- 1Institute of Mountain Emergency Medicine, Eurac Research, Bolzano, Italy
- 2Healthcare Research and Innovation Program, IRCS-HTA, Bruno Kessler Foundation, Trento, Italy
- 3ITER Center for Balance and Rehabilitation Research (ICBRR), Rome, Italy
Technological advancements are opening the possibility of prolonged monitoring of physiological parameters under daily-life conditions, with potential applications in sport science and medicine, and in extreme environments. Among emerging wearable technologies, in-ear devices or hearables possess technical advantages for long-term monitoring, such as non-invasivity, unobtrusivity, good fixing, and reduced motion artifacts, as well as physiological advantages related to the proximity of the ear to the body trunk and the shared vasculature between the ear and the brain. The present scoping review was aimed at identifying and synthesizing the available evidence on the use and performance of in-ear monitoring of physiological parameters, with focus on applications in sport science, sport medicine, occupational medicine, and extreme environment settings. Pubmed, Scopus, and Web of Science electronic databases were systematically searched to identify studies conducted in the last 10 years and addressing the measurement of three main physiological parameters (temperature, heart rate, and oxygen saturation) in healthy subjects. Thirty-nine studies were identified, 24 performing temperature measurement, 12 studies on heart/pulse rate, and three studies on oxygen saturation. The collected evidence supports the premise of in-ear sensors as an innovative and unobtrusive way for physiological monitoring during daily-life and physical activity, but further research and technological advancement are necessary to ameliorate measurement accuracy especially in more challenging scenarios.
Introduction
Physiological parameter trackers are an evolving technology that allow to monitor and collect physiological data, such as heart rate (HR), temperature (T), oxygen saturation (SpO2), and energy consumption (Bunn et al., 2019). These wearables are conceived for multiple uses from bench-to-bedside to daily-life conditions, and for recreational, clinical, and research purposes (Poh and Kittler, 2012; Leboeuf et al., 2014; Skaiaa et al., 2015; Strapazzon et al., 2015; Budidha and Kyriacou, 2018; Bunn et al., 2019). Wearables are appealing technologies in fields, such as sport science, sport medicine, occupational medicine, and other out-of-hospital medical situations, where device portability and unobtrusivity may represent key advantages.
To satisfy these settings, a device must be accurate and reliable under multiple environmental and activity conditions, and it should be also lightweight, easy to wear and handle, minimally invasive, and discrete to favor long-term monitoring (Leboeuf et al., 2014). Additional requirements may be needed to extend the use of these devices to settings with challenging environmental conditions. Since several physiological parameters are estimated by devices relying on photo-plethysmography (PPT, measurement of light absorbance/reflectance from the vascular bed) from different peripheral body areas, environmental conditions that seriously impact perfusion may compromise the reliability of measurements. To overcome these restrictions, the application of sensors on better-perfused areas has been proposed (Rosenberg and Pedersen, 1990; Clayton et al., 1991; Kyriacou, 2006; Budidha and Kyriacou, 2018). However, application sites on the skin may experience functional difficulties, such as attachment problems and motion artifacts (Clayton et al., 1991; Budidha and Kyriacou, 2018), while sites within body cavities (naso-pharyngeal, gastro-intestinal, etc.) may limit general applicability due to the expertise required for application (semi-invasivity) and potential discomfort.
The ear canal has been proposed as a promising measurement site for physiological parameters, potentially able to combine minimal invasivity and wearability with reliable and accurate recordings in different settings (Poh and Kittler, 2012; Budidha and Kyriacou, 2014; Leboeuf et al., 2014; Bunn et al., 2019). Thanks to its close position to the central nervous system and major vasculature, it can provide better signal quality and more stability. The area is slightly influenced by the sympathetic nerve activity in conditions leading to low perfusion states, resulting in an adequate blood flow, higher quality of PPT signals, and reliable pulse rate (PR) and SpO2 monitoring. The site provides good fixation and unobtrusivity, facilitating long-term monitoring in daily-life conditions, and it offers protection from challenging environmental conditions (low temperature, sunlight) for in-field applications in challenging conditions (Budidha and Kyriacou, 2014, 2015).
In this context, the aim of the present scoping review was to identify and summarize the studies conducted in the last 10 years on the measurements of physiological parameters from the human ear canal in healthy subjects. In particular, the search was focused on the monitoring of three basic parameters, such as T, HR/PR, and SpO2, in settings related to sport science and occupational medicine, including challenging environmental scenarios where such measurements may be hindered. The structure of the scoping review is the following. Sections Ear Anatomy and Vascularization and Measurement of Physiological Parameters From the Ear Canal provide basic introductory concepts on ear anatomy and vasculature and on the techniques applied for physiological monitoring from the ear canal. Section Scoping Review Methods describes the search strategy and data extraction process. Section Results summarizes the studies identified by the systematic search for the three main outcomes (i.e., T, HR/PR, and SpO2). For each parameter, a brief introduction of its importance in the field of sport science/medicine and occupational medicine is provided, together with the selective advantages of in-ear measurement in that context. Finally, Section Limitations and Future Developments provides a general discussion about potential limitations and pitfalls of hearables, as well as future challenges and perspectives of such technology.
Ear Anatomy and Vascularization
The measurement of physiological parameters from the ear canal in proximity of the tympanic membrane (TM) is strictly related to the characteristic vasculature of this area. The basic aspects of ear anatomy and vasculature are schematized in Figure 1. In terms of vasculature (Figure 1B), the brain and the TM are both supplied by the basilar artery and the internal carotid artery (Benzinger and Taylor, 1963; McCarthy and Heusch, 2006). The basilar artery supplies the TM via the internal auditory artery. The internal carotid artery provides blood to the TM via the artery of the pterygoid canal and the carotico-tympanic branch, which have anastomoses with a vascular circle formed by several branches of the external carotid artery, such as the anterior tympanic artery, posterior auricular artery, stylomastoid artery, and maxillary artery (Berkovitz, 2005; McCarthy and Heusch, 2006). The anastomoses with branches of the internal carotid arteries are crucial for monitoring physiological parameters. The internal carotid arteries (one on each side of the head) are the main blood supply to the circle of Willis and to the brain, including crucial sites for T regulation like the hypothalamus. In-ear measurement of core T assumes that the TM is supplied by blood from the same sources that supply the brain, which guarantees thermal equilibrium between the two sites. The measurement of SpO2 and PR mostly relies on the acquisition of PPT signals, whose quality is also related to blood supply. Ear vascularization can guarantee adequate flow even in pathophysiological situations, such as in accidentally hypothermic patients where even cerebral autoregulation is progressively lost (Paal et al., 2018; Gaasch et al., 2020).
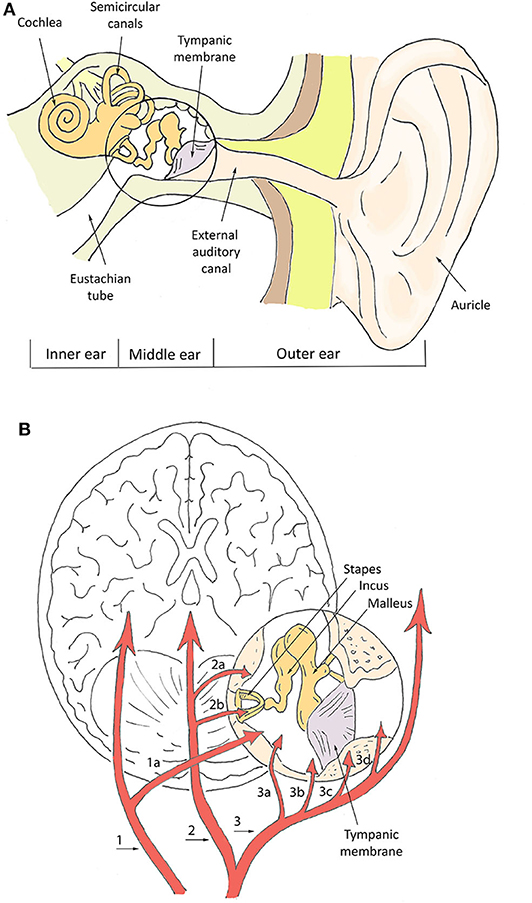
Figure 1. Schematization of ear canal anatomy (A) and vasculature (B). In (B), numbers indicate the main vessels and specifically: the basilar artery (1), internal auditory artery (1a), internal carotid artery (2), artery of pterygoid canal (2a), carotico-tympanic artery (2b), external carotid artery (3), maxillary artery (3a), stylomastoid artery (3b), posterior auricular artery (3c), anterior tympanic artery (3d).
On the other hand, given the frailty of the TM, some conditions affecting its integrity have been hypothesized to impact in-ear physiological measurements (Tasli and Gökgöz, 2018). Several studies showed that pathological conditions, such as acute external otitis, cerumen obturans, and previous major ear surgery may significantly alter the measurement of core T (García Callejo et al., 2004; Schmäl et al., 2006; Tasli and Gökgöz, 2018). Differently, small perforation (<10 mm) in the central low-irrorated area of the TM, otitis media, fluid in the middle ear, ventilation tubes, and minor surgery seem to have negligible effects (García Callejo et al., 2004; Schmäl et al., 2006; Tasli and Gökgöz, 2018).
Measurement of Physiological Parameters From the Ear Canal
The monitoring of T, HR/PR, and SpO2 from the ear canal relies on different measuring principles and devices, which are briefly summarized in Figure 2. T measurement relies on thermometric approaches, which work in direct or indirect mode. In direct mode thermometers, the output T corresponds to the T of the sensor, which is thermally coupled to the measuring site (Ring et al., 2010). Thermistor-based thermometers work in direct mode and utilize the intrinsic property of materials, such as metallic oxides, to change their electrical resistance as a function of T. Thermistor-based thermometers include a metallic probe, usually encapsulated in an impermeable material, such as a soft rubber earplug, an electronic circuitry to measure the resistance change and a microprocessor for calibration and data display (Chen, 2019). Potential limitations of direct approaches may include a slow time response to reach thermal equilibrium with the measuring site, and difficulties in placing the sensor close to the desired body site. In indirect or adjust-mode thermometers, the output T is the result of a signal adjustment or conversion, based on clinical data and physiological and anatomical properties, which corrects for differences between the measuring and sensor sites. The use of signal processing tools to estimate T results in fast response time, but may reduce actual accuracy (Ring et al., 2010). Infrared (IR) thermometers, the most widespread methodology to measure tympanic T, are adjusted-mode thermometers, based on the detection of the IR radiation emitted by the TM and its conversion to an electrical signal. IR tympanic thermometers (IRTT) are usually composed of a probe tip with lenses to focus the IR light, a sensing electronic module (thermopile or pyroelectric sensor) for signal transduction, and a microprocessor circuitry for data calibration and display (Chen, 2019). Although standard requirements for IRTT recommend clinical accuracy of ±0.3°C for target T in the range 33–42°C at ambient temperatures of 16–33°C (ISO 80601-2-56, 2017), actual accuracy in the clinical setting may be affected by several factors, related—but not limited—to patient variability (gender, age, thermoregulation, handedness), local phenomena (excessive cerumen, ear major infection or surgery, tissue cooling due to repeated measurements), operator's experience, device maintenance, and environmental conditions (humidity, temperature). The latter may particularly affect accuracy during in-field applications (Ring et al., 2010; Sund-Levander and Grodzinsky, 2013).
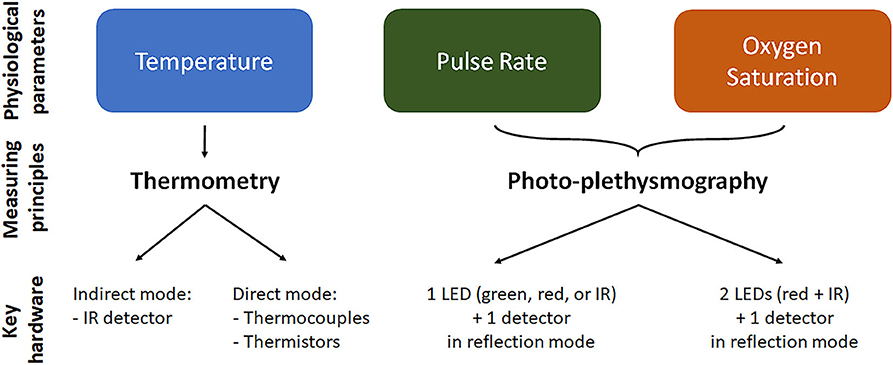
Figure 2. Physiological parameter measurement in the ear canal. For each of the parameter we reported the mainly used measurement technique and the key elements/working configuration of the measuring device. Temperature is measured by thermometry approach using indirect infrared (IR) thermometers or direct thermopile or thermocouple thermometers. Oxygen saturation and pulse rate are measured using photo-plethysmography, where light is emitted at two or one wavelength, respectively, and the reflected light is detected and analyzed. LED, light emitting diode.
The measurement of PR and SpO2 from the ear canal is mainly based on optical sensing techniques, although also piezo-electric sensors and IR thermography techniques have been proposed for PR measurements. In optical techniques, a peripheral site with high vascular density is illuminated by a light source and the light reflected by the vascular bed (PPG signal) is measured by a photo-detector (see Figure 2). The PPG signal is composed by a direct constant component, which includes absorption/reflectance due to arterial blood, venous blood, and capillaries, and by a pulsatile alternating component, which is synchronous with the heart beat and is representative of tissue perfusion (Foo et al., 2013). The pulsatile component can be directly used to estimate PR. Devices for in-ear PR measurement include as principal elements one light emitting diode (LED) for light emission at a single wavelength (red, IR, or green), one photo-detector to measure the reflected light, and a microprocessor to control the LED and calculate PR values (Poh et al., 2012). PR measurements using red or IR light can be affected by IR rays in sunlight, preventing stable operation for outdoors applications, thus indoors or semi-indoors usage is recommended. For outdoors PR measurement, a green light source is usually preferred, being less susceptibility to ambient light (Lemay et al., 2014; Parak, 2018).
With respect to PR, the measurement of SpO2 requires light emission and detection at two or more different wavelengths. SpO2 is defined as the fraction of oxyhemoglobin (i.e., hemoglobin bound with oxygen) to deoxyhemoglobin (i.e., hemoglobin not bound with oxygen), which can be optically distinguished by their distinct light absorbance/reflectance at different wavelengths (i.e., oxyhemoglobin absorbs greater amounts of IR and lower amounts of red light than deoxyhemoglobin). The simplest but conventional approach to measure SpO2 is to analyze the light of the two wavelengths reflected from the pulsatile added volume of the oxygenated arterial blood and, specifically, calculating the absorption rate given by the double ratio of the pulsatile and non-pulsatile components of red light to IR light reaching the photo-detector (Foo et al., 2013). Devices to measure SpO2 include two LEDs, emitting red and IR light, a photo-detector to measure the reflected light, and a microprocessor controlling led switching and converting absorption rate values into SpO2 values.
Standard requirements for optical assessment of physiological parameters (ISO 80601-2-61, 2017) recommend that PR accuracy is of ±3% in the clinical range 40–240 bpm, while SpO2 accuracy is ±2% in the clinical range 84–100% and ±3% in the clinical range 70–100%. Nevertheless, technical and physiological factors may interfere with optical measurements affecting accuracy. These factors include motion artifact from physical movement, misalignment/distance between the skin and the optical sensor, geometry of the sensor, variation in the intensity and spectrum of the light source, ambient light, variations in skin color/tone, size, and depth of the vascular area, poor tissue perfusion (Lemay et al., 2014; Parak, 2018; Bent et al., 2020). In particular, working in reflection mode, in-ear devices may be affected by high shunt light (i.e., the amount of direct light traveling from the LED to the detector without propagation over the pulsing blood in the biological tissue) and to the lower signal amplitude with respect to transmission mode signals (Budidha and Kyriacou, 2014; Lemay et al., 2014; Parak, 2018). To improve PPG signals, optimal designing of sensor geometry and LED-photodetector distance based on the used wavelength is crucial, but also alternative sensor configurations, such as “circummission” mode have been proposed (Buschmann and Huang, 2010).
Post-acquisition stages (e.g. filtering, amplification, and noise reduction modules), directly integrated in in-ear devices or in post-processing systems, are often required to condition the acquired signals and improve accuracy (Venema et al., 2012; Budidha and Kyriacou, 2014). The energy consumption of optical in-ear device is mainly related to LED driving, analog-to-digital conversion, and microprocessor operations (Vogel et al., 2009). Additional energy costs can be attributed to the presence of wireless communication modules for data transmission to other devices (e.g. smartphones and computers), where applications are available for data visualization and post-processing. Rechargeable long-term batteries in most devices usually guarantee hours of monitoring time.
Scoping Review Methods
The scoping review and the meta-analysis were conducted following the guidelines of the Preferred Reporting Items for Systematic Review and Meta-Analysis (PRISMA) extension for scoping reviews (PRISMA-ScR) (Tricco et al., 2018).
Eligibility Criteria
The literature search was performed to identify studies performing and assessing in-ear monitoring of physiological parameters, namely T, HR/PR, and SpO2, in the last 10 years. The search strategy design was schematized by the inclusion criteria (Table 1), categorized according to the broad Population—Concept—Context (PCC) mnemonic recommended for scoping reviews (Peters, 2016; Munn et al., 2018). The scoping review was focused on applications in physiological research, sport science, and occupational medicine, including challenging environmental conditions. Thus, only studies on healthy subjects during daily activities were included, while studies considering patients in clinical/rehabilitation care or monitoring of pathological conditions and sleep disorders were excluded. Studies were eligible only if physiological parameters were measured directly from the ear canal, while studies on devices simply worn on the auricle were excluded. Since the reliability of ear canal measurement was a relevant issue, studies were included only if reference/comparative measurements of the same variable were reported. The search was restricted to articles published in English in peer-reviewed journals. No restriction on study design was posed. Abstracts presentations, conference proceedings, and reviews were excluded.
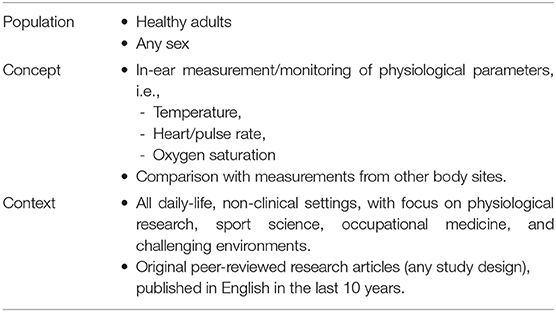
Table 1. Inclusion criteria for the scoping review summarized according to the Population-Concept-Context (PCC) mnemonic, recommended for scoping reviews (Peters, 2016; Munn et al., 2018).
Information Sources, Search Strategy, and Study Selection
A systematic search was performed in Pubmed, Scopus, and ISI Web of Science electronic databases to identify primary references from January 2010 to December 2019. The following search string was used: (“earbud” OR “earpiece” OR “earable” OR “hearable” OR “headphone” OR “ear device” OR “ear sensor” OR “in-ear device” OR “in-ear sensor” OR “ear canal sensor” OR “ear canal device” OR “ear canal” OR “tympanic device” OR “tympanic sensor”) AND (“physiological parameter” OR “physiological monitoring” OR “physiological index” OR “physiological signal” OR “vitals” OR “vital parameter” OR “vital sign” OR “temperature” OR “oxygen saturation” OR “oximetry” OR “oximeter” OR “photoplethysmography” OR “heart rate” OR “heart frequency”). The database search was followed by a review of the citations from eligible studies. Studies were selected based on title and abstract using the online platform Rayyan (Ouzzani et al., 2016). Selected studies were read thoroughly to identify those suitable for inclusion in the scoping review.
Data Extraction
Two reviewers (MM and AM) independently extracted the demographic and experimental data from the selected studies. When disagreement occurred, they reviewed the papers together to reach joint conclusions. For each study the following relevant information were extracted and summarized: the characteristics of the in-ear device under evaluation and of the reference and/or comparators; the experimental setting/protocol for the evaluation and the investigated study group; the main results of the study with focus on the device performance and/or critical issues.
Results
Study Selection
The database search identified a total of 447 relevant references once the duplicates were removed (Figure 3). A total of 394 references were excluded after reading title and abstract, and 53 were retrieved for further evaluation. Of these, 14 studies were excluded because they did not fulfill the inclusion criteria, mostly due to the absence of reference/comparator measurements (8 studies). Following the selection process, 39 studies were included in the scoping review. Of these, 24 studies performed a measurement of T, 12 studies of HR/PR, and three studies of SpO2. The studies are described in the next paragraphs and briefly summarized in Tables 2–4.
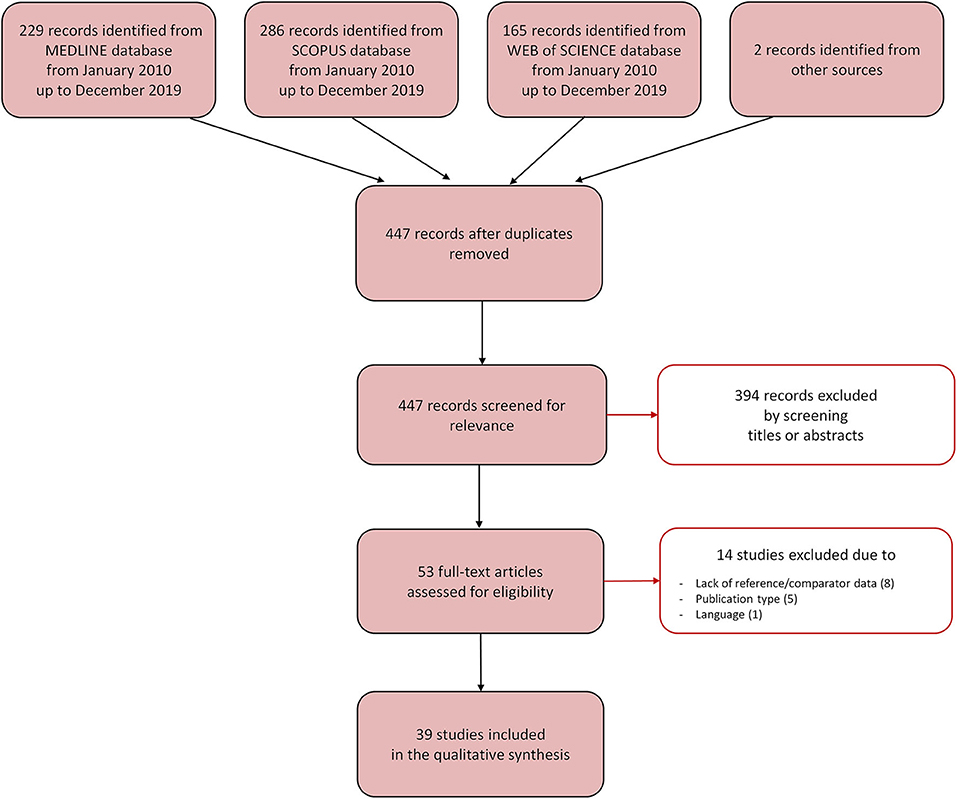
Figure 3. Selection process for the studies included in the scoping review. The Preferred Reporting Items for Systemic Reviews and Meta-Analyses [PRISMA-sc (Tricco et al., 2018)] flow diagram depicts the number of records identified, included and excluded, and the reasons for exclusion, through the different phases of the scoping review.
Measurement of Temperature
Introduction
The monitoring of T is an essential component of physiological research and sport science, and a fundamental diagnostic parameter in emergency and occupational medicine for guiding treatment and triage decisions (Pasquier et al., 2019; Strapazzon et al., 2019). In sport science, the measurement of core T is critical when evaluating research questions related to heat production, such as heat acclimatization and body cooling, exercise intensity, and effect of different environmental conditions on physiological state and performance. Monitoring of core T can be used to guarantee intensity and safety during intense heat exercise and heat tolerance tests, and to prevent exertional heat stroke (American College of Sports Medicine et al., 2007) or unexpected cooling (Procter et al., 2018). Indeed, a decrease in core T per se may significantly impair physiological functions even in a healthy subject and should be timely detected for proper triage (Danzl and Pozos, 1994; Paal et al., 2018). On the other hand, core T is considered a pivotal parameter also in occupational medicine, for instance to identify subjects at risk of heat stress disorders (Nagano et al., 2010).
Although invasive measurements, such as those performed in the pulmonary artery or lower third of the esophagus, remain the gold standard for assessment of core T, these sites are not practical in many situations, including the described settings (Strapazzon et al., 2014). The ear canal was proposed as a promising alternative site to measure core T, thanks to the functional relation between the TM and the hypothalamic central thermoreceptors via shared blood supply (McCarthy and Heusch, 2006), the non-invasivity, the respect of basic hygiene standards, the relative independence from external environmental conditions, the wearability, and the fast monitoring response (Gunga et al., 2009; Strapazzon et al., 2014).
Results and Discussion
Twenty-four studies, summarized in Table 2, analyzed the suitability of the ear canal as measuring site for core T under different physiological and environmental conditions. The works differed in terms of sensors (IR or thermistor-based devices) and device technology (market or prototype devices), the core T reference/comparator (e.g., esophageal, rectal, or gastrointestinal), as well as for the protocols and scenarios within which the measurements were performed. As well, differences in populations (i.e., healthy volunteers or athletes) and demographic variables among studies were present, which may have introduced further variability in T measurements (Heusch et al., 2006; McCarthy and Heusch, 2006).
Most of the studies (sixteen) performed in-ear T monitoring by IR thermometers. IR measurements were shown to display high correlation with oral and forehead measurements in resting individuals (p < 0.01) (Basak et al., 2013). More variable results were obtained in the studies analyzing the effect of exercise in different environmental conditions (Gagnon et al., 2010; Bagley et al., 2011; Fogt et al., 2017; Harmanci et al., 2018; Morán-Navarro et al., 2019), where microclimate changes in the ear canal and changes in blood flow to the skin are known to impact measurements (Patel et al., 1996; Jensen et al., 2000; Casa et al., 2005; Kistemaker et al., 2006). Of note, tympanic measurements of T were not always compared to the “gold standard” site for core T (esophageal) (Strapazzon et al., 2014). Tympanic probes were shown to display different performance—with respect to other T monitoring sites—when compared to ingestible sensors during rest, exercise, and recovery (also under wind conditions) with a bias ranging from −0.7 to 1.4°C (p < 0.001) (Morán-Navarro et al., 2019). Tympanic devices reflected core T measured by gastrointestinal pills in 14 healthy subjects exercising in a hot, humid environment, although different IR tympanic thermometers displayed diverse response (limits of agreement varying from ± 1.90 to ± 2.15°C), highlighting the need for appropriate selection and validation of single devices (Fogt et al., 2017). Harmanci et al. demonstrated that tympanic T tracked lower values than ingestible sensors during the progress of exercise (p < 0.05) (Harmanci et al., 2018). Bagley et al. observed that tympanic T was significantly lower than rectal T during both rest and exercise under cold experimental conditions (bias = −1.4°C, p < 0.0005) (Bagley et al., 2011). Gagnon and co-workers found that tympanic T increased at a rate similar to rectal T, but was consistently lower than esophageal T (p < 0.01) in healthy subjects exercising in a hot temperature-controlled chamber (Gagnon et al., 2010). When immersing subjects in cold water or during recovery, tympanic T remained significantly lower than rectal T, suggesting an effect of physiologic differences in regional blood flow during and after exertional heat stress and of the buffering influence of rectal dense tissue mass around the probe (Gagnon et al., 2010). Overall, these results should be interpreted with caution since rectal and gastrointestinal sites have different behaviors and known limitations in dynamic conditions that may limit their validity as T reference/comparator with respect to the esophageal site (Lee et al., 2000; Lim et al., 2008; Wilkinson et al., 2008; Strapazzon et al., 2014).
Effects on tympanic T measurements of local temperature changes in areas proximal or distant from the ear were further investigated in four studies using either IR (Kallmünzer et al., 2011) and thermistor-based thermometers (Flouris and Cheung, 2010a,b; Suzuki et al., 2010; Basset et al., 2011). Local cooling of the head and neck was shown to modify tympanic T measurement to a larger extent than rectal T (−1.7 and −0.65°C from baseline, respectively, p < 0.05) (Kallmünzer et al., 2011). On the other hand, Basset et al. showed that tympanic and esophageal T were almost unaffected (−0.3 ± 0.3°C and −0.1 ± 0.3°C from baseline, respectively) by cooling of the lower body surface (Basset et al., 2011), while rectal T underwent a significant drop (−1.0 ± 0.4°C from baseline, p < 0.05). These results were further expanded in two studies by Flouris and Cheung, who investigated tympanic T changes in healthy subjects performing exercise or entering hot and cold water tanks (Flouris and Cheung, 2010a,b). Although delayed with respect to rectal, skin, and exhaled breath T, and different in absolute values (p < 0.05), tympanic T measurements displayed a significant agreement and correlation with other monitoring sites (coefficient of determination r2 ranging from 0.20 to 0.96 with rectal T).
Five studies analyzed the effects of cold environments on tympanic T measurements, in simulated conditions (Teunissen et al., 2011; Skaiaa et al., 2015; Strapazzon et al., 2015) and sport/competition settings (Bhangu and Parmar, 2010; Muth et al., 2010), to investigate the device suitability for hypothermia prevention. Tympanic T, measured by a commercial tympanic thermistor sensor under different environmental conditions (i.e., ambient air with or without local/wind insulation; snow or icy water in ear canal) displayed significantly (p < 0.006) lower values than rectal T (bias ranging from −1.5/−3.2°C at 5 min to −1.2/−2.0°C at 10 min) (Skaiaa et al., 2015). Similar results were obtained by Strapazzon et al. comparing a tympanic probe to an esophageal one. However, ear insulation was able to reduce bias by 52% (from 2.9 to 1.5°C) in the ambient setting and by 82% (7.2–1.3°C) in the low T setting (Strapazzon et al., 2015). An inter-individual variability was observed in the degree of deviation of tympanic from esophageal T (Strapazzon et al., 2015), which was consistent with variability in physiological factors (vascularization, conductivity, and tissue perfusion) and anatomical differences (length, width, shape) affecting probe placement. The impact of cold environmental conditions on tympanic T and the mitigating effect of insulating the ear thermistor from environmental changes were confirmed during a rest, exercise, and recovery protocol (Teunissen et al., 2011). Of note, wind had an immediate cooling effect on tympanic T, which was not sufficiently counteracted by insulation (bias = −1.73 ± 0.11°C and −2.49 ± 0.04°C in covered and uncovered ear condition, respectively) (Teunissen et al., 2011). The negative impact of extreme environmental conditions on tympanic measurements was observed also for IR probes in cold environments, such as swimming in cold water (Muth et al., 2010) and outdoor endurance events (Bhangu and Parmar, 2010). In relation to swimming, it was suggested that the water in the ear canal could significantly influence T measurements, either by creating a cool “buffer zone” between the probe and the TM and/or by cooling down the ear canal wall and the TM (Muth et al., 2010).
Given the limitations of IR thermometers, three technical studies proposed technological improvements to address the effect of anatomical and environmental factors on T measurements (Yamakoshi et al., 2010; Ota et al., 2017; Chaglla et al., 2018). To reduce the impact of individual ear morphology and environmental conditions, Chaglla et al. (2018) proposed a graphene-inked sensor, obtained from a commercial IR thermopile and fixed by a 3D-printed hook-type enclosure. The sensing device was developed to continuously measure T from the TM and to display it on a smartphone. It included a microcontroller processing unit and a Bluetooth module for wireless connectivity to a smartphone application and was powered with a rechargeable lithium-polymer battery (3.7 V) providing continuous operation for at least 4 h. The sensor reduced the influence of ambient T (mean bias = −0.36°C), with respect to a reference commercial sensor (mean bias = −0.51°C). Similar to these results, Ota et al. showed that a wearable IR sensor, combined with a hearing aid and inserted in a 3D-printed device, could provide a more reliable T estimation than a skin sensor (Ota et al., 2017). Together with the sensor module the device included a Bluetooth transceiver (powered by a 3.7 V lithium battery) for wireless data transmission to a mobile application interface displaying T values in real time. This approach was further corroborated by results from Yamakoshi et al., who reported good correlation of T values measured by an in-ear customized molded IR sensor with values measured by a thermistor-based tympanic sensor (r2 = 0.97, p < 0.01, bias = −0.01°C) and by gastrointestinal pills (r2 = 0.86, p < 0.001, bias = −0.27°C) in subjects under hyperthermic conditions. The earpiece device, connected to a full-face helmet, sensor amplifier, and signal processor, was also preliminarily tested in-field on professional drivers during real racing conditions and displayed good technical functioning, although no comparison with core T were performed in this setting (Yamakoshi et al., 2013).
Five studies analyzed the potential of in-ear T measurements for occupational medicine for prevention of heat illness (Nagano et al., 2010; Lee et al., 2011; Pryor et al., 2012; Keene et al., 2015; Nakada et al., 2017). The studies reported controversial results. Nagano et al. showed that tympanic T, measured by a thermocouple probe with tight sealing in the ear canal, closely tracked both esophageal and rectal T with slight underestimation during simulated rest-work cycles at increasing environmental T (Nagano et al., 2010). IR tympanic probes underestimated core T obtained by gastrointestinal pills in firefighters performing work in heat chambers (bias ranging from 1.3 ± 0.5°C to 1.0 ± 0.8°C when entering the chamber and following exercise) (Keene et al., 2015) and during exercise with thermal protective clothing in a warm room (Pryor et al., 2012). By using a telemetry system consisting of an ear probe with IR sensor technology, a transmitter, and a wireless data receiver, Lee and co-workers showed an overall high level of agreement between tympanic and rectal T, both at rest and during exercise conditions, in healthy volunteers, although bias varied with clothing levels. Tympanic T was lower than rectal T in control conditions and when wearing a high-density polyethylene coverall, while it reached and exceeded rectal T for a polyvinyl chloride coverall at environmental T of 25 and 32°C (Lee et al., 2011). Nevertheless, given the slow response of rectal measurements, comparisons of tympanic and rectal T away from steady-state conditions should be interpreted with caution (Lee et al., 2000; Lim et al., 2008; Strapazzon et al., 2014). Interestingly, by using a customized IR device, equipped with a silicon mold to fit into the ear and capable of measuring T at three different locations from the TM, Nakada et al. demonstrated that inner and outer T measurements along the ear canal could accurately estimate esophageal T under different physiological and environmental conditions simulating elevated ambient T (Nakada et al., 2017).
Measurement of Heart/Pulse Rate
Introduction
The measure of HR/PR, eventually complemented by heart rate variability parameters, represents an inexpensive, non-invasive, and time-efficient way to monitor cardiovascular performance and autonomic nervous system status (Buchheit, 2014). In sport science, measures of resting, exercise, and recovery HR are used as surrogate markers of fatigue, fitness, and endurance performance status, with implications for optimization of training loads and enhancement of performance (Buchheit, 2014; Schneider et al., 2018). Thanks to the wide diffusion of PPG technology in wearable sensors, PR has become a readily available parameter and real-world PR values, acquired during daily-life activities, are gaining increasing importance (Poh and Kittler, 2012; Bunn et al., 2019). However, acquisition of PPG signals and accuracy of PR values during physical activity and exercise modes in indoor or outdoor settings may be compromised by motion artifacts, noise, and reduced signal quality (Poh et al., 2010; Tomita, 2016). The ear canal bears potential for HR/PR monitoring during exercise, since the head is less affected by motion artifacts, orthostatic pressure modulations, and low perfusion problems than other extremities.
Results and Discussion
Twelve studies reporting data on earbud devices for HR/PR monitoring are summarized in Table 3. The majority (eight) of the studies used PPG-based sensors for monitoring PR, one study used electrodes and a microphone embedded in a earbud for measuring an ear-ECG (von Rosenberg et al., 2017), and three studies proposed alternative techniques, such as IR thermography (de Graaf et al., 2019), mechanical photoplethysmography (Goverdovsky et al., 2017), and pressure measurement by piezoelectric sensors (Park et al., 2015). Although non-optical methods may have the advantage of no light source and reduced power consumption, the performed studies suggested the feasibility of HR/PR measurements only under basic resting conditions in small groups of subjects. Sensitivity to user's motion may impair performance under exercise conditions, requiring further optimization of the electronics and sensor placements within the earpiece, and noise reduction in the readout circuitry. On the other hand, methods allowing to acquire an ECG, instead of a pulsatile signal, suggested the possibility to trace cardiac activity beyond HR estimation. After demonstrating in a simulation model the possibility to trace ECG from ear or head locations, von Rosenberg et al. showed in resting subjects that cardiac cycles extracted from ear-ECG correlated well with those extracted from Lead 1 ECG. Although better performance was obtained using the Lead 1 ECG to guide R-wave identification (r2 = 0.92), good performance could be achieved also for the stand-alone device, where the microphone signal was used to guide wave identification (r2 = 0.81) (von Rosenberg et al., 2017).
A more extensive validation of in-ear devices, including tests during exercise sessions at different intensity levels, was performed in the studies using PPG-based sensors. Four studies evaluated the performance of commercial in-ear devices (Boudreaux et al., 2018; Higgins et al., 2018; Bunn et al., 2019; Passler et al., 2019). Bunn et al. (2019) compared the performance of a commercial earbud system and a wrist-watch for PR monitoring with that of a chest strap used as benchmark. The earbud device, transmitting information via Bluetooth to a smartphone, demonstrated consistent performance throughout three exercise protocols (bias ranging from 0.8 to −3.6 bpm during treadmill/outdoor sessions and high-intensity session, respectively), with slightly reduced agreement with the benchmark during high-intensity exercise where it slightly underestimated PR. The earbud displayed higher performance than the wrist-watch in all exercise sessions but especially in the high-intensity session, where the wrist-watch exhibited poor performance due to substantial arm motion. High agreement between a commercial wireless exercise earpiece (connected via Bluetooth with a smartphone) and a reference chest strap (r2 = 0.94) was reported by Higgins et al. during maximal graded exercise on a treadmill (Higgins et al., 2018). However, the authors pointed out occasional displacement problems of both devices when exercise intensity increased. Passler et al. compared the performance of two commercial in-ear PR trackers with a reference ECG at rest and during subject-specific stress tests on a cyclo-ergometer (Passler et al., 2019). Both devices displayed accurate PR measurements in either condition (mean absolute percentage errors ranging from 2.5 to 3.2% for PR <90 bpm and from 1.3 to 1.4 for PR >100 bpm), despite a tendency to PR underestimation and exposure to motion artifacts. An extended comparison of eight commercial PR monitoring systems, including an in-ear device which transmitted real-time PR data via Bluetooth to a smartphone application, was performed by Boudreaux et al. during trials of graded cycling and standard resistance exercise at maximal load (Boudreaux et al., 2018). The devices displayed different performance under different exercise conditions. Only the in-ear device and a chest-worn device displayed limited bias with respect to ECG data in both cycling and resistance exercise sessions, the in-ear device displaying the best performance during the resistance exercise (mean absolute percentage error of 6.24%). In all devices a greater underestimation of HR was observed as exercise intensity increased.
Two additional studies implemented and tested prototype devices for PR monitoring (Poh and Kittler, 2012; Leboeuf et al., 2014). Poh and Kittler (2012) developed the “Heartphone system,” embedding a reflective IR photosensor in a fully-integrated unobtrusive wireless headset, powered by a rechargeable lithium polymer battery. The system architecture included a processing control unit with active filters to reduce electrical noise and motion artifacts, an analog-to-digital converter, a PPG peak detection algorithm, and a communication system (either serial and wireless) to send PR values to a custom application on mobile devices or laptops. The device showed robust PR measurements under conditions of moderate motion, with high agreement with ECG-derived HR measurements when users were standing, cycling, or walking (bias ranging from −0.07 to 0.67 bpm), but lower performance was expected under vigorous exercise. Leboeuf et al. (2014) tested an earbud PPG sensor including an accelerometer. The earbud was designed to be pluggable via a detachable connector to a wireless “medallion,” which housed a microprocessor and a Bluetooth chipset, and granted several hours of measurement time. The device, tested during a treadmill exercise protocol including rest to peak performance steps, showed accurate PR monitoring throughout all activity levels with high correlation (r2 = 0.98) and low bias (−0.2 ± 4.4%) when compared with the benchmark ECG device. High performance in PR estimation also during extreme physical activity was obtained thanks to the capability of characterizing motion noise through the accelerometer and of attenuating motion artifacts from the optical signal in real-time. To provide a thorough characterization of the physiological state during exercise, the authors combined PR and contextual accelerometry values in a statistical model to estimate total energy expenditure and aerobic capacity. Total energy expenditure could be reliably estimated, while lower accuracy was observed for aerobic capacity.
Technical aspects related to PPG signal filtering and PR estimation from in-ear devices were addressed in two additional studies (Vogel et al., 2009; Tomita et al., 2018). A filtering approach, based on dual PPG signal acquisition in both ears and asynchronous noise removal, was proposed by Tomita et al. and tested under various movement and exercise conditions (Tomita et al., 2018). The approach demonstrated higher performance (bias within ±5 bpm in 95% of measurements) than accelerometer-based filtering, at the cost of doubling the number of sensors and signals to be acquired. Vogel et al. compared the performance of time-domain and frequency-domain algorithms in estimating PR from PPG signals acquired by an in-ear MORES sensor (Vogel et al., 2009), connected with a small body box containing the signal acquisition and communication circuitry and granting hours of monitoring. The authors showed better performance of the frequency-domain method when working on either IR and red-light PPG signals, with the highest performance obtained using IR signals (bias of −0.59 ± 1.33 bpm with respect to a finger sensor).
Measurement of Oxygen Saturation
Introduction
SpO2 is the physiological parameter which tracks the amount of oxygen dissolved in arterial blood or transported oxygen bound to hemoglobin, as indirectly measured at suitable peripheral sites (i.e., finger, toe, forehead, and ear) by pulse oximetry. Since the brain, as well as the muscles, requires a constant oxygen supply to support the high metabolic rate production necessary to remain electrically active (Williams et al., 2019), SpO2 monitoring is of relevance to detect possible risk conditions not only in clinical settings, but also in physiological studies, sport science, and occupational medicine (Costello et al., 2020; Pham et al., 2020; Stensrud et al., 2020). A reduction in oxygen availability, as experienced at high altitudes or in particular challenging situations, can have a detrimental effect on brain and muscles function, inducing performance decline and increasing the risk of errors and injuries (Hoiland et al., 2016). The parameter is also crucial to evaluate adaptation processes and performance when exercising or training at altitude or in hyperbaric conditions (Chapman, 2013). In high-risk environments for workers (firefighters or military personnel) and athletes, SpO2 information may help to prevent injuries, such as exertional heat illness (Pham et al., 2020). Advances in medical instrumentation and considerable improvements in optoelectronics have made pulse oximetry integrable in wearable devices (Foo et al., 2013), opening the possibility of long-term oxygen monitoring in the mentioned settings. On the other hand, in the presence of local or systemic vasoconstriction during sport activities or cold environmental conditions, poor peripheral perfusion at the wrist, finger, and toe, may make these sites inappropriate to accurately estimate SpO2 (Budidha and Kyriacou, 2018). The ear canal may represent a more reliable site for the assessment of SpO2, since it is less subject to vasoconstriction and motion artifacts and it guarantees suitable blood flux and PPG signal quality (Budidha and Kyriacou, 2014).
Results and Discussion
The three studies, which analyzed pulse oximetry measurements from the ear canal, are summarized in Table 4. All the studies analyzed prototype devices (Venema et al., 2012; Budidha and Kyriacou, 2014, 2018) that applied dual wavelength emission in reflection configuration. Performance was tested under various conditions. In two studies measurements were performed under challenging conditions inducing vasoconstriction, such as cold immersion test (Budidha and Kyriacou, 2014) and cold-exposure (Budidha and Kyriacou, 2018), while in the third study the device was calibrated under hypoxic conditions (Venema et al., 2012). The two studies performing vasoconstrictive maneuvers consistently showed that SpO2 measurements performed in the ear canal were minimally affected by vasoconstriction, while reference measurements performed on the fingertip were significantly impaired by the reduced blood flow. In particular, the amplitude of both red and IR PPG waveforms underwent just minor changes (+2.5 and −1.2%, respectively) after ice water immersion of the right hand with respect to baseline recordings, while the amplitude of both right and left index finger PPG signals resulted almost halved (Budidha and Kyriacou, 2014). Similar, in subjects undergoing artificially-induced hypothermia by means of cold exposure at 10°C, the normalized pulse amplitude of red and IR light PPGs presented a maximal decrease of 13% for the ear sensor with respect to a drop of >80% for the finger sensor. The decrease in signal amplitude in the finger sensor resulted in measurement failure (i.e., SpO2 < 90%) in one third of the subjects, while failure in ear measurement was obtained only in one subject out of 15 (Budidha and Kyriacou, 2018). These results supported the reliability of PPG signal quality from the ear canal during vasoconstriction, but they did not provide information on the actual accuracy of the estimated SpO2 values with respect to gold standard values. A single study examined this aspect and evaluated the performance of ear-in pulse oximeters in comparison with blood gas analysis under realistic clinical conditions with various levels of hypoxia (Venema et al., 2012). Specifically, SpO2 was measured by a PPG sensor embedded in an individually-customized ear mold for measurement in the ear canal, in healthy subjects wearing a breathing mask that regulated the blood oxygen level of the inspired air. During the tests blood was saturated in steps from 100% to 70–77% and blood samples from the radial artery were taken at each step to perform reference blood gas analysis. The study showed good correlation (r2 = 0.96) between absorbance rates and corresponding reference SpO2 values at the individual level (Venema et al., 2012). However, the global calibration curve obtained from all measurements showed unacceptable accuracy, due to the presence of offsets between individual calibration curves. Intra-individual variability among calibration curves enabled the estimation of SpO2 only in relative terms. Absolute reliable in-ear oxygen saturation measurements could be obtained in the study using an individual single-point calibration (i.e., performing an initial SpO2 measurement with a reference device). Alternative compensation strategies may rely on the use of a third wavelength to compensate for the offset without the necessity of single-point calibration (Venema et al., 2012).
Limitations and Future Developments
The large set of research studies presented in this review proves the wide interest for hearables as suitable instruments for physiological parameters monitoring, potentially able to satisfy commercial and research-oriented markets. The data presented in this review suggest the capability of the devices to provide accurate measurements in multiple conditions, but point out the necessity of further improvements to guarantee reliability of measurements in more challenging scenarios, such as during intense exercise, under extreme climatic conditions, and/or dynamic conditions. These aspects are of primary importance to grant applicability in sport science and physiological research, as well as in emergency and occupational care. In general, a certain degree of variability in accuracy and performance was observed among studies. Although devices were often constructed based on similar principles, proprietary differences in technology and algorithms for signal processing may partially explain these differences. More importantly, heterogeneity in protocols (e.g., differences in the type, intensity, and duration of activities performed during the validation) and settings (e.g., simulation of extreme environments), as well as in the type of reference measures, were present among studies which may further hinder comparisons and a pooled evaluation of accuracy. Moreover, mismatch between requested standards and in-field accuracy may further explain differences among studies, which fosters further debate regarding the accuracy, validity, and reliability of in-ear measurements methods (Ring et al., 2010; Sund-Levander and Grodzinsky, 2013). Increasing the accuracy of measurements may rely on the improvement of signal/data acquisition and device stability, potentially through the implementation of new materials, as exemplified by graphene-coated T sensors (Chaglla et al., 2018). Probe development for T measurement should pursue personalization to adapt the device to the specific anatomy of each user (Muir et al., 2001; Venema et al., 2012; Ota et al., 2017), allowing a better insulation from external conditions (Teunissen et al., 2011; Strapazzon et al., 2015). For PPG-based measurements, accuracy may be improved by optimizing sensor design and wavelength [e.g., optimal LED-photodiode distance, shunt-light reduction (Buschmann and Huang, 2010)], but also by developing suitable signal processing and advanced algorithms for noise removal (Poh et al., 2010; Poh and Kittler, 2012; Tomita et al., 2018). Motion artifact removal and noise cancellation techniques are a central area of interest for the improvement of PPG-based monitoring devices for either PR and SpO2 measurement, and adaptive noise cancellation using integrated accelerometers as reference is considered a promising technique (Poh et al., 2010). Calibration issues and model optimization on larger populations under different conditions are relevant for obtaining reliable SpO2 measurements (Venema et al., 2012), as well as energy and metabolic parameters from PR values and accelerometric information (Leboeuf et al., 2014; Boudreaux et al., 2018). The use of advanced post-processing algorithms, allowing the extraction of respiratory traces and HR variability parameters from in-ear signals, may further extend the set of monitored physiological parameters and should be analyzed in future studies (Poh and Kittler, 2012). In addition, the possibility of measuring an ear-ECG instead of simply measuring a pulsatile signal may enable the identification and examination of heart conditions, such myocardial infarction or arrhythmias, which may be helpful for emergency medicine, sport medicine, and occupational medicine settings (von Rosenberg et al., 2017).
Further research is also needed to address limitations evidenced by the present review. The literature on in-ear SpO2 measurements is sparse and focused on signal quality issues. Calibration needs to be further addressed and algorithms need optimization on large study groups in comparison with gold-standard references (Venema et al., 2012). The growing demands for remote personal health monitoring for healthy geriatrics in residential homes (Foo et al., 2013), further stimulated by the recent COVID-19 pandemic, has promoted further research on in-ear SpO2 measurement for long-term monitoring. Recent data about a full in-ear SpO2 monitor, tested in resting subjects during a sequence of normal breathing and breath-holds (Davies et al., 2020), showed good agreement between in-ear and finger measurements, with a root mean square error of 1.47% and just slight overestimation of SpO2 by the ear device. Of note, the device showed a faster response to track saturation changes induced by the breath hold protocol than the finger sensor (Davies et al., 2020). As concerns T, tympanic measurements have been shown to track body T and potentially brain T, but this assumption should be considered with caution. Despite the shared vasculature with carotid artery, branches of the external carotid artery could introduce variability associated to the size, origin, and therefore relative flow through each component artery (McCarthy and Heusch, 2006). These aspects—together with biasing factors related to environmental conditions, operators training, in-ear local phenomena, and adequate maintenance of the probes—may represent further sources of variability (Bridges and Thomas, 2009; Ring et al., 2010; Sund-Levander and Grodzinsky, 2013) with impact on T measurements in different physiological and pathophysiological conditions. Thus, the actual reliability and range of validity of in-ear physiological parameters need further tests, especially in challenging environments and in real patients. As well, technical challenges in terms of power, cost, size, weight, functionality, and packaging needs further efforts in view of long-term applications and more challenging settings. Such efforts will be potentially rewarded, since in-ear technology is integrable with smart devices, and thus it may be packaged and sold with smartphones as audio earbuds in large volumes of units per year (Leboeuf et al., 2014). Thanks to their unobstrusivity and comfort, hearables may reach a larger consumer audience, with potential benefit in the promotion of healthy lifestyles and preventive healthcare, and they may offer a novel non-invasive site for monitoring workers and patients in out-of-hospital settings.
Conclusive Remarks
The accumulating evidence presented in this review supports the promise of in-ear sensors as an innovative and unobtrusive way to monitor vitals in daily-life and during physical activity, although applications in more challenging environmental scenarios and intense exercise settings require further improvements in terms of accuracy. Further research addressing physiological and technical aspects is strongly encouraged to better understand the peculiar anatomical and vascular features of this unique body site in order to ameliorate measurement accuracy and device response in different contexts.
Data Availability Statement
All relevant data is contained within the article.
Author Contributions
MM and AM designing the study, performing the literature search, and drafting the manuscript. GS designed the study and critically revised the manuscript for important intellectual content. All authors contributed to manuscript revision, read, and approved the submitted version.
Funding
The research leading to these results has received funding from the FESR Program 2014–2020 of the Autonomous Province of Bolzano – Alto Adige, under Grant Agreement [513/2019]/Project number [FESR 1114], [Development of innovative sensors for monitoring vital parameters in emergency medicine, MedSENS].
Conflict of Interest
The authors declare that the research was conducted in the absence of any commercial or financial relationships that could be construed as a potential conflict of interest.
Acknowledgments
The authors thank the Department of Innovation, Research and University of the Autonomous Province of Bozen/Bolzano for covering the Open Access publication costs.
References
American College of Sports Medicine, Armstrong, L. E., Casa, D. J., Millard-Stafford, M., Moran, D. S., Pyne, S. W., et al. (2007). American College of sports medicine position stand. Exertional heat illness during training and competition. Med. Sci. Sports Exerc. 39, 556–572. doi: 10.1249/MSS.0b013e31802fa199
Bagley, J. R., Judelson, D. A., Spiering, B. A., Beam, W. C., Bartolini, J. A., Washburn, B. V., et al. (2011). Validity of field expedient devices to assess core temperature during exercise in the cold. Aviat. Space Environ. Med. 82, 1098–1103. doi: 10.3357/ASEM.3102.2011
Basak, T., Aciksoz, S., Tosun, B., Akyuz, A., and Acikel, C. (2013). Comparison of three different thermometers in evaluating the body temperature of healthy young adult individuals. Int. J. Nurs. Pract. 19, 471–478. doi: 10.1111/ijn.12097
Basset, F., Cahill, F., Handrigan, G., DuCharme, M., Cheung, S., Basset, F. A., et al. (2011). The effect of lower body cooling on the changes in three core temperature indices. Physiol. Meas. 32, 385–394. doi: 10.1088/0967-3334/32/4/001
Bent, B., Goldstein, B. A., Kibbe, W. A., and Dunn, J. P. (2020). Investigating sources of inaccuracy in wearable optical heart rate sensors. NPJ Digital Med. 3, 1–9. doi: 10.1038/s41746-020-0226-6
Benzinger, T. H., and Taylor, G. W. (1963). “Cranial measurements of internal temperature in man,” in Temperature - Its Measurement and Control in Science and Industry (New York, NY: Reinhold), 111–120.
Berkovitz, B. K. B. (2005). “External and middle ear.” in Gray's Anatomy. The Anatomical Basis of Clinical Practice (Edinburgh: Elsevier Churchill Livingstone), 649–661.
Bhangu, A., and Parmar, R. (2010). Detection and management of hypothermia at a large outdoor endurance event in the United kingdom. Wilderness Environ. Med. 21, 141–145. doi: 10.1016/j.wem.2009.12.003
Boudreaux, B., Hebert, E., Hollander, D., Williams, B., Cormier, C., Naquin, M., et al. (2018). Validity of wearable activity monitors during cycling and resistance exercise. Med. Sci. Sports Exerc. 50, 624–633. doi: 10.1249/MSS.0000000000001471
Bridges, E., and Thomas, K. (2009). Noninvasive measurement of body temperature in critically ill patients. Crit. Care Nurse 29, 94–97. doi: 10.4037/ccn2009132
Buchheit, M. (2014). Monitoring training status with HR measures: do all roads lead to Rome? Front. Physiol. 5:73. doi: 10.3389/fphys.2014.00073
Budidha, K., and Kyriacou, P. A. (2014). The human ear canal: investigation of its suitability for monitoring photoplethysmographs and arterial oxygen saturation. Physiol. Meas. 35, 111–128. doi: 10.1088/0967-3334/35/2/111
Budidha, K., and Kyriacou, P. A. (2015). Investigation of photoplethysmography and arterial blood oxygen saturation from the ear-canal and the finger under conditions of artificially induced hypothermia. Conf. Proc. IEEE Eng. Med. Biol. Soc. 2015, 7954–7957. doi: 10.1109/EMBC.2015.7320237
Budidha, K., and Kyriacou, P. A. (2018). In vivo investigation of ear canal pulse oximetry during hypothermia. J. Clin. Monit. Comput. 32, 97–107. doi: 10.1007/s10877-017-9975-4
Bunn, J., Wells, E., Manor, J., and Webster, M. (2019). Evaluation of earbud and wristwatch heart rate monitors during aerobic and resistance training. Int. J. Exerc. Sci. 12, 374–384.
Buschmann, J. P., and Huang, J. (2010). New ear sensor for mobile, continuous and long term pulse oximetry. Conf. Proc. IEEE Eng. Med. Biol. Soc. 2010, 5780–5783. doi: 10.1109/IEMBS.2010.5627835
Casa, D. J., Armstrong, L. E., Ganio, M. S., and Yeargin, S. W. (2005). Exertional heat stroke in competitive athletes. Curr. Sports Med. Rep. 4, 309–317. doi: 10.1097/01.CSMR.0000306292.64954.da
Chaglla, E., J., S., Celik, N., and Balachandran, W. (2018). Measurement of core body temperature using graphene-inked infrared thermopile sensor. Sensors 18:3315. doi: 10.3390/s18103315
Chapman, R. F. (2013). The individual response to training and competition at altitude. Br. J. Sports Med. 47(Suppl. 1), i40–44. doi: 10.1136/bjsports-2013-092837
Chen, W. (2019). Thermometry and interpretation of body temperature. Biomed. Eng. Lett. 9, 3–17. doi: 10.1007/s13534-019-00102-2
Clayton, D. G., Webb, R. K., Ralston, A. C., Duthie, D., and Runciman, W. B. (1991). Pulse oximeter probes. A comparison between finger, nose, ear and forehead probes under conditions of poor perfusion. Anaesthesia 46, 260–265. doi: 10.1111/j.1365-2044.1991.tb11492.x
Costello, J. T., Bhogal, A. S., Williams, T. B., Bekoe, R., Sabir, A., Tipton, M. J., et al. (2020). Effects of normobaric hypoxia on oxygen saturation variability. High Alt. Med. Biol. 21, 76–83. doi: 10.1089/ham.2019.0092
Danzl, D. F., and Pozos, R. S. (1994). Accidental hypothermia. N. Engl. J. Med. 331, 1756–1760. doi: 10.1056/NEJM199412293312607
Davies, H. J., Williams, I., Peters, N. S., and Mandic, D. P. (2020). In-Ear Measurement of Blood Oxygen Saturation: An Ambulatory Tool Needed To Detect The Delayed Life-Threatening Hypoxaemia in COVID-19. arXiv:2006.04231 [eess]. Available online at: http://arxiv.org/abs/2006.04231 (accessed July 28, 2020).
de Graaf, G., Kuratomi Cruz, D., Haartsen, J. C., Hooijschuur, F., and French, P. J. (2019). Heart rate extraction in a headphone using infrared thermometry. IEEE Trans. Biomed. Circuits Syst. 13, 1052–1062. doi: 10.1109/TBCAS.2019.2930312
Flouris, A. D., and Cheung, S. S. (2010a). Thermometry and calorimetry assessment of sweat response during exercise in the heat. Eur. J. Appl. Physiol. 108, 905–911. doi: 10.1007/s00421-009-1302-4
Flouris, A. D., and Cheung, S. S. (2010b). The validity of tympanic and exhaled breath temperatures for core temperature measurement. Physiol. Meas. 31, N35–N42. doi: 10.1088/0967-3334/31/5/N01
Fogt, D. L., Henning, A. L., Venable, A. S., and McFarlin, B. K. (2017). Non-invasive measures of core temperature versus ingestible thermistor during exercise in the heat. Int. J. Exerc. Sci. 10, 225–233.
Foo, J. Y. A., Chua, K. P., and Tan, X. J. A. (2013). Clinical applications and issues of oxygen saturation level measurements obtained from peripheral sites. J. Med. Eng. Technol. 37, 388–395. doi: 10.3109/03091902.2013.816380
Gaasch, M., Putzer, G., Schiefecker, A. J., Martini, J., Strapazzon, G., Ianosi, B., et al. (2020). Cerebral autoregulation is impaired during deep Hypothermia-A porcine multimodal neuromonitoring study. Ther. Hypothermia Temp. Manag. 10, 122–127. doi: 10.1089/ther.2019.0009
Gagnon, D., Lemire, B. B., Jay, O., and Kenny, G. P. (2010). Aural canal, esophageal, and rectal temperatures during exertional heat stress and the subsequent recovery period. J. Athl. Train 45, 157–163. doi: 10.4085/1062-6050-45.2.157
García Callejo, F. J., Platero Zamarreño, A., Sebastián Gil, E., Marco Sanz, M., Alpera Lacruz, R. J., and Martínez Beneyto, M. P. (2004). Otologic determining factors on infra-red tympanic thermometry in children. Acta Otorrinolaringol. Esp. 55, 107–113. doi: 10.1016/S0001-6519(04)78492-7
Goverdovsky, V., von Rosenberg, W., Nakamura, T., Looney, D., Sharp, D. J., Papavassiliou, C., et al. (2017). Hearables: multimodal physiological in-ear sensing. Sci. Rep. 7:6948. doi: 10.1038/s41598-017-06925-2
Gunga, H.-C., Werner, A., Stahn, A., Steinach, M., Schlabs, T., Koralewski, E., et al. (2009). The double sensor-a non-invasive device to continuously monitor core temperature in humans on earth and in space. Respir. Physiol. Neurobiol. 169(Suppl. 1), S63–S68. doi: 10.1016/j.resp.2009.04.005
Harmanci, H., Karavelioglu, M., Kayhan, M., Gunduz, B., Harmanci, H., Karavelioglu, M. B., et al. (2018). Comparison of two methods for determination of core temperature during running velocity at maximal lactate steady state. Med. Dello Sport. 71, 164–173. doi: 10.23736/S0025-7826.18.02824-7
Heusch, A. I., Suresh, V., and McCarthy, P. W. (2006). The effect of factors such as handedness, sex and age on body temperature measured by an infrared “tympanic” thermometer. J. Med. Eng. Technol. 30, 235–241. doi: 10.1080/03091900600711324
Higgins, T., Lloyd, T., Manalansan, K., McDonald, S., Thomas, B., Higgins, T. R., et al. (2018). Comparisons in heart rate readings between the bioconnected wireless exercise earpiece and a Polar T31-coded chest strap during a GXT. J. Hum. Sport Exerc. 13, 530–540. doi: 10.14198/jhse.2018.133.05
Hoiland, R. L., Bain, A. R., Rieger, M. G., Bailey, D. M., and Ainslie, P. N. (2016). Hypoxemia, oxygen content, and the regulation of cerebral blood flow. Am. J. Physiol. Regul. Integr. Comp. Physiol 310, R398–R413. doi: 10.1152/ajpregu.00270.2015
ISO 80601-2-56 (2017). Medical Electrical Equipment — Part 2-56: Particular Requirements for Basic Safety and Essential Performance of Clinical Thermometers for Body Temperature Measurement. ISO. Available online at: https://www.iso.org/cms/render/live/en/sites/isoorg/contents/data/standard/06/73/67348.html (accessed August 17, 2020).
ISO 80601-2-61 (2017). Medical Electrical Equipment — Part 2-61: Particular Requirements for Basic Safety and Essential Performance of Pulse Oximeter Equipment. ISO. Available online at: https://www.iso.org/cms/render/live/en/sites/isoorg/contents/data/standard/06/79/67963.html (accessed August 17, 2020).
Jensen, B. N., Jensen, F. S., Madsen, S. N., and Løssl, K. (2000). Accuracy of digital tympanic, oral, axillary, and rectal thermometers compared with standard rectal mercury thermometers. Eur. J. Surg. 166, 848–851. doi: 10.1080/110241500447218
Kallmünzer, B., Beck, A., Schwab, S., and Kollmar, R. (2011). Local head and neck cooling leads to hypothermia in healthy volunteers. Cerebrovasc. Dis. 32, 207–210. doi: 10.1159/000329376
Keene, T., Brearley, M., Bowen, B., and Walker, A. (2015). Accuracy of tympanic temperature measurement in firefighters completing a simulated structural firefighting task. Prehosp. Disaster Med. 30, 461–465. doi: 10.1017/S1049023X15005038
Kistemaker, J. A., Den Hartog, E. A., and Daanen, H. A. M. (2006). Reliability of an infrared forehead skin thermometer for core temperature measurements. J. Med. Eng. Technol. 30, 252–261. doi: 10.1080/03091900600711381
Kyriacou, P. A. (2006). Pulse oximetry in the oesophagus. Physiol. Meas 27, R1–35. doi: 10.1088/0967-3334/27/1/R01
Leboeuf, S. F., Aumer, M. E., Kraus, W. E., Johnson, J. L., and Duscha, B. (2014). Earbud-based sensor for the assessment of energy expenditure, HR, and VO2max. Med. Sci. Sports Exerc. 46, 1046–1052. doi: 10.1249/MSS.0000000000000183
Lee, J.-Y., Nakao, K., Takahashi, N., Son, S.-Y., Bakri, I., and Tochihara, Y. (2011). Validity of infrared tympanic temperature for the evaluation of heat strain while wearing impermeable protective clothing in hot environments. Ind. Health 49, 714–725. doi: 10.2486/indhealth.MS1291
Lee, S. M., Williams, W. J., and Fortney Schneider, S. M. (2000). Core temperature measurement during supine exercise: esophageal, rectal, and intestinal temperatures. Aviat. Space Environ. Med. 71, 939–945. doi: 10.2486/indhealth.ms1291
Lemay, M., Bertschi, M., Sola, J., Renevey, P., Parak, J., and Korhonen, I. (2014). “Application of optical heart rate monitoring,” in Werable Sensors: Fundamentals, Implementation and Applications, ed E. Savonov and M. R. Neuman (Amsterdam: Elsevier), 105–129.
Lim, C. L., Byrne, C., and Lee, J. K. (2008). Human thermoregulation and measurement of body temperature in exercise and clinical settings. Ann. Acad. Med. Singap. 37, 347–353.
McCarthy, P. W., and Heusch, A. I. (2006). The vagaries of ear temperature assessment. J. Med. Eng. Technol. 30, 242–251. doi: 10.1080/03091900600711415
Morán-Navarro, R., Courel-Ibáñez, J., Martínez-Cava, A., Conesa-Ros, E., Sánchez-Pay, A., Mora-Rodriguez, R., et al. (2019). Validity of skin, oral and tympanic temperatures during exercise in the heat: effects of wind and sweat. Ann. Biomed. Eng. 47, 317–331. doi: 10.1007/s10439-018-02115-x
Muir, I. H., Bishop, P. A., Lomax, R. G., and Green, J. M. (2001). Prediction of rectal temperature from ear canal temperature. Ergonomics. 44, 962–972. doi: 10.1080/00140130110068933
Munn, Z., Peters, M. D. J., Stern, C., Tufanaru, C., McArthur, A., and Aromataris, E. (2018). Systematic review or scoping review? Guidance for authors when choosing between a systematic or scoping review approach. BMC Med. Res. Methodol. 18:143. doi: 10.1186/s12874-018-0611-x
Muth, C.-M., Shank, E., Hauser, B., Radermacher, P., Groger, M., and Ehrmann, U. (2010). Infrared ear thermometry in water-related accidents-not a good choice. J. Emerg. Med. 38, 417–421. doi: 10.1016/j.jemermed.2007.10.061
Nagano, C., Tsutsui, T., Monji, K., Sogabe, Y., Idota, N., and Horie, S. (2010). Technique for continuously monitoring core body temperatures to prevent heat stress disorders in workers engaged in physical labor. J. Occup. Health 52, 167–175. doi: 10.1539/joh.L9160
Nakada, H., Horie, S., Kawanami, S., Inoue, J., Iijima, Y., Sato, K., et al. (2017). Development of a method for estimating oesophageal temperature by multi-locational temperature measurement inside the external auditory canal. Int. J. Biometeorol. 61, 1545–1554. doi: 10.1007/s00484-017-1333-1
Ota, H., Chao, M., Gao, Y., Wu, E., Tai, L.-C., Chen, K., et al. (2017). 3D printed “Earable” smart devices for real-time detection of core body temperature. ACS Sens. 2, 990–997. doi: 10.1021/acssensors.7b00247
Ouzzani, M., Hammady, H., Fedorowicz, Z., and Elmagarmid, A. (2016). Rayyan—a web and mobile app for systematic reviews. Syst. Rev. 5:210. doi: 10.1186/s13643-016-0384-4
Paal, P., Brugger, H., and Strapazzon, G. (2018). Accidental hypothermia. Handb. Clin. Neurol. 157, 547–563. doi: 10.1016/B978-0-444-64074-1.00033-1
Parak, J. (2018). Evaluation of Wearable Optical Heart Rate Monitoring Sensors. Tampere: Tampere University of Technology.
Park, J.-H., Jang, D.-G., Park, J. W., and Youm, S.-K. (2015). Wearable sensing of in-ear pressure for heart rate monitoring with a piezoelectric sensor. Sensors 15, 23402–23417. doi: 10.3390/s150923402
Pasquier, M., Rousson, V., Darocha, T., Bouzat, P., Kosiński, S., Sawamoto, K., et al. (2019). Hypothermia outcome prediction after extracorporeal life support for hypothermic cardiac arrest patients: an external validation of the HOPE score. Resuscitation 139, 321–328. doi: 10.1016/j.resuscitation.2019.03.017
Passler, S., Muller, N., Senner, V., Passler, S., Mueller, N., and Senner, V. (2019). In-ear pulse rate measurement: a valid alternative to heart rate derived from electrocardiography? Sensors 19:3641. doi: 10.3390/s19173641
Patel, N., Smith, C. E., Pinchak, A. C., and Hagen, J. F. (1996). Comparison of esophageal, tympanic, and forehead skin temperatures in adult patients. J. Clin. Anesth. 8, 462–468. doi: 10.1016/0952-8180(96)00103-1
Peters, M. D. J. (2016). In no uncertain terms: the importance of a defined objective in scoping reviews. JBI Database Syst. Rev. Implement Rep. 14, 1–4. doi: 10.11124/jbisrir-2016-2838
Pham, S., Yeap, D., Escalera, G., Basu, R., Wu, X., Kenyon, N. J., et al. (2020). Wearable sensor system to monitor physical activity and the physiological effects of heat exposure. Sensors 20:855. doi: 10.3390/s20030855
Poh, M., Kim, K., Goessling, A., Swenson, N., Picard, R., Poh, M.-Z., et al. (2012). Cardiovascular monitoring using earphones and a mobile device. IEEE Pervas. Comp. 11, 18–26. doi: 10.1109/MPRV.2010.91
Poh, M.-Z., Swenson, N. C., and Picard, R. W. (2010). Motion-tolerant magnetic earring sensor and wireless earpiece for wearable photoplethysmography. IEEE Trans. Inf. Technol. Biomed. 14, 786–794. doi: 10.1109/TITB.2010.2042607
Poh, N., and Kittler, J. (2012). A unified framework for biometric expert fusion incorporating quality measures. IEEE Trans. Pattern Anal. Mach. Intell. 34, 3–18. doi: 10.1109/TPAMI.2011.102
Procter, E., Brugger, H., and Burtscher, M. (2018). Accidental hypothermia in recreational activities in the mountains: a narrative review. Scand. J. Med. Sci. Sports 28, 2464–2472. doi: 10.1111/sms.13294
Pryor, R. R., Seitz, J. R., Morley, J., Suyama, J., Guyette, F. X., Reis, S. E., et al. (2012). Estimating core temperature with external devices after exertional heat stress in thermal protective clothing. Prehosp. Emerg. Care 16, 136–141. doi: 10.3109/10903127.2011.614047
Ring, E. F. J., McEvoy, H., Jung, A., Zuber, J., and Machin, G. (2010). New standards for devices used for the measurement of human body temperature. J. Med. Eng. Technol. 34, 249–253. doi: 10.3109/03091901003663836
Rosenberg, J., and Pedersen, M. H. (1990). Nasal pulse oximetry overestimates oxygen saturation. Anaesthesia 45, 1070–1071. doi: 10.1111/j.1365-2044.1990.tb14892.x
Schmäl, F., Loh-van den Brink, M., and Stoll, W. (2006). Effect of the status after ear surgery and ear pathology on the results of infrared tympanic thermometry. Eur. Arch. Otorhinolaryngol. 263, 105–110. doi: 10.1007/s00405-005-0966-6
Schneider, C., Hanakam, F., Wiewelhove, T., Döweling, A., Kellmann, M., Meyer, T., et al. (2018). Heart rate monitoring in team sports-a conceptual framework for contextualizing heart rate measures for training and recovery prescription. Front. Physiol. 9:639. doi: 10.3389/fphys.2018.00639
Skaiaa, S. C., Bratteb,ø, G., Aßmus, J., and Thomassen, Ø. (2015). The impact of environmental factors in pre-hospital thermistor-based tympanic temperature measurement: a pilot field study. Scand. J. Trauma Resusc. Emerg. Med. 23:72. doi: 10.1186/s13049-015-0148-5
Stensrud, T., Rossvoll, Ø., Mathiassen, M., Melau, J., Illidi, C., Østgaard, H. N., et al. (2020). Lung function and oxygen saturation after participation in Norseman Xtreme Triathlon. Scand. J. Med. Sci. Sports. 30, 1008–1016. doi: 10.1111/sms.13651
Strapazzon, G., Forti, A., Rauch, S., and Brugger, H. (2019). The integration of prehospital standard operating procedures and in-hospital HOPE score for management of hypothermic patients in cardiac arrest. Resuscitation 141, 212–213. doi: 10.1016/j.resuscitation.2019.05.041
Strapazzon, G., Procter, E., Paal, P., and Brugger, H. (2014). Pre-hospital core temperature measurement in accidental and therapeutic hypothermia. High Alt. Med. Biol. 15, 104–111. doi: 10.1089/ham.2014.1008
Strapazzon, G., Procter, E., Putzer, G., Avancini, G., Dal Cappello, T., Überbacher, N., et al. (2015). Influence of low ambient temperature on epitympanic temperature measurement: a prospective randomized clinical study. Scand. J. Trauma Resusc. Emerg. Med. 23:90. doi: 10.1186/s13049-015-0172-5
Sund-Levander, M., and Grodzinsky, E. (2013). Assessment of body temperature measurement options. Br. J. Nurs. 22, 942, 944–950. doi: 10.12968/bjon.2013.22.16.942
Suzuki, T., Wada, K., Wada, Y., Kagitani, H., Arioka, T., Maeda, K., et al. (2010). The validity of mass body temperature screening with ear thermometers in a warm thermal environment. Tohoku J. Exp. Med. 222, 89–95. doi: 10.1620/tjem.222.89
Tasli, H., and Gökgöz, M. C. (2018). Does central tympanic membrane perforation affect infrared tympanic thermometer measurements in adults? J. Otol. 13, 128–130. doi: 10.1016/j.joto.2018.09.001
Teunissen, L., de Haan, A., de Koning, J., Clairbois, H., Daanen, H., Teunissen, L. P. J., et al. (2011). Limitations of temperature measurement in the aural canal with an ear mould integrated sensor. Physiol. Measure. 32, 1403–1416. doi: 10.1088/0967-3334/32/9/004
Tomita, Y. (2016). Asynchronous noise removal for earbud-based PPG sensors. Conf. Proc. IEEE Eng. Med. Biol. Soc. 2016, 259–262. doi: 10.1109/EMBC.2016.7590689
Tomita, Y., Mitsukura, Y., Tomita, Y., and Mitsukura, Y. (2018). An earbud-based photoplethysmography and its application. Electron. Commun. Japn. 101, 32–38. doi: 10.1002/ecj.12019
Tricco, A. C., Lillie, E., Zarin, W., O'Brien, K. K., Colquhoun, H., Levac, D., et al. (2018). PRISMA Extension for Scoping Reviews (PRISMA-ScR): checklist and explanation. Ann. Intern. Med. 169, 467–473. doi: 10.7326/M18-0850
Venema, B., Blanik, N., Blazek, V., Gehring, H., Opp, A., and Leonhardt, S. (2012). Advances in reflective oxygen saturation monitoring with a novel in-ear sensor system: results of a human hypoxia study. IEEE Trans. Biomed. Eng. 59, 2003–2010. doi: 10.1109/TBME.2012.2196276
Vogel, S., Hülsbusch, M., Hennig, T., Blazek, V., and Leonhardt, S. (2009). In-ear vital signs monitoring using a novel microoptic reflective sensor. IEEE Trans. Inf. Technol. Biomed. 13, 882–889. doi: 10.1109/TITB.2009.2033268
von Rosenberg, W., Chanwimalueang, T., Goverdovsky, V., Peters, N., Papavassiliou, C., Mandic, D., et al. (2017). Hearables: feasibility of recording cardiac rhythms from head and in-ear locations. R Soc. Open Sci. 4:171214. doi: 10.1098/rsos.171214
Wilkinson, D. M., Carter, J. M., Richmond, V. L., Blacker, S. D., and Rayson, M. P. (2008). The effect of cool water ingestion on gastrointestinal pill temperature. Med. Sci. Sports Exerc. 40, 523–528. doi: 10.1249/MSS.0b013e31815cc43e
Williams, T. B., Corbett, J., McMorris, T., Young, J. S., Dicks, M., Ando, S., et al. (2019). Cognitive performance is associated with cerebral oxygenation and peripheral oxygen saturation, but not plasma catecholamines, during graded normobaric hypoxia. Exp. Physiol. 104, 1384–1397. doi: 10.1113/EP087647
Yamakoshi, T., Matsumura, K., Rolfe, P., Tanaka, N., Yamakoshi, Y., and Takahashi, K. (2013). A novel method to detect heat illness under severe conditions by monitoring tympanic temperature. Aviat. Space Environ. Med. 84, 692–700. doi: 10.3357/ASEM.3542.2013
Keywords: wearables, earbuds, physiological monitoring, temperature, oxygen saturation, heart rate, hypothermia, heat exercise
Citation: Masè M, Micarelli A and Strapazzon G (2020) Hearables: New Perspectives and Pitfalls of In-Ear Devices for Physiological Monitoring. A Scoping Review. Front. Physiol. 11:568886. doi: 10.3389/fphys.2020.568886
Received: 02 June 2020; Accepted: 02 September 2020;
Published: 16 October 2020.
Edited by:
Volker Scheer, Fondation de la science des sports ultra, FranceReviewed by:
Danilo Mandic, Imperial College London, United KingdomToshiyo Tamura, Waseda University, Japan
Copyright © 2020 Masè, Micarelli and Strapazzon. This is an open-access article distributed under the terms of the Creative Commons Attribution License (CC BY). The use, distribution or reproduction in other forums is permitted, provided the original author(s) and the copyright owner(s) are credited and that the original publication in this journal is cited, in accordance with accepted academic practice. No use, distribution or reproduction is permitted which does not comply with these terms.
*Correspondence: Giacomo Strapazzon, Z2lhY29tby5zdHJhcGF6em9uQGV1cmFjLmVkdQ==
†These authors have contributed equally to this work