF membrane, (3) the membrane was blocked using a blocking buffer, (4) the membrane was incubated with monoclonal antibody to GFP or GADPH diluted 1000× (Bio-Transduction Lab, China), (5) the membrane was washed, (6) the membrane was incubated with the of goat anti-mouse IgG-HRP diluted 1000× (Bio-Transduction Lab, China), (7) the secondary antibody was stained by a BeyoECL Star kit (Beyotime Biotechnology, China) based on the manufacturer’s instructions, and (8) the images were acquired using chemiluminescence. The polyclonal antibody against GLUT4 was synthesized based on the cloned GLUT4 sequence.
Nucleotide Sequence and Bioinformatics and Statistical Analyses
The corresponding amino acid sequence was derived from the full-length cDNA sequence by NAman. The ProtParam tool was used to analyze the basic properties of the proteins1. PSORT Prediction software was used for the subcellular localization analysis2. For the domain analysis, NCBI conserved domains was used to identify the domain and functionality of the sequence (Marchler-Bauer et al., 2017)3. The SUMO and phosphorylation analyses were performed online using http://www.abcepta.com/sumoplot and http://www.cbs.dtu.dk/services/NetPhos/, respectively. The glycosylation analysis was performed via http://www.cbs.dtu.dk/services/NetNGlyc. Homologous sequence alignment was completed using ClustalX software, while the tertiary structure was predicted using the SWISS-MODEL (Biasini et al., 2014)4. A search for sequences similar to the GLUT4 amino acid sequence was carried out with the BLASTP program (Altschul et al., 1997) based on the NCBI website database, and the search results were verified with cluster analysis. MEGA7 software (Kumar et al., 2016) was used to perform multiple sequence alignment and build a phylogenetic tree (Saitou and Nei, 1987) with a bootstrap coefficient of 1000 based on the neighbor-joining (NJ) method. The evolutionary distance was calculated using the Poisson correction method (Zuckerkandl and Pauling, 1965).
The data collected from various parameters were subjected to a homogeneity test where necessary. Statistical analysis was performed using SPSS 16.0. Turkey’s test was used to show where the significant differences existed between the means. Data were expressed as means with SEM (M ± SEM).
Results
Cloning and Characterization of Blunt Snout Bream GLUT4 cDNA
(1) The results showed that the GLUT4 cDNA sequence of blunt snout bream was 2868 bp long, and the mRNA contains a 5′-UTR region of 513 bp and a 3′-UTR region of 837 bp (GenBank accession no: MT447093). Through sequence alignment and bioinformatics analysis, it was inferred that the sequence was a full-length cDNA sequence (Figure 1). The GLUT4 cDNA sequence of the blunt snout bream has an open reading frame of 1518 bp and encodes 505 amino acids (Figure 1). GLUT4 had a theoretical isoelectric point and molecular weight were 6.41 and 55.47 kDa, respectively.
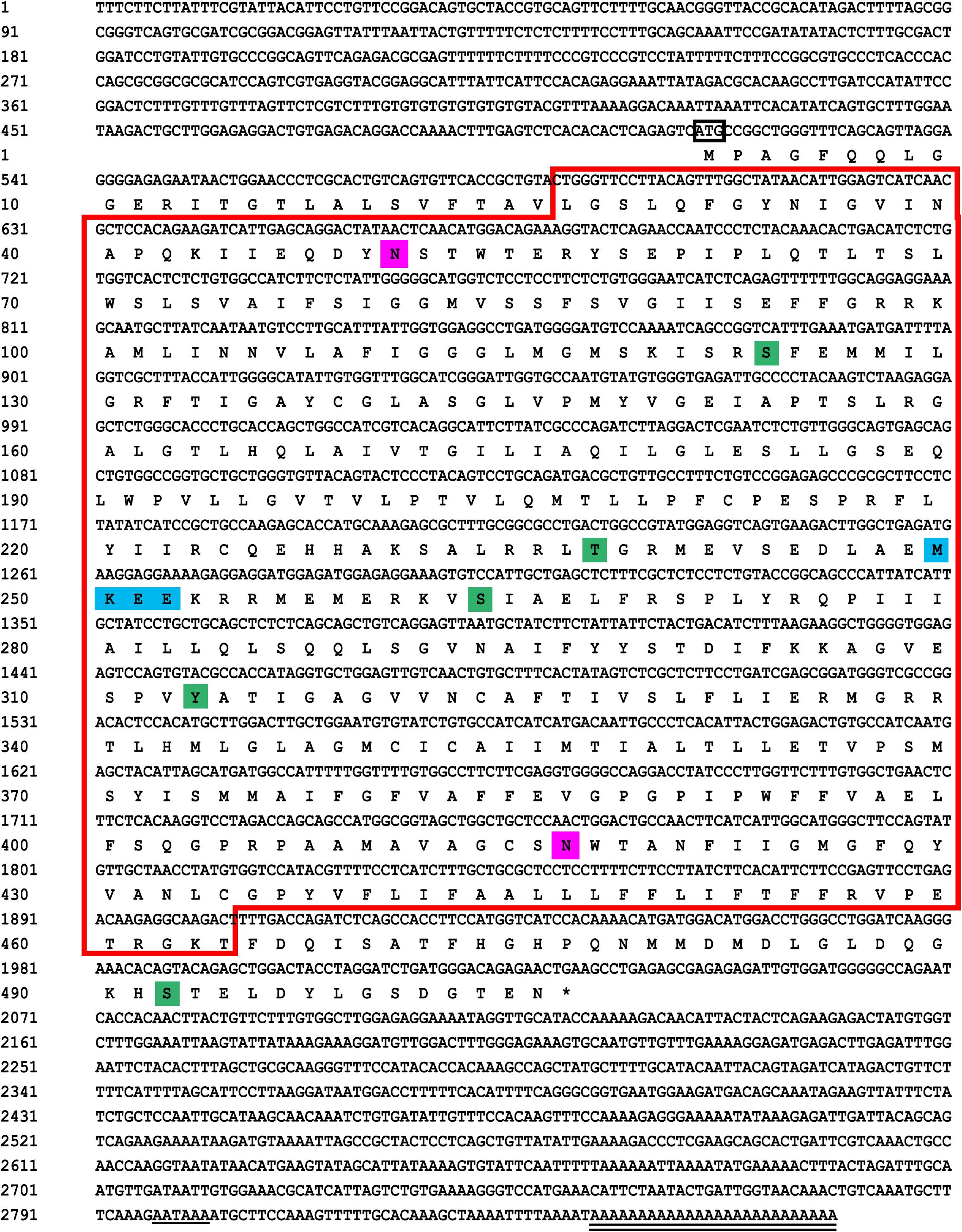
Figure 1. Sequencing results and amino acid sequence analysis of GLUT4 in Megalobrama amblycephala. The black box represents the ATG start codon; * represents the stop codon; underline represents the Poly (A) tail signal; double underline represents the poly (A) sequence; red box represents the possible Class 1 glucose transporters (GLUTs) of the Major Facilitator Superfamily structural domain (cd17431); purple shadow represents the possible N glycosylation sites; green shadow represents the possible phosphorylation site; blue shadow represents the possible SUMO site.
(2) Compared with the results of BLASTP analysis of the NCBI database, the Glut4 of blunt snout bream protein had the highest homology with Cypriniformes fish. The sequence similarity of GLUT4 in Anabarilius grahami (ROL45632.1), Sinocyclocheilus anshuiensis (XP_016324872.1), and Triplophysa tibetana (KAA0723724.1) was 99, 95, and 92%, respectively. Based on a phylogenetic tree generated from the GLUT1–4 amino acid sequences, glucose transporter cDNA from M. amblycephala was clustered with GLUT4 of Cypriniformes fish, and GLUT1, 2, 3, and 4 were clustered together (Figure 2).
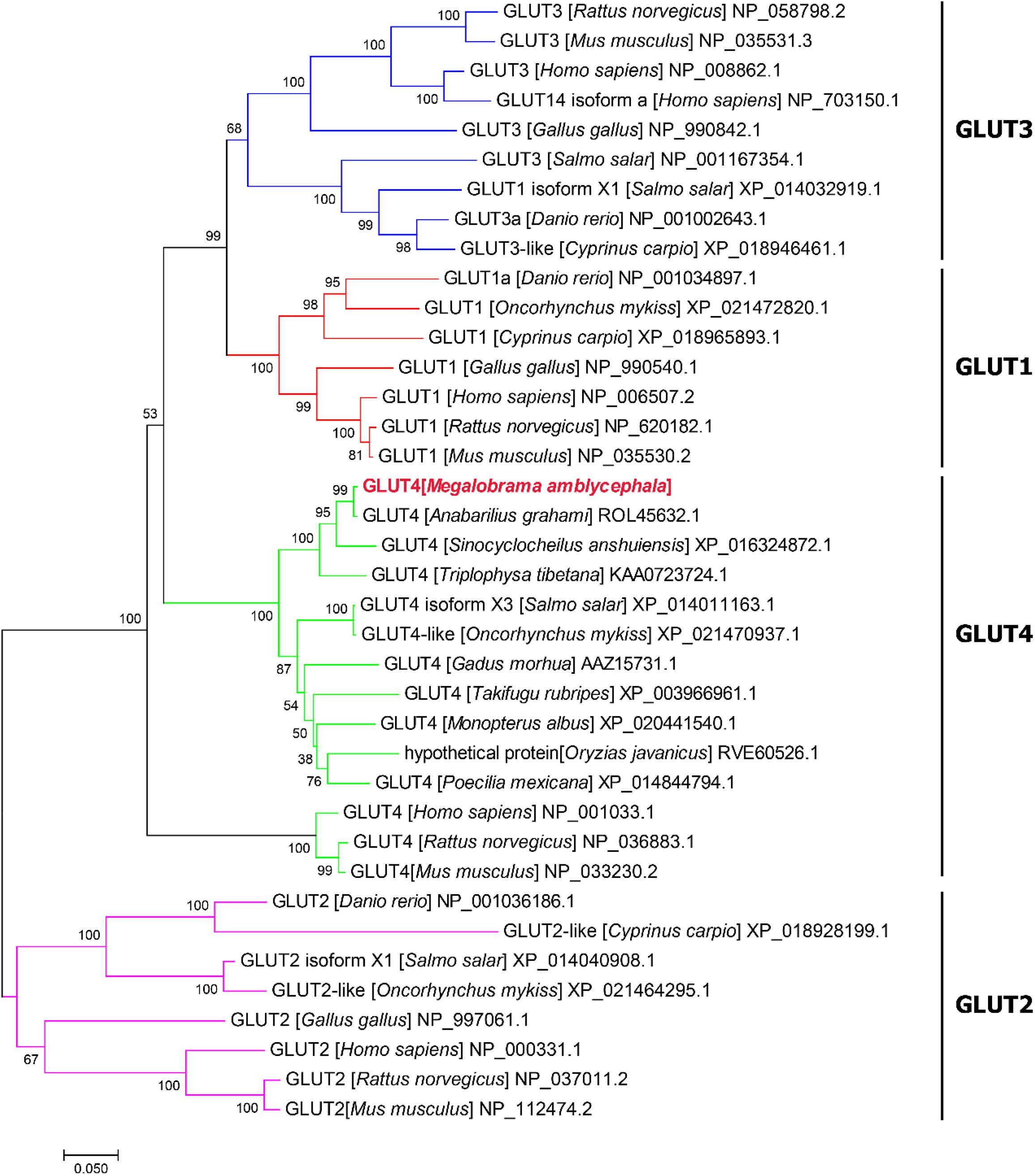
Figure 2. Phylogenetic tree of known vertebrate GLUT protein sequences. A phylogenetic tree was constructed with the complete protein sequence of blunt snout bream (Megalobrama amblycephala) GLUT4 (GenBank accession no: QLE11221), and a number of protein sequences corresponding to various vertebrate GLUTs.
(3) Based on the analysis of subcellular localization, GLUT4 was mainly found in the plasma membrane, accounting for 60.9% of the total expressed. Based on the results of GLUT4 similarity analysis, GLUT4 in aquatic animals contained multiple functional sites that regulated its intracellular transport, but it was also mutated. Compared with the data on homo_sapiens and mus_musculus, some of the mutations observed in the GLUT4 amino acid sequence of aquatic animals were FQQI mutated to FQQL, LL mutated to MM, and TELEY mutated to TELDY (Figure 3).
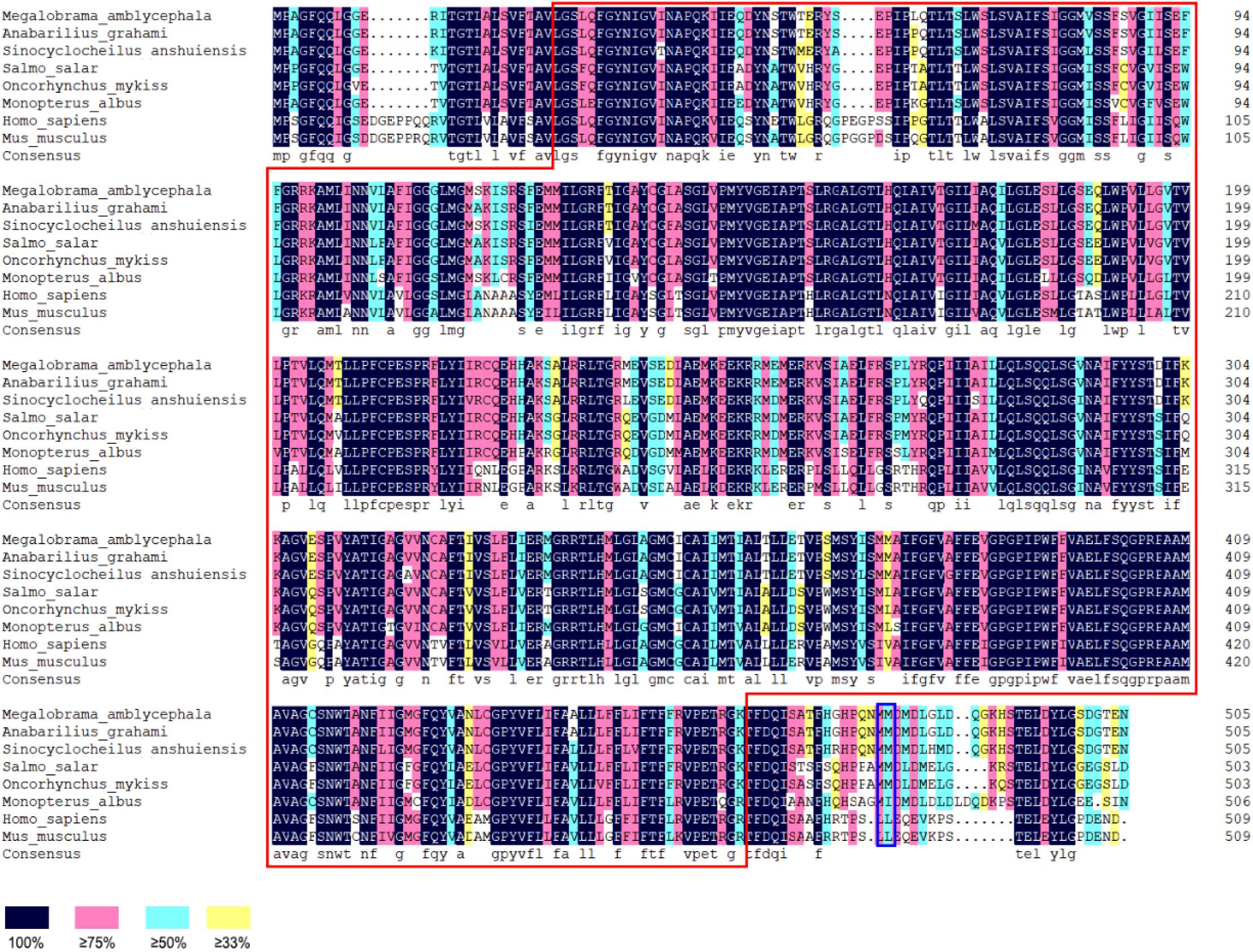
Figure 3. Homologous alignment of GLUT4 amino acid sequences in different species. Red box represents the Class 1 glucose transporters (GLUTs) of the Major Facilitator Superfamily structural domain (cd17431); blue box represents the dileucine internalization motif.
(4) Based on the results of the predicted tertiary structure, the possible transmembrane tertiary structure of GLUT4 was an outwardly closed conformation as shown in Figure 4A with blue for the N terminus and red for the C terminus and a transmembrane protein composed of a 12th α-helix with no β-pleated sheet, which formed a hollow channel and was specific for monosaccharides transported across the membrane (Figure 4B).
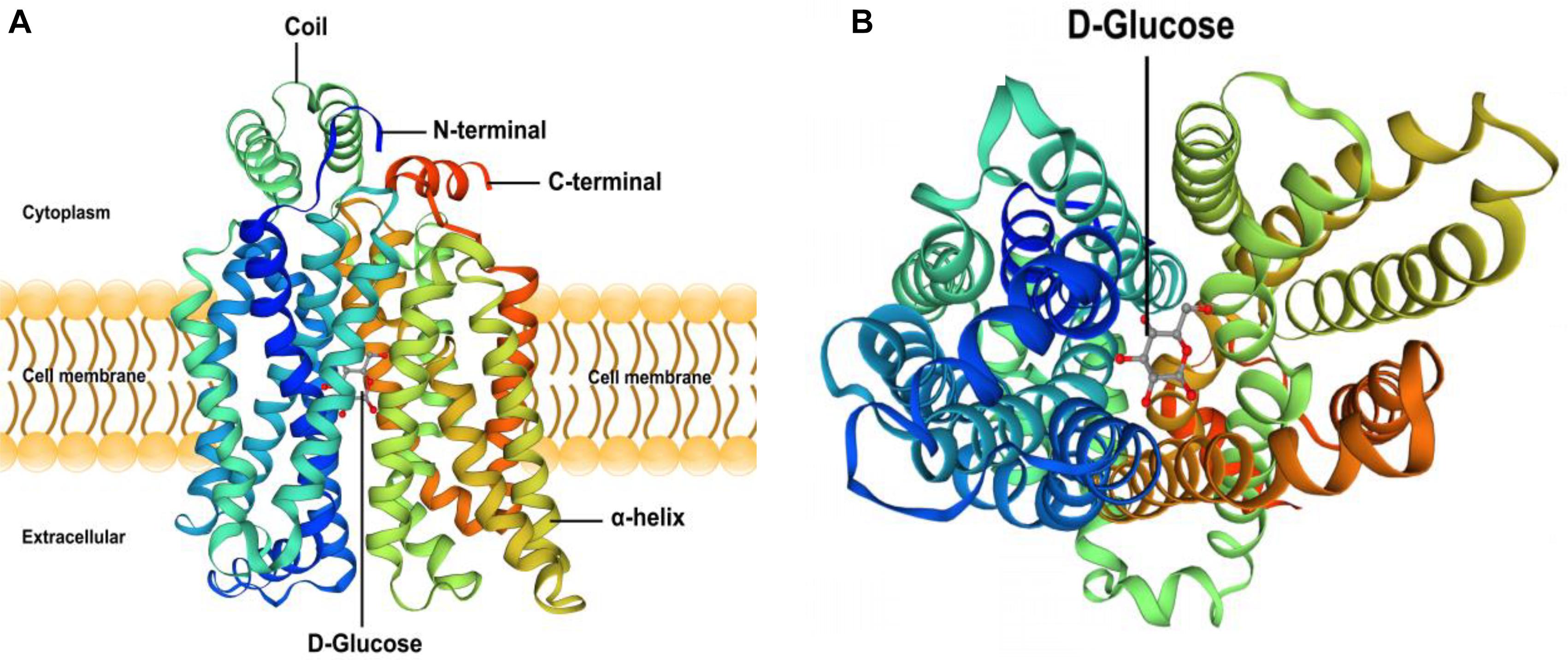
Figure 4. Protein 3D structure prediction of GLUT4 in Megalobrama amblycephala. Panel (A) shows that blue is the N-terminal, red is the C-terminal in (A), GLUT4 was a transmembrane protein composed of a 12th-α-helix, with no β-pleated sheet, which formed a hollow channel and specifically identified monosaccharides transported across the membrane. Panel (B) shows that GLUT4 perspective to the bottom of the 3D conformation.
Tissue Distribution of GLUT4
Glucose transporter 4 mRNA was constitutively expressed in the muscle, intestine and liver. The highest mRNA level was observed in the muscle, and the mRNA levels of GLUT4 were not detected in the spleen, gill, kidney, heart, or red blood cells (Figure 5). Furthermore, immunohistochemical staining showed GLUT4 to be a kind of carrier protein embedded in the cell membrane to transport glucose and existed in the cell membrane (Figure 6).
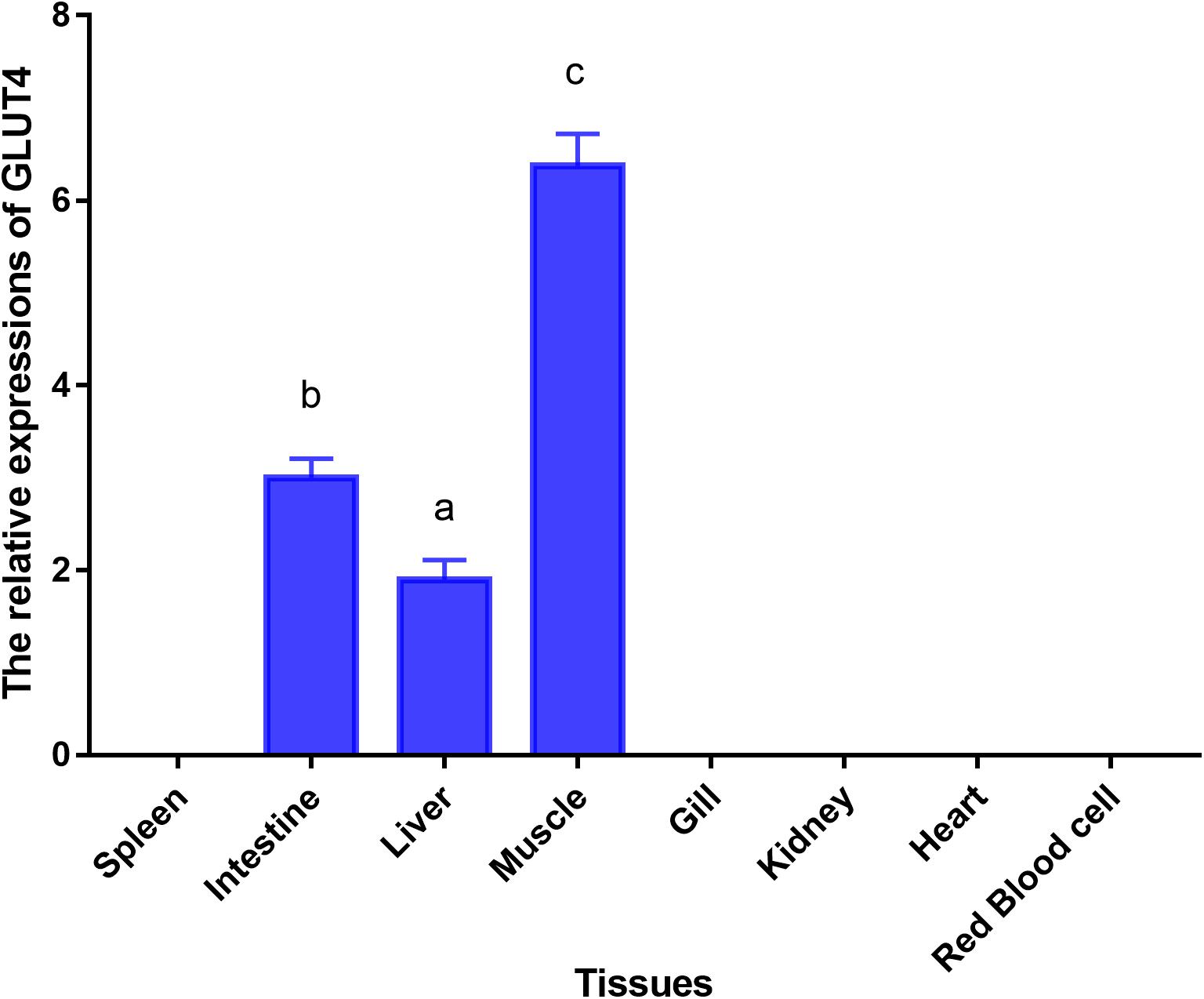
Figure 5. Tissue-specific mRNA expressions of GLUT4 in Megalobrama amblycephala. Vertical bars represent mean ± SE values for triplicate samples. Mean values with unlike small letters above bars are significantly different (P < 0.05).
Continual Glucose Feeding Trial
Blood glucose level peaked within 1 h, however, the insulin levels peaked at 6 h. Furthermore, GLUT4 mRNA and protein levels of muscle showed an upward trend with an increase in feeding time, although, after 12 h, the expression and protein levels dropped sharply (Figures 7, 8).
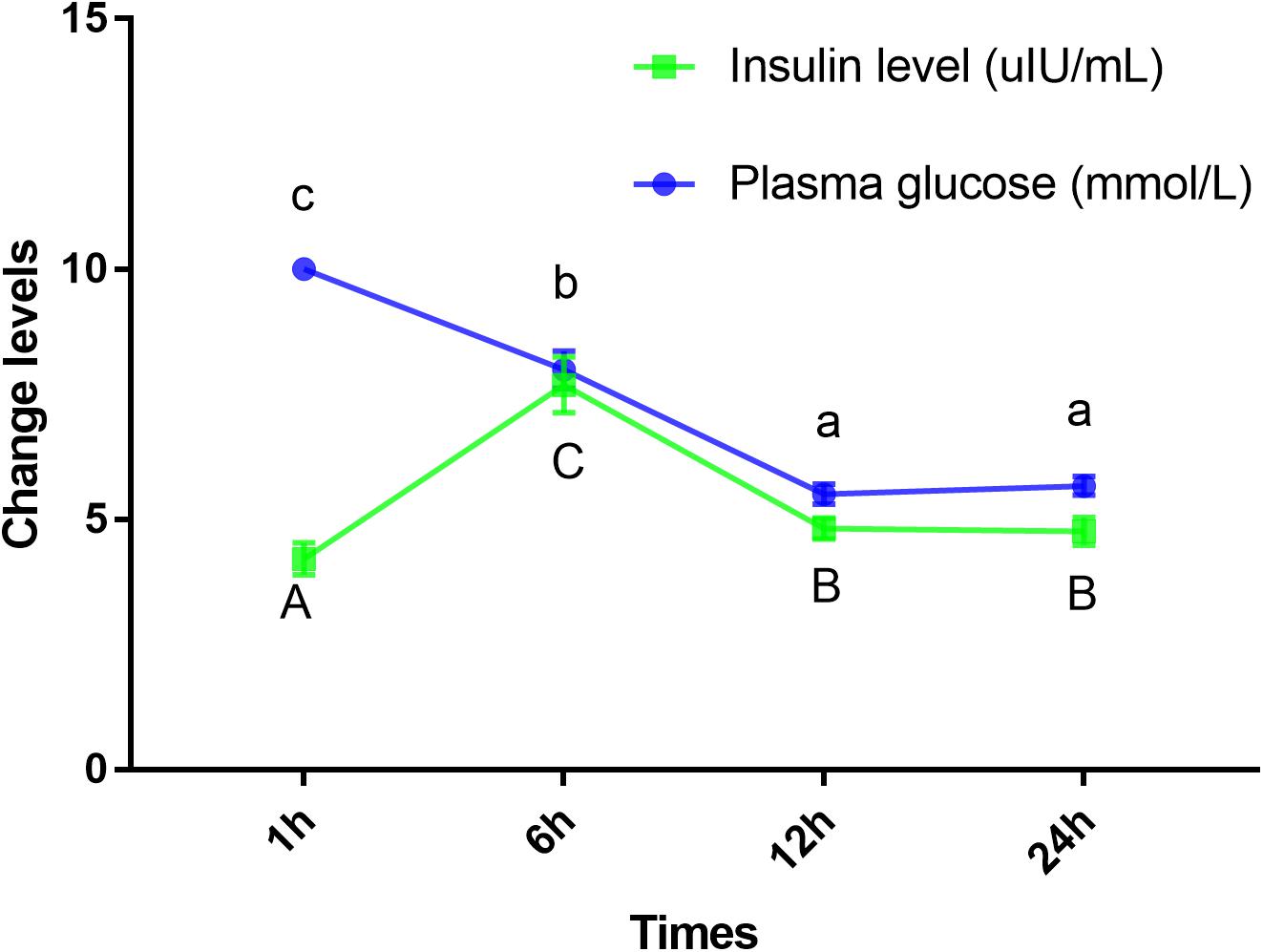
Figure 7. Insulin and glucose levels of plasma in Megalobrama amblycephala. Points represent mean ± SE values for triplicate samples. Mean values with unlike small letters or big letters in points are significantly different (P < 0.05).
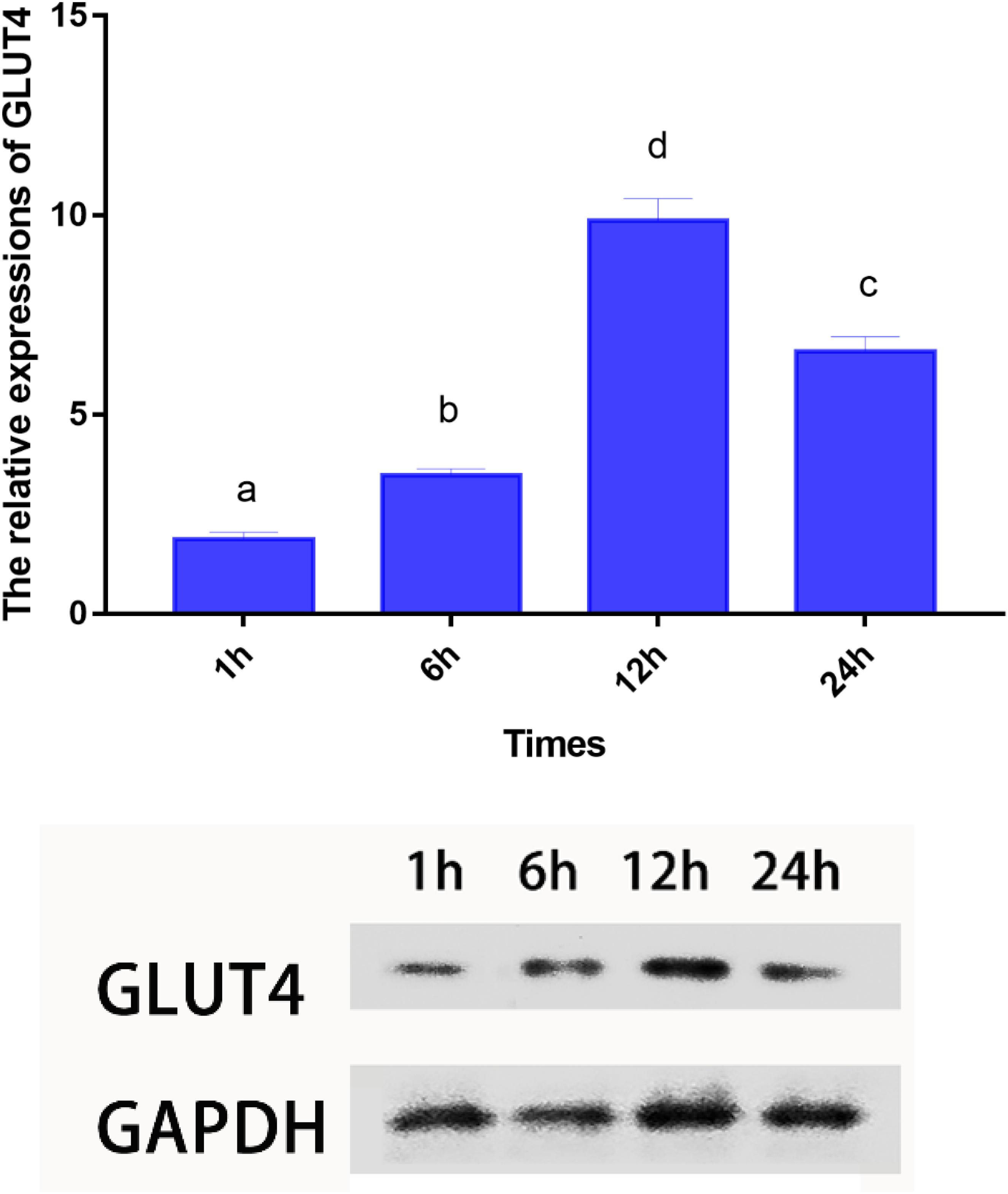
Figure 8. mRNA and protein expressions of GLUT4 in muscle of Megalobrama amblycephala after glucose intake. Vertical bars represent mean ± SE values for triplicate samples. Mean values with unlike small letters above bars are significantly different (P < 0.05).
Discussion
Glucose transporter 4 has been identified in mammals (James et al., 1988) and some fish species (Capilla et al., 2004a; Li et al., 2018). In this study, the full-length cDNA of one glucose transporter from M. amblycephala (GenBank accession no: MT447093) was identified. Compared with the results of the BLASTP analysis using the NCBI database, the amino acid sequence of this gene showed the highest homology with Cypriniformes fish and glucose transporter cDNA from M. amblycephala clustered with GLUT4 of Cypriniformes fish based on a phylogenetic tree generated using GLUT1–4 amino acid sequences. Based on these results, the cDNA was categorized as GLUT4 of blunt snout bream (MaGLUT4). The results of the similarity analysis of GLUT4 among species showed that GLUT4 in aquatic animals contained multiple functional sites that regulated its intracellular transport, but it also had mutations. Compared with the homo_sapiens and mus_musculus sequence data, some mutations were observed in the GLUT4 amino acid sequence of aquatic animals including FQQI mutated to FQQL, LL mutated to MM and TELEY mutated to TELDY, indicating that the function and molecular characterization of GLUT4 probably further evolved depending on species differences. In humans, studies have revealed that the FQQI motif in the N terminus of GLUT4 plays an important regulatory role in the translocation of GLUT4 from the cell surface to the cell (Garippa et al., 1994), and TELEY, an acid cluster at one end, plays an important role in sorting and transporting GLUT4 within the cell under basic conditions (Blot and Mcgraw, 2008). Any mutation in either motif of GLUT4 alters the way GLUT4 is transported within the cell (Blot and Mcgraw, 2008). Additionally, it was noted that the LL functional sites, considered important regulators of GLUT4 translocation from intracellular vesicles to cell membranes and for endocytosis in mammals (Pessin et al., 1999; Al-Hasani et al., 2002; Capilla et al., 2007), were absent from the GLUT4 amino acid sequences in aquatic animals, which may be one of the reasons why fish are less tolerant to glucose than higher vertebrates.
In mammals, the skeletal, adipose tissue, and cardiac muscle are the main expression tissues of GLUT4, and are referred to insulin-sensitive tissues (Marín-Juez et al., 2014). In skeletal muscle, GLUT4, accounting for approximately 70% of the basal glucose transport of this tissue, is the major transporter expressed (Zorzano et al., 2005). In the present study, GLUT4 mRNA was constitutively expressed in the muscle, intestine, and liver, with the highest GLUT4 expression level observed in the muscle. Similar to the findings of the present study, the GLUT4 tissue expression pattern was also reported to be mainly distributed in the muscle tissue in mammals (Duehlmeier et al., 2007; Marín-Juez et al., 2014). In aquatic animals, the GLUT4 tissue expression pattern at the mRNA level has been examined in some species. The highest GLUT4 expression level was observed in the muscle in brown trout (Planas et al., 2000), tilapia (Oreochromis niloticus × Oreochromis aureus) (Chen, 2016), cobia (Rachycentron canadum) (Chen, 2016), pompano (Trachinotus ovatus) (Chen, 2016) and pearl gentian grouper (Epinephelus fuscoguttatus ♀ × Epinephelus lanceolatus ♂) (Li et al., 2018). However, in Atlantic cod (Acipenser oxyrinchus), GLUT4 was mainly distributed in the heart (Hall et al., 2006). The reason for the difference in the distribution of GLUT4 in different fish species remains unknown.
In this study, based on the analysis of sub-cellular localization, MaGLUT4 was mainly found in the plasma membrane, accounting for 60.9% of the total. Hence, MaGLUT4 might also be a membrane protein that acts as a transporter. Based on the immunohistochemical analysis, MaGLUT4 mainly existed in the muscle cell membrane. According to these results, MaGLUT4 was a kind of carrier protein embedded in the cell membrane to transport glucose, residing mainly in the cell membrane and playing a transmembrane transport role in blunt snout bream. The possible transmembrane tertiary structure of MaGLUT4 had an outwardly closed conformation, shown in Figure 4A as blue for the N-terminus and red for the C-terminus, with a transmembrane protein composed of a 12th α-helix with no β-pleated sheet, indicating that these structures formed a hollow channel that was specific for the monosaccharides transported across the membrane. The transition from an outwardly open to an outwardly closed conformation required a significant local rearrangement of the extracellular portion of the transmembrane segment, for which the C-terminal domain could provide the primary substrate-binding site. However, the possible tertiary transmembrane structure of GLUT4 in pompano was a β-pleated sheet (Chen, 2016). In the present study, the newly discovered MaGLUT4 possessed 12 transmembrane domains, one of the characteristics of GLUT4 in mammals (Joost and Thorens, 2001) and fish (Capilla et al., 2004a; Hall et al., 2006). Furthermore, this structure was in agreement with GLUTs reported in other fish, such as rainbow trout (Teerijoki et al., 2000), tilapia (O. niloticus) (Hrytsenko et al., 2010), gilthead seabream (Sparus aurata) (Balmaceda-Aguilera et al., 2012) and grouper (Epinephelus coioides) (Liu et al., 2017). These results indicated that GLUT4 amino acid sequences in fish were highly conserved compared to those in humans and mammals (Planas et al., 2000; Capilla et al., 2004a), a situation similar among other transporters. Overall, these results confirmed that MaGLUT4 was a structural and functional homolog of GLUT4 in mammals and other fish. However, the important difference was that GLUT4 in fish had a lower affinity for glucose and wider substrate specificity than mammalian GLUT4 (Díaz et al., 2007).
Mammals have a high capacity for glucose metabolism, and after consuming carbohydrates, blood glucose levels can return to normal within 1–2 h; however, the carbohydrate tolerance of fish is low and often accompanied by persistent hyperglycemia after carbohydrates are ingested (Moon, 2001). In this study, the plasma glucose levels peaked within approximately 1 h; furthermore, blood glucose levels returned to normal within 12 h, which indicated that blunt snout bream could digest glucose. These results were similar to those reported on other species, such as juvenile tilapia (Shiau and Chuang, 1995) and carp (Cyprinus carpio) (Stone, 2003). However, these results differed from those reported in carnivorous fish, such as white sturgeon (Acipenser transmontanus) (Hung, 1991), chinook salmon (Oncorhynchus tshawytscha) (Mazur et al., 1992), and rainbow trout (Polakof et al., 2012) where blood glucose peaked at least 6 h after carbohydrate intake with the recovery of basal blood glucose levels at least 18 h later. It was regrettable that the specific metabolic regulation mechanism was still unclear. Studies have shown that insulin is the most important regulator of blood glucose balance in mammals and fish (Viegas et al., 2013), and exogenous insulin could effectively reduce the blood glucose level of fish (Deroos et al., 1985). In the present study, the highest plasma insulin levels were observed within 6 h, which was consistent with previous results showing that ingesting digestible carbohydrates caused hyperglycemia and increased insulin levels (Capilla et al., 2004a, b). Interestingly, in this study, the blood glucose peaked within 1 h; while the highest level of insulin was observed after 6 h. These results indicated that fish might not respond to changes in plasma glucose in a timely manner. Therefore, the results of this study could be used to infer that this observation may be related to the delayed response mechanism of blood glucose changes, such as the delayed response of insulin to blood glucose changes, which might be an important cause of digestible carbohydrate intolerance in fish. This delay may be caused by the fish being stimulated to secrete glucagon and somatostatin hormones under the stress of hyperglycemia, thereby inhibiting the secretion of insulin and delaying the change in insulin in response to plasma glucose (Ronner and Scarpa, 1984; Harmon et al., 1991). Andoh (2015) also reported that the regulation of insulin levels in the plasma of barfin flounder (Verasper moseri), was mainly the result of release from Brockman bodies, not through transcription and translation, indicating that the mechanism of insulin release in teleosts might be different from that of glucose-dependent release in mammals (Hemre et al., 2002). As has been widely reported, insulin could activate GLUT4 (Lizunov et al., 2013), and the GLUT4 protein could be translocated from intracellular compartments to the plasma membrane to increase the uptake of glucose both in mammals (Bryant et al., 2002) and in fish (Lu et al., 2018). In this study, the mRNA and protein levels of GLUT4 showed an upward trend with an increase in feeding time; however, after 12 h, the expression level decreased sharply, indicating that GLUT4 was activated to maintain glucose homeostasis, thereby regulating and enhancing glucose metabolism in this fish. Similarly, dietary carbohydrate levels upregulated the expression of GLUT4 in giant grouper larvae (E. lanceolatus) (Lu et al., 2018), pearl gentian grouper (Li et al., 2018), and North Pacific spiny dogfish (Squalus suckleyi) (Deck et al., 2017) which contributes to the regulation of glucose metabolism. In fish, it has been proven that glucose can stimulate the secretion of insulin in a mammalian-like manner (Moon, 2001; Hrytsenko et al., 2008) which might account for the elevated mRNA and protein levels of GLUT4 in this study. Notably, some delays were observed in the changes of insulin and the expression of GLUT4 compared with the changes in plasma glucose. Similar to the results showing genetic enhancement in farmed tilapia, changes in insulin level and GLUT4 mRNA levels were delayed compared with the absorption rate of carbohydrates (Liu et al., 2018). Additionally, there was also a delayed response between changes in blood glucose and changes in insulin and mRNA levels of the genes in grouper (Mao, 2014). Overall, the results of this study indicated that plasma glucose adjustments caused by carbohydrate intake could be affected by the delayed action of insulin. This finding might explain why changes in the mRNA and protein levels of GLUT4 lagged notably behind the changes in blood glucose. Therefore, this delay in insulin level changes and GLUT4 activation might be an important reason for glucose intolerance in this fish.
Conclusion
In general, we cloned the full length of MaGLUT4 and identified its functions and localization. MaGLUT4 is mainly distributed in the muscle and located in the cell membrane, where it played a role in transporting glucose into cells and regulating blood glucose. Furthermore, some delays were observed in the changes in insulin and the expression of GLUT4 compared with the changes in plasma glucose.
Data Availability Statement
The raw data supporting the conclusions of this article will be made available by the authors, without undue reservation. Requests to access these datasets should be directed to MR.
Ethics Statement
The handling of the juveniles was in accordance with the Ministry of Agriculture, China and international animal welfare laws, guidelines and policies (Food and Agriculture Organization [FAO], 2004) and was approved by the Institutional Animal Care and Ethics Committee of Nanjing Agricultural University, Nanjing, China [Permit number: SYXK (Su) 2011-0036].
Author Contributions
XG and MR designed the study. HL carried out the experiments and wrote the manuscript. SM reviewed the manuscript. KJ provided technical assistance. HM provided technical guidance. All authors contributed to the article and approved the submitted version.
Funding
This work was supported by the National Key R&D Program of China (2018YFD0900400), Central Public-interest Scientific Institution Basal Research Fund, Freshwater Fisheries Research Center, CAFS (No. 2020JBFR01), and the National Natural Science Foundation of China, NSFC (31772820).
Conflict of Interest
HM was employed by company Tongwei Co., Ltd.
The remaining authors declare that the research was conducted in the absence of any commercial or financial relationships that could be construed as a potential conflict of interest.
Acknowledgments
The participants are appreciated for their contribution to this study.
Footnotes
- ^ http://web.expasy.org/protparam
- ^ http://psort1.hgc.jp/form.html
- ^ https://www.ncbi.nlm.nih.gov/Structure/cdd/docs/cdd_search.html
- ^ https://swissmodel.expasy.org
References
Al-Hasani, H., Kunamneni, R. K., Dawson, K., Hinck, C. S., Müller-Wieland, D., and Cushman, S. W. (2002). Roles of the N-and C-termini of GLUT4 in endocytosis. J. Cell Sci. 115, 131–140. doi: 10.1080/15216540210809
Altschul, S. F., Madden, T. L., Schäffer, A. A., Zhang, J., Zhang, Z., Miller, W., et al. (1997). Gapped BLAST and PSI-BLAST: a new generation of protein database search programs. Nucleic Acids Res. 25, 3389–3402. doi: 10.1093/nar/25.17.3389
Andoh, T. (2015). Plasma insulin levels are regulated by release, rather than transcription or translation, in barfin flounder, Verasper moseri. Comp. Biochem. Physiol. A Mol. Integr. Physiol. 184, 27–33. doi: 10.1016/j.cbpa.2015.01.020
Arnesen, P., and Krogdahl, A. (1993). Crude and pre-extruded products of wheat as nutrient sources in extruded diets for Atlantic salmon (Salmo salar, L) grown in seawater. Aquaculture 118, 105–117. doi: 10.1016/0044-8486(93)90284-6
Balmaceda-Aguilera, C., Martos-Sitcha, J. A., Mancera, J. M., and Mart_ınez-Rodr_ıguez, G. (2012). Cloning and expression pattern of facilitative glucose transporter 1 (GLUT1) in gilthead sea bream Sparus aurata in response to salinity acclimation. Comp. Biochem. Physiol. A. Mol. Integr. Physiol. 163, 38–46. doi: 10.1016/j.cbpa.2012.04.026
Barron, C. C., Bilan, P. J., Tsakiridis, T., and Tsiani, E. (2016). Facilitative glucose transporters: implications for cancer detection, prognosis, and treatment. Metabolism 65, 124–139. doi: 10.1016/j.metabol.2015.10.007
Becker, C., Sevilla, L., Tomàs, E., Palacin, M., Zorzano, A., and Fischer, Y. (2001). The endosomal compartment is an insulin-sensitive recruitment site for GLUT4 and GLUT1 glucose transporters in cardiac myocytes. Endocrinology 142, 5267–5276. doi: 10.1210/endo.142.12.8555
Biasini, M., Bienert, S., Waterhouse, A., Arnold, K., Studer, G., Schmidt, T., et al. (2014). SWISS-MODEL: modeling protein tertiary and quaternary structure using evolutionary information. Nucleic Acids Res. 42, W252–W258. doi: 10.1093/nar/gku340
Blot, V., and Mcgraw, T. (2008). Molecular mechanisms controlling GLUT4 intracellular retention. Mol. Biol. Cell 19, 3477–3487. doi: 10.1091/mbc.e08-03-0236
Brant, A. M., Jess, T. J., Milligan, G., Brown, C. M., and Gould, G. W. (1993). Immunological analysis of glucose transporters expressed in different regions of the rat brain and central nervous system. Biochem. Biophys. Res. Commun. 192, 1297–1302. doi: 10.1006/bbrc.1993.1557
Bryant, N. J., Govers, R., and James, D. E. (2002). Regulated transport of the glucose transporter GLUT4. Nat. Rev. Mol. Cell. Biol. 3, 267–277. doi: 10.1038/nrm782
Capilla, E., Díaz, M., Albalat, A., Navarro, I., Pessin, J. E., Keller, K., et al. (2004a). Functional characterization of an insulin-responsive glucose transporter (GLUT4) from fish adipose tissue. Am. J. Physiol. Endocrinol. Metab. 287, E348–E357. doi: 10.1152/ajpendo.00538.2003
Capilla, E., Médale, F., Panserat, S., Vachot, C., Rema, P., Gomes, E., et al. (2004b). Response of hexokinase enzymes and the insulin system to dietary carbohydrates in the common carp. Cyprinus Carpio. Reprod. Nutr. Dev. 44, 1–10. doi: 10.1051/rnd:2004027
Capilla, E., Suzuki, N., Pessin, J. E., and Hou, J. C. Q. (2007). The glucose transporter 4 FQQI motif is necessary for akt substrate of 160-kilodalton-dependent plasma membrane translocation but not golgi-localized (Gamma)-Ear-containing arf-binding protein-dependent entry into the insulin-responsive storage compartment. Mol. Endocrinol. 21, 3087–3099. doi: 10.1210/me.2006-0476
Charon, M. J., Brosius, F. C., Alper, S. L., and Lodish, H. F. (1989). A glucose transport protein expressed predominately in insulin-responsive tissues. Proc. Natl. Acad. Sci. U.S.A. 86, 2535–2539. doi: 10.1073/pnas.86.8.2535
Chen, Q. (2016). Comparison Study on Clone and Expression of Glucose Transporter 4(GLUT4) in Different Fish Species. Zhanjiang: Guangdong Ocean University, doi: 10.7666/d.Y3102778
Dalziel, A. C., Laporte, M., Guderley, H., and Bernatchez, L. (2018). Do differences in the activities of carbohydrate metabolism enzymes between Lake Whitefish ecotypes match predictions from transcriptomic studies? Comp. Biochem. Physiol. B Biochem. Mol. Biol. 224, 138–149. doi: 10.1016/j.cbpb.2017.08.001
Deck, C. A., Anderson, W. G., and Walsh, P. J. (2017). Effects of glucose and insulin administration on glucose transporter expression in the North Pacific spiny dogfish (Squalus suckleyi). Gen. Comp. Endocrinol. 247, 46–52. doi: 10.1016/j.ygcen.2017.01.016
Deroos, R., Deroos, C. C., Werner, C. S., and Werner, H. (1985). Plasma levels of glucose, alanine, lactate, and β-hydroxybutyrate in the unfed spiny dogfish shark (Squalus acanthias) after surgery and following mammalian insulin infusion. Gen. Comp. Endocrinol. 58, 28–43. doi: 10.1016/0016-6480(85)90133-9
Díaz, M., Antonescu, C. N., Capilla, E., Klip, A., and Planas, J. V. (2007). Fish glucose transporter (glut)-4 differs from rat glut4 in its traffic characteristics but can translocate to the cell surface in response to insulin in skeletal muscle cells. Endocrinology 148, 5248–5257. doi: 10.1210/en.2007-0265
Duehlmeier, R., Sammet, K., Widdel, A., von Engelhardt, W., Wernery, U., Kinne, J., et al. (2007). Distribution patterns of the glucose transporters GLUT4 and GLUT1 in skeletal muscles of rats (Rattus norvegicus), pigs (Sus scrofa), cows (Bos taurus), adult goats, goat kids (Capra hircus), and camels (Camelus dromedarius). Comp. Biochem. Physiol. A Mol. Integr. Physiol. 146, 274–282. doi: 10.1016/j.cbpa.2006.10.029
Food and Agriculture Organization [FAO] (2004). in National Aquaculture Legislation Overview-China. National Aquaculture Legislation Overview (NALO) Fact Sheets, ed. M. Spreij (Rome: FAO Fisheries and Aquaculture Department).
Fukatmoto, H., Kayano, T., Buse, J., Edwards, Y., Pilch, P. F., Bell, G. I., et al. (1989). Cloning and characterization of the major insulin-responsive glucose transporter expressed in human skeletal muscle and other insulin-responsive tissues. J. Biol. Chem. 264, 7776–7779. doi: 10.1111/j.1432-1033.1989.tb14792.x
Gao, W., Liu, Y. J., Tian, L. X., Mai, K. S., Liang, G. Y., Yang, H. J., et al. (2010). Effect of dietary carbohydrate-to-lipid ratios on growth performance, body composition, nutrient utilization and hepatic enzymes activities of herbivorous grass carp (Ctenopharyngodon idella). Aquac. Nutr. 16, 327–333. doi: 10.1111/j.1365-2095.2009.00668.x
Garippa, R. J., Judge, T. W., James, D. E., and McGraw, T. E. (1994). The amino terminus of GLUT4 functions as an internalization motif but not all intracellular retention signal when substituted for the transfemn receptor cytoplasmic domain. J. Cell Biol. 124, 705–715. doi: 10.1083/jcb.124.5.705
Habte-Tsion, H. M., Liu, B., Ren, M. C., Xie, J., Ge, X. P., Chen, R., et al. (2015). A deficiency or an excess of dietary threonine level affects weight gain, enzyme activity, immune response and immune-related gene expression in juvenile blunt snout bream (Megalobrama amblycephala). Fish Shellfish Immunol. 42, 439–446. doi: 10.1016/j.fsi.2014.11.021
Hall, J. R., Short, C. E., and Driedzic, W. R. (2006). Sequence of Atlantic cod (Gadus morhua) GLUT4, GLUT2 and GPDH: developmental stage expression, tissue expression and relationship to starvation-induced changes in blood glucose. J. Exp. Biol. 209, 4490–4502. doi: 10.1242/jeb.02532
Harmon, J. S., Eilertson, C. D., Sheridan, M. A., and Plisetskaya, E. M. (1991). Insulin suppression is associated with hypersomatostatinemia and hyperglucagonemia in glucose-injected rainbow trout. Am. J. Physiol. 261, R609–R613. doi: 10.1152/ajpregu.1991.261.3.R609
Hemre, G. I., and Hansen, T. (1998). Utilization of different dietary starch sources and tolerance to glucose loading in Atlantic salmon during parr-smolt transformation. Aquaculture 161, 145–157. doi: 10.1016/S0044-8486(97)00266-4
Hemre, G. I., Mommsen, T. P., and Krogdahl, A. (2002). Carbohydrates in fish nutrition: effects on growth, glucose metabolism and hepatic enzymes. Aquac. Nutr. 8, 175–194. doi: 10.1046/j.1365-2095.2002.00200.x
Honorato, C. A., Almeida, L. C., Da Silva Nunes, C., Carneiro, D. J., and Moraes, G. (2010). Effects of processing on physical characteristics of diets with distinct levels of carbohydrates and lipids: the outcomes on the growth of pacu (Piaractus mesopotamicus). Aquac. Nutr. 16, 91–99. doi: 10.1111/j.1365-2095.2008.00644.x
Hrytsenko, O., Pohajdak, B., Xu, B. Y., Morrison, C., Vantol, B., and Wright, J. R. Jr. (2010). Cloning and molecular characterization of the glucose transporter 1 in tilapia (Oreochromis niloticus). Gen. Comp. Endocrinol. 165, 293–303. doi: 10.1016/j.ygcen.2009.07.017
Hrytsenko, O., Wright, J. R. Jr., and Pohajdak, B. (2008). Regulation of insulin gene expression and insulin production in Nile tilapia (Oreochromis niloticus). G Xen. Comp. Endocrinol. 155, 328–340. doi: 10.1016/j.ygcen.2007.05.006
Hung, S. S. (1991). Carbohydrate utilization by white sturgeon as assessed by oral administration tests. J. Nutr. 121, 1600–1605. doi: 10.1093/jn/121.10.1600
James, D. E., Brown, R., Navarro, J., and Pilch, P. F. (1988). Insulin-regulatable tissues express a unique insulin-sensitive glucose transport protein. Nature 333, 183–185. doi: 10.1038/333183a0
Jin, J. Y., Médale, F., Kamalam, B. S., Aguirre, P., Véron, V., and Panserat, S. (2014). Comparison of glucose and lipid metabolic gene expressions between fat and lean Lines of rainbow trout after a glucose load. PLoS One 9:e105548. doi: 10.1371/journal.pone.0105548
Joost, H. G., and Thorens, B. (2001). The extended GLUT-family of sugar/polyol transport facilitators: nomenclature, sequence characteristics, and potential function of its novel members. Mol. Membr. Biol. 18, 247–256. doi: 10.1080/09687680110090456
Kaestner, K. H., Christy, R. J., McLenithan, J. C., Braiterman, L. T., Cornelius, P., Pekala, P. H., et al. (1989). Sequence, tissue distribution, and differential expression of mRNA for a putative insulin-responsive glucose transporter in mouse 3T3-L1 adipocytes. Proc. Natl. Acad. Sci. U.S.A. 86, 3150–3154. doi: 10.1073/pnas.86.9.3150
Kamalam, B. S., Medale, F., and Panserat, S. (2017). Utilisation of dietary carbohydrates in farmed fishes: new insights on influencing factors, biological limitations and future strategies. Aquaculture 467, 3–27. doi: 10.1016/j.aquaculture.2016.02.007
Kumar, S., Stecher, G., and Tamura, K. (2016). MEGA7: molecular evolutionary genetics analysis version 7.0 for bigger datasets. Mol. Biol. Evol. 33, 1870–1874. doi: 10.1093/molbev/msw054
Li, S. L., Li, Z. Q., Sang, C. Y., Zhang, J. C., Chen, N. S., and Huang, X. X. (2018). Glucose transporters in pearl gentian grouper (Epinephelus fuscoguttatus ♀ X E. lanceolatus ♂): molecular cloning, characterization, tissue distribution and their expressions in response to dietary carbohydrate level. Aqua. Res. 49, 253–264. doi: 10.1111/are.13455
Liang, H. L., Mokrani, A., Chisomo-Kasiya, H., Ji, K., Ge, X. P., Ren, M. C., et al. (2019). Dietary leucine affects glucose metabolism and lipogenesis involved in TOR/PI3K/Akt signaling pathway for juvenile blunt snout bream Megalobrama amblycephala. Fish Physiol. Biochem. 45, 719–732. doi: 10.1007/s10695-018-0594-x
Liang, H. L., Mokrani, A., Kasiya, H. C., Ogwok-Manas, W. A., Mi, H. F., Ji, K., et al. (2018). Molecular characterization and identification of facilitative glucose transporter 2 (GLUT2) and its expression and of the related glycometabolism enzymes in response to different starch levels in blunt snout bream (Megalobrama amblycephala). Fish Physiol. Biochem. 44, 869–883. doi: 10.1007/s10695-018-0477-1
Liu, H., Dong, X., Chi, S., Yang, Q., Zhang, S., Chen, L., et al. (2017). Molecular cloning of glucose transporter 1 in grouper Epinephelus coioides and effects of an acute hyperglycemia stress on its expression and glucose tolerance. Fish Physiol. Biochem. 43, 103–114. doi: 10.1007/s10695-016-0271-x
Liu, H. Y., Chen, Q., Tan, B. P., Dong, X. H., Chi, S. Y., Yang, Q. H., et al. (2018). Effects of dietary carbohydrate levels on growth, glucose tolerance, glucose homeostasis and GLUT4 gene expression in Tilapia nilotica. Aqua. Res. 49, 3735–3745. doi: 10.1111/are.13841
Lizunov, V. A., Stenkula, K., Troy, A., Cushman, S. W., and Zimmerberg, J. (2013). Insulin regulates Glut4 confinement in plasma membrane clusters in adipose cells. PLoS One 8:e57559. doi: 10.1371/journal.pone.0057559
Lu, S., Wu, X., Gao, Y., Gatlin, D. M., Wu, M., Yao, W., et al. (2018). Effects of dietary carbohydrate sources on growth, digestive enzyme activity, gene expression of hepatic GLUTs and key enzymes involved in glycolysis-gluconeogenesis of giant grouper Epinephelus lanceolatus larvae. Aquaculture 484, 343–350. doi: 10.1016/j.aquaculture.2017.07.033
Mao, Y. B. (2014). A Comparative Study on Glucose Tolerance Ability in Different Fish Species. Zhanjiang: Guangdong Ocean University.
Marchler-Bauer, A., Bo, Y., Han, L. Y., He, J. N., Lanczycki, C. J., Lu, S. N., et al. (2017). CDD/SPARCLE: functional classification of proteins via subfamily domain architectures. Nucleic Acids Res. 45, 200–203. doi: 10.1093/nar/gkw1129
Marín-Juez, R., Capilla, E., Carvalho-Simoes, F., Camps, M., and Planas, J. V. (2014). “Structural and functional evolution of glucose transporter 4(GLUT4): a look at GLUT4 in fish,” in Glucose Homeostasis, ed. L. Szablewski (London: IntechOpen Limited), 174. doi: 10.5772/58094
Mazur, C. N., Higgs, D. A., Plisetskaya, E. M., and March, B. E. (1992). Utilization of dietary starch and glucose tolerance in juvenile Chinook salmon (Oncorhynchus tshawytscha) of different strains in seawater. Fish Physiol. Biochem. 10, 303–313. doi: 10.1007/BF00004479
Ministry of Agriculture and Rural Affairs of the People’s Republic of China (2019). Chinese Fisheries Yearbook. Beijing: Chinese Agricultural Press.
Moon, T. W. (2001). Glucose intolerance in teleost fish: fact or fiction? Comp. Biochem. Physiol. B Biochem. Mol. Biol. 129, 243–249. doi: 10.1016/s1096-4959(01)00316-5
Pessin, J. E., Thurmond, D. C., Elmendorf, J. S., Coker, K. J., and Okada, S. (1999). Molecular basis of insulin-stimulated GLUT4 vesicle trafficking. Location! Location! Location! J. Biol Chem. 274, 2593–2596. doi: 10.1074/jbc.274.5.2593
Pfaffl, M. W. (2001). A new mathematical model for relative quantification in real-time RT-PCR. Nucleic Acids Res. 29, 2002–2007. doi: 10.1093/nar/29.9.e45
Planas, J. V., Capilla, E., and Gutiérrez, J. (2000). Molecular identification of a glucose transporter from fish muscle. FEBS Lett. 481, 266–270. doi: 10.1016/s0014-5793(00)02020-2
Polakof, S., Panserat, S., Soengas, J. L., and Moon, T. W. (2012). Glucose metabolism in fish: a review. J. Comp. Physiol. B 182, 1015–1045. doi: 10.1007/s00360-012-0658-7
Ren, M. C., Habte-Tsion, H. M., Liu, B., Miao, L. H., Ge, X. P., and Xie, J. (2017). Dietary isoleucine requirement of juvenile blunt snout bream. Megalobrama amblycephala. Aquac. Nutr. 23, 322–330. doi: 10.1111/anu.12396
Ren, M. C., Habte-Tsion, H. M., Xie, J., Liu, B., Zhou, Q. L., Ge, X. P., et al. (2015). Effects of dietary carbohydrate source on growth performance, diet digestibility and liver glucose enzyme activity in blunt snout bream. Megalobrama amblycephala. Aquaculture 438, 75–81. doi: 10.1016/j.aquaculture.2015.01.008
Ronner, P., and Scarpa, A. (1984). Difference in glucose dependency of insulin and somatostatin release. Am. J. Physiol. 246, E506–E509. doi: 10.1152/ajpendo.1984.246.6.E506
Rose, A. J., and Richer, E. A. (2005). Skeletal muscle glucose uptake during exercise: how is it regulated? Physiology 20, 260–270. doi: 10.1152/physiol.00012.2005
Saitou, N., and Nei, M. (1987). The neighbor-joining method: a new method for reconstructing phylogenetic trees. Mol. Biol. Evol. 4, 406–425. doi: 10.1093/oxfordjournals.molbev.a040454
Shi, Y. S. (1997). Utilization of carbohydrate in warmwater fish with particular reference to tilapia, Oreochromis niloticus x O. aureus. Aquaculture 151, 79–96. doi: 10.1016/S0044-8486(96)01491-3
Shiau, S. Y., and Chuang, J. C. (1995). Utilization of disaccharides by juvenile tilapia, Oreochromis niloticus X O. aureus. Aquaculture 133, 249–256. doi: 10.1016/0044-8486(95)00018-W
Slot, J. W., Geuze, H. J., Gigengack, S., James, D. E., and Lienhard, G. E. (1991). Translocation of the glucose transporter GLUT4 in cardiac myocytes of the rat. Proc. Natl. Acad. Sci. U.S.A. 88, 7815–7819. doi: 10.1073/pnas.88.17.7815
Stone, D. A. J. (2003). Dietary carbohydrate utilization by fish. Rev. Fish. Sci. 11, 337–369. doi: 10.1080/10641260390260884
Teerijoki, H., Krasnov, A., Pitkänen, T. I., and Mölsä, H. (2000). Cloning and characterization of glucose transporter in teleost fish rainbow trout (Oncorhynchus mykiss). Biochim. Biophys. Acta 1494, 290–294. doi: 10.1016/s0167-4781(00)00216-5
Viegas, I., Rito, J., González, J. D., Jarak, I., Carvalho, R. A., Metón, I., et al. (2013). Effects of food-deprivation and refeeding on the regulation and sources of blood glucose appearance in European seabass (Dicentrarchus labrax L.). Comp. Biochem. Physiol. A Mol. Integr. Physiol. 166, 399–405. doi: 10.1016/j.cbpa.2013.07.013
Wilson, R. P., and Poe, W. E. (1987). Apparent inability of channel catfish to utilize dietary mone-and disaccharides as energy sources. J. Nutr. 117, 280–285. doi: 10.1093/jn/117.2.280
Wright, J. R. J., O’Hali, W., Yang, H., Han, X. X., and Bonen, A. (1998). GLUT-4 Deficiency and severe peripheral resistance to insulin in the teleost fish tilapia. Gen Comp. Endocrinol. 111, 20–27. doi: 10.1006/gcen.1998.7081
Zhou, P. P., Wang, M. Q., Xie, F. J., Deng, D. F., and Zhou, Q. C. (2016). Effects of dietary carbohydrate to lipid ratios on growth performance, digestive enzyme and hepatic carbohydrate metabolic enzyme activities of large yellow croaker (Larmichthys crocea). Aquaculture 452, 45–51. doi: 10.1016/j.aquaculture.2015.10.010
Zhou, Z., Ren, Z., Zeng, H., and Yao, B. (2008). Apparent digestibility of various feedstuffs for blunt snout bream, Megalobrama amblycephala. Aquac. Nutr. 4, 153–165. doi: 10.1111/j.1365-2095.2007.00515.x
Zorzano, A., Palacín, M., and Gumà, A. (2005). Mechanisms regulating GLUT4 glucose transporter expression and glucose transport in skeletal muscle. Acta. Physiol. Scand. 183, 43–58. doi: 10.1111/j.1365-201X.2004.01380.x
Keywords: blunt snout bream, glucose transporter 4, glycometabolism, delay action, insulin
Citation: Liang H, Maulu S, Ji K, Ge X, Ren M and Mi H (2020) Functional Characterization of Facilitative Glucose Transporter 4 With a Delay Responding to Plasma Glucose Level in Blunt Snout Bream (Megalobrama amblycephala). Front. Physiol. 11:582785. doi: 10.3389/fphys.2020.582785
Received: 13 July 2020; Accepted: 15 September 2020;
Published: 15 October 2020.
Edited by:
Jianmin Zhao, Yantai Institute of Coastal Zone Research, ChinaReviewed by:
Erchao Li, Hainan University, ChinaChunnuan Zhang, Henan University of Science and Technology, China
Copyright © 2020 Liang, Maulu, Ji, Ge, Ren and Mi. This is an open-access article distributed under the terms of the Creative Commons Attribution License (CC BY). The use, distribution or reproduction in other forums is permitted, provided the original author(s) and the copyright owner(s) are credited and that the original publication in this journal is cited, in accordance with accepted academic practice. No use, distribution or reproduction is permitted which does not comply with these terms.
*Correspondence: Mingchun Ren, cmVubWNAZmZyYy5jbg==; Haifeng Mi, bWloZkB0b25nd2VpLmNvbQ==