- Department of Plant Protection, Institute of Vegetables and Flowers, Chinese Academy of Agricultural Sciences, Beijing, China
Deoxythymidine triphosphate (dTTP) is essential for DNA synthesis and cellular growth in all organisms. Here, genetic capacity analysis of the pyrimidine pathway in insects and their symbionts revealed that dTTP is a kind of metabolic input in several host insect/obligate symbiont symbiosis systems, including Bemisia tabaci MED/Candidatus Portiera aleyrodidarum (hereafter Portiera). As such, the roles of dTTP on both sides of the symbiosis system were investigated in B. tabaci MED/Portiera. Dietary RNA interference (RNAi) showed that suppressing dTTP production significantly reduced the density of Portiera, significantly repressed the expression levels of horizontally transferred essential amino acid (EAA) synthesis-related genes, and significantly decreased the reproduction of B. tabaci MED adults as well as the hatchability of their offspring. Our results revealed the regulatory role of dTTP in B. tabaci MED/Portiera and showed that dTTP synthesis-related genes could be potential targets for controlling B. tabaci as well as other sucking pests.
Introduction
Sucking insects harbor intracellular symbionts such as obligate symbionts and facultative symbionts, which affect the fitness of their host in many ways (Baumann, 2005; Ferrari and Vavre, 2011; Douglas, 2015). These intracellular symbionts provide essential nutrients for their hosts (Zientz et al., 2004; Douglas, 2015), protect their hosts from natural enemies and stress (Oliver et al., 2003; Oliver and Higashi, 2019; Zhang et al., 2019) and suppress the plant defense of their hosts (Frago et al., 2012). Because obligate symbionts have reduced genomes and lose many functional genes (McCutcheon and Moran, 2011; Moran and Bennett, 2014), they generally require their host to provide metabolic inputs (Martinez-Cano et al., 2014; Wilson and Duncan, 2015). To date, metabolic inputs supplied by hosts have been shown to directly participate in the metabolism of obligate symbionts. For example, Portiera relies on host-provided phosphoenolpyruvic acid, erythrose-4P, pyruvate and 5-phosphoribosyl diphosphate for synthesizing EAA (Xie et al., 2018). Similar trends have also been identified in other complementary EAA biosynthesis pathways in the Acyrthosiphon pisum/Buchnera aphidicola (hereafter Buchnera) symbiosis system (Wilson et al., 2010), Pachypsylla venusta/Carsonella ruddii symbiosis system (Sloan et al., 2014), Planococcus citri/Tremblaya princeps symbiosis system (Husnik et al., 2013), and Nilaparvata lugens/yeast-like symbiont symbiosis system (Xue et al., 2014). In addition to directly participating in the metabolism of obligate symbionts, the regulatory role of metabolic inputs has also been revealed. A previous study reported that cystathionine input determined methionine production in the A. pisum/Buchnera symbiosis system (Russell et al., 2014).
The compound dTTP, which is produced by thymidylate synthase via de novo biosynthesis or thymidine kinase via pyrimidine salvage, is a kind of pyrimidine nucleotide and is essential for housed bacteria as well as bacterial pathogens (Samant et al., 2008; Hashimoto et al., 2012; Wilson et al., 2012; Leija et al., 2016; Yang et al., 2017). As free-living bacteria encode all genes involved in dTTP synthesis, dTTP is not frequently considered an essential exogenous nutrient in bacteria (Turnbough and Switzer, 2008). However, several obligate symbionts of insects have been reported to be unable to produce dTTP (Zientz et al., 2004; Degnan et al., 2011; Moran and Bennett, 2014; Waterworth et al., 2020). As there is a great demand for dTTP throughout the life cycle of symbionts, these findings indicated that dTTP is likely a kind of metabolic input in several insect/obligate symbiont symbiosis systems. Among dTTP synthetic genes, it has been shown that thymidylate synthase and thymidine kinase served key roles (Carter, 1956; Hartman and Buchanan, 1959). Considering that functions of the two genes have been revealed by previous publications which indicated the two genes are critical for the growth of bacterial pathogens (Fivian-Hughes et al., 2012; Hashimoto et al., 2012; Wilson et al., 2012; Leija et al., 2016; Yang et al., 2017), thymidylate synthase and thymidine kinase have been put forward as molecular targets for bacterial pathogens in recent years (Choi et al., 2016; Leija et al., 2016).
Bemisia tabaci, which comprises a number of cryptic species (De Barro et al., 2011) and causes huge economic lost (Liu et al., 2012; Ning et al., 2015; Pan et al., 2015), harbors an obligate symbiont, Portiera, as well complexes of facultative symbionts (Chiel et al., 2007; Pan et al., 2012b). To date, the genomes of several B. tabaci cryptic species and their symbionts have been sequenced (Jiang et al., 2012; Rao et al., 2012a,b; Santos-Garcia et al., 2012, 2015; Chen et al., 2016, 2019; Xie et al., 2017, 2018). Additionally, several horizontally transferred EAA biosynthesis-related genes have been identified in B. tabaci (Luan et al., 2015; Chen et al., 2016; Xie et al., 2018). In terms of compensating for Portiera gene loss in EAA biosynthesis (Luan et al., 2015; Xie et al., 2018), those genes provided an easy route for evaluating EAA biosynthetic levels in obligate symbiont. As EAA biosynthesis is the majority metabolism in Portiera (Baumann, 2005; Luan et al., 2015; Xie et al., 2018), those HTGs provided an easy route for evaluating metabolism of Portiera.
The genomes of many sucking insects and those of their obligate symbionts are currently available, so global views on various metabolic interactions, such as metabolic inputs, in insect/obligate symbiont symbiosis systems are feasible (Douglas, 2018). In this study, genetic capacity analysis of the pyrimidine pathway, especially dTTP, was first performed in several obligate symbionts and host insects. The B. tabaci MED/Portiera symbiosis system was then applied to investigate the roles of dTTP supplied on both sides of the symbiosis system. Two dTTP synthesis-related genes of B. tabaci MED, thymidylate synthase (BtTS) and thymidine kinase (BtTK), were identified and cloned. By the silencing of these two genes, the influences of blocking dTTP production on Portiera density and EAA biosynthesis were investigated. Furthermore, the possible involvement of these two genes in B. tabaci MED management was also investigated.
Materials and Methods
Genetic Capacity Analysis of the Pyrimidine Pathway in Insects and Their Obligate Symbionts
For genetic capacity analysis of the pyrimidine pathway, genes that are involved in these processes were identified as follows. Initially, predicted protein sets of B. tabaci, Portiera, and other selected species (detailed information of which is shown in Supplementary Tables 1, 2) were annotated by KOBAS 2.0 (Wu et al., 2006). Genes that are part of the pyrimidine pathway were then selected. In addition, the sequences of known pyrimidine pathway-related proteins of A. pisum, Apis mellifera, Bombyx mori, Aedes aegypti, Drosophila melanogaster, Escherichia coli K-12 MG1655, Bacillus subtilis subsp. subtilis 168 and Staphylococcus aureus subsp. aureus N315 (MRSA/VSSA) were also used as queries to search against both predicted proteomes (BLASTP, e-value cutoff of 1E-3) and genome assembly sequences (TBLASTN, e-value cutoff of 1E-5) of selected species (Camacho et al., 2009). In the end, all hits were pooled and verified by searching via BLAST against the nr (NCBI) database.
Insect Strain
Bemisia tabaci MED was collected from fields in Beijing, China, in 2009. Since then, the B. tabaci MED insects have been reared on cotton (Gossypium herbaceum L. cv. Zhongmian 49) in a glasshouse (Pan et al., 2012a). Every three generations, the purity of the strain was monitored as previously described (Chu et al., 2010).
RNA Isolation, Gene Cloning and Quantitative Real-Time PCR Assays
Total RNA of each sample was extracted by using TRIzol reagent (Invitrogen, Carlsbad, CA, United States) following the manufacturer’s instructions. The integrity of the extracted RNA was checked with 1% agarose gel electrophoresis. The quality of the extracted RNA was then measured via a NanoDrop 2000 instrument (Thermo Scientific, Wilmington, DE, United States), and the concentration of each RNA sample was adjusted to 1 μg/μL.
For gene cloning, RNA from the B. tabaci MED whole body was used to prepare cDNA with a PrimeScript II First strand cDNA Synthesis Kit (Takara, Dalian, China). Gene-specific full-length primers (the primer pairs used are listed in Supplementary Table 3) were designed. The PCR was performed on an S1000 Thermal Cycler PCR System (Applied Biosystems, Foster City, CA, United States) using La Taq (Takara, Dalian, China). After amplification, bands of the expected size were purified and sequenced.
For qRT-PCR assays, each RNA was used to prepare cDNA with a PrimeScript RT Kit containing gDNA Eraser (Takara, Dalian, China). Gene-specific primers were then designed (the primer pairs used are listed in Supplementary Table 3). The qRT-PCR assay was performed on a QuantStudio 3 device (Applied Biosystems, Foster City, United States) using 2 × SuperReal PreMix Plus reagent (Tiangen, Beijing, China). Elongation factor 1 alpha (EF1α) was used as a reference gene (Li et al., 2013), and expression variation among samples was evaluated via the 2–ΔΔCt method (Livak and Schmittgen, 2001).
RNAi Construct and Gene Silencing
The RNAi constructs were generated and applied as previously described (Yang et al., 2016), and dsRNA for enhanced green fluorescent protein (EGFP) was used as a negative control. Before performing the RNAi assay, dsRNA for BtTS, BtTK and EGFP was prepared using a T7 RiboMAX Express RNAi system (Promega, Madison, United States) (the primers used are listed in Supplementary Table 3) and dissolved in 200 μL of artificial diet [100 μg of dsRNA, 5% yeast extract and 30% sucrose (wt/vol)]. The artificial diet was then fed to B. tabaci MED adults (mixture of both females and males) in feeding chambers. Each treatment involved six feeding chambers, and 70 newly emerged B. tabaci MED adults were introduced into each feeding chamber. The feeding chambers were then incubated in an environmental chamber at 25°C under a photoperiod of 14 L:10 D and a relative humidity (RH) of 70%.
Determining EAA Biosynthesis Rates and Portiera Density
After silencing for 2 and 4 days, the mortality of B. tabaci MED adults was recorded. The surviving B. tabaci MED adults from each replication were then collected and divided into two groups. The first group contained 25 B. tabaci MED adults that were used to determine the RNAi silencing efficiency and the effects of silenced targeted genes on the expression of EAA biosynthesis-related genes. Thirty of the other surviving B. tabaci MED adults constituted the second group and were used to determine the Portiera density of each whitefly individual. Before determining the Portiera density of the individual insects, genomic DNA of each individual insect was extracted as previously described (Zheng et al., 2017). The density of Portiera in the B. tabaci MED adults was then assessed via a QuantStudio 3 device (Applied Biosystems, United States) using 2 × SuperReal PreMix Plus (Tiangen, Beijing, China). The protocol for the density of Portiera determination was the same as that described previously (Shan et al., 2016). The relative density of Portiera was quantified via their 16S rRNA gene, while the β-actin gene (nuclear gene) of the whiteflies was used as an internal standard for normalization. All the primer pairs used are listed in Supplementary Table 3.
Impact of BtTS and BtTK on Reproduction and Offspring Hatchability
To assess the effects of suppressing dTTP production on B. tabaci MED females, BtTS and BtTK of B. tabaci MED females were first knocked down via RNAi as described above. At 2 days after the induction of silencing, five B. tabaci MED females were released into one clip cages on health cotton plants. Three days after the release of the females, the number of eggs that were deposited on the leaves within the clip cages were counted. Later, egg hatchability was calculated. Each clip cage was considered one biological replicate, and each treatment had 20 replicates.
Statistical Analysis
SPSS version 23.0 (SPSS Inc., Chicago, IL, United States) was used for statistical analysis. Differences among treatments were evaluated by one-way ANOVA in conjunction with Tukey’s test (p < 0.05).
Results
Genetic Capacity Analysis of the Pyrimidine Pathway in Selected Insects and Symbionts
To ensure whether dTTP was a metabolic input that originated from host insects, genome-wide genetic capacity analysis of the pyrimidine pathway was performed. The results showed that Portiera encodes only one gene involved in the pyrimidine pathway (Figure 1), while B. tabaci MED encodes all the genes involved in the pyrimidine pathway (Figure 2). The results also showed that among selected obligate symbionts, only those of aphids (labeled Buchnera), stink bugs (labeled Pantoea) and tsetse flies (labeled Wigglesworthia) encode the whole set of genes for uridine monophosphate biosynthesis. No selected obligate symbiont was found to encode a complete set of genes for the pyrimidine salvage pathway and pyrimidine degradation (Figure 1). Though several obligate symbionts lacked the majority of pyrimidine pathway genes, their host insects still encoded them (Figure 2).
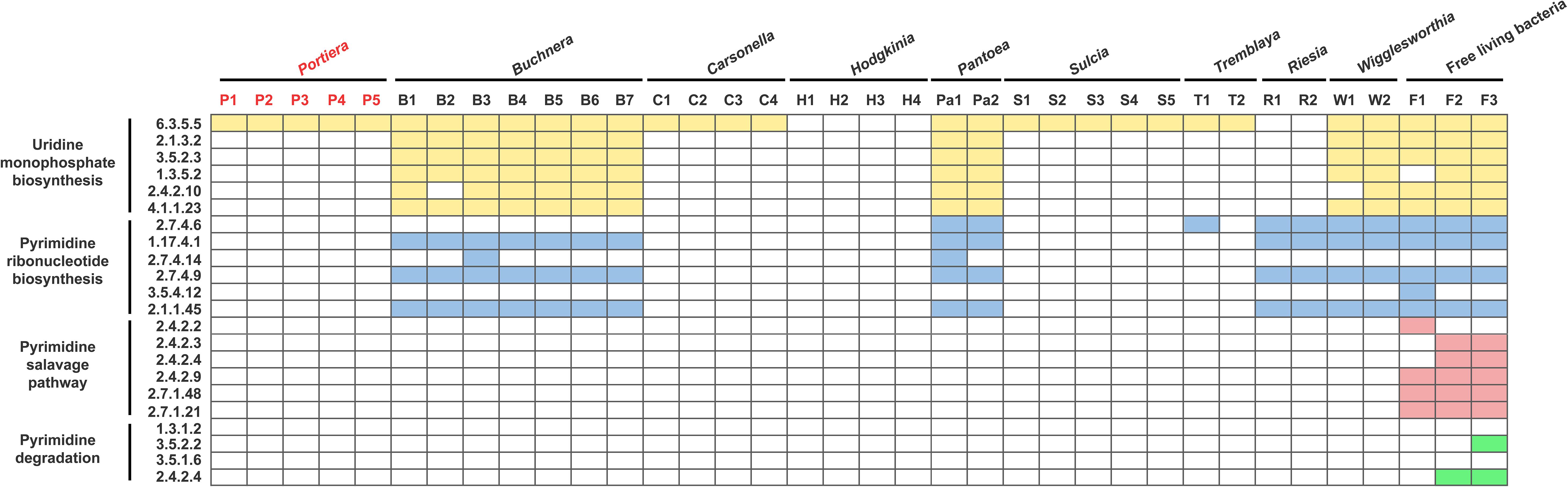
Figure 1. Content of genes involved in the pyrimidine pathway of obligate symbionts. The boxes representing genes involved in uridine monophosphate biosynthesis are colored yellow; pyrimidine ribonucleotide biosynthesis, blue; the pyrimidine salvage pathway, red; and pyrimidine degradation, green. The biosynthetic processes are shown in their reaction order. The EC number of each gene is also shown. For the obligate symbiont Portiera, the genes present are colored red. Detailed information on the select symbionts is listed in Supplementary Table 1.
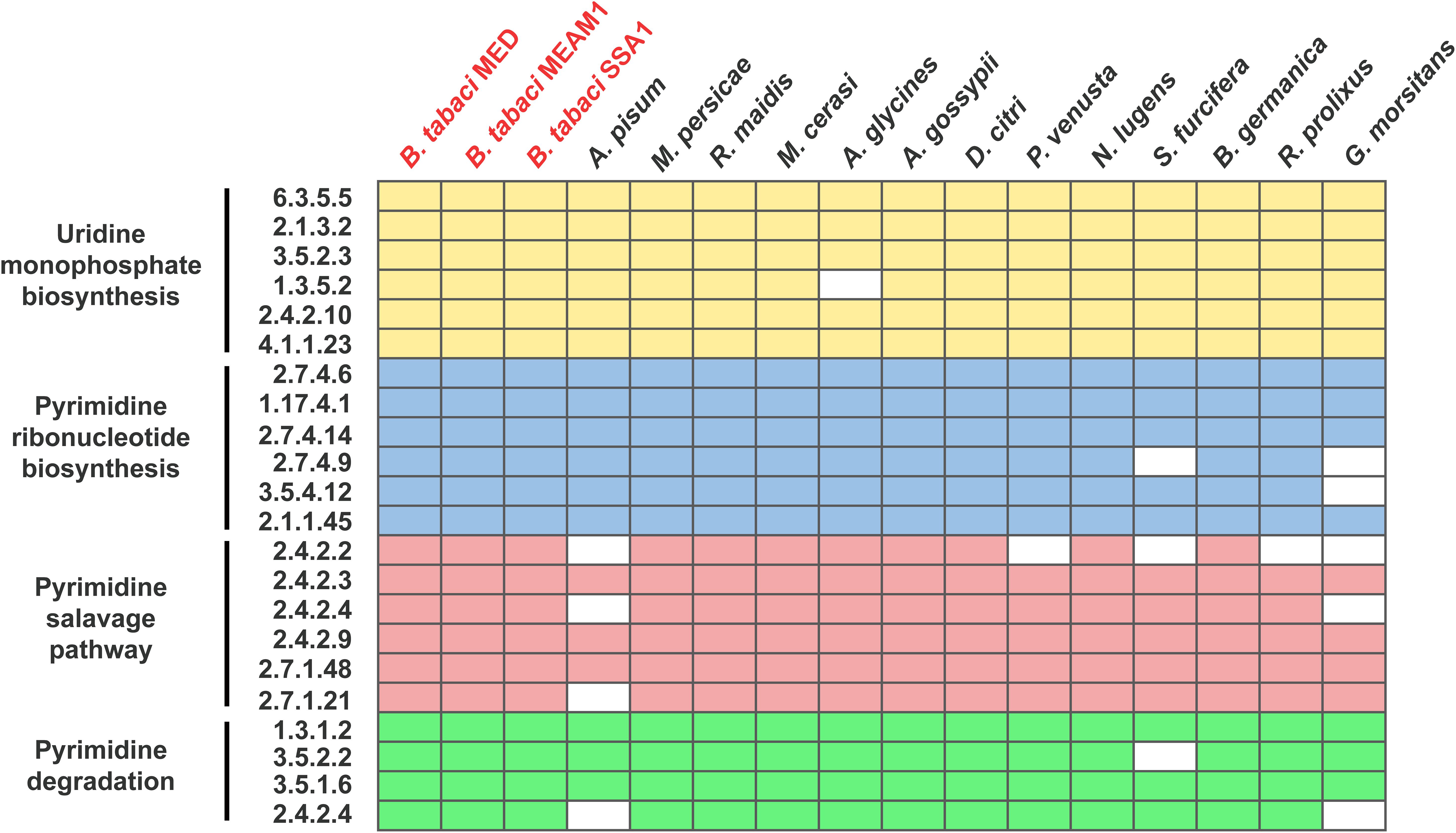
Figure 2. Content of genes involved in the pyrimidine pathway of host insects. The boxes representing genes involved in uridine monophosphate biosynthesis are colored yellow; pyrimidine ribonucleotide biosynthesis, blue; the pyrimidine salvage pathway, red; and pyrimidine degradation, green. Biosynthetic processes are shown in their linear order. The EC number of each gene is also shown. B. tabaci is colored red. Detailed information on the select insects is listed in Supplementary Table 2.
Cloning, Phylogenetic Analysis and Expression Pattern Analysis
Before investigating the impacts of dTTP on Portiera, two important dTTP synthetic genes, BtTS and BtTK, were firstly cloned (Figures 3A,D). Phylogenetic analysis showed that BtTS and BtTK were relatively clustered with their homologous proteins in hemipteran insects (Figures 3B,E). The expression patterns of two genes showed that BtTS was most highly expressed in 3rd nymph stage while BtTK was most highly expressed in 1st and 2nd nymph stage (Figures 3C,F).
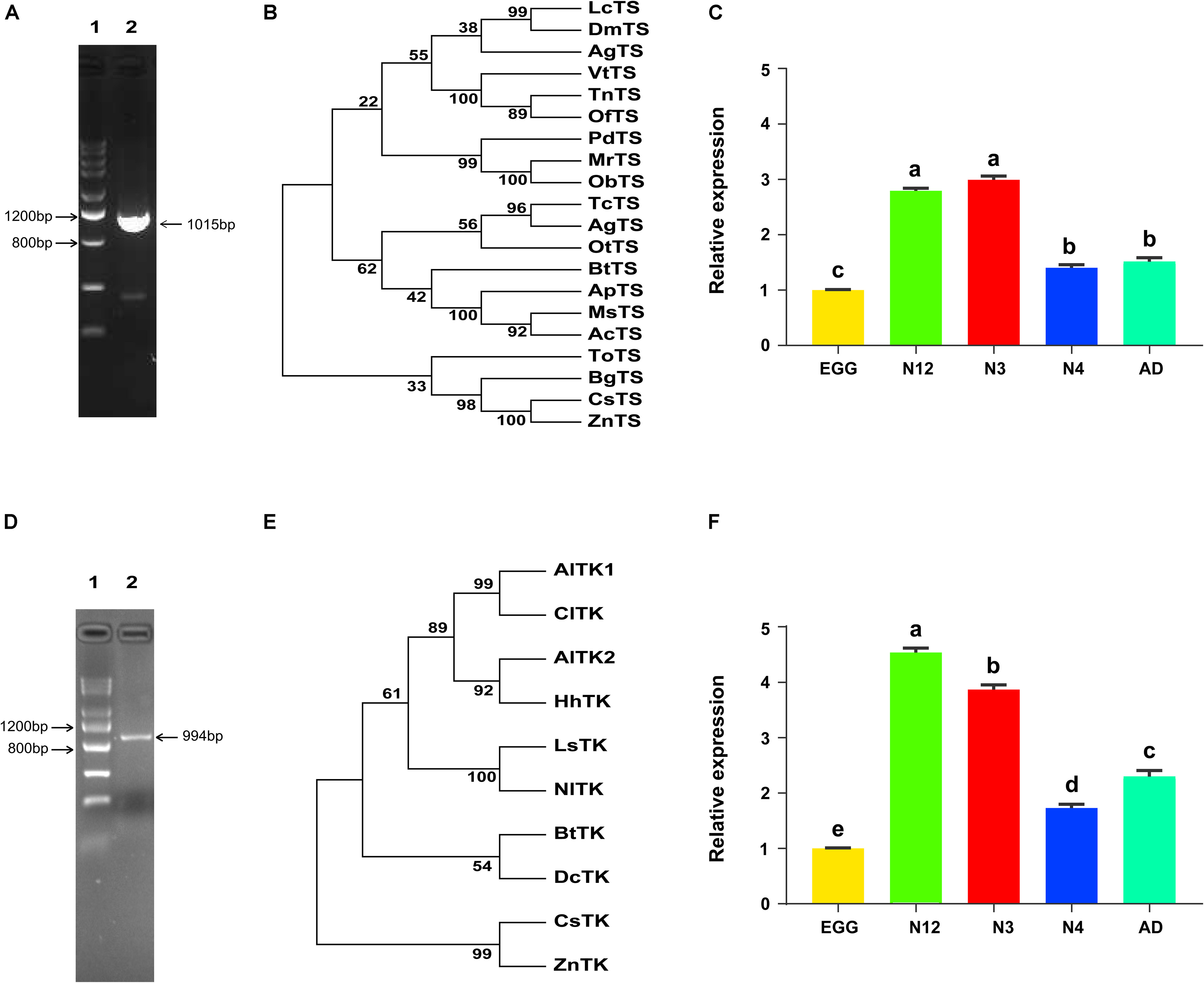
Figure 3. Cloning, phylogenetic analysis and expression patterns of both BtTS and BtTK. (A,D) Complete coding sequence amplification of BtTS and BtTK from MED. For panel (A), line 1, Tiangen DNA marker III; line 2, BtTS. For panel (D), line 1, Tiangen DNA marker III; line 2, BtTK. (B,E) Phylogenetic tree of BtTS and BtTK from MED. LcTS, Lucilia cuprina, XP_023302452.1; DmTS, Drosophila melanogaster, NP_001285570.1; AgTS, Anopheles gambiae, XP_311491.3; VtTS, Vanessa tameamea, XP_026491376.1; TnTS, Trichoplusia ni, XP_026747379.1; OfTS, Ostrinia furnacalis, XP_028163869.1; PdTS, Polistes dominula, XP_015173877.1; MrTS, Megachile rotundata, XP_003705109.1; ObTS, Osmia bicornis, XP_029051538.1; TcTS, Tribolium castaneum, XP_008191570.1; AgTS, Anoplophora glabripennis, XP_018568721.1; OtTS, Onthophagus taurus, XP_022918259.1; ApTS, Acyrthosiphon pisum, NP_001155666.1; MsTS, Melanaphis sacchari, XP_025205323.1; AcTS, Aphis craccivora, KAF0760635.1; FoTS, Frankliniella occidentalis, KAE8752504.1; BgTS, Blattella germanica, PSN45388.1; CsTS, Cryptotermes secundus, XP_023702879.1; ZnTS, Zootermopsis nevadensis, XP_021913580.1; AlTK1, Apolygus lucorum, KAE9428612.1; ClTK, Cimex lectularius, XP_014254368.1; AlTK2, Apolygus lucorum, KAE9423970.1; HhTK, Halyomorpha halys, XP_014279550.1; LsTK, Laodelphax striatellus, RZF40272.1; NlTK, Nilaparvata lugens, XP_022188850.1; DcTK, Diaphorina citri, XP_026679347.1; CsTK, Cryptotermes secundus, XP_023714579.1; ZnTK, Zootermopsis nevadensis, KDR10834.1. (C,F) Expression patterns of BtTS and BtTK across developmental stages. Egg, egg stage; N12, first and second nymph; N3, third nymph; N4. fourth nymph; AD, adult. The values shown are the means and standard errors, and the different letters indicate treatment differences at p < 0.05 (one-way ANOVA with Tukey’s test).
Impact of BtTS and BtTK Silencing on EAA Biosynthesis and Portiera Density
To determine the effects of silencing BtTS and BtTK on B. tabaci MED adults and Portiera, RNAi constructs were applied to B. tabaci MED adults. The qRT-PCR results showed that upon silencing for 2 days (BtTS, F2,15 = 99.100, p < 0.0001; BtTK, F2,15 = 124.046, p < 0.0001) and 4 days (BtTS, F2,15 = 338.345, p < 0.0001; BtTK, F2,15 = 256.058, p < 0.0001), the expression levels of BtTS and BtTK significantly decreased (Figures 4A,C). Compared with control treatments in which B. tabaci MED adults were fed a normal diet or dsEGFP, feeding B. tabaci MED adults with dsBtTS and dsBtTK significantly decreased the expression levels of EAA biosynthesis-related genes (Figures 4B,D and Supplementary Table 4). For BtTS, silencing for 2 days (F3,716 = 7.236, p = 0.015) and 4 days (F3,716 = 5.880, p = 0.027) significantly reduced the density of Portiera (Figures 5A,B). The density of Portiera was also significantly reduced when BtTK was silenced for 2 days (p = 0.003) and 4 days (p = 0.033). Knocking down BtTS (2 days, F3,20 = 0.131, p = 0.999; 4 days, F3,20 = 0.144, p = 0.942) and BtTK (2 days, p = 0.989; 4 days, p = 0.992) did not cause a significant lethal effect (Figures 6A,B).
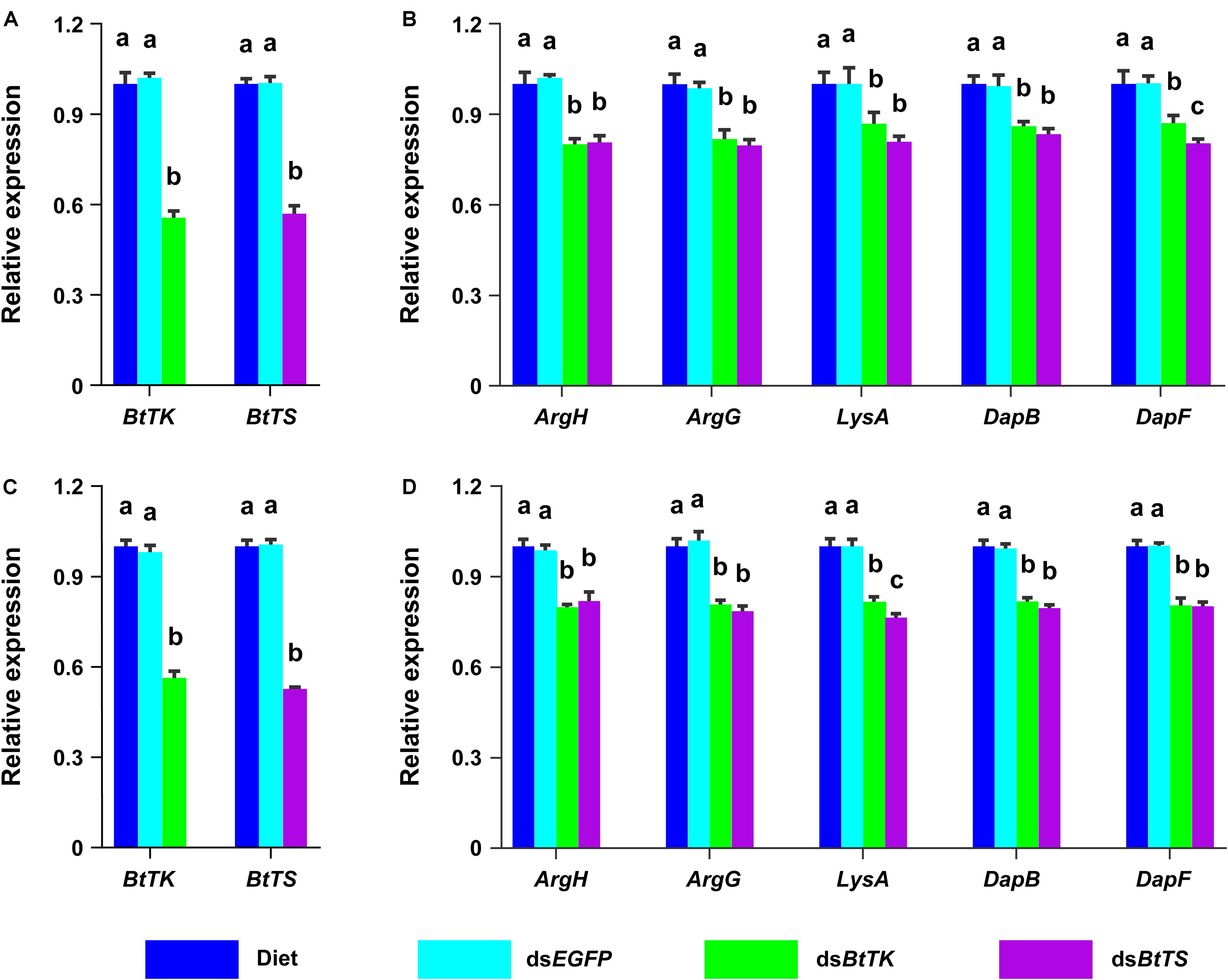
Figure 4. Temporal expression profiles of BtTK, BtTS, and EAA biosynthetic HTGs in B. tabaci MED treated with dsRNA or artificial diet. (A) Expression profiles of BtTK and BtTS in B. tabaci MED that had been treated for 2 days with RNAi constructs. (B) Expression profiles of EAA biosynthetic HTGs in B. tabaci MED after 2 days of treatment. (C) Expression profiles of BtTK and BtTS in B. tabaci MED after 4 days of treatment. (D) Expression profiles of EAA biosynthetic HTGs in B. tabaci MED after 4 days of treatment. For each gene, transcript levels in group of adults fed the artificial diet were normalized to one. The values shown are the means and standard errors, and the different letters indicate treatment differences at p < 0.05 (one-way ANOVA with Tukey’s test).
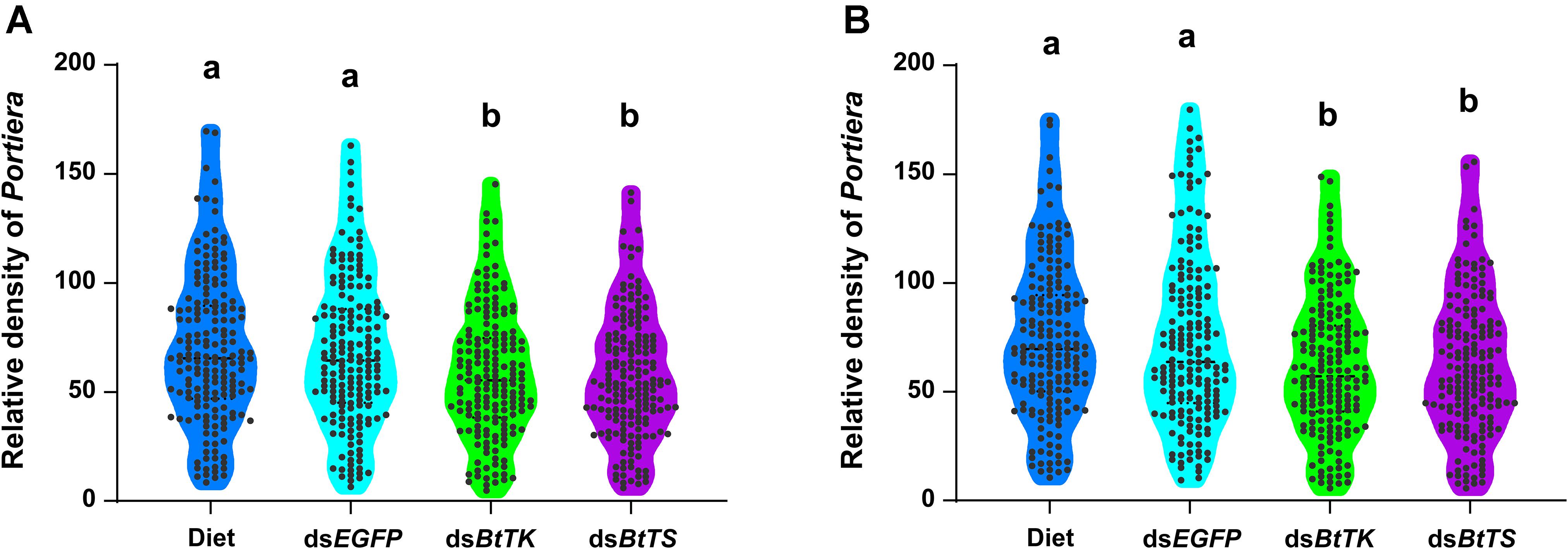
Figure 5. Temporal dynamics of Portiera in B. tabaci MED fed dsRNA or artificial diet. (A) Density of Portiera in B. tabaci MED after 2 days of treatment. (B) The density of Portiera in B. tabaci MED after 4 days of treatment. The changes in symbiont density were measured in terms of the number of 16S rRNA gene copies per β-actin gene copy. The different letters indicate treatment differences at p < 0.05 (one-way ANOVA with Tukey’s test).
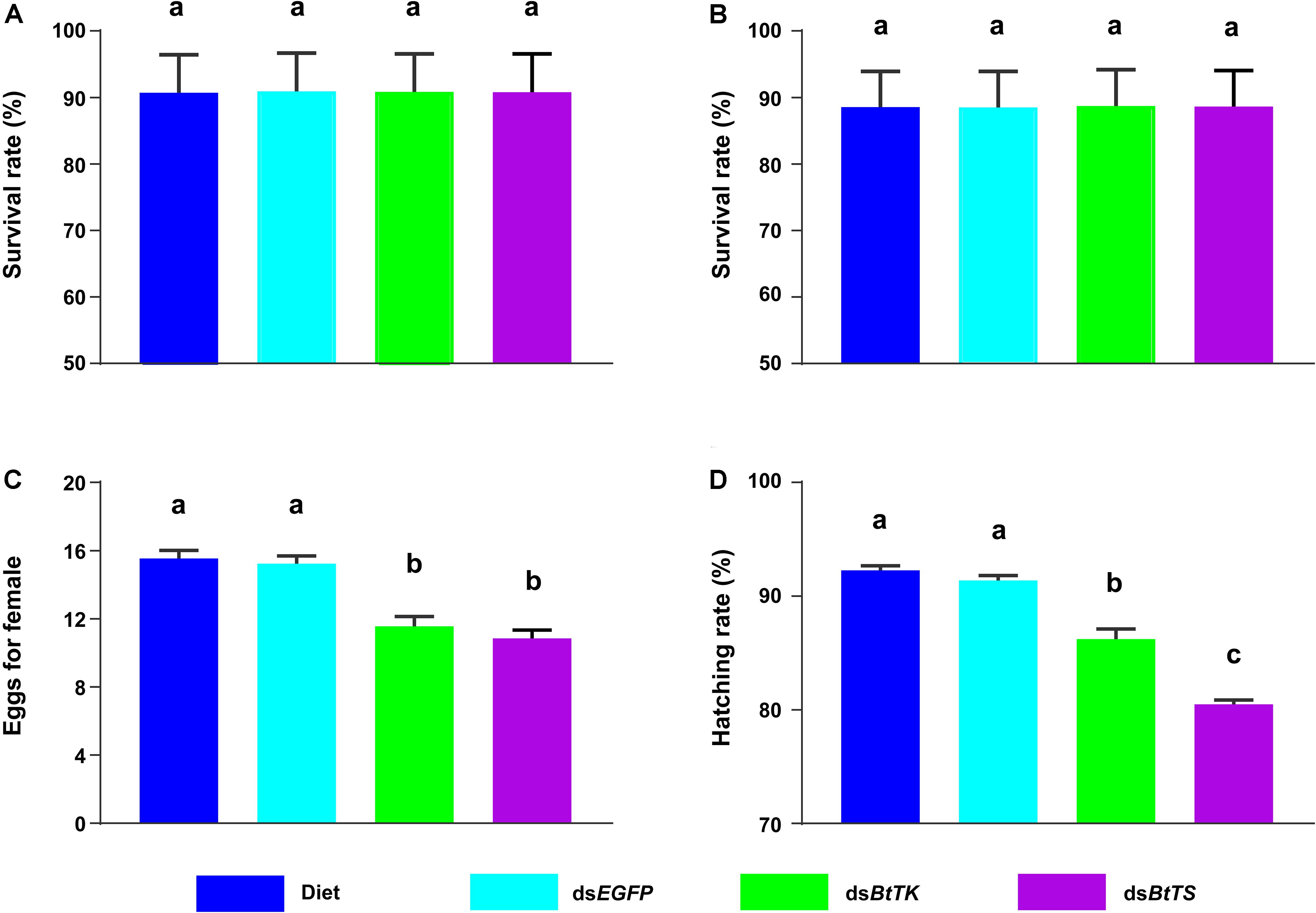
Figure 6. Effects of BtTK and BtTS silencing on B. tabaci MED. (A) Survival of B. tabaci MED adults upon treatment with dsRNA or artificial diet for 2 days. (B) Survival of B. tabaci MED adults after treatment with dsRNA or artificial diet for 4 days. (C) Effects of dsRNA or artificial diet on the egg production of B. tabaci females. (D) Effects of dsRNA or artificial diet on the hatchability of B. tabaci offspring. The values shown are the means and standard errors, and the different letters indicate treatment differences at p < 0.05 (one-way ANOVA with Tukey’s test).
Impact of BtTS and BtTK on Female Production and Offspring Hatchability
The effects of silencing BtTS and BtTK on the fitness of B. tabaci MED were also measured. Suppressing BtTS significantly decreased the reproduction of B. tabaci MED females (F3,76 = 23.986, p < 0.0001, Figure 6C) and the hatchability of B. tabaci MED offspring (F3,76 = 91.020, p < 0.0001, Figure 6D). Furthermore, suppressing BtTK also significantly decreased the reproduction of B. tabaci MED females (p < 0.0001, Figure 6C) and the hatchability of B. tabaci MED offspring (p < 0.0001, Figure 6D). Though no significant difference in the reproduction of B. tabaci MED females was observed between the dsBtTS-treated groups and dsBtTK-treated groups (p = 0.730), suppressing BtTK expression more significantly reduced hatchability than did suppressing BtTS expression (p < 0.0001; Figure 6D).
Discussion
Here, a genetic capacity survey revealed that many obligate symbionts required their insects’ hosts to supply dTTP. Two dTTP synthesis-related genes, BtTS and BtTK, were then identified and cloned. Later, the influences of blocking dTTP production on Portiera and B. tabaci MED were investigated. Our results showed that suppressing dTTP production greatly repressed EAA biosynthesis, significantly decreased Portiera density and caused a serious decline in B. tabaci MED.
Because of genomic decay, obligate symbionts rely on their hosts to supply metabolic inputs for synthesizing essential nutrients (Moran et al., 2008; McCutcheon and Moran, 2011; Moran and Bennett, 2014). To date, host-supplied metabolic inputs have been largely reported in the EAA biosynthesis of obligate symbionts (as described in the introduction). Here, we found that many selected obligate symbionts such as Portiera were not able to produce dTTP, while their host insects retained such capacities. In view of the great demand for dTTP across whole life cycles, our study revealed that, except for EAA biosynthesis, dTTP is another kind of metabolic input in several insect/obligate symbiont symbiosis systems.
The metabolic input cystathionine was previously reported to determine methionine production in A. pisum/Buchnera symbiosis systems (Russell et al., 2014). It has also been proposed that metabolic inputs control obligate symbiont growth in insects (Ankrah et al., 2018). Here, our results showed that blocking dTTP production repressed the expression level of EAA synthetic HTGs and significantly reduced the density of Portiera. Given that those HTGs compensated for the gene loss of Portiera and that the majority of reactions of EAA biosynthesis were still afforded by Portiera (Luan et al., 2015; Xie et al., 2018), our observations indicated that suppressing BtTS and BtTK slowed the EAA biosynthesis of Portiera. Taken together, our results indicated that dTTP is applied as a regulator to control obligate symbionts in the B. tabaci MED/Portiera symbiosis system.
In addition, we also observed that blocking dTTP production significantly decreased the reproduction of B. tabaci MED adults and the hatchability of their offspring. The supply of enough EAAs is essential for insect reproduction (Roy et al., 2018; Toshima and Schleyer, 2019). EAAs also construct vitellogenin, which is a nutrient needed in egg hatching (Franz, 1979). As Portiera supplies EAAs for B. tabaci MED (Baumann, 2005; Xie et al., 2018), the negative effects we observed were likely caused by the fact that blocking dTTP production reduced the density of Portiera as well as EAA biosynthesis. As B. tabaci causes severe unfitness, BtTS and BtTK were suggested to be potential targets for symbiont-targeted B. tabaci MED management. Antibiotics have also been suggested to serve as novel pesticides for symbiont-targeted B. tabaci MED management (Zhang et al., 2015). Though also causing a series of negative effects on B. tabaci MED, the release of high concentrations of antibiotics was shown to take a heavy toll on the environment, such as influencing the structures and activities of microbes in the environment (Martinez, 2009). As silencing the two targeted genes blocked dTTP production only in B. tabaci MED and was not detrimental to environmental microbes, it seems that managing B. tabaci MED by silencing dTTP synthesis-related genes such as BtTS and BtTK is more friendly to the environment than managing B. tabaci MED by using antibiotics.
In addition to B. tabaci MED, other sucking insects such as psyllids and mealybugs are also important agricultural pests and harbor obligate symbionts (Baumann, 2005). Since obligate symbionts are essential for the survival of their hosts (Husnik et al., 2013; Sloan et al., 2014) and cannot be acquired from the environment (Koga et al., 2012; Luan et al., 2018), these obligate symbionts have been proposed as novel targets for pest control (Douglas, 2015). Here, we showed that the two dTTP synthesis-related genes that produce the dTTP that regulates the growth and metabolism of Portiera were potential targets for symbiont-targeted B. tabaci MED management. Interestingly, we also found that obligate symbionts of psyllids and mealybugs lack the capacities for biosynthesising dTTP. The results indicated that those symbionts may also require their hosts to supply dTTP. As dTTP is essential for cellular growth that is required throughout the whole life cycle, it is probable that genes involved in dTTP production could also serve as molecular targets for psyllid and mealy bug management.
In summary, we showed that suppressing dTTP production caused a series of negative effects on both Portiera and B. tabaci MED. Our results indicated that two dTTP synthetic genes, BtTS and BtTK, could be used as molecular targets for B. tabaci MED management. The study demonstrated a regulatory mechanism in the MED/Portiera system and likely revealed new molecular targets for whitefly pests and even management of other sucking insects.
Data Availability Statement
All datasets presented in this study are included in the article/Supplementary Material.
Author Contributions
ZY and YZ designed the research. YZ and CG conceived the experiments. YZ, YH, and JX analyzed the data. ZY and JZ drafted the manuscript. WX, ZG, XY, WX, SW, QW, and YZ revised and finalized the manuscript. All authors contributed to the article and approved the submitted version.
Funding
This research was supported by the National Key R&D Program of China (2019YFD1002100), the National Natural Science Foundation of China (31672032), China Agriculture Research System (CARS-24-C-02), China Postdoctoral Science Foundation (2018M641562), and the Beijing Key Laboratory for Pest Control and Sustainable Cultivation of Vegetables and the Science and Technology Innovation Program of the Chinese Academy of Agricultural Sciences (CAAS-ASTIP-IVFCAAS).
Conflict of Interest
The authors declare that the research was conducted in the absence of any commercial or financial relationships that could be construed as a potential conflict of interest.
Supplementary Material
The Supplementary Material for this article can be found online at: https://www.frontiersin.org/articles/10.3389/fphys.2020.574749/full#supplementary-material
References
Ankrah, N. Y. D., Chouaia, B., and Douglas, A. E. (2018). The cost of metabolic interactions in symbioses between insects and bacteria with reduced genomes. MBio 9:e1433–18. doi: 10.1128/mBio.01433-18
Baumann, P. (2005). Biology bacteriocyte-associated endosymbionts of plant sap-sucking insects. Annu. Rev. Microbiol. 59, 155–189. doi: 10.1146/annurev.micro.59.030804.121041
Camacho, C., Coulouris, G., Avagyan, V., Ma, N., Papadopoulos, J., Bealer, K., et al. (2009). BLAST+: architecture and applications. BMC Bioinform. 10:421. doi: 10.1186/1471-2105-10-421
Carter, C. E. (1956). Metabolism of purines and pyrimidines. Annu. Rev. Biochem. 25, 123–146. doi: 10.1146/annurev.bi.25.070156.001011
Chen, W., Wosula, E. N., Hasegawa, D. K., Casinga, C., Shirima, R. R., Fiaboe, K. K. M., et al. (2019). Genome of the African cassava whitefly Bemisia tabaci and distribution and genetic diversity of cassava-colonizing whiteflies in Africa. Insect Biochem. Mol. Biol. 110, 112–120. doi: 10.1016/j.ibmb.2019.05.003
Chen, W. B., Hasegawa, D. K., Kaur, N., Kliot, A., Pinheiro, P. V., Luan, J. B., et al. (2016). The draft genome of whitefly Bemisia tabaci MEAM1, a global crop pest, provides novel insights into virus transmission, host adaptation, and insecticide resistance. BMC Biol. 14:110. doi: 10.1186/s12915-016-0321-y
Chiel, E., Gottlieb, Y., Zchori-Fein, E., Mozes-Daube, N., Katzir, N., Inbar, M., et al. (2007). Biotype-dependent secondary symbiont communities in sympatric populations of Bemisia tabaci. Bull. Entomol. Res. 97, 407–413. doi: 10.1017/s0007485307005159
Choi, M., Karunaratne, K., and Kohen, A. (2016). Flavin-dependent thymidylate synthase as a new antibiotic target. Molecules 21:654. doi: 10.3390/molecules21050654
Chu, D., Wan, F. H., Zhang, Y. J., and Brown, J. K. (2010). Change in the biotype composition of Bemisia tabaci in Shandong Province of China from 2005 to 2008. Environ. Entomol. 39, 1028–1036. doi: 10.1603/EN09161
De Barro, P. J., Liu, S. S., Boykin, L. M., and Dinsdale, A. B. (2011). Bemisia tabaci: a statement of species status. Annu. Rev. Entomol. 56, 1–19. doi: 10.1146/annurev-ento-112408-085504
Degnan, P. H., Ochman, H., and Moran, N. A. (2011). Sequence conservation and functional constraint on intergenic spacers in reduced genomes of the obligate symbiont Buchnera. PLoS Genet. 7:e1002252. doi: 10.1371/journal.pgen.1002252
Douglas, A. E. (2015). Multiorganismal insects: diversity and function of resident microorganisms. Annu. Rev. Entomol. 60, 17–34. doi: 10.1146/annurev-ento-010814-020822
Douglas, A. E. (2018). Omics and the metabolic function of insect-microbial symbioses. Curr. Opin. Insect Sci. 29, 1–6. doi: 10.1016/j.cois.2018.05.012
Ferrari, J., and Vavre, F. (2011). Bacterial symbionts in insects or the story of communities affecting communities. Philos. Trans. R. Soc. Lond. B Biol. Sci. 366, 1389–1400. doi: 10.2307/41148993
Fivian-Hughes, A. S., Houghton, J., and Davis, E. O. (2012). Mycobacterium tuberculosis thymidylate synthase gene thyX is essential and potentially bifunctional, while thyA deletion confers resistance to p-aminosalicylic acid. Microbiology 158:308. doi: 10.1099/mic.0.053983-0
Frago, E., Dicke, M., and Godfray, H. C. (2012). Insect symbionts as hidden players in insect-plant interactions. Trends Ecol. Evol. 27, 705–711. doi: 10.1016/j.tree.2012.08.013
Franz, E. (1979). Insect vitellogenin: identification, biosynthesis, and role in vitellogenesis. Adv. In Insect Phys. 14, 49–108. doi: 10.1016/S0065-2806(08)60051-X
Hartman, S. C., and Buchanan, J. M. (1959). Nucleic acids, purines, pyrimidines (nucleotide synthesis). Annu. Rev. Biochem. 28, 365–410. doi: 10.1146/annurev.bi.28.070159.002053
Hashimoto, M., Morales, J., Fukai, Y., Suzuki, S., Takamiya, S., Tsubouchi, A., et al. (2012). Critical importance of the de novo pyrimidine biosynthesis pathway for Trypanosoma cruzi growth in the mammalian host cell cytoplasm. Biochem. Bioph. Res. Co. 417, 1002–1006. doi: 10.1016/j.bbrc.2011.12.073
Husnik, F., Nikoh, N., Koga, R., Ross, L., Duncan, R. P., Fujie, M., et al. (2013). Horizontal gene transfer from diverse bacteria to an insect genome enables a tripartite nested mealybug symbiosis. Cell 153, 1567–1578. doi: 10.1016/j.cell.2013.05.040
Jiang, Z. F., Xia, F., Johnson, K. W., Bartom, E., Tuteja, J. H., Stevens, R., et al. (2012). Genome sequences of the primary endosymbiont “Candidatus Portiera aleyrodidarum” in the whitefly Bemisia tabaci B and Q biotypes. J. Bacteriol. 194, 6678–6679. doi: 10.1128/JB.01841-12
Koga, R., Meng, X. Y., Tsuchida, T., and Fukatsu, T. (2012). Cellular mechanism for selective vertical transmission of an obligate insect symbiont at the bacteriocyte-embryo interface. Proc. Natl. Acad. Sci. U.S.A. 109, E1230–E1237. doi: 10.1073/pnas.1119212109
Leija, C., Rijo-Ferreira, F., Kinch, L. N., Grishin, N. V., Nischan, N., Kohler, J. J., et al. (2016). Pyrimidine salvage enzymes are essential for de novo biosynthesis of deoxypyrimidine nucleotides in Trypanosoma brucei. PLoS Pathog. 12:e1006010. doi: 10.1371/journal.ppat.1006010
Li, R., Xie, W., Wang, S., Wu, Q., Yang, N., Yang, X., et al. (2013). Reference gene selection for qRT-PCR analysis in the sweetpotato whitefly, Bemisia tabaci (Hemiptera: Aleyrodidae). PLoS One 8:e53006. doi: 10.1371/journal.pone.0053006
Liu, B., Yan, F., Chu, D., Pan, H., Jiao, X., Xie, W., et al. (2012). Difference in feeding behaviors of two invasive whiteflies on host plants with different suitability: implication for competitive displacement. Int. J Biol. Sci. 8, 697–706. doi: 10.7150/ijbs.4108
Livak, K. J., and Schmittgen, T. D. (2001). Analysis of relative gene expression data using real-time quantitative PCR and the 2–ΔΔCt Method. Methods 25, 402–408. doi: 10.1006/meth.2001.1262
Luan, J., Sun, X., Fei, Z., and Douglas, A. E. (2018). Maternal inheritance of a single somatic animal cell displayed by the bacteriocyte in the whitefly Bemisia tabaci. Curr. Biol. 28, 459–465. doi: 10.1016/j.cub.2017.12.041
Luan, J. B., Chen, W., Hasegawa, D. K., Simmons, A. M., Wintermantel, W. M., Ling, K. S., et al. (2015). Metabolic coevolution in the bacterial symbiosis of whiteflies and related plant sap-feeding insects. Genome Biol. Evol. 7, 2635–2647. doi: 10.1093/gbe/ew170
Martinez, J. L. (2009). Environmental pollution by antibiotics and by antibiotic resistance determinants. Environ. Pollut. 157, 2893–2902. doi: 10.1016/j.envpol.2009.05.051
Martinez-Cano, D. J., Reyes-Prieto, M., Martinez-Romero, E., Partida-Martinez, L. P., Latorre, A., Moya, A., et al. (2014). Evolution of small prokaryotic genomes. Front. Microbiol. 5:742. doi: 10.3389/fmicb.2014.00742
McCutcheon, J. P., and Moran, N. A. (2011). Extreme genome reduction in symbiotic bacteria. Nat. Rev. Microbiol. 10, 13–26. doi: 10.1038/nrmicro2670
Moran, N. A., and Bennett, G. M. (2014). The tiniest tiny genomes. Annu. Rev. Microbiol. 68, 195–215. doi: 10.1146/annurev-micro-091213-112901
Moran, N. A., McCutcheon, J. P., and Nakabachi, A. (2008). Genomics and evolution of heritable bacterial symbionts. Annu. Rev. Genet. 42, 165–190. doi: 10.1146/annurev.genet.41.110306.130119
Ning, W., Shi, X., Liu, B., Pan, H., Wei, W., Zeng, Y., et al. (2015). Transmission of tomato yellow leaf curl virus by Bemisia tabaci as affected by whitefly sex and biotype. Sci. Rep. 5:10744. doi: 10.1038/srep10744
Oliver, K. M., and Higashi, C. H. (2019). Variations on a protective theme: Hamiltonella defensa infections in aphids variably impact parasitoid success. Curr. Opin. Insect Sci. 32, 1–7. doi: 10.1016/j.cois.2018.08.009
Oliver, K. M., Russell, J. A., Moran, N. A., and Hunter, M. S. (2003). Facultative bacterial symbionts in aphids confer resistance to parasitic wasps. Proc. Natl. Acad. Sci. U. S. A. 100, 1803–1807. doi: 10.1073/pnas.0335320100
Pan, H., Chu, D., Yan, W., Su, Q., Liu, B., Wang, S., et al. (2012a). Rapid spread of tomato yellow leaf curl virus in China is aided differentially by two invasive whiteflies. PloS One 7:e34817. doi: 10.1371/journal.pone.0034817
Pan, H., Li, X., Ge, D., Wang, S., Wu, Q., Xie, W., et al. (2012b). Factors affecting population dynamics of maternally transmitted endosymbionts in Bemisia tabaci. PloS One 7:e30760. doi: 10.1371/journal.pone.0030760
Pan, H., Preisser, E., Chu, D., Wang, S., Wu, Q., Yves, C., et al. (2015). Insecticides promote viral outbreaks by altering herbivore competition. Ecol. Appl 25, 1585–1595. doi: 10.1890/14-0752.1
Rao, Q., Wang, S., Su, Y. L., Bing, X. L., Liu, S. S., and Wang, X. W. (2012a). Draft genome sequence of “Candidatus Hamiltonella defensa”, an endosymbiont of the whitefly Bemisia tabaci. J. Bacteriol. 194:3558. doi: 10.1128/JB.00069-12
Rao, Q., Wang, S., Zhu, D. T., Wang, X. W., and Liu, S. S. (2012b). Draft genome sequence of Rickettsia sp. strain MEAM1, isolated from the whitefly Bemisia tabaci. J. Bacteriol. 194, 4741–4742. doi: 10.1128/JB.00909-12
Roy, S., Saha, T. T., Zou, Z., and Raikhel, A. S. (2018). Regulatory pathways controlling female insect reproduction. Annu. Rev. Entomol. 63, 489–511. doi: 10.1146/annurev-ento-020117-043258
Russell, C. W., Poliakov, A., Haribal, M., Jander, G., van Wijk, K. J., and Douglas, A. E. (2014). Matching the supply of bacterial nutrients to the nutritional demand of the animal host. Proc. Royal. Soc. B 281:20141163. doi: 10.1098/rspb.2014.1163
Samant, S., Lee, H., Ghassemi, M., Chen, J., Cook, J. L., Mankin, A. S., et al. (2008). Nucleotide biosynthesis is critical for growth of bacteria in human blood. PLoS Pathog. 4:e37. doi: 10.1371/journal.ppat.0040037
Santos-Garcia, D., Farnier, P. A., Beitia, F., Zchori-Fein, E., Vavre, F., Mouton, L., et al. (2012). Complete genome sequence of “Candidatus Portiera aleyrodidarum” BT-QVLC, an obligate symbiont that supplies amino acids and carotenoids to Bemisia tabaci. J. Bacteriol. 194, 6654–6655. doi: 10.1128/JB.01793-12
Santos-Garcia, D., Vargas-Chavez, C., Moya, A., Latorre, A., and Silva, F. J. (2015). Genome evolution in the primary endosymbiont of whiteflies sheds light on their divergence. Genome Biol. Evol. 7, 873–888. doi: 10.1093/gbe/evv038
Shan, H. W., Zhang, C. R., Yan, T. T., Tang, H. Q., Wang, X. W., Liu, S. S., et al. (2016). Temporal changes of symbiont density and host fitness after rifampicin treatment in a whitefly of the Bemisia tabaci species complex. Insect Sci. 23, 200–214. doi: 10.1111/1744-7917.12276
Sloan, D. B., Nakabachi, A., Richards, S., Qu, J., Murali, S. C., Gibbs, R. A., et al. (2014). Parallel histories of horizontal gene transfer facilitated extreme reduction of endosymbiont genomes in sap-feeding insects. Mol. Biol. Evol. 31, 857–871. doi: 10.1093/molbev/msu004
Toshima, N., and Schleyer, M. (2019). Neuronal processing of amino acids in Drosophila: from taste sensing to behavioural regulation. Curr. Opin. Insect Sci. 36, 39–44. doi: 10.1016/j.cois.2019.07.007
Turnbough, C. L., and Switzer, R. L. (2008). Regulation of pyrimidine biosynthetic gene expression in bacteria: repression without repressors. Microbiol. Mol. Biol. R. 72, 266–300. doi: 10.1128/MMBR.00001-08
Waterworth, S. C., Flórez, L. V., Rees, E. R., Hertweck, C., Kaltenpoth, M., and Kwan, J. C. (2020). Horizontal gene transfer to a defensive symbiont with a reduced genome in a multipartite beetle microbiome. MBio 11:e02430–19. doi: 10.1101/780619
Wilson, A. C., Ashton, P. D., Calevro, F., Charles, H., Colella, S., Febvay, G., et al. (2010). Genomic insight into the amino acid relations of the pea aphid with its symbiotic bacterium Buchnera aphidicola. Insect Mol. Biol. 19(Suppl. 2) 249–258. doi: 10.1111/j.1365-2583.2009.00942.x
Wilson, A. C., and Duncan, R. P. (2015). Signatures of host/symbiont genome coevolution in insect nutritional endosymbioses. Proc. Natl. Acad. Sci. U.S.A. 112, 10255–10261. doi: 10.1073/pnas.1423305112
Wilson, Z. N., Gilroy, C. A., Boitz, J. M., Ullman, B., and Yates, P. A. (2012). Genetic Dissection of pyrimidine biosynthesis and salvage in Leishmania donovani. J. Biol. Chem. 287, 12759–12770. doi: 10.1074/jbc.M112.346502
Wu, J., Mao, X., Cai, T., Luo, J., and Wei, L. (2006). KOBAS server: a web-based platform for automated annotation and pathway identification. Nucleic Acids Res. 34, 720–724. doi: 10.1093/nar/gkl167
Xie, W., Chen, C., Yang, Z., Guo, L., Yang, X., Wang, D., et al. (2017). Genome sequencing of the sweetpotato whitefly Bemisia tabaci MED/Q. Gigascience 6, 1–7. doi: 10.1093/gigascience/gix018
Xie, W., Yang, X., Chen, C., Yang, Z., Guo, L., Wang, D., et al. (2018). The invasive MED/Q Bemisia tabaci genome: a tale of gene loss and gene gain. BMC Genom. 19:68. doi: 10.1186/s12864-018-4448-9
Xue, J., Zhou, X., Zhang, C. X., Yu, L. L., Fan, H. W., Wang, Z., et al. (2014). Genomes of the rice pest brown planthopper and its endosymbionts reveal complex complementary contributions for host adaptation. Genome Biol. 15:521. doi: 10.1186/s13059-014-0521-0
Yang, H. J., Bogomolnaya, L., McClelland, M., and Andrews-Polymenis, H. (2017). De novo pyrimidine synthesis is necessary for intestinal colonization of Salmonella Typhimurium in chicks. PLoS One 12:e0183751. doi: 10.1371/journal.pone.0183751
Yang, X., He, C., Xie, W., Liu, Y., Xia, J., Yang, Z., et al. (2016). Glutathione S-transferases are involved in thiamethoxam resistance in the field whitefly Bemisia tabaci Q (Hemiptera: Aleyrodidae). Pestic. Biochem. Physiol. 134, 73–78. doi: 10.1016/j.pestbp.2016.04.003
Zhang, B., Leonard, S. P., Li, Y., and Moran, N. A. (2019). Obligate bacterial endosymbionts limit thermal tolerance of insect host species. Proc. Natl. Acad. Sci. U.S.A. 116, 24712–24718. doi: 10.1073/pnas.1915307116
Zhang, C. R., Shan, H. W., Xiao, N., Zhang, F. D., Wang, X. W., Liu, Y. Q., et al. (2015). Differential temporal changes of primary and secondary bacterial symbionts and whitefly host fitness following antibiotic treatments. Sci. Rep. 5:15898. doi: 10.1038/srep15898
Zheng, H. X., Xie, W., Wang, S. L., Wu, Q. J., Zhou, X. M., and Zhang, Y. J. (2017). Dynamic monitoring (B versus Q) and further resistance status of Q-type Bemisia tabaci in China. Crop Prot. 94, 115–122. doi: 10.1016/j.cropro.2016.11.035
Keywords: Bemisia tabaci MED, candidatus portiera aleyrodidarum, deoxythymidine triphosphate, RNAi, pest control
Citation: Yang Z, Gong C, Hu Y, Zhong J, Xia J, Xie W, Yang X, Guo Z, Wang S, Wu Q and Zhang Y (2021) Two Deoxythymidine Triphosphate Synthesis-Related Genes Regulate Obligate Symbiont Density and Reproduction in the Whitefly Bemisia tabaci MED. Front. Physiol. 11:574749. doi: 10.3389/fphys.2020.574749
Received: 21 June 2020; Accepted: 04 September 2020;
Published: 24 February 2021.
Edited by:
Peng He, Guizhou University, ChinaReviewed by:
Huipeng Pan, South China Agricultural University, ChinaHongbo Jiang, Southwest University, China
Copyright © 2021 Yang, Gong, Hu, Zhong, Xia, Xie, Yang, Guo, Wang, Wu and Zhang. This is an open-access article distributed under the terms of the Creative Commons Attribution License (CC BY). The use, distribution or reproduction in other forums is permitted, provided the original author(s) and the copyright owner(s) are credited and that the original publication in this journal is cited, in accordance with accepted academic practice. No use, distribution or reproduction is permitted which does not comply with these terms.
*Correspondence: Youjun Zhang, emhhbmd5b3VqdW5AY2Fhcy5jbg==
†These authors have contributed equally to this work