- Department of Biomedical and Clinical Sciences, Linköping University, Linköping, Sweden
Voltage-gated potassium channels of the KV7 family are expressed in many tissues. The physiological importance of KV7 channels is evident from specific forms of disorders linked to dysfunctional KV7 channels, including variants of epilepsy, cardiac arrhythmia and hearing impairment. Thus, understanding how KV7 channels are regulated in the body is of great interest. This Mini Review focuses on the effects of polyunsaturated fatty acids (PUFAs) on KV7 channel activity and possible underlying mechanisms of action. By summarizing reported effects of PUFAs on KV7 channels and native KV7-mediated currents, we conclude that the generally observed effect is a PUFA-induced increase in current amplitude. The increase in current is commonly associated with a shift in the voltage-dependence of channel opening and in some cases with increased maximum conductance. Auxiliary KCNE subunits, which associate with KV7 channels in certain tissues, may influence PUFA effects, though findings are conflicting. Both direct and indirect activating PUFA effects have been described, direct effects having been most extensively studied on KV7.1. The negative charge of the PUFA head-group has been identified as critical for electrostatic interaction with conserved positively charged amino acids in transmembrane segments 4 and 6. Additionally, the localization of double bonds in the PUFA tail tunes the apparent affinity of PUFAs to KV7.1. Indirect effects include those mediated by PUFA metabolites. Indirect inhibitory effects involve KV7 channel degradation and re-distribution from lipid rafts. Understanding how PUFAs regulate KV7 channels may provide insight into physiological regulation of KV7 channels and bring forth new therapeutic strategies.
Introduction
It is well established that lipids can influence the function of voltage-gated ion channels and their organization in the membrane (Dart, 2010; Elinder and Liin, 2017). Specific members within the KV7 family of voltage-gated potassium channels have been under intense study, owing to their physiological regulation by the phospholipid PIP2 (phosphatidylinositol 4,5-bisphosphate; reviewed in Zaydman and Cui, 2014; Taylor and Sanders, 2017). However, several years of studies have revealed that unesterified, so called free fatty acids, may also regulate KV7 channel function. Polyunsaturated fatty acids (PUFAs) in particular have emerged as interesting KV7 modulators. This Mini Review will provide a brief essential background on KV7 channels and PUFAs, followed by a summary of our present understanding of PUFAs as KV7 channel modulators and potential future developments.
Physiological Role and General Architecture of KV7 Channels
The KV7 family of voltage-gated potassium channels, of which there are five different isoforms, termed KV7.1 to KV7.5, are encoded by the KCNQ genes. The tissue distribution of KV7 subtypes varies, and the channels serve different physiological roles (Figure 1A). KV7.1 (in complex with the auxiliary KCNE1 protein, see further details below) is most famous for generating the slow current IKs in cardiomyocytes, important for cardiac repolarization (Barhanin et al., 1996; Sanguinetti et al., 1996), while also maintaining the ionic balance of endolymph in the inner ear (Neyroud et al., 1997). Heteromers of KV7.2 and KV7.3 generate the neuronal M-current (IM) important for stabilizing the negative resting membrane potential of neurons and thereby regulating excitability (Wang et al., 1998; Cooper et al., 2000). KV7.4 also contributes to maintaining the ionic balance of endolymph (Kubisch et al., 1999; Kharkovets et al., 2000), as well as contributing to the negative membrane potential in smooth muscle cells at rest (Jepps et al., 2011; Chadha et al., 2014; Jepps et al., 2015). KV7.5 is suggested to form heteromers with other neuronal and smooth muscle KV7 subtypes and contribute to their function in these tissues (Lerche et al., 2000; Chadha et al., 2014). Because of their important role in physiology, dysfunctional KV7 channels are often linked to disorders characterized by abnormal potassium ion conductance, including cardiac arrhythmia, hearing impairment, epilepsy, pain, and hypertension (Barrese et al., 2018). For a more extensive overview of expression and physiological and pathological implications of KV7 channels, we recommend recent reviews on this topic (Barrese et al., 2018; Miceli et al., 2018).
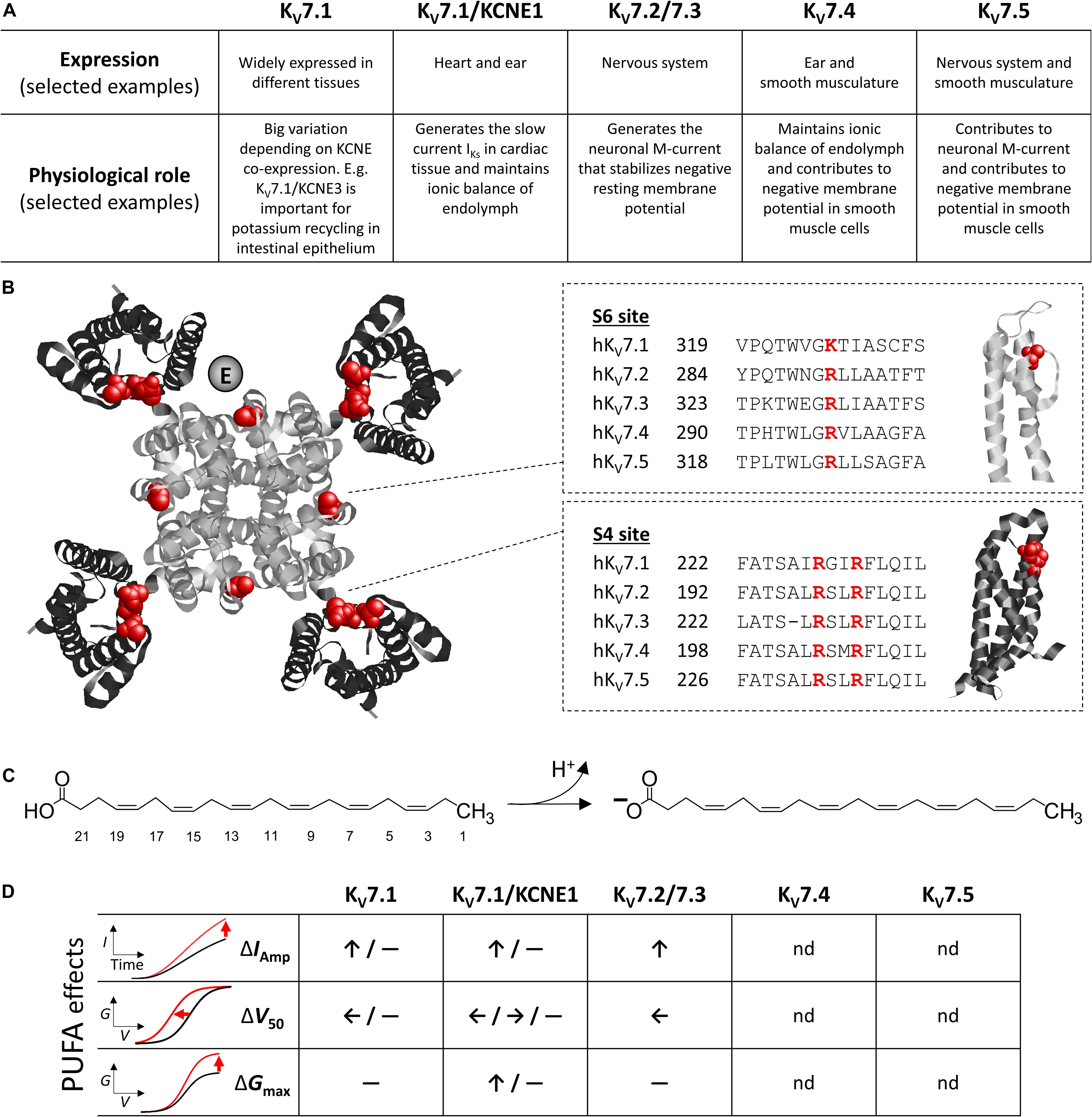
Figure 1. Overview of KV7 channel and PUFA molecular structures, and typified PUFA effects. (A) Schematic overview of KV7 subtype expression and functional role. Note that these are examples, as some KV7 subtypes have widespread expression and function. (B) Top view of KV7.1 (PDB: 5VMS) with central pore domain in gray and peripheral voltage-sensing domains in black. Putative localization of KCNE at one KV7.1 channel subunit is indicated [each subunit may accommodate one KCNE subunit (Sun and MacKinnon, 2020)]. Experimentally identified positively charged residues important for PUFA effects in KV7.1 (Liin et al., 2018) are highlighted in red. Sequence alignment of the S6 site (important for Gmax effect) and S4 site (important for V50 effect) of all KV7 isoforms are provided along with a side view of relevant channel domain. (C) Structure of the PUFA DHA, which has a carboxyl head linked to a 22-carbon long aliphatic tail with six cis double bonds. Deprotonation of the carboxyl head occurs at pH exceeding the pKa of the head-group, this endows DHA with a single negative charge. (D) Schematic overview of PUFA effect on current amplitude (IAmp), mid-point of the G(V) curve (V50), and maximum conductance (Gmax) on indicated KV7 subtypes. Please refer to Table 1 for further details. nd denotes not determined.
Each KCNQ gene encodes one KV7 subunit, composed of six transmembrane segments (helices, referred to as S1–S6) and intracellular N and C termini (Jentsch, 2000). Four such KV7 subunits assemble into functional tetrameric KV7 channels, which can be either homomeric or heteromeric (Schwake et al., 2003; Schwake et al., 2006). A cryogenic electron microscopy structure of KV7.1 visualizes how transmembrane segments S5–S6 of all four subunits form the central pore domain, whereas S1 to S4 of each subunit form the peripheral voltage-sensing domains (Figure 1B; Sun and MacKinnon, 2017). The determined structure reveals a domain swapped architecture, meaning that the voltage-sensing domain of one subunit lies adjacent to the pore-forming segments of its neighboring subunit (Sun and MacKinnon, 2017). Conserved, positively charged arginines in S4 of the voltage-sensing domain act as the primary voltage-sensing residues in KV7 channels (Panaghie and Abbott, 2007; Miceli et al., 2008). Under hyperpolarized conditions, S4 is in a “downwards,” internal, conformation, and the gate (located in the internal part of S6) of the central ion conducting pore is closed (Cui, 2016). Upon depolarization, S4 moves via intermediate conformations to an “upwards,” external, conformation (Barro-Soria et al., 2014; Zaydman et al., 2014; Taylor et al., 2020), which triggers opening of the S6 gate and potassium conductance through the pore (Cui, 2016; Hou et al., 2020). Endogenous or exogenous ligands and auxiliary proteins may interact with and modulate the activity of KV7 channels by altering S4 movement, gate opening, or the coupling between the two. For instance, auxiliary KCNE subunits (KCNE1-5) interact with KV7 channels to modulate their expression and biophysical properties (Abbott, 2016; Barrese et al., 2018). Several studies assign the single-transmembrane segment of KCNE subunits to a space between neighboring voltage-sensing domains (see Figure 1B for putative KCNE localization; e.g., Chung et al., 2009; Xu et al., 2013; Sun and MacKinnon, 2020).
Structure and Properties of PUFAs
Polyunsaturated fatty acids are naturally occurring lipids known to modulate the activity of numerous voltage-gated ion channels (reviewed in Elinder and Liin, 2017). PUFA characteristics include a carboxylic acid “head-group” and an unbranched, aliphatic hydrocarbon “tail” with at least two double bonds in cis geometry (Figure 1C). The carboxyl head-group is either uncharged or negatively charged, depending on the protonation status (Figure 1C). The pKa value of carboxyl heads of PUFAs in proximity of ion channels approaches 7.4 (Hamilton, 1998; Liin et al., 2015), and so approximately 50% of PUFA head-groups are expected to be deprotonated and negatively charged at physiological pH. The cis double bonds allow polyunsaturated tails to bend and explore geometries that are not possible in the absence of cis double bonds (Feller, 2008; Yazdi et al., 2016). PUFAs are typically described according to the number of carbons and double bonds in the tail or their omega classification. Figure 1C shows the structure of docosahexaenoic acid (DHA), which has 22 carbons and 6 double bonds in its tail (22:6) and is an omega-3 PUFA (i.e., the first double bond is at the 3rd carbon from the methyl end). Examples of other physiologically relevant PUFAs that can be obtained via diet or synthesized from other essential fatty acids in the human body are arachidonic acid (AA, 20:4, omega-6), linoleic acid (LA, 18:2, omega-6), alpha-linolenic acid (ALA, 18:3, omega-3), and eicosapentaenoic acid (EPA, 20:5, omega-3). Reported physiological levels of PUFAs in plasma, serum, or cerebrospinal fluid are around 10–50 μM, but may reach higher levels during, for instance, excessive dietary PUFA intake (Conquer and Holub, 1998; Fraser et al., 2003; Brouwer et al., 2006).
Reported PUFA Effects on KV7 Channels and Currents
Table 1 summarizes reported PUFA effects on heterologously expressed KV7 channels and isolated native currents generated by KV7 channels. Notably, PUFA effects have thus far only been studied on KV7.1–KV7.3. A majority of studies have explored the acute effects of extracellular application of AA, ALA, DHA, EPA, or LA. These effects are achieved within the range of minutes after PUFA application. The most consistently observed PUFA effect on heterologously expressed KV7 channels is an increased current amplitude at a range of negative voltages, an effect reported for several PUFAs tested on KV7.1 and heteromeric KV7.2/7.3 channels (Table 1). Several studies report a PUFA-induced shift in the conductance versus voltage [G(V)] curve toward more negative voltages (described as negative shift in V50 in Table 1; Liin et al., 2015; Moreno et al., 2015; Liin et al., 2016a). Additionally, PUFAs may increase the maximum conductance observed at the most positive voltages (described as increase in Gmax in Table 1; Bohannon et al., 2020). Both the shift in V50 and increase in Gmax may contribute to the overall increase in current amplitude at different voltages. For clarity, we will refer to the three effects as “increase in current amplitude,” “shift in V50,” and “increase in Gmax.” Figure 1D schematically illustrates PUFA effects on each of these parameters on different KV7 subtypes, whereas Table 1 reflects the complexity of effects and highlights examples of reported effects at specific PUFA concentrations. Typically, PUFA concentrations of 7 μM or higher are required to induce significant effects. Note that PUFA effects on current amplitude have typically been quantified at relatively depolarized voltages, which may not be physiologically relevant. However, because PUFAs generally also shift V50 toward negative voltages, the relative increase in current amplitude is larger at less depolarized voltages. Although studies rarely quantify PUFA effects in a more physiologically relevant voltage range, a prominent increase in current amplitude at less depolarized voltages is observed in several studies (Doolan et al., 2002; Liin et al., 2015; Moreno et al., 2015; Liin et al., 2016a).
In addition, PUFA effects on opening and closing kinetics have been described (Doolan et al., 2002; Moreno et al., 2015). However, effects on kinetics appear complex, with a range of described effects varying from PUFA to PUFA (Doolan et al., 2002; Moreno et al., 2015). Moreover, prolonged PUFA exposure over several days triggers a plethora of convoluted effects resulting in either negative or positive shift in V50, depending on PUFA (Moreno et al., 2015).
Reported PUFA effects on native KV7 currents largely agree with effects on heterologously expressed KV7 channels (Table 1). In a majority of guinea pig ventricular cardiomyocytes, acute application of DHA increases IKs amplitude (presumably generated by KV7.1/KCNE1 channels; Moreno et al., 2015). However, in a subset of cardiomyocytes DHA instead decreases IKs amplitude (Moreno et al., 2015). Several studies observe increases in IM amplitude induced by AA or DHA in bullfrog sympathetic neurons (which presumably is generated by KV7.2/7.3 channels; Villarroel, 1993, 1994; Yu, 1995).
Impact of KCNE Subunits on PUFA Effects
Because KCNE subunits associate with KV7 channels in multiple tissues, evaluating the impact of KCNE co-expression on PUFA effects is of interest. Auxiliary subunits have been shown to affect the response of other KV channels to PUFAs. For instance, DHA, or AA augmentation of the Slo1 channel is potentiated or enabled, respectively, when the channel is co-expressed with specific β subunits (Sun et al., 2007; Hoshi et al., 2013a). Furthermore, AA modulation of KV4 channel inactivation kinetics is only observed if KV4.2 or KV4.3 are co-expressed with their KChIP subunit (Holmqvist et al., 2001). We found that neither KCNE1 nor KCNE2 co-expression with KV7.2/7.3 affected the ability of DHA to shift V50 of KV7.2/7.3 (Liin et al., 2016a). However, the occurrence and physiological relevance of complexes formed by KV7.2/7.3 and KCNE subunits remains questionable at present. Conflicting results are found regarding the impact of KCNE1 co-expression with KV7.1 on PUFA effects (Table 1 and Figure 1D). Doolan et al. (2002) report that KCNE1 is required for DHA-mediated effects on KV7.1 current amplitude, as they find that 20 μM of DHA increases the current amplitude of KV7.1/KCNE1, but not KV7.1. By comparison, 20 μM of EPA slowed activation kinetics for KV7.1/KCNE1 without affecting current amplitude (Doolan et al., 2002). In contrast, we report that DHA at concentrations of 7 μM or higher shifts V50 of KV7.1 toward negative voltages and that the presence of KCNE1 largely abolishes this V50 effect (Liin et al., 2015; Bohannon et al., 2020). However, we observe a DHA-induced increase in KV7.1/KCNE1 current amplitude and Gmax (Bohannon et al., 2020). A third study, by Moreno and colleagues, reports that 20 μM of DHA or EPA increases KV7.1/KCNE1 current amplitude and shifts V50 of KV7.1/KCNE1 toward negative voltages (Moreno et al., 2015). However, no EPA effect is observed on KV7.1 alone (Moreno et al., 2015). The reason for these conflicting findings is not clear. One contributing factor may be the use of different expression systems (Xenopus oocytes versus mammalian COS7 cells). As previously remarked (Valenzuela, 2016), higher PUFA concentrations may be required in experiments with Xenopus oocytes than for mammalian cells to induce comparable effects. Another aspect to consider may be the method of PUFA application. We note larger DHA effects on current amplitude upon constant DHA perfusion compared to a single DHA application (Liin et al., 2015; Bohannon et al., 2020). Both Doolan et al., and Moreno et al., describe results employing PUFA perfusion.
PUFA Properties Important for KV7 Effects
Previous studies have shown that PUFAs may interact directly with diverse ion channels to modulate their activity (Elinder and Liin, 2017). Moreno et al. (2015) concluded that the concurrent increase in KV7.1/KCNE1 current amplitude and negative shift in V50 implicate an effect on channel gating as the primary mechanism. We made the same observation in our initial work (Liin et al., 2015). In subsequent work we have since explored this possibility utilizing PUFA analogs, site-directed mutagenesis, and pH manipulation.
We find that a negatively charged PUFA head-group is critical to the shift in V50 of KV7.1 and KV7.2/7.3 toward negative voltages. Fully deprotonated PUFAs (promoted by an alkaline extracellular solution) cause larger negative V50 shifts (Liin et al., 2015; Larsson et al., 2018; Bohannon et al., 2019). Protonated PUFAs and uncharged PUFA analogs (PUFA methyl esters) are unable to shift V50 (Liin et al., 2015, 2016a). Positively charged PUFA analogs (PUFA amines) instead shift V50 toward more positive voltages (Liin et al., 2015, 2016a; Larsson et al., 2018). This set of experiments implies an electrostatic mechanism of action underlying PUFA effects on V50, and shows that the magnitude of this electrostatic effect is determined by the protonation status of the PUFA head-group. Notably, we observe clear activating effects by PUFAs on KV7.1/KCNE1 (comparable to those on KV7.1 alone) during experimental conditions that promote PUFA deprotonation (Liin et al., 2015; Larsson et al., 2018). This suggests that the lack of PUFA effects on KV7.1/KCNE1 in our hands is because of KCNE1-induced protonation of the PUFA head-group. Moreover, these findings suggest that provided the head-group of a given PUFA analog has a low pKa value (and thus is deprotonated at physiological pH) said PUFA analog can be utilized to achieve greater effects on KV7.1 alone at physiological pH while simultaneously retaining activating effects on KV7.1/KCNE1 at physiological pH.
The PUFA head-group is not the sole determinant of the magnitude of PUFA effects. The first indication of this came from studies by Doolan et al. (2002) and Moreno et al. (2015) both of which show DHA induces overall greater effects on KV7.1/KCNE1 than EPA does. This is in agreement with our comparison of 16 PUFAs with different tail properties, in which we find that PUFAs with double bonds proximal to the head-group have a higher apparent affinity to KV7.1/KCNE1, compared with PUFAs with more distal double bonds (Bohannon et al., 2019). In contrast, there was either no or weak correlation between tail length or number of double tail bonds and KV7.1/KCNE1 effects (Bohannon et al., 2019). The proximal localization of double bonds relative to the head-group of DHA may contribute to the relatively greater effect, when compared to EPA at specific concentrations. The mechanistic basis for why proximal double bonds enhance PUFA interaction with KV7.1 remains unknown, but may be related to flexibility in the hydrocarbon tail. Studies of PUFA interaction with the Slo1-β1 BK channel suggest that bending at specific carbons distally in the hydrocarbon tail are required for interaction with the channel (Tian et al., 2016). Speculatively, greater bending capabilities close to the head-group may be required for high affinity binding of PUFAs to KV7.1.
Proposed Direct PUFA Sites of Action in KV7
Polyunsaturated fatty acid analogs with lower head-group pKa values have been instrumental in determining the mechanism of action of PUFAs on KV7.1. PUFA analogs with glycine or taurine head-groups have estimated pKa values that are 1 and 5.5 units lower than that of carboxyl head-groups, respectively, (Bohannon et al., 2020), and are examples of PUFA analogs with exaggerated acute effects on V50 of both KV7.1 and KV7.1/KCNE1 at physiological pH (Liin et al., 2015). In experiments utilizing DHA-glycine (DHA-Gly) or AA-taurine (N-AT), we have shown that PUFA analogs interact with S4 to shift V50. This is observed in voltage-clamp fluorometry experiments that track S4 movement as a PUFA-induced shift in S4 movement toward negative voltages (Liin et al., 2015, 2016b). Individual mutations of the two top arginines in S4 of KV7.1 into uncharged glutamines (R228Q and R231Q) either reduce or abrogate entirely the ability of PUFAs to shift V50 of KV7.1 and KV7.1/KCNE1 (Liin et al., 2015, 2018). These findings suggest that electrostatic interaction between the negative PUFA head-group and these positively charged arginines in S4 facilitates the upward S4 movement, which contributes to the PUFA effect on V50 (illustrated as “S4 site” in Figure 1B).
Although charge-neutralizing mutation of S4 arginines removes the V50 effect of PUFAs, an increase in Gmax of KV7.1 and KV7.1/KCNE1 is still observed (Liin et al., 2018). This increase in Gmax is also electrostatic in nature, as it is abolished following mutation of a lysine at the top of S6 into an uncharged glutamine (Liin et al., 2018). Experiments combined with molecular dynamics simulations suggest that electrostatic interaction between the negative PUFA head-group and the positively charged lysine at position 326 of S6 (illustrated as “S6 site” in Figure 1B) triggers conformational changes in the ion-conducting pore in and near the selectivity filter. These conformational changes appear to promote potassium ion conductance in a way that contributes to the PUFA effect on Gmax. Altogether, these findings suggest that PUFAs have at least two independent sites of electrostatic action in KV7.1 channels. Similar sites have been suggested for KV7.2 and, to some extent, KV7.3 (Larsson et al., 2020).
Proposed Indirect PUFA Effects on KV7
Besides direct effects, PUFAs may influence KV7 channels through other mechanisms, including altering bilayer organization and properties or generating active PUFA metabolites. Indication of additional pathways of PUFA modulation of KV7 channels comes from prolonged exposure (48 h) of KV7.1/KCNE1 channels to DHA or EPA (Moreno et al., 2015). For instance, prolonged DHA or EPA exposure reduces total KV7.1 protein levels in COS7 cells, presumably by inducing protein degradation (Moreno et al., 2015). Prolonged exposure also triggers spatial redistribution of KV7.1/KCNE1 in the membrane, presumably by disruption of lipid rafts (Moreno et al., 2015). It has been speculated that mechanical properties of the membrane (such as thickness and stiffness) may contribute to altered KV7.1/KCNE1 behavior in different membrane microdomains (Moreno et al., 2015). Given that PUFAs regulate other ion channels through modulating the mechanical properties of membranes (Boland and Drzewiecki, 2008), the possible contributions of such indirect PUFA effects on KV7 channels in different microdomains warrants further studies.
Regarding the influence of active metabolites, AA is central in oxygenase-driven metabolic pathways that generate leukotrienes and prostaglandins (Shimizu and Wolfe, 1990). Treatment of bullfrog sympathetic neurons with an inhibitor of the lipoxygenase pathway reduces IM and prevents AA-mediated increases in IM amplitude (Villarroel, 1993; Yu, 1995), presumably by impeding downstream AA metabolites that modulate IM effects (Yu, 1995). These findings highlight the multifaceted nature of PUFA signaling and the challenges of dissecting underlying mechanisms in more complex cellular systems and longer time scales.
Future Directions
Several studies report that micromolar concentrations of PUFAs increase KV7.1-7.3 currents and have provided insights into mechanisms of how acute and prolonged PUFA exposure impacts KV7 channels. However, there are still several open questions. For instance:
(i) Given the conservation among KV7 channels of charged residues identified to be crucial to PUFA effects (Figure 1B), are the effects and underlying mechanisms of action conserved among all KV7 isoforms?
(ii) What is the physiological relevance of PUFA effects reported on heterologously expressed KV7 channels? PUFA have been reported to alter cardiac and neuronal excitability [e.g., by shortening action potential duration and QT interval, and increasing the threshold for action potential firing (e.g., Leaf et al., 2003; Boland and Drzewiecki, 2008; DeGiorgio and Taha, 2016; Liin et al., 2016a; Skarsfeldt et al., 2020)], and vascular tone [by promoting vascular smooth muscle relaxation (e.g., Hoshi et al., 2013b; Limbu et al., 2018)]. However, as PUFAs act on many types of channels, the extent of Kv7 contribution to such general effects remain to be determined.
(iii) Can a mechanistic understanding of PUFA modulation of KV7 channels open up new therapeutic avenues? For instance, how viable is modulation of circulating PUFA levels for the tuning of neuronal and cardiac excitability, and what are the prospects of PUFA analogs as pharmacological KV7 channel activators?
To conclude, PUFAs modulate the activity of KV7 channels through a range of mechanisms. Studies of heterologously expressed KV7 channels have revealed important insights into mechanisms underlying KV7 channel activation via direct PUFA-channel interactions. However, several aspects of PUFA modulation of KV7 channels remain unclear and require further studies. This is particularly true for comprehending the compounded results of both direct and indirect PUFA effects in differing time scales and cellular systems.
Author Contributions
All authors designed the study and wrote the manuscript.
Funding
This project has received funding from the European Research Council (ERC) under the European Union’s Horizon 2020 research and innovation program (grant agreement No. 850622).
Conflict of Interest
A patent application (#62/032,739) including a description of the interaction of charged lipophilic compounds with the KV7.1 channel has been submitted by the University of Miami with SL identified as one of the inventors.
The remaining authors declare that the research was conducted in the absence of any commercial or financial relationships that could be construed as a potential conflict of interest.
Acknowledgments
We thank Dr. H. Peter Larsson, University of Miami, and Dr. Fredrik Elinder, Linköping University, for comments on the manuscript.
References
Abbott, G. W. (2016). “Chapter 1 - The KCNE family of ion channel regulatory subunits,” Ion Channels in Health and Disease. G. S. Pitt (Boston, MA: Academic Press), 1–24. doi: 10.1016/b978-0-12-802002-9.00001-7
Barhanin, J., Lesage, F., Guillemare, E., Fink, M., Lazdunski, M., and Romey, G. (1996). K(V)LQT1 and lsK (minK) proteins associate to form the I(Ks) cardiac potassium current. Nature 384, 78–80. doi: 10.1038/384078a0
Barrese, V., Stott, J. B., and Greenwood, I. A. (2018). KCNQ-Encoded potassium channels as therapeutic targets. Annu. Rev. Pharmacol. Toxicol. 58, 625–648. doi: 10.1146/annurev-pharmtox-010617-052912
Barro-Soria, R., Rebolledo, S., Liin, S. I., Perez, M. E., Sampson, K. J., Kass, R. S., et al. (2014). KCNE1 divides the voltage sensor movement in KCNQ1/KCNE1 channels into two steps. Nat. Commun. 5:3750. doi: 10.1038/ncomms4750
Behe, P., Sandmeier, K., and Meves, H. (1992). The effect of arachidonic acid on the M current of NG108-15 neuroblastoma x glioma hybrid cells. Pflugers. Arch. 422, 120–128. doi: 10.1007/bf00370411
Bohannon, B. M., Perez, M. E., Liin, S. I., and Larsson, H. P. (2019). omega-6 and omega-9 polyunsaturated fatty acids with double bonds near the carboxyl head have the highest affinity and largest effects on the cardiac IK s potassium channel. Acta Physiol. (Oxf) 225:e13186. doi: 10.1111/apha.13186
Bohannon, B. M., Wu, X., Wu, X., Perez, M. E., Liin, S. I., and Larsson, H. P. (2020). Polyunsaturated fatty acids produce a range of activators for heterogeneous IKs channel dysfunction. J. Gen. Physiol. 152:e201912396.
Boland, L. M., and Drzewiecki, M. M. (2008). Polyunsaturated fatty acid modulation of voltage-gated ion channels. Cell Biochem. Biophys. 52, 59–84. doi: 10.1007/s12013-008-9027-2
Brouwer, I. A., Zock, P. L., Camm, A. J., Bocker, D., Hauer, R. N., Wever, E. F., et al. (2006). Effect of fish oil on ventricular tachyarrhythmia and death in patients with implantable cardioverter defibrillators: the study on omega-3 fatty acids and ventricular arrhythmia (SOFA) randomized trial. JAMA 295, 2613–2619.
Chadha, P. S., Jepps, T. A., Carr, G., Stott, J. B., Zhu, H. L., Cole, W. C., et al. (2014). Contribution of kv7.4/kv7.5 heteromers to intrinsic and calcitonin gene-related peptide-induced cerebral reactivity. Arterioscler. Thromb. Vasc. Biol. 34, 887–893. doi: 10.1161/atvbaha.114.303405
Chung, D. Y., Chan, P. J., Bankston, J. R., Yang, L., Liu, G., Marx, S. O., et al. (2009). Location of KCNE1 relative to KCNQ1 in the I(KS) potassium channel by disulfide cross-linking of substituted cysteines. Proc. Natl. Acad. Sci. U.S.A. 106, 743–748. doi: 10.1073/pnas.0811897106
Conquer, J. A., and Holub, B. J. (1998). Effect of supplementation with different doses of DHA on the levels of circulating DHA as non-esterified fatty acid in subjects of Asian Indian background. J. Lipid Res. 39, 286–292.
Cooper, E. C., Aldape, K. D., Abosch, A., Barbaro, N. M., Berger, M. S., Peacock, W. S., et al. (2000). Colocalization and coassembly of two human brain M-type potassium channel subunits that are mutated in epilepsy. Proc. Natl. Acad. Sci. U.S.A. 97, 4914–4919. doi: 10.1073/pnas.090092797
Cui, J. (2016). Voltage-dependent gating: novel Insights from KCNQ1 Channels. Biophys. J. 110, 14–25. doi: 10.1016/j.bpj.2015.11.023
Dart, C. (2010). Lipid microdomains and the regulation of ion channel function. J. Physiol. 588, 3169–3178. doi: 10.1113/jphysiol.2010.191585
DeGiorgio, C. M., and Taha, A. Y. (2016). Omega-3 fatty acids (-3 fatty acids) in epilepsy: animal models and human clinical trials. Exp. Rev. Neurother. 16, 1141–1145. doi: 10.1080/14737175.2016.1226135
Doolan, G. K., Panchal, R. G., Fonnes, E. L., Clarke, A. L., Williams, D. A., and Petrou, S. (2002). Fatty acid augmentation of the cardiac slowly activating delayed rectifier current (IKs) is conferred by hminK. FASEB J. 16, 1662–1664. doi: 10.1096/fj.02-0084fje
Elinder, F., and Liin, S. I. (2017). Actions and mechanisms of polyunsaturated fatty acids on voltage-gated ion channels. Front. Physiol. 8:43. doi: 10.3389/fphys.2017.00043
Feller, S. E. (2008). Acyl chain conformations in phospholipid bilayers: a comparative study of docosahexaenoic acid and saturated fatty acids. Chem. Phys. Lipids 153, 76–80. doi: 10.1016/j.chemphyslip.2008.02.013
Fraser, D. D., Whiting, S., Andrew, R. D., Macdonald, E. A., Musa-Veloso, K., and Cunnane, S. C. (2003). Elevated polyunsaturated fatty acids in blood serum obtained from children on the ketogenic diet. Neurology 60, 1026–1029. doi: 10.1212/01.wnl.0000049974.74242.c6
Holmqvist, M. H., Cao, J., Knoppers, M. H., Jurman, M. E., Distefano, P. S., Rhodes, K. J., et al. (2001). Kinetic modulation of Kv4-mediated A-current by Arachidonic Acid is dependent on potassium channel interacting proteins. J. Neurosci. 21, 4154–4161. doi: 10.1523/jneurosci.21-12-04154.2001
Hoshi, T., Tian, Y., Xu, R., Heinemann, S. H., and Hou, S. (2013a). Mechanism of the modulation of BK potassium channel complexes with different auxiliary subunit compositions by the omega-3 fatty acid DHA. Proc. Natl. Acad. Sci. U.S.A. 110, 4822–4827. doi: 10.1073/pnas.1222003110
Hoshi, T., Wissuwa, B., Tian, Y., Tajima, N., Xu, R., Bauer, M., et al. (2013b). Omega-3 fatty acids lower blood pressure by directly activating large-conductance Ca2+-dependent K+ channels. Proc. Natl. Acad. Sci. U.S.A. 110, 4816–4821. doi: 10.1073/pnas.1221997110
Hou, P., Kang, P. W., Kongmeneck, A. D., Yang, N. D., Liu, Y., Shi, J., et al. (2020). Two-stage electro-mechanical coupling of a KV channel in voltage-dependent activation. Nat. Commun. 11, 676.
Jentsch, T. J. (2000). Neuronal KCNQ potassium channels: physiology and role in disease. Nat. Rev. Neurosci. 1, 21–30. doi: 10.1038/35036198
Jepps, T. A., Carr, G., Lundegaard, P. R., Olesen, S. P., and Greenwood, I. A. (2015). Fundamental role for the KCNE4 ancillary subunit in Kv7.4 regulation of arterial tone. J. Physiol. 593, 5325–5340. doi: 10.1113/jp271286
Jepps, T. A., Chadha, P. S., Davis, A. J., Harhun, M. I., Cockerill, G. W., Olesen, S. P., et al. (2011). Downregulation of Kv7.4 channel activity in primary and secondary hypertension. Circulation 124, 602–611. doi: 10.1161/circulationaha.111.032136
Kharkovets, T., Hardelin, J. P., Safieddine, S., Schweizer, M., El-Amraoui, A., Petit, C., et al. (2000). KCNQ4, a K+ channel mutated in a form of dominant deafness, is expressed in the inner ear and the central auditory pathway. Proc. Natl. Acad. Sci. U.S.A. 97, 4333–4338. doi: 10.1073/pnas.97.8.4333
Kubisch, C., Schroeder, B. C., Friedrich, T., Lutjohann, B., El-Amraoui, A., Marlin, S., et al. (1999). KCNQ4, a novel potassium channel expressed in sensory outer hair cells, is mutated in dominant deafness. Cell 96, 437–446. doi: 10.1016/s0092-8674(00)80556-5
Larsson, J. E., Karlsson, U., Wu, X., and Liin, S. I. (2020). Combining endocannabinoids with retigabine for enhanced M-channel effect and improved KV7 subtype selectivity. J. Gen. Physiol. 152:e202012576.
Larsson, J. E., Larsson, H. P., and Liin, S. I. (2018). KCNE1 tunes the sensitivity of KV7.1 to polyunsaturated fatty acids by moving turret residues close to the binding site. Elife 7:e37257.
Leaf, A., Xiao, Y. F., Kang, J. X., and Billman, G. E. (2003). Prevention of sudden cardiac death by n-3 polyunsaturated fatty acids. Pharmacol. Ther. 98, 355–377. doi: 10.1016/s0163-7258(03)00039-1
Lerche, C., Scherer, C. R., Seebohm, G., Derst, C., Wei, A. D., Busch, A. E., et al. (2000). Molecular cloning and functional expression of KCNQ5, a potassium channel subunit that may contribute to neuronal M-current diversity. J. Biol. Chem. 275, 22395–22400. doi: 10.1074/jbc.m002378200
Liin, S. I., Karlsson, U., Bentzen, B. H., Schmitt, N., and Elinder, F. (2016a). Polyunsaturated fatty acids are potent openers of human M-channels expressed in Xenopus laevis oocytes. Acta Physiol. (Oxf) 218, 28–37.
Liin, S. I., Larsson, J. E., Barro-Soria, R., Bentzen, B. H., and Larsson, H. P. (2016b). Fatty acid analogue N-arachidonoyl taurine restores function of IKs channels with diverse long QT mutations. Elife 5:e20272.
Liin, S. I., Silvera Ejneby, M., Barro-Soria, R., Skarsfeldt, M. A., Larsson, J. E., Starck Harlin, F., et al. (2015). Polyunsaturated fatty acid analogs act antiarrhythmically on the cardiac IKs channel. Proc. Natl. Acad. Sci. U.S.A. 112, 5714–5719. doi: 10.1073/pnas.1503488112
Liin, S. I., Yazdi, S., Ramentol, R., Barro-Soria, R., and Larsson, H. P. (2018). Mechanisms underlying the dual effect of polyunsaturated fatty acid analogs on Kv7.1. Cell Rep. 24, 2908–2918. doi: 10.1016/j.celrep.2018.08.031
Limbu, R., Cottrell, G. S., and McNeish, A. J. (2018). Characterisation of the vasodilation effects of DHA and EPA, n-3 PUFAs (fish oils), in rat aorta and mesenteric resistance arteries. PLoS one 13:e0192484. doi: 10.1371/journal.pone.0192484
Miceli, F., Soldovieri, M. V., Ambrosino, P., Manocchio, L., Mosca, I., and Taglialatela, M. (2018). Pharmacological targeting of neuronal Kv7.2/3 channels: a focus on chemotypes and receptor sites. Curr. Med. Chem. 25, 2637–2660. doi: 10.2174/0929867324666171012122852
Miceli, F., Soldovieri, M. V., Hernandez, C. C., Shapiro, M. S., Annunziato, L., and Taglialatela, M. (2008). Gating consequences of charge neutralization of arginine residues in the S4 segment of K(v)7.2, an epilepsy-linked K+ channel subunit. Biophys. J. 95, 2254–2264. doi: 10.1529/biophysj.107.128371
Moreno, C., de la Cruz, A., Oliveras, A., Kharche, S. R., Guizy, M., Comes, N., et al. (2015). Marine n-3 PUFAs modulate IKs gating, channel expression, and location in membrane microdomains. Cardiovasc. Res. 105, 223–232. doi: 10.1093/cvr/cvu250
Neyroud, N., Tesson, F., Denjoy, I., Leibovici, M., Donger, C., Barhanin, J., et al. (1997). A novel mutation in the potassium channel gene KVLQT1 causes the Jervell and Lange-Nielsen cardioauditory syndrome. Nat. Genet. 15, 186–189. doi: 10.1038/ng0297-186
Panaghie, G., and Abbott, G. W. (2007). The role of S4 charges in voltage-dependent and voltage-independent KCNQ1 potassium channel complexes. J. Gen. Physiol. 129, 121–133. doi: 10.1085/jgp.200609612
Sanguinetti, M. C., Curran, M. E., Zou, A., Shen, J., Spector, P. S., Atkinson, D. L., et al. (1996). Coassembly of K(V)LQT1 and minK (IsK) proteins to form cardiac I(Ks) potassium channel. Nature 384, 80–83. doi: 10.1038/384080a0
Schwake, M., Athanasiadu, D., Beimgraben, C., Blanz, J., Beck, C., Jentsch, T. J., et al. (2006). Structural determinants of M-type KCNQ (Kv7) K+ channel assembly. J. Neurosci. 26, 3757–3766. doi: 10.1523/jneurosci.5017-05.2006
Schwake, M., Jentsch, T. J., and Friedrich, T. (2003). A carboxy-terminal domain determines the subunit specificity of KCNQ K+ channel assembly. EMBO Rep. 4, 76–81. doi: 10.1038/sj.embor.embor715
Shimizu, T., and Wolfe, L. S. (1990). Arachidonic acid cascade and signal transduction. J. Neurochem. 55, 1–15. doi: 10.1111/j.1471-4159.1990.tb08813.x
Skarsfeldt, M. A., Liin, S. I., Larsson, H. P., and Bentzen, B. H. (2020). Polyunsaturated fatty acid-derived IKs channel activators shorten the QT interval ex-vivo and in-vivo. Acta Physiol. (Oxf) e13471. doi: 10.1111/apha.13471
Sun, J., and MacKinnon, R. (2017). Cryo-EM Structure of a KCNQ1/CaM complex reveals insights into congenital long QT syndrome. Cell 169, 1042–1050.
Sun, J., and MacKinnon, R. (2020). Structural basis of human KCNQ1 modulation and gating. Cell 180, 340–347.
Sun, X., Zhou, D., Zhang, P., Moczydlowski, E. G., and Haddad, G. G. (2007). Beta-subunit-dependent modulation of hSlo BK current by arachidonic acid. J. Neurophysiol. 97, 62–69. doi: 10.1152/jn.00700.2006
Taylor, K. C., Kang, P. W., Hou, P., Yang, N. D., Kuenze, G., Smith, J. A., et al. (2020). Structure and physiological function of the human KCNQ1 channel voltage sensor intermediate state. Elife 9:e53901.
Taylor, K. C., and Sanders, C. R. (2017). Regulation of KCNQ/Kv7 family voltage-gated K(+) channels by lipids. Biochim. Biophys. Acta Biomembr. 1859, 586–597. doi: 10.1016/j.bbamem.2016.10.023
Tian, Y., Aursnes, M., Hansen, T. V., Tungen, J. E., Galpin, J. D., Leisle, L., et al. (2016). Atomic determinants of BK channel activation by polyunsaturated fatty acids. Proc. Natl. Acad. Sci. U.S.A. 113, 13905–13910. doi: 10.1073/pnas.1615562113
Valenzuela, C. (2016). M-channels and n-3 polyunsaturated fatty acids: role in pain and epilepsy. Acta Physiol. (Oxf) 218, 7–9.
Villarroel, A. (1993). Suppression of neuronal potassium A-current by arachidonic acid. FEBS Lett. 335, 184–188. doi: 10.1016/0014-5793(93)80726-b
Villarroel, A. (1994). On the role of arachidonic acid in M-current modulation by muscarine in bullfrog sympathetic neurons. J. Neurosci. 14(11 Pt 2), 7053–7066. doi: 10.1523/jneurosci.14-11-07053.1994
Wang, H. S., Pan, Z., Shi, W., Brown, B. S., Wymore, R. S., Cohen, I. S., et al. (1998). KCNQ2 and KCNQ3 potassium channel subunits: molecular correlates of the M-channel. Science 282, 1890–1893. doi: 10.1126/science.282.5395.1890
Xu, Y., Wang, Y., Meng, X. Y., Zhang, M., Jiang, M., Cui, M., et al. (2013). Building KCNQ1/KCNE1 channel models and probing their interactions by molecular-dynamics simulations. Biophys. J. 105, 2461–2473. doi: 10.1016/j.bpj.2013.09.058
Yazdi, S., Stein, M., Elinder, F., Andersson, M., and Lindahl, E. (2016). The molecular basis of polyunsaturated fatty acid interactions with the shaker voltage-gated potassium channel. PLoS Comput. Biol. 12:e1004704. doi: 10.1371/journal.pcbi.1004704
Yu, S. P. (1995). Roles of arachidonic acid, lipoxygenases and phosphatases in calcium-dependent modulation of M-current in bullfrog sympathetic neurons. J. Physiol. 487, 797–811. doi: 10.1113/jphysiol.1995.sp020919
Zaydman, M. A., and Cui, J. (2014). PIP2 regulation of KCNQ channels: biophysical and molecular mechanisms for lipid modulation of voltage-dependent gating. Front. Physiol. 5:195. doi: 10.3389/fphys.2014.00195
Keywords: docosahexaenoic acid, KCNE, KCNQ, KV7, lipid, polyunsaturated fatty acid, voltage-gated potassium channel
Citation: Larsson JE, Frampton DJA and Liin SI (2020) Polyunsaturated Fatty Acids as Modulators of KV7 Channels. Front. Physiol. 11:641. doi: 10.3389/fphys.2020.00641
Received: 18 March 2020; Accepted: 20 May 2020;
Published: 11 June 2020.
Edited by:
Thomas Andrew Jepps, University of Copenhagen, DenmarkReviewed by:
Jennifer Beth Stott, St George’s, University of London, United KingdomXavier Gasull, University of Barcelona, Spain
Anna Wiktorowska-Owczarek, Medical University of Lodz, Poland
Copyright © 2020 Larsson, Frampton and Liin. This is an open-access article distributed under the terms of the Creative Commons Attribution License (CC BY). The use, distribution or reproduction in other forums is permitted, provided the original author(s) and the copyright owner(s) are credited and that the original publication in this journal is cited, in accordance with accepted academic practice. No use, distribution or reproduction is permitted which does not comply with these terms.
*Correspondence: Sara I. Liin, c2FyYS5saWluQGxpdS5zZQ==
†ORCID: Sara I. Liin, orcid.org/0000-0001-8493-0114
‡These authors share first authorship