- 1School of Biomedical Sciences, Faculty of Medicine, The University of Queensland, St Lucia, QLD, Australia
- 2Department of Biochemistry and Molecular Biology, Monash Biomedicine Discovery Institute, Monash University, Clayton, VIC, Australia
The human genome contains ∼29 bitter taste receptors (T2Rs), which are responsible for detecting thousands of bitter ligands, including toxic and aversive compounds. This sentinel function varies between individuals and is underpinned by naturally occurring T2R polymorphisms, which have also been associated with disease. Recent studies have reported the expression of T2Rs and their downstream signaling components within non-gustatory tissues, including the heart. Though the precise role of T2Rs in the heart remains unclear, evidence points toward a role in cardiac contractility and overall vascular tone. In this review, we summarize the extra-oral expression of T2Rs, focusing on evidence for expression in heart; we speculate on the range of potential ligands that may activate them; we define the possible signaling pathways they activate; and we argue that their discovery in heart predicts an, as yet, unappreciated cardiac physiology.
Extra-Oral Expression of Bitter T2Rs
TAS2R/T2Rs (gene and protein) were first discovered within type II taste receptor cells in the tongue and act as sentinels in protecting against the ingestion of potentially toxic substances (Chandrashekar et al., 2000; Lu et al., 2017). Since these pioneering studies, T2R expression has been reported in a multitude of extra-oral tissues, including the gut, lungs, brain, and heart (Shah et al., 2009; Foster et al., 2013; Garcia-Esparcia et al., 2013), but their complete function(s) in physiology and pathophysiology remain to be defined. In Table 1, we have summarized the location, expression profile and proposed function for the T2R family across a range of human tissues and cells. In regard to function, we would offer a note of caution that a number of studies (listed in Table 1) have proposed functions based on stimulation with various bitter compounds in the micromolar to millimolar range where the selectivity and specificity toward T2Rs may reasonably be questioned. Despite this, the expression of T2Rs within the cardiovascular system, particularly the heart and vasculature, has gained significant interest in recent years. Following our initial discovery of TAS2Rs within the heart (Foster et al., 2013), a number of subsequent studies have focused on the vasculature (Lund et al., 2013; Manson et al., 2014; Upadhyaya et al., 2014; Chen et al., 2017). An unambiguous definition of their function has, however, lagged behind the capacity to demonstrate their expression.
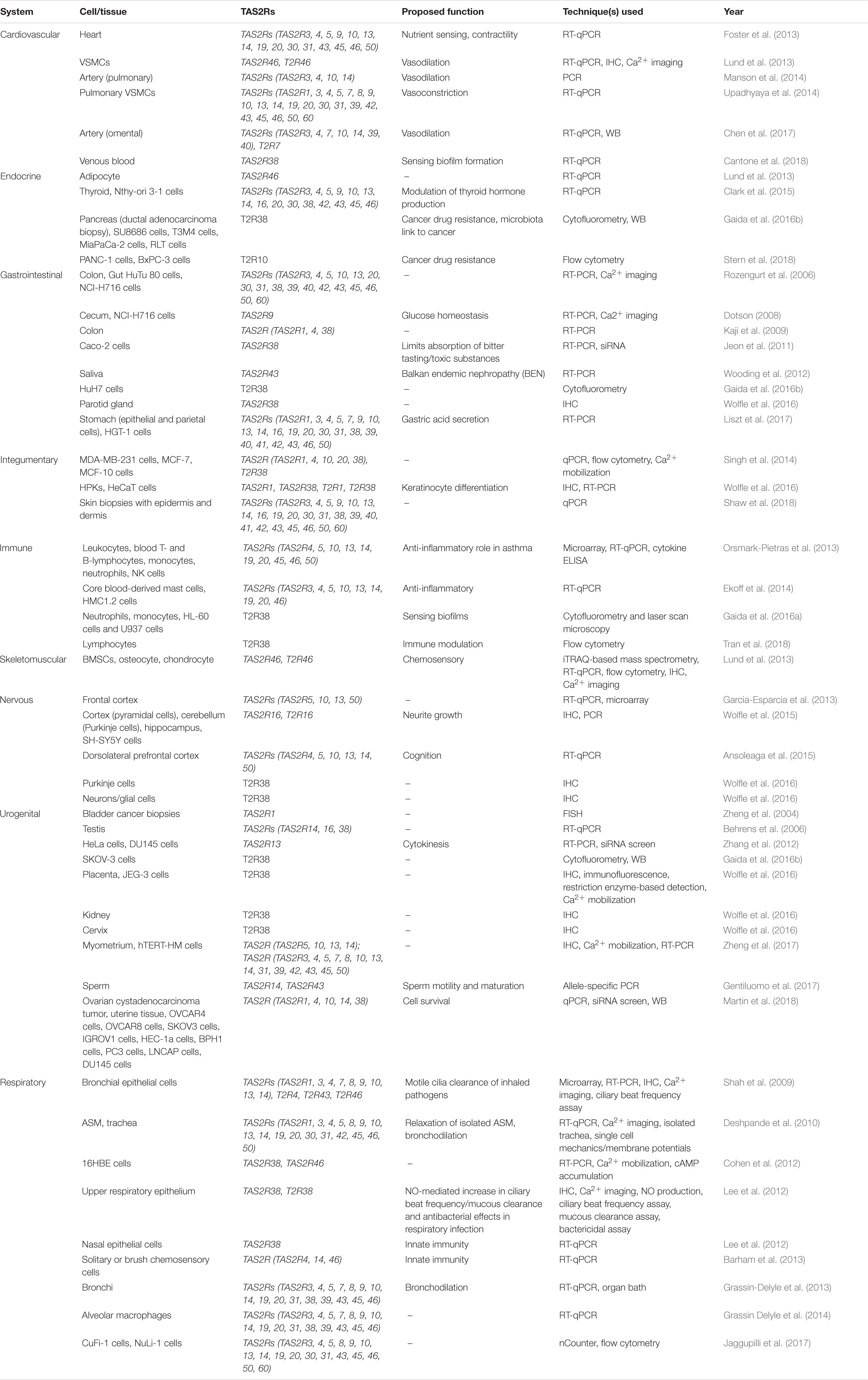
Table 1. Distribution, expression profile, proposed function, and technique used for the detection of extra-oral TAS2R/T2R expression.
The expression of TAS2Rs in different tissues and cell lines has been examined using RT-PCR, qPCR, microarray techniques as well as RNAseq (Flegel et al., 2013). Most recently, Jaggupilli et al. (2017) used nCounter gene expression analysis to characterize the expression of the 29 human TAS2Rs in a variety of cell lines (Table 1). Their results showed that TAS2R14 and TAS2R20 were highly expressed; TAS2R3, −4, −5, −10, −13, −19, and −50 were moderately expressed; TAS2R8, −9, −21 and −60 had low level of expression; and TAS2R7, −16, −38, −39, −40, −41, and −42 were barely detectable. The nCounter technique relies on hybridization of complementary probes (spanning 100 nucleotide bases) for each gene, and hence, TAS2R30, −31, −43, −45, and −46 could not be accurately discerned from one another, as they share >92% homology. Nevertheless, this data clearly shows that some T2Rs are broadly and differentially expressed, whereas others are more restricted in their tissue distribution.
Model Systems for Expressing T2Rs and Defining Their Function
In attempting to define the function of, and to identify ligands for, the T2Rs, researchers have established heterologous expression systems in human cells (e.g., HEK293 or HEK293T) (Meyerhof et al., 2010). However, the use of these cells for understanding the underlying mechanisms and signaling pathways within cardiovascular tissues/cells has obvious limitations. Firstly, due to the insufficient cell surface targeting of T2Rs in heterologous cells (Chandrashekar et al., 2000), chimeric T2Rs encompassing the amino terminus of the rat somatostatin receptor subtype 3 are often used to improve expression and functionality (Bufe et al., 2002; Behrens et al., 2006). Furthermore, a chimeric G protein consisting of the Gα16 and 44 amino acids of gustducin attached to the carboxyl terminus is widely used in calcium mobilization assays (Liu et al., 2003; Ueda et al., 2003). Gα16 has been coined the ‘universal adaptor’ due to its ability to interact with numerous GPCRs and provides a robust readout for receptor activation, including for T2Rs (Ueda et al., 2003). While these artificial heterologous systems have proven useful in identifying ligands for orphan receptors (Meyerhof et al., 2010) and interrogating the structure-function aspects of T2Rs (Brockhoff et al., 2010), the field is now moving toward more relevant cellular models with endogenous receptors and signaling partners (Freund et al., 2018).
Studies using the aforementioned heterologous expression system have demonstrated that the majority of T2Rs form oligomers, both homodimers and heterodimers (Kuhn et al., 2010). However, unlike the situation for umami/sweet taste sensation (requiring dimerization of T1R1/T1R2 and T1R1/T1R3), T2R homodimers did not appear to alter the pharmacology of the receptors, nor do they have obvious influence on protein expression or membrane localization (Kuhn et al., 2010). In contrast, Kim et al. (2016) used immuno-fluorescent microscopy to show that the co-expression of the adrenergic (ADRβ2) receptor with T2R14 resulted in a ∼3-fold increase in cell-surface expression of T2R14. Co-immunoprecipitation and biomolecular fluorescence complementation experiments confirmed that the increase of cell-surface expression was attributed to the formation of T2R14:ADRβ2 heterodimers. These complexes may be particularly important in heart where the actions of adrenergic receptors are well described. Interestingly, co-immunoprecipitation and co-internalization of ADRβ2:M71 OR (mouse 71 olfactory receptor) was observed in response to their specific ligands (Hague et al., 2004). These seminal observations in heterologous systems need to be confirmed and extended with endogenous models to clarify our understanding of how T2Rs function in vivo and to define their potential modulation of (or by) established GPCRs.
Another important issue in considering model expression systems for studying T2Rs is the requirement for appropriate accessory proteins and correct post-translational processing. It is now well-established that chemosensory receptors [e.g., odorant (McClintock et al., 1997) and pheromone (Loconto et al., 2003) receptors] rely on endogenous proteins in order to be targeted to the cell-surface. A study by Behrens et al. (2006) demonstrated that certain members of the receptor-transporting protein (RTP) and receptor expression enhancing protein (REEP) families enhance cell-surface localization and functionality of certain TAS2Rs, likely through protein–protein interactions. Furthermore, it was shown that varying combinations of these proteins are expressed endogenously within tissues (circumvallate papillae and testis) that express TAS2R genes. Interestingly, the human heart differentially expresses REEP 1, 2, 3, 5, and 6 across heart regions (Doll et al., 2017), suggesting that efficient cell-surface TAS2R expression may also be region specific. Nonetheless, these trafficking proteins do not universally promote T2R functionality, for instance T2R14 showed no increase in capacity to mobilize calcium when co-expressed with either RTP or REEP (Behrens et al., 2006). There is accumulating evidence that the degree of T2R membrane insertion is dependent on the specific tissue. As T2Rs are detected in a myriad of tissues, multiple endogenous mechanisms may contribute to their appropriate expression and localization. As for many GPCRs, N-glycosylation of T2Rs is important for cell-surface localization–Reichling et al. (2008) reported that glycosylation of the second extracellular loop is essential for the recruitment (via association with the cellular chaperone calnexin) and insertion of TAS2Rs in the cell membrane; moreover, the function of non-glycosylated TAS2R16 could be rescued when co-expressed with RTP3 and RTP4.
The Cardiac Gpcr Repertoire Includes T2Rs
The human heart expresses over 200 different GPCRs (Wang et al., 2018), some of which are critical for regulating cardiac morphology and function (Capote et al., 2015). Intriguingly, the gene transcripts for more than half of the TAS2R family were detected in both left ventricle and right atria (Foster et al., 2013) ranging in abundance between that observed for two classically important cardiac GPCRs – the angiotensin II type 1 receptor and β1-adrenergic receptor (ADRβ1). It is notable that the expression of TAS2R14 was equivalent to that of ADRβ1 in the left ventricle. These findings are supported by publicly available Illumina Human BodyMap 2.0 project RNA-seq dataset (Flegel et al., 2013), which showed widespread TAS2R expression in human tissues and highest expression of TAS2R14 in heart. It is important, however, to note that T2Rs are not uniformly detected by all techniques, with TAS2R9, TAS2R39, and TAS2R45 not detected in the Illumina RNA-seq data set, but detected by qPCR (Foster et al., 2013). These differences could reflect individual variations, noting the body map is from one patient or the more specific nature of RNA-seq over qPCR. Interestingly, the expression of TAS2Rs are differentially regulated with age in mice (Foster et al., 2013), but not with sex or in heart failure (Foster et al., 2015a). Furthermore, analysis of the publicly available GTEx LDACC and BioGPS Human Cell Type and Tissue Gene Expression Profiles RNA-seq datasets, highlight the expression of GNAT3 (the taste receptor specific G protein, GαGustducin) in a variety of human tissues, including the heart.
We previously investigated the factors contributing to cardiac TAS2R gene expression in silico (Foster et al., 2015a). Similar to rodent Tas2rs, there was no evidence of enrichment for particular transcription factor binding sites in the proximal promoter regions of the human TAS2R genes. However, we observed thatTAS2R14 (the most abundantly expressed) had the strongest evidence of regulatory activity in its promoter region, i.e., active methylation marks overlapping with the DNase I hypersensitivity cluster. On this basis, although we cannot rule out the presence of specific transcription factors that regulate TAS2R gene expression, we reason that the proximal regulatory regions for some, but not all, TAS2R genes might show a basal level of transcriptional activity. This, combined with their multigene cluster expression profiles could facilitate preferential transcription of the specific TAS2Rs (Foster et al., 2015a).
The heart is made up of 2–3 billion cardiomyocytes and yet these cells constitute less than a third of all heart tissue (Tirziu et al., 2010). The remaining, more than two thirds of the heart consists of smooth muscle, fibroblasts, other connective tissue cells, endothelial cells, sinoatrial cells, atrioventricular cells, Purkinje cells, pluripotent cardiac stem cells, mast cells, and other immune system-related cells (Tirziu et al., 2010). We have demonstrated that certain Tas2rs (rodent) were expressed within both cardiomyocytes and fibroblasts, as well as their downstream signaling effectors (Gnat3, Plcβ2, Trpm5) (Foster et al., 2013). These data suggest that specific cells within the heart may express varying populations of TAS2R, similar to that seen in other systems (Table 1). As technology advances, including single cell sequencing and proteomics (Uhlen et al., 2015), the topography of T2Rs within the heart will provide insight into how these receptors function within this system.
Signaling and Function of T2Rs Within the Cardiovascular System
The binding of bitter ligands to T2Rs results in a conformation change in the receptor allowing it to interact with GαGustducin and Gβ1/3γ13 (Huang et al., 1999), which then activate subsequent downstream pathways (Yan et al., 2001). Knockout (KO) studies have provided conclusive evidence supporting these signaling pathways. Mice lacking either PLCβ2 or TRPM5 exhibited diminished or ablated taste responses to bitter compounds (Zhang et al., 2003). Furthermore, GαGustducin KO mice had increased levels of cAMP, compared to the wild-type mice as well as displaying severely impaired responses to the tested compounds (Clapp et al., 2008). As with Gαi-family G proteins, GαGustducin can decrease the levels of cAMP, via the activation of phosphodiesterases, which has been observed in response to two bitter compounds, denatonium and strychnine (Yan et al., 2001). Finally, mice that were genetically modified to express novel human T2Rs demonstrated a strong aversive response to ligands that was not evident in wild-type mice (Mueller et al., 2005).
With the discovery of TAS2R expression in cardiac tissue (Foster et al., 2013), defining the signaling transduction pathway is of particular interest, yet there is limited evidence for the presence of all the classical taste signaling components in heart. The expression of GαGustducin has been shown in human heart tissue, and is particularly enriched in cardiomyocytes (BioGPS Human Cell Type and Tissue Gene Expression Profiles RNA-seq datasets). However, studies have not observed Gγ13 (Huang et al., 1999) or TRPM5 (Demir et al., 2014). TRPM4 is present within human heart tissues (Guinamard et al., 2004; Demir et al., 2014), however, both TRPM4 and TRMP5 are considered necessary for taste signal transduction (Dutta Banik et al., 2018). Hence, alternative signal transduction pathways that could mediate the effects of taste receptors in the cardiovascular system should be considered.
A study by Ueda et al. (2003) demonstrated that T2R16 could couple to a chimeric G protein consisting of the N-terminus of Gα16 and the last 44 amino acids of either GαGustducin, Gαt2or Gαi2. Furthermore, the expression of all three of these Gα subunits have been identified in taste receptor cells, with the frequency of Gαi2 being higher than that of GαGustducin (Ueda et al., 2003). Figure 1 shows a comparison of GαGustducin and Gαi2, highlighting the highly conserved amino acid residues and the region known to interact with TAS2Rs. Substitution of glycine352 for proline in GαGustducin disrupts T2R interaction with GαGustducin, although its coupling to the Gβγ and effector molecules was preserved (Ruiz-Avila et al., 2001). This suggests that the extreme C terminus of both GαGustducin and Gαi2 are capable of, and necessary for T2R:G protein coupling and transduction. Importantly, in human airway smooth muscle (HASM), the reported expression of Gαi2 expression was 100-fold higher than that of GαGustducin and T2R14 was shown to couple to all Gαi proteins, particularly Gαi2 (Kim et al., 2017). The use of pertussis toxin was able to abrogate the T2R mediated relaxation in HASM (Kim et al., 2017), consistent with previous studies, where T2Rs has been shown to couple with inhibitory signaling pathways (Ozeck et al., 2004). The actions of T2R may also include other inhibitory type processes, such as described by Zhang et al. (2013) in airway smooth muscle cells (Lu et al., 2017). Taken together these observations suggest that depending on the level of G protein expression and the strength of the subsequent signal, T2Rs likely couple and signal in a cell/tissue specific manner, which may include (or not) GαGustducin.
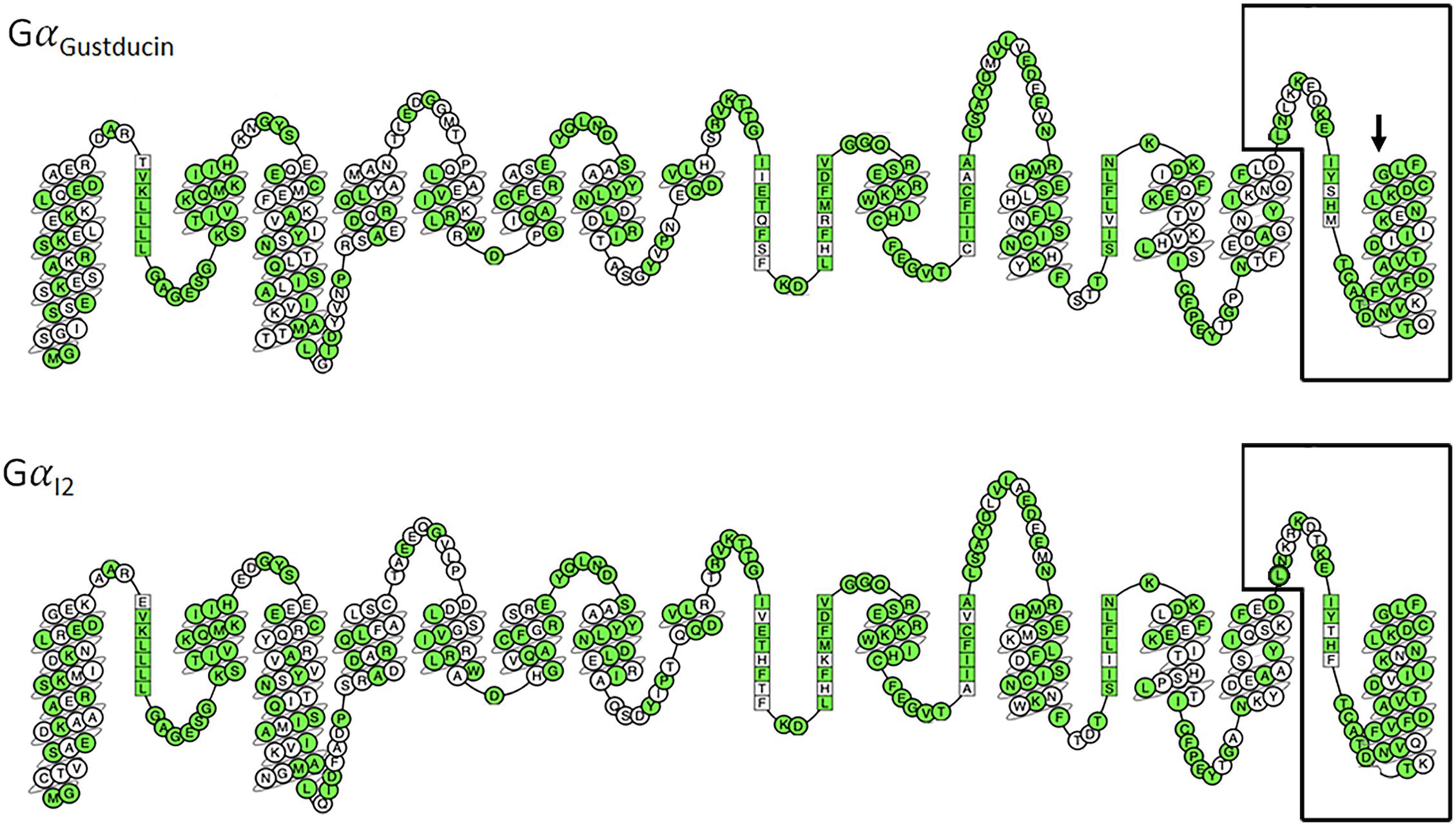
Figure 1. Comparison of 2D snake representations of GαGustducin and Gαi2 [constructed via GPCRdb (Isberg et al., 2016)]. Amino acids are labeled following the Ballesteros–Weinstein numbering system. Conserved amino acids are colored green. The final 44 amino acids are known to be necessary for T2R coupling and signaling (Ueda et al., 2003) and are highlighted by black boxes. Arrow points to the amino acid (Glycine352) in GαGustducin that disrupts T2R coupling when mutated to proline (Ruiz-Avila et al., 2001).
Indeed, T2R signaling within cardiac cells might reasonably reflect those described for the respiratory system and vascular systems (summarized in Figure 2). The heart is known to express specific varying combinations of Gα (including Gαi2), Gβγ and various signaling effector molecules (Doll et al., 2017). In a series of experiments in Langendorff-perfused mouse hearts, we observed dose-dependent negative inotropic effects in response to bitter ligands (Foster et al., 2014). A ∼40% decrease in left ventricular developed pressure and an increase in aortic pressure in response to sodium thiocynate were shown to be Gαi-dependent. Some alterations in cardiovascular physiology were not attributed to G proteins (not blocked by pertussis toxin and gallein), however, it was shown that rodents express GNAT3 (GαGustducin) in their cardiomyocytes (Foster et al., 2013). This further supports the premise that T2Rs can signal through various G proteins. While there is no clear consensus on the precise mechanism, there is an agreement that bitter ligands mediate contractile responses in the vasculature. One study demonstrated a transient drop in blood pressure upon intravenous injection of denatonium benzoate into rats (Lund et al., 2013). Additionally, Manson et al. (2014) attributed the endothelium-independent relaxation of precontracted human pulmonary arteries to the application of bitter ligands for T2Rs (3, 4, 10, and 14). In contrast, denatonium benzoate has been shown to enhance the tone of endothelium-denuded rat aorta rings, which was attributed to specific Tas2r activation (Tas2r40, 108, 126, 135, 137, 143) via GαGustducin (Liu et al., 2020). Whether the actions of T2Rs in cardiomyocytes have a direct effect on the force and strength of contraction of individual myocytes remains to be determined. Equally, there is a possibility that these receptors may be expressed in other cell populations, including the specific cells of the conduction system (SA node, AV node, Purkinje Fibers).
Naturally Occurring Polymorphisms and Disease
GPCRs and their respective ligands have profound homeostatic and regulatory effects on the cardiovascular system. Not surprisingly, mutations and modifications of cardiovascular GPCRs, G proteins and their regulatory proteins are linked to dysfunction and disease (Foster et al., 2015b). T2Rs are one of the most heterogenous and unique families of GPCRs and are now considered as a separate group of receptors (Di Pizio and Niv, 2015). According to the HGNC database, there are 39 genetically diverse and highly polymorphic TAS2R single exon genes that encode for 29 functional T2Rs (and 10 non-coding pseudogenes) in humans (Devillier et al., 2015). This is in contrast to the majority of literature that cite the existence of only 25 functional T2Rs (Meyerhof et al., 2010; Lossow et al., 2016). On average, TAS2R genes contain four single nucleotide polymorphisms (SNPs) of which the vast majority are non-synonyms mutations that encode amino acid substitutions (Kim et al., 2005). Table 2 outlines all of the non-synonymous SNPs present within the population and their penetrance. These TAS2R genes are located on chromosomes 5, 7, and 12 (Adler et al., 2000; Foster et al., 2015a), with dense clustering on chromosomes 7 and 12. The close proximity is thought to underpin the enormous variation and diversification of the T2R repertoire within humans.
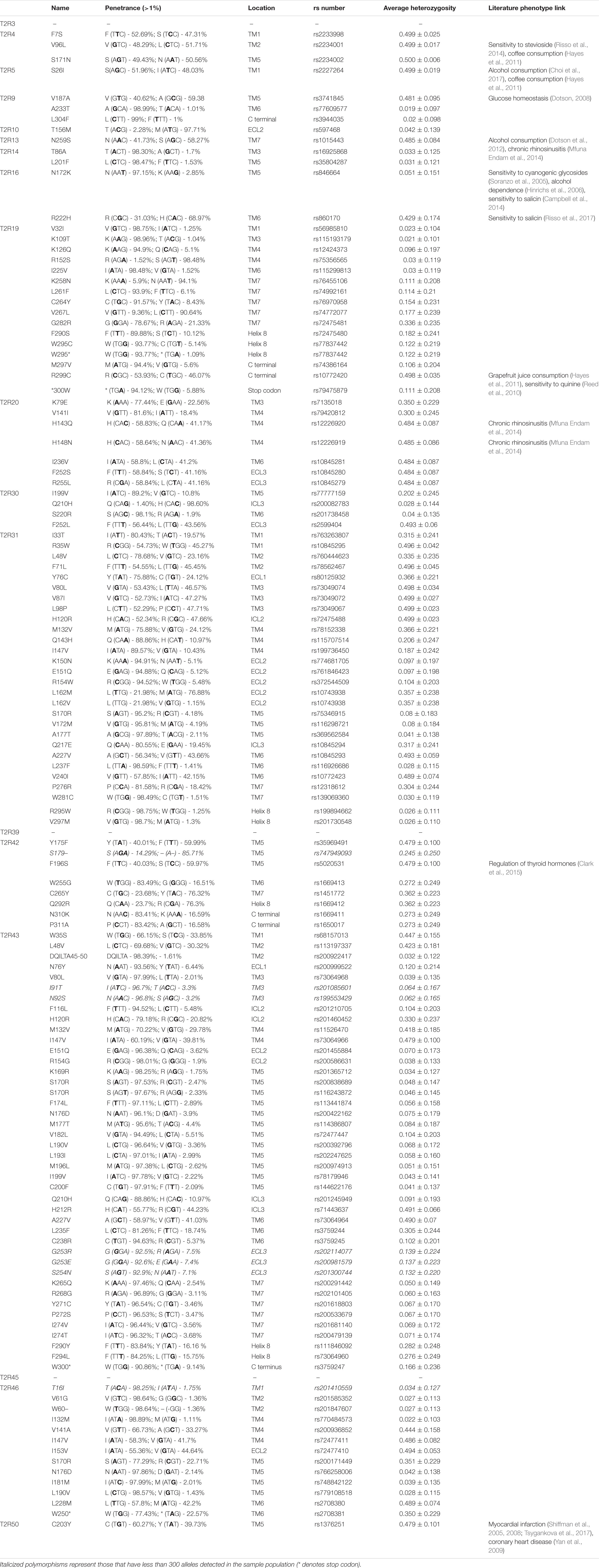
Table 2. List of polymorphisms in human cardiac-expressed TAS2Rs (penetrance > 1% in the population) sourced from UCSC Genome Browser and NCBI SNP databases.
The importance of uncovering the primary function of T2Rs in the heart is supported by the critical role they play within the respiratory system. TAS2R38 is expressed in all aspects of the upper and lower respiratory tracts including sinonasal epithelial cells, bronchial epithelial cells, bronchial smooth muscle, and pulmonary vasculature smooth muscle (Shah et al., 2009; Grassin-Delyle et al., 2013; Upadhyaya et al., 2014; Devillier et al., 2015). Application of phenylthiocarbamide (PTC) or two quorum sensing molecules (C4HSL and C12HSL) secreted by Pseudomonas aeruginosa were shown to increase mucociliary clearance, bronchodilation, and production of bactericidal levels nitric oxide in explanted human tissue samples and primary airway–liquid interface cultures (Lee et al., 2012). This supports the recent finding that T2Rs play a role in innate immunity, as quorum sensing molecules serve to communicate between bacterial populations, allowing them to establish themselves during infection (Lee et al., 2014). Bitter taste receptors, particularly TAS2R38, are a unique and diverse family of GPCRs due to the number of their naturally occurring genetic variants (Kim et al., 2005). Compared to the functional (PAV) haplotype, individuals with the non-functional (AVI) haplotype were shown to be more susceptible to respiratory infections as the receptor was unable to detect the compounds and respond appropriately (Lee et al., 2012). A similar result was seen with regard to oral innate immunity (Gil et al., 2015). TAS2R38 PAV/PAV mRNA was upregulated ∼4.3-fold in response to Streptococcus mutans bacteria (over the unstimulated control) whereas the AVI/AVI was only ∼1.2-fold. Furthermore, the level of hBD-2 (antimicrobial peptide) induced was highest in those with the PAV/PAV genotype (Gil et al., 2015). On this basis, the authors concluded that a person’s T2R38 genotype determines oral innate immunity.
Natural polymorphisms are no longer thought only to account for differences in oral bitter taste perception (Roudnitzky et al., 2016). It is now recognized that these polymorphisms also influence other important aspects of our physiology including alcohol dependence, eating behavior, longevity, glucose homeostasis and regulation of thyroid hormones (Dotson, 2008; Hayes et al., 2011; Campa et al., 2012; Clark et al., 2015). There are 132 naturally occurring non-synonymous polymorphisms for cardiac-expressed T2Rs and it is clear that the majority of these remain uncharacterized (Table 3). One polymorphism that is of particular interest is T2R50-rs1376251, as debate remains in the literature over its potential association with myocardial infarction and coronary heart disease (Shiffman et al., 2008; Tepper et al., 2008; Yan et al., 2009; Koch et al., 2011; Ivanova et al., 2017; Tsygankova et al., 2017). There are also polymorphisms outside of the taste receptor coding region, or those that result in synonymous mutations that have been associated with changes in physiology. Of note, T2R14 rs3741843 has been associated with decreased sperm motility (Gentiluomo et al., 2017). Individuals that were homozygous carriers for the (G) allele, encoding arginine (R – AGG), showed a decreased sperm progressive motility compared to heterozygotes and homozygotes for the (A) allele, which encodes arginine (R – AGA). The authors rationalized using in silico analysis that T2R14 regulates the expression of T2R43. Furthermore, an upstream mutation of TAS2R3 rs11763979 can regulate the expression of WEE2 antisense RNA one (WEE2-AS1), which increases the expression of WEE2 within the testis. WEE2 is a protein tyrosine kinase involved in the regulation of cell cycle progression (Nakanishi et al., 2000). Overexpression of WEE2 in the testis was hypothesized to increase the number of abnormal sperm cells (Gentiluomo et al., 2017). Despite recent progress, it is unclear the full extent to which polymorphisms can influence T2R physiology, although it is clear investigation into their effects is warranted.
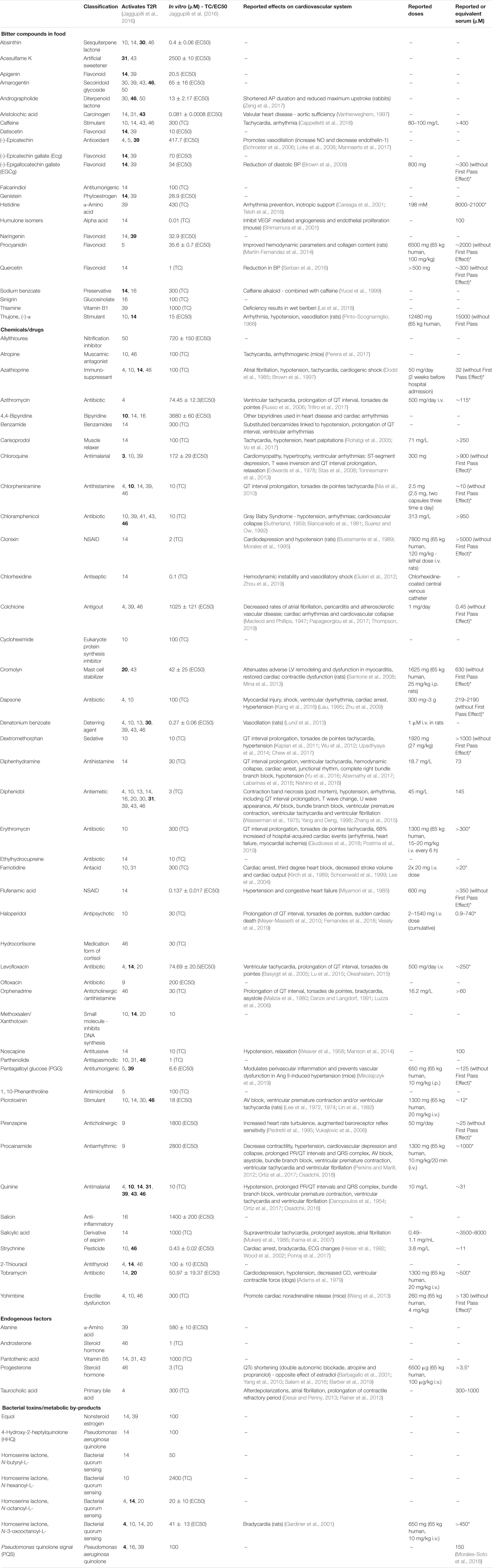
Table 3. List of ligands known to activate cardiac specific T2Rs and their classification, number of T2Rs activated [bold indicates the receptor corresponding to the lowest threshold (TC) or effective concentration (EC50) in vitro concentration], reported effects in the cardiovascular system and corresponding dose/serum (* = based on 5.5 L of blood in human body, or without First Pass Effect of liver).
Potential Cardiovascular T2R Ligands
T2Rs are unique as they lack most of the conserved motifs of the class A GPCR family (Lagerstrom and Schioth, 2008). The intracellular loops – regions necessary for signal transduction and feedback modulation (Moreira, 2014), were shown to be more conserved across T2Rs than the extracellular loops that are generally implicated in receptor binding (Meyerhof, 2005). Using T2R14 as an example, Nowak et al. (2018) demonstrated that in vitro mutagenesis of 19 receptor mutants (all within the binding pocket) retained the ability to bind at least one of the 7 tested agonists while some improved signaling compared to the wild type. These results are consistent with previous literature that ligands bind within the transmembrane and extracellular domain regions (Brockhoff et al., 2010; Upadhyaya et al., 2015). Interestingly, of the highly expressed cardiac T2Rs, T2R10, T2R14, and T2R46 were shown to bind a wide array of ligands, which is considered disproportional in comparison to the others (Meyerhof et al., 2010). Over 75% of the list of ligands in Table 3 were shown to activate these three broadly tuned T2Rs.
Universally, researchers have used chemicals that ‘taste bitter’ to test for potential ligands. However, if heart tissue expresses over half of the T2Rs family, a major question arises - what is the source of ligands for these T2Rs within the cardiovascular system? We would argue there are four major sources: (1) bitter compounds in food, (2) endogenously produced factors, (3) bacterial metabolic by-products and toxins and (4) chemicals/drugs (outlined in Table 3).
The post-prandial concentration of bitter compounds in the blood increases. One perhaps common example of this is caffeine, which reportedly modulates calcium signaling via interaction with the ryanodine receptor (Kong et al., 2008). Interestingly, caffeine also activates T2R10, -14, and -46 (Meyerhof et al., 2010; Cappelletti et al., 2018) at concentrations that occur in blood post-prandially and which are equivalent to levels that modulate the ryanodine receptor (Kong et al., 2008). In the gut, caffeine activation of T2R has been linked to gastric secretion (Liszt et al., 2017). Caffeine may also act as a stimulate for the central nervous system via the antagonism of adenosine receptors (Fisone et al., 2004). Hence in considering the homeostatic consequence of bitter compounds (such as caffeine) one must also accept that at high concentrations they are interacting with multiple receptor systems. We would anticipate that many bitter compounds in food would have actions on both T2Rs and other targets.
Another interesting possibility is that the body produces endogenous factors that could activate T2Rs. Currently, alanine, pantothenic acid (vitamin B5), steroids (androsterone and progesterone) and taurocholic acid (primary bile acid) have all been identified as ligands for specific receptors (Ji et al., 2014; Lossow et al., 2016). Potentially, the cardiotonic steroids may be ligands for cardiac-expressed T2Rs, although ouabain has already been shown not to be an agonist in vitro (Meyerhof et al., 2010), despite being able to augment calcium transients in arterial smooth muscle (Arnon et al., 2000). The other members of this family could also be investigated as potential ligands for cardiac T2Rs. Whether hormones/factors produced by other tissues, or indeed paracrine factors released from cardiac cells, can bind and activate cardiac T2Rs remains to be determined, but is an area of intense interest.
A more provocative idea is that colonizing bacteria, in complex organisms, could produce bitter compounds, including metabolic by-products and other signaling molecules that alter our physiology via T2Rs. A recent study showed commensal bacteria are enable to synthesize GPCR ligands that mimic human signaling molecules (Cohen et al., 2017). Broad screening for bacterial metabolites that activate GPCRs (Chen et al., 2019; Colosimo et al., 2019) have identified numerous candidates, but unfortunately these screens have not included the taste receptors. Interestingly, an olfactory receptor (Olfr78) has been reported to respond to short chain fatty acids produced by gut bacteria (Pluznick et al., 2013). Olfr78 KO mice had elevated blood pressure when treated with antibiotics. As for the T2Rs, T2R38 although its expression is low in the heart, was shown to be broadly tuned for seven bacterial metabolites (Verbeurgt et al., 2017). It is also worth noting that during infections bacterial toxins could be ‘bitter’ and interact with T2Rs once they reached a certain concentration in the blood. One example is quorum sensing molecules - when they reach a certain concentration, bacteria produce a biofilm in order to evade and survive the host immune defense system (Davies et al., 1998). Therefore, it is plausible that T2Rs may alter cardiovascular physiology in response to systemic infections such as sepsis where dramatic cardiovascular changes are observed, e.g., decreased myocardial contractility, vasodilation, endothelial injury and increased heart rate (Singer et al., 2016).
Finally, it is important to address the possibility that off-target activation of T2Rs may play a role beyond normal physiology and mediate unexpected responses to therapeutic drugs, many of which are bitter. Indeed, the possibility that T2Rs act as the mediators of off-target drug effects due to the prevalence of their expression throughout the body has been discussed previously (Clark et al., 2012). There are numerous drugs/chemicals that have specific, detrimental cardiovascular effects and, moreover, these chemicals have been shown to activate specific T2Rs at concentrations to those that elicit these adverse effects.
Future Directions
The continuous, proper functioning of the heart is fundamental to life. The discovery of T2Rs expressed in cardiac cells predicts important (but yet to be appreciated) roles in heart physiology, as well as its response to external challenges (e.g., diet, metabolic changes, infections, and drugs). Research and knowledge regarding the physiology of T2Rs within the human heart is challenging, primarily due to the constraints of readily acquiring suitable human heart tissue samples. Furthermore, the lack of homology between rodent Tas2rs and human T2Rs (Foster et al., 2013), limits the utility of gene modified animal models to directly inform human physiology. Additionally, the 29 T2Rs (and their many variants) have been historically difficult to heterologously express on the cell membrane of model cells, and this has impeded further investigation of their signaling properties.
It is important to note, that researchers have ectopically expressed human T2Rs in mice and this has provided strong confirmation that a given ligand (tastants) can activate a specific human T2R (Mueller et al., 2005). Perhaps future experiments might extend this approach to develop transgenic mice expressing human T2Rs in a cell-specific context. Stimulation of these receptors with ligands that selectively bind and activate only human T2Rs could provide important insights into the physiological role(s) of T2Rs in human tissues.
Another critical objective will be to develop appropriate cardiac models that express endogenous receptors and recapitulate cardiac physiology. One major advance in cardiovascular research has been the development of induced pluripotent stem cell-derived human cardiomyocytes (Hudson et al., 2012; Soong et al., 2012) and human cardiac organoids (Nugraha et al., 2019). These models will offer the unique opportunity to modulate T2R expression in cardiomyocytes and to thereby investigate bitter ligand-driven changes in cardiac gene transcription, as well as to define alterations in cardiac contractility and function.
Finally, the ultimate goal will be to attribute T2R-mediated expression, activation and signaling to definitive changes in human cardiovascular function in vivo. In order for this to succeed, the following challenges need to be resolved - the promiscuity of bitter receptor–ligand interactions, the elucidation of tissue-specific T2R signaling, as well as the lack of definitive research tools (e.g., selective antibodies to T2Rs and specific receptor antagonists). We anticipate that studies focused on examining the functionality (or lack thereof) for the various highly penetrant, cardiac-expressed T2R polymorphisms may provide the means for unambiguously attributing T2R activation to a specific physiological outcome. Analogous to the advances made with non-functional T2R38 variants (T2R38AVI) in the lung, we predict that non-functional, cardiac-expressed T2Rs can be identified and these will prove to be critical in providing the necessary controls for investigating explanted cardiac tissues.
Author Contributions
CB, SF, and WT designed the scope and structure of the review. CB collated data. CB, SF, and WT wrote and edited the final manuscript. CB produced the figures and tables in consultation with SF and WT.
Conflict of Interest
The authors declare that the research was conducted in the absence of any commercial or financial relationships that could be construed as a potential conflict of interest.
References
Abernathy, A., Alsina, L., Greer, J., and Egerman, R. (2017). Transient fetal tachycardia after intravenous diphenhydramine administration. Obstet. Gynecol. 130, 374–376. doi: 10.1097/AOG.0000000000002147
Adams, H. R., Parker, J. L., and Mathew, B. P. (1979). Cardiovascular manifestations of acute antibiotic toxicity during E coli endotoxin shock in anesthetized dogs. Circ. Shock 6, 391–404.
Adler, E., Hoon, M. A., Mueller, K. L., Chandrashekar, J., Ryba, N. J., and Zuker, C. S. (2000). A novel family of mammalian taste receptors. Cell 100, 693–702. doi: 10.1016/s0092-8674(00)80705-9
Ansoleaga, B., Garcia-Esparcia, P., Pinacho, R., Haro, J. M., Ramos, B., and Ferrer, I. (2015). Decrease in olfactory and taste receptor expression in the dorsolateral prefrontal cortex in chronic schizophrenia. J. Psychiatr. Res. 60, 109–116. doi: 10.1016/j.jpsychires.2014.09.012
Arnon, A., Hamlyn, J. M., and Blaustein, M. P. (2000). Ouabain augments Ca(2+) transients in arterial smooth muscle without raising cytosolic Na(+). Am. J. Physiol. Heart Circ. Physiol. 279, H679–H691. doi: 10.1152/ajpheart.2000.279.2.H679
Barbagallo, M., Dominguez, L. J., Licata, G., Shan, J., Bing, L., Karpinski, E., et al. (2001). Vascular effects of progesterone : role of cellular calcium regulation. Hypertension 37, 142–147. doi: 10.1161/01.hyp.37.1.142
Barber, M., Nguyen, L. S., Wassermann, J., Spano, J. P., Funck-Brentano, C., and Salem, J. E. (2019). Cardiac arrhythmia considerations of hormone cancer therapies. Cardiovasc. Res. 115, 878–894. doi: 10.1093/cvr/cvz020
Barham, H. P., Cooper, S. E., Anderson, C. B., Tizzano, M., Kingdom, T. T., Finger, T. E., et al. (2013). Solitary chemosensory cells and bitter taste receptor signaling in human sinonasal mucosa. Int. Forum. Allergy Rhinol. 3, 450–457. doi: 10.1002/alr.21149
Basyigit, I., Kahraman, G., Ilgazli, A., Yildiz, F., and Boyaci, H. (2005). The effects of levofloxacin on ECG parameters and late potentials. Am. .J Ther. 12, 407–410. doi: 10.1097/01.mjt.0000127358.38755.c5
Behrens, M., Bartelt, J., Reichling, C., Winnig, M., Kuhn, C., and Meyerhof, W. (2006). Members of RTP and REEP gene families influence functional bitter taste receptor expression. J. Biol. Chem. 281, 20650–20659. doi: 10.1074/jbc.M513637200
Biancaniello, T., Meyer, R. A., and Kaplan, S. (1981). Chloramphenicol and cardiotoxicity. J. Pediatr. 98, 828–830.
Brockhoff, A., Behrens, M., Niv, M. Y., and Meyerhof, W. (2010). Structural requirements of bitter taste receptor activation. Proc. Natl. Acad. Sci. U.S.A. 107, 11110–11115. doi: 10.1021/jf403387p
Brown, A. L., Lane, J., Coverly, J., Stocks, J., Jackson, S., Stephen, A., et al. (2009). Effects of dietary supplementation with the green tea polyphenol epigallocatechin-3-gallate on insulin resistance and associated metabolic risk factors: randomized controlled trial. Br. J. Nutr. 101, 886–894. doi: 10.1017/S0007114508047727
Brown, G., Boldt, C., Webb, J. G., and Halperin, L. (1997). Azathioprine-induced multisystem organ failure and cardiogenic shock. Pharmacotherapy 17, 815–818.
Bufe, B., Hofmann, T., Krautwurst, D., Raguse, J.-D., and Meyerhof, W. (2002). The human TAS2R16 receptor mediates bitter taste in response to β-glucopyranosides. Nat. Genet. 32, 397–401. doi: 10.1038/ng1014
Bustamante, D., Morales, M., Pelissier, T., Saavedra, H., Miranda, H. F., and Paeile, C. (1989). Experimental cardio-depressant effects of clonixin. Gen. Pharmacol. 20, 605–608. doi: 10.1016/0306-3623(89)90094-3
Campa, D., De Rango, F., Carrai, M., Crocco, P., Montesanto, A., Canzian, F., et al. (2012). Bitter taste receptor polymorphisms and human aging. PLoS One 7:e45232. doi: 10.1371/journal.pone.0045232
Campbell, M. C., Ranciaro, A., Zinshteyn, D., Rawlings-Goss, R., Hirbo, J., Thompson, S., et al. (2014). Origin and differential selection of allelic variation at TAS2R16 associated with salicin bitter taste sensitivity in Africa. Mol. Biol. Evol. 31, 288–302. doi: 10.1093/molbev/mst211
Cantone, E., Negri, R., Roscetto, E., Grassia, R., Catania, M. R., Capasso, P., et al. (2018). In vivo biofilm formation, gram-negative infections and TAS2R38 polymorphisms in CRSw NP Patients. Laryngoscope 128, E339–E345. doi: 10.1002/lary.27175
Capote, L. A., Mendez Perez, R., and Lymperopoulos, A. (2015). GPCR signaling and cardiac function. Eur. J. Pharmacol. 763, 143–148.
Cappelletti, S., Piacentino, D., Fineschi, V., Frati, P., Cipolloni, L., and Aromatario, M. (2018). Caffeine-related deaths: manner of deaths and categories at risk. Nutrients 10:611. doi: 10.3390/nu10050611
Careaga, G., Salazar, D., Tellez, S., Sanchez, O., Borrayo, G., and Arguero, R. (2001). Clinical impact of histidine-ketoglutarate-tryptophan (HTK) cardioplegic solution on the perioperative period in open heart surgery patients. Arch. Med. Res. 32, 296–299. doi: 10.1016/s0188-4409(01)00296-x
Chandrashekar, J., Mueller, K. L., Hoon, M. A., Adler, E., Feng, L., Guo, W., et al. (2000). T2Rs function as bitter taste receptors. Cell 100, 703–711. doi: 10.1016/s0092-8674(00)80706-0
Chen, J. G., Ping, N. N., Liang, D., Li, M. Y., Mi, Y. N., Li, S., et al. (2017). The expression of bitter taste receptors in mesenteric, cerebral and omental arteries. Life Sci. 170, 16–24. doi: 10.1016/j.lfs.2016.11.010
Chen, H., Nwe, P. K., Yang, Y., Rosen, C. E., Bielecka, A. A., Kuchroo, M., et al. (2019). A forward chemical genetic screen reveals gut microbiota metabolites that modulate host physiology. Cell 177, 1217.e18–1231.e18. doi: 10.1016/j.cell.2019.03.036
Chew, D. S., Rezazadeh, S., and Miller, R. J. (2017). Recurrent syncope in the emergency department: a lethal cause not for the faint hearted. JAMA Intern. Med. 177, 874–876. doi: 10.1001/jamainternmed.2017.0580
Choi, J. H., Lee, J., Yang, S., and Kim, J. (2017). Genetic variations in taste perception modify alcohol drinking behavior in Koreans. Appetite 113, 178–186. doi: 10.1016/j.appet.2017.02.022
Clapp, T. R., Trubey, K. R., Vandenbeuch, A., Stone, L. M., Margolskee, R. F., Chaudhari, N., et al. (2008). Tonic activity of Galpha-gustducin regulates taste cell responsivity. FEBS Lett. 582, 3783–3787. doi: 10.1016/j.febslet.2008.10.007
Clark, A. A., Dotson, C. D., Elson, A. E., Voigt, A., Boehm, U., Meyerhof, W., et al. (2015). TAS2R bitter taste receptors regulate thyroid function. Faseb J. 29, 164–172. doi: 10.1096/fj.14-262246
Clark, A. A., Liggett, S. B., and Munger, S. D. (2012). Extraoral bitter taste receptors as mediators of off-target drug effects. FASEB J. 26, 4827–4831. doi: 10.1096/fj.12-215087
Cohen, L. J., Esterhazy, D., Kim, S.-H., Lemetre, C., Aguilar, R. R., Gordon, E. A., et al. (2017). Commensal bacteria make GPCR ligands that mimic human signalling molecules. Nature 549, 48–53. doi: 10.1038/nature25997
Cohen, S. P., Buckley, B. K., Kosloff, M., Garland, A. L., Bosch, D. E., Cheng, G., et al. (2012). Regulator of G-protein signaling-21 (RGS21) is an inhibitor of bitter gustatory signaling found in lingual and airway epithelia. J. Biol. Chem. 287, 41706–41719. doi: 10.1074/jbc.M112.423806
Colosimo, D. A., Kohn, J. A., Luo, P. M., Piscotta, F. J., Han, S. M., Pickard, A. J., et al. (2019). Mapping interactions of microbial metabolites with human g-protein-coupled receptors. Cell Host Microbe 26, 273.e7–282.e7. doi: 10.1016/j.chom.2019.07.002
Danopoulos, E., Angelopoulos, B., Moulopoulos, S. D., and Romanos, A. (1954). [Electrocardiographic studies on cardiotoxic effect of quinine in dogs]. Z. Kreislaufforsch 43, 856–861.
Danze, L. K., and Langdorf, M. I. (1991). Reversal of orphenadrine-induced ventricular tachycardia with physostigmine. J. Emerg. Med. 9, 453–457. doi: 10.1016/0736-4679(91)90217-4
Davies, D. G., Parsek, M. R., Pearson, J. P., Iglewski, B. H., Costerton, J. W., and Greenberg, E. P. (1998). The involvement of cell-to-cell signals in the development of a bacterial biofilm. Science 280, 295–298. doi: 10.1126/science.280.5361.295
Demir, T., Yumrutas, O., Cengiz, B., Demiryurek, S., Unverdi, H., Kaplan, D. S., et al. (2014). Evaluation of TRPM (transient receptor potential melastatin) genes expressions in myocardial ischemia and reperfusion. Mol. Biol. Rep. 41, 2845–2849. doi: 10.1007/s11033-014-3139-0
Desai, M. S., and Penny, D. J. (2013). Bile acids induce arrhythmias: old metabolite, new tricks. Heart 99, 1629–1630. doi: 10.1136/heartjnl-2013-304546
Deshpande, D. A., Wang, W. C. H., Mcilmoyle, E. L., Robinett, K. S., Schillinger, R. M., An, S. S., et al. (2010). Bitter taste receptors on airway smooth muscle bronchodilate by a localized calcium flux and reverse obstruction. Nat. Med. 16, 1299–1304. doi: 10.1038/nm.2237
Devillier, P., Naline, E., and Grassin-Delyle, S. (2015). The pharmacology of bitter taste receptors and their role in human airways. Pharmacol. Ther. 155, 11–21. doi: 10.1016/j.pharmthera.2015.08.001
Di Pizio, A., and Niv, M. Y. (2015). Promiscuity and selectivity of bitter molecules and their receptors. Bioorg. Med. Chem. 23, 4082–4091. doi: 10.1016/j.bmc.2015.04.025
Dodd, H. J., Tatnall, F. M., and Sarkany, I. (1985). Fast atrial fibrillation induced by treatment of psoriasis with azathioprine. Br. Med. J. 291:706. doi: 10.1136/bmj.291.6497.706
Doll, S., Dressen, M., Geyer, P. E., Itzhak, D. N., Braun, C., Doppler, S. A., et al. (2017). Region and cell-type resolved quantitative proteomic map of the human heart. Nat. Commun. 8:1469. doi: 10.1038/s41467-017-01747-2
Dotson, C. D., Wallace, M. R., Bartoshuk, L. M., and Logan, H. L. (2012). Variation in the gene TAS2R13 is associated with differences in alcohol consumption in patients with head and neck cancer. Chem. Sen. 37, 737–744. doi: 10.1093/chemse/bjs063
Dotson, C. D. C. D. (2008). Bitter taste receptors influence glucose homeostasis. PloS One 3:e3974. doi: 10.1371/journal.pone.0003974
Dutta Banik, D., Martin, L. E., Freichel, M., Torregrossa, A. M., and Medler, K. F. (2018). TRPM4 and TRPM5 are both required for normal signaling in taste receptor cells. Proc. Natl. Acad. Sci. U.S.A. 115, E772–E781. doi: 10.1073/pnas.1718802115
Edwards, A. C., Meredith, T. J., and Sowton, E. (1978). Complete heart block due to chronic chloroquine toxicity managed with permanent pacemaker. Br. Med. J. 1, 1109–1110. doi: 10.1136/bmj.1.6120.1109
Ekoff, M., Choi, J.-H., James, A., Dahlén, B., Nilsson, G., and Dahlén, S.-E. (2014). Bitter taste receptor (TAS2R) agonists inhibit IgE-dependent mast cell activation. J. Allergy Cli. Immunol. 134, 475–478. doi: 10.1016/j.jaci.2014.02.029
Fernandes, F. M., Silva, E. P., Martins, R. R., and Oliveira, A. G. (2018). QTc interval prolongation in critically ill patients: prevalence, risk factors and associated medications. PLoS One 13:e0199028. doi: 10.1371/journal.pone.0199028
Fisone, G., Borgkvist, A., and Usiello, A. (2004). Caffeine as a psychomotor stimulant: mechanism of action. Cell Mol. Life Sci. 61, 857–872. doi: 10.1007/s00018-003-3269-3
Flegel, C., Manteniotis, S., Osthold, S., Hatt, H., and Gisselmann, G. (2013). Expression profile of ectopic olfactory receptors determined by deep sequencing. PloS One 8:e55368. doi: 10.1371/journal.pone.0055368
Foster, S. R., Blank, K., Hoe, L. E. S., Behrens, M., Meyerhof, W., Peart, J. N., et al. (2014). Bitter taste receptor agonists elicit G-protein-dependent negative inotropy in the murine heart. Faseb J. 28, 4497–4508. doi: 10.1096/fj.14-256305
Foster, S. R., Porrello, E. R., Purdue, B., Chan, H.-W., Voigt, A., Frenzel, S., et al. (2013). Expression, regulation and putative nutrient-sensing function of taste GPCRs in the heart. PLoS One 8:e64579. doi: 10.1371/journal.pone.0064579
Foster, S. R., Porrello, E. R., Stefani, M., Smith, N. J., Molenaar, P., Dos Remedios, C. G., et al. (2015a). Cardiac gene expression data and in silico analysis provide novel insights into human and mouse taste receptor gene regulation. Naunyn Schmiedeberg’s Arch. Pharmacol. 388, 1009–1027. doi: 10.1007/s00210-015-1118-1
Foster, S. R., Roura, E., Molenaar, P., and Thomas, W. G. (2015b). G protein-coupled receptors in cardiac biology: old and new receptors. Biophys. Rev. 7, 77–89. doi: 10.1007/s12551-014-0154-2
Freund, J. R., Mansfield, C. J., Doghramji, L. J., Adappa, N. D., Palmer, J. N., Kennedy, D. W., et al. (2018). Activation of airway epithelial bitter taste receptors by Pseudomonas aeruginosa quinolones modulates calcium, cyclic-AMP, and nitric oxide signaling. J. Biol. Chem. 293, 9824–9840. doi: 10.1074/jbc.RA117.001005
Gaida, M. M., Dapunt, U., and Hansch, G. M. (2016a). Sensing developing biofilms: the bitter receptor T2R38 on myeloid cells. Pathog Dis. 74:ftw004. doi: 10.1093/femspd/ftw004
Gaida, M. M., Mayer, C., Dapunt, U., Stegmaier, S., Schirmacher, P., Wabnitz, G. H., et al. (2016b). Expression of the bitter receptor T2R38 in pancreatic cancer: localization in lipid droplets and activation by a bacteria-derived quorum-sensing molecule. Oncotarget 7, 12623–12632. doi: 10.18632/oncotarget.7206
Garcia-Esparcia, P., Schlüter, A., Carmona, M., Moreno, J., Ansoleaga, B., Torrejón-Escribano, B., et al. (2013). Functional genomics reveals dysregulation of cortical olfactory receptors in parkinson disease: novel putative chemoreceptors in the human brain. J. Neuropathol.Exp. Neurol. 72, 524–539. doi: 10.1097/NEN.0b013e318294fd76
Gardiner, S. M., Chhabra, S. R., Harty, C., Williams, P., Pritchard, D. I., Bycroft, B. W., et al. (2001). Haemodynamic effects of the bacterial quorum sensing signal molecule, N-(3-oxododecanoyl)-L-homoserine lactone, in conscious, normal and endotoxaemic rats. Br. J. Pharmacol. 133, 1047–1054. doi: 10.1038/sj.bjp.0704174
Gentiluomo, M., Crifasi, L., Luddi, A., Locci, D., Barale, R., Piomboni, P., et al. (2017). Taste receptor polymorphisms and male infertility. Hum. Reprod. 32, 2324–2331. doi: 10.1093/humrep/dex305
Gil, S., Coldwell, S., Drury, J. L., Arroyo, F., Phi, T., Saadat, S., et al. (2015). Genotype-Specific Regulation of Oral Innate Immunity by T2R38 Taste Receptor. Mol. Immunol. 68, 663–670. doi: 10.1016/j.molimm.2015.10.012
Giudicessi, J. R., Ackerman, M. J., and Camilleri, M. (2018). Cardiovascular safety of prokinetic agents: a focus on drug-induced arrhythmias. Neurogastroenterol. Motil. 30:e13302. doi: 10.1111/nmo.13302
Grassin Delyle, S., Abrial, C., Brollo, M., Fayad-Kobeissi, S., Naline, E., and Devillier, P. (2014). “Characterization of the expression and the role of bitter taste receptors in human lung parenchyma and macrophages,” in D36. Sepsis, Acute Respiratory Distress Syndrome, and Acute Lung Injury, (New York, NY: American Thoracic Society), A5749–A5749.
Grassin-Delyle, S., Abrial, C., Fayad-Kobeissi, S., Brollo, M., Faisy, C., Alvarez, J.-C., et al. (2013). The expression and relaxant effect of bitter taste receptors in human bronchi. Respir. Res. 14, 134–134. doi: 10.1186/1465-9921-14-134
Guinamard, R., Chatelier, A., Demion, M., Potreau, D., Patri, S., Rahmati, M., et al. (2004). Functional characterization of a Ca(2+)-activated non-selective cation channel in human atrial cardiomyocytes. J. Physiol. 558, 75–83. doi: 10.1113/jphysiol.2004.063974
Guleri, A., Kumar, A., Morgan, R. J., Hartley, M., and Roberts, D. H. (2012). Anaphylaxis to chlorhexidine-coated central venous catheters: a case series and review of the literature. Surg. Infect. 13, 171–174. doi: 10.1089/sur.2011.011
Hague, C., Uberti, M. A., Chen, Z., Bush, C. F., Jones, S. V., Ressler, K. J., et al. (2004). Olfactory receptor surface expression is driven by association with the β<sub>2</sub>-adrenergic receptor. Proc. Natl.Acad. Sci. U.S.A. 101:13672. doi: 10.1073/pnas.0403854101
Hayes, J. E., Wallace, M. R., Knopik, V. S., Herbstman, D. M., Bartoshuk, L. M., and Duffy, V. B. (2011). Allelic variation in TAS2R bitter receptor genes associates with variation in sensations from and ingestive behaviors toward common bitter beverages in adults. Chem. Sens. 36, 311–319. doi: 10.1093/chemse/bjq132
Heiser, J. M., Daya, M. R., Magnussen, A. R., Norton, R. L., Spyker, D. A., Allen, D. W., et al. (1992). Massive strychnine intoxication: serial blood levels in a fatal case. J. Toxicol. Clin. Toxicol. 30, 269–283. doi: 10.3109/15563659209038638
Hinrichs, A. L., Wang, J. C., Bufe, B., Kwon, J. M., Budde, J., Allen, R., et al. (2006). Functional variant in a bitter-taste receptor (hTAS2R16) influences risk of alcohol dependence. Am. J. Hum. Genet. 78, 103–111. doi: 10.1086/499253
Huang, L., Shanker, Y. G., Dubauskaite, J., Zheng, J. Z., Yan, W., Rosenzweig, S., et al. (1999). Ggamma13 colocalizes with gustducin in taste receptor cells and mediates IP3 responses to bitter denatonium. Nat. Neurosci. 2, 1055–1062. doi: 10.1038/15981
Hudson, J., Titmarsh, D., Hidalgo, A., Wolvetang, E., and Cooper-White, J. (2012). Primitive cardiac cells from human embryonic stem cells. Stem Cells Dev. 21, 1513–1523. doi: 10.1089/scd.2011.0254
Ihama, Y., Ageda, S., Fuke, C., and Miyazaki, T. (2007). Autopsy case of aspirin intoxication: distribution of salicylic acid and salicyluric acid in body fluid and organs. Chudoku Kenkyu 20, 375–380.
Isberg, V., Mordalski, S., Munk, C., Rataj, K., Harpsoe, K., Hauser, A. S., et al. (2016). GPCRdb: an information system for G protein-coupled receptors. Nucleic Acids Res. 44, D356–D364. doi: 10.1093/nar/gkw1218
Ivanova, A. A., Maksimov, V. N., Orlov, P. S., Ivanoshchuk, D. E., Savchenko, S. V., and Voevoda, M. I. (2017). Association of the genetic markers for myocardial infarction with sudden cardiac death. Indian Heart J. 69(Suppl. 1), S8–S11. doi: 10.1016/j.ihj.2016.07.016
Jaggupilli, A., Howard, R., Upadhyaya, J. D., Bhullar, R. P., and Chelikani, P. (2016). Bitter taste receptors: novel insights into the biochemistry and pharmacology. Int. J. Biochem. Cell Biol. 77, 184–196. doi: 10.1016/j.biocel.2016.03.005
Jaggupilli, A., Singh, N., Upadhyaya, J., Sikarwar, A. S., Arakawa, M., Dakshinamurti, S., et al. (2017). Analysis of the expression of human bitter taste receptors in extraoral tissues. Mol. Cell Biochem. 426, 137–147. doi: 10.1007/s11010-016-2902-z
Jeon, T.-I., Seo, Y.-K., and Osborne, T. F. (2011). Gut bitter taste receptor signalling induces ABCB1 through a mechanism involving CCK. Biochem. J. 438, 33–37. doi: 10.1042/BJ20110009
Ji, M., Su, X., Su, X., Chen, Y., Huang, W., Zhang, J., et al. (2014). Identification of novel compounds for human bitter taste receptors. Chem. Biol. Drug Des. 84, 63–74. doi: 10.1111/cbdd.12293
Kaji, I., Karaki, S., Fukami, Y., Terasaki, M., and Kuwahara, A. (2009). Secretory effects of a luminal bitter tastant and expressions of bitter taste receptors, T2Rs, in the human and rat large intestine. Am. J. Physiol. Gastrointest. Liver Physiol. 296, G971–G981. doi: 10.1152/ajpgi.90514.2008
Kang, K. S., Kim, H. I., Kim, O. H., Cha, K. C., Kim, H., Lee, K. H., et al. (2016). Clinical outcomes of adverse cardiovascular events in patients with acute dapsone poisoning. Clin. Exp. Emerg. Med. 3, 41–45. doi: 10.15441/ceem.15.088
Kaplan, B., Buchanan, J., and Krantz, M. J. (2011). QTc prolongation due to dextromethorphan. Int. J. Cardiol. 148, 363–364.
Kim, D., Pauer, S. H., Yong, H. M., An, S. S., and Liggett, S. B. (2016). beta2-adrenergic receptors chaperone trapped bitter taste receptor 14 to the cell surface as a heterodimer and exert unidirectional desensitization of taste receptor function. J. Biol. Chem. 291, 17616–17628. doi: 10.1074/jbc.M116.722736
Kim, D., Woo, J. A., Geffken, E., An, S. S., and Liggett, S. B. (2017). Coupling of airway smooth muscle bitter taste receptors to intracellular signaling and relaxation is via galphai1,2,3. Am. J. Respir. Cell Mol. Biol. 56, 762–771. doi: 10.1165/rcmb.2016-0373OC
Kim, U., Wooding, S., Ricci, D., Jorde, L. B., and Drayna, D. (2005). Worldwide haplotype diversity and coding sequence variation at human bitter taste receptor loci. Hum. Mutat 26, 199–204. doi: 10.1002/humu.20203
Kirch, W., Halabi, A., Linde, M., Santos, S. R., and Ohnhaus, E. E. (1989). Negative effects of famotidine on cardiac performance assessed by noninvasive hemodynamic measurements. Gastroenterology 96, 1388–1392. doi: 10.1016/0016-5085(89)90503-9
Koch, W., Hoppmann, P., Schomig, A., and Kastrati, A. (2011). Variations of specific non-candidate genes and risk of myocardial infarction: a replication study. Int. J. Cardiol. 147, 38–41. doi: 10.1016/j.ijcard.2009.07.028
Kong, H., Jones, P. P., Koop, A., Zhang, L., Duff, H. J., and Chen, S. R. (2008). Caffeine induces Ca2+ release by reducing the threshold for luminal Ca2+ activation of the ryanodine receptor. Biochem. J. 414, 441–452. doi: 10.1042/BJ20080489
Kuhn, C., Bufe, B., Batram, C., and Meyerhof, W. (2010). Oligomerization of TAS2R bitter taste receptors. Chem. Senses 35, 395–406. doi: 10.1093/chemse/bjq027
Labarinas, S., Meulmester, K., Greene, S., Thomas, J., Virk, M., and Erkonen, G. (2018). Extracorporeal cardiopulmonary resuscitation after diphenhydramine ingestion. J. Med. Toxicol. 14, 253–256. doi: 10.1007/s13181-018-0672-6
Lagerstrom, M. C., and Schioth, H. B. (2008). Structural diversity of G protein-coupled receptors and significance for drug discovery. Nat. Rev. Drug Discov. 7, 339–357. doi: 10.1038/nrd2518
Lau, G. (1995). A fatal case of drug-induced multi-organ damage in a patient with Hansen’s disease: dapsone syndrome or rifampicin toxicity? Forensic. Sci. Int. 73, 109–115. doi: 10.1016/0379-0738(95)01719-y
Lee, K. W., Kayser, S. R., Hongo, R. H., Tseng, Z. H., and Scheinman, M. M. (2004). Famotidine and long QT syndrome. Am. J. Cardiol. 93, 1325–1327. doi: 10.1016/j.amjcard.2004.02.025
Lee, R. J., Chen, B., Redding, K. M., Margolskee, R. F., and Cohen, N. A. (2014). Mouse nasal epithelial innate immune responses to Pseudomonas aeruginosa quorum-sensing molecules require taste signaling components. Innate Immun. 20, 606–617. doi: 10.1177/1753425913503386
Lee, R. J., Xiong, G., Kofonow, J. M., Chen, B., Lysenko, A., Jiang, P., et al. (2012). T2R38 taste receptor polymorphisms underlie susceptibility to upper respiratory infection. J. Clin. Invest. 122, 4145–4159. doi: 10.1172/JCI64240
Lee, T. M., Li, R. C., and Chai, C. Y. (1974). Effects of diphenylhydantoin on cardiac arrhythmias induced by picrotoxin. Chin. J. Physiol. 21, 219–229.
Lee, T. M., Yang, K. L., Kuo, J. S., and Chai, C. Y. (1972). Importance of sympathetic mechanism on cardiac arrhythmias induced by picrotoxin. Exp. Neurol. 36, 389–398.
Lei, Y., Zheng, M. H., Huang, W., Zhang, J., and Lu, Y. (2018). Wet beriberi with multiple organ failure remarkably reversed by thiamine administration: a case report and literature review. Medicine 97:e0010. doi: 10.1097/MD.0000000000010010
Lin, C. J., Chen, Y. T., Kuo, J. S., and Lee, A. Y. (1992). Antiarrhythmic action of naloxone. Suppression of picrotoxin-induced cardiac arrhythmias in the rat. Jpn. Heart. J. 33, 365–372. doi: 10.1536/ihj.33.365
Liszt, K. I., Ley, J. P., Lieder, B., Behrens, M., Stöger, V., Reiner, A., et al. (2017). Caffeine induces gastric acid secretion via bitter taste signaling in gastric parietal cells. Proc. Natl. Acad. Sci. U.S.A. 114:E6260. doi: 10.1073/pnas.1703728114
Liu, A. M., Ho, M. K., Wong, C. S., Chan, J. H., Pau, A. H., and Wong, Y. H. (2003). Galpha(16/z) chimeras efficiently link a wide range of G protein-coupled receptors to calcium mobilization. J. Biomol. Screen 8, 39–49. doi: 10.1177/1087057102239665
Liu, M., Qian, W., Subramaniyam, S., Liu, S., and Xin, W. (2020). Denatonium enhanced the tone of denuded rat aorta via bitter taste receptor and phosphodiesterase activation. Eur. J. Pharmacol. 872:172951. doi: 10.1016/j.ejphar.2020.172951
Loconto, J., Papes, F., Chang, E., Stowers, L., Jones, E. P., Takada, T., et al. (2003). Functional expression of murine V2R pheromone receptors involves selective association with the M10 and M1 families of MHC Class Ib Molecules. Cell 112, 607–618. doi: 10.1016/s0092-8674(03)00153-3
Loke, W. M., Hodgson, J. M., Proudfoot, J. M., Mckinley, A. J., Puddey, I. B., and Croft, K. D. (2008). Pure dietary flavonoids quercetin and (-)-epicatechin augment nitric oxide products and reduce endothelin-1 acutely in healthy men. Am. J. Clin. Nutr. 88, 1018–1025. doi: 10.1093/ajcn/88.4.1018
Lossow, K., Hubner, S., Roudnitzky, N., Slack, J. P., Pollastro, F., Behrens, M., et al. (2016). Comprehensive analysis of mouse bitter taste receptors reveals different molecular receptive ranges for orthologous receptors in mice and humans. J. Biol. Chem. 291, 15358–15377. doi: 10.1074/jbc.M116.718544
Lu, P., Zhang, C.-H., Lifshitz, L. M., and Zhuge, R. (2017). Extraoral bitter taste receptors in health and disease. 149, 181–119. doi: 10.1085/jgp.201611637
Lu, Z. K., Yuan, J., Li, M., Sutton, S. S., Rao, G. A., Jacob, S., et al. (2015). Cardiac risks associated with antibiotics: azithromycin and levofloxacin. Expert. Opin. Drug. Saf. 14, 295–303. doi: 10.1517/14740338.2015.989210
Lund, T. C., Kobs, A. J., Kramer, A., Nyquist, M., Kuroki, M. T., Osborn, J., et al. (2013). Bone marrow stromal and vascular smooth muscle cells have chemosensory capacity via bitter taste receptor expression. PLoS One 8:e58945. doi: 10.1371/journal.pone.0058945
Luzza, F., Raffa, S., Saporito, F., and Oreto, G. (2006). Torsades de pointes in congenital long QT syndrome following low-dose orphenadrine. Int. J. Clin. Pract. 60, 606–608. doi: 10.1111/j.1368-5031.2006.00764.x
Macleod, J. G., and Phillips, L. (1947). Hypersensitivity to colchicine. Ann. Rheum. Dis. 6, 224–229.
Malizia, E., Sarcinelli, L., Pascarella, M., Ambrosini, M., Smeriglio, M., and Russo, A. (1980). Cardiotoxicity from orphenadrine intoxication in humans. Arch. Toxicol. Suppl. 4, 425–427. doi: 10.1007/978-3-642-67729-8_98
Mannaerts, D., Faes, E., Goovaerts, I., Stoop, T., Cornette, J., Gyselaers, W., et al. (2017). Flow-mediated dilation and peripheral arterial tonometry are disturbed in preeclampsia and reflect different aspects of endothelial function. Am. J. Physiol. Regul. Integr. Comp. Physiol. 313, R518–R525. doi: 10.1152/ajpregu.00514.2016
Manson, M. L., Säfholm, J., Al-Ameri, M., Bergman, P., Orre, A.-C., Swärd, K., et al. (2014). Bitter taste receptor agonists mediate relaxation of human and rodent vascular smooth muscle. Eur. J. Pharmacol. 740, 302–311. doi: 10.1016/j.ejphar.2014.07.005
Martin, L. T. P., Nachtigal, M. W., Selman, T., Nguyen, E., Salsman, J., Dellaire, G., et al. (2018). Bitter taste receptors are expressed in human epithelial ovarian and prostate cancers cells and noscapine stimulation impacts cell survival. Mol. Cell. Biochem. 454, 203–214. doi: 10.1007/s11010-018-3464-z
Martin-Fernandez, B., De Las Heras, N., Valero-Munoz, M., Ballesteros, S., Yao, Y. Z., Stanton, P. G., et al. (2014). Beneficial effects of proanthocyanidins in the cardiac alterations induced by aldosterone in rat heart through mineralocorticoid receptor blockade. PLoS One 9:e111104. doi: 10.1371/journal.pone.0111104
McClintock, T. S., Landers, T. M., Gimelbrant, A. A., Fuller, L. Z., Jackson, B. A., Jayawickreme, C. K., et al. (1997). Functional expression of olfactory-adrenergic receptor chimeras and intracellular retention of heterologously expressed olfactory receptors. Brain Res. Mol. Brain Res. 48, 270–278. doi: 10.1016/s0169-328x(97)00099-5
Meyerhof, W. (2005). Elucidation of mammalian bitter taste. Rev. Physiol. Biochem. Pharmacol. 154, 37–72.
Meyerhof, W., Batram, C., Kuhn, C., Brockhoff, A., Chudoba, E., Bufe, B., et al. (2010). The molecular receptive ranges of human TAS2R bitter taste receptors. Chem. Sen. 35, 157–170.
Meyer-Massetti, C., Cheng, C. M., Sharpe, B. A., Meier, C. R., and Guglielmo, B. J. (2010). The FDA extended warning for intravenous haloperidol and torsades de pointes: how should institutions respond? J. Hosp. Med,. 5, E8–E16.
Mfuna Endam, L., Filali-Mouhim, A., Boisvert, P., Boulet, L.-P., Bossé, Y., and Desrosiers, M. (2014). Genetic variations in taste receptors are associated with chronic rhinosinusitis: a replication study. Int. Forum Allergy Rhinol. 4, 200–206.
Mikolajczyk, T. P., Nosalski, R., Skiba, D. S., Koziol, J., Mazur, M., Justo-Junior, A. S., et al. (2019). 1,2,3,4,6-Penta-O-galloyl-beta-d-glucose modulates perivascular inflammation and prevents vascular dysfunction in angiotensin II-induced hypertension. Br. J. Pharmacol. 176, 1951–1965.
Mina, Y., Rinkevich-Shop, S., Konen, E., Goitein, O., Kushnir, T., Epstein, F. H., et al. (2013). Mast cell inhibition attenuates myocardial damage, adverse remodeling, and dysfunction during fulminant myocarditis in the rat. J. Cardiovasc. Pharmacol. Ther. 18, 152–161.
Miyamori, I., Sakai, T., Ito, T., Sugihara, N., Ikeda, M., Takeda, Y., et al. (1985). Reversible hyperkalemia induced by flufenamic acid in asymptomatic hyporeninemic patient. Jpn. J. Med. 24, 269–272.
Morales, M. A., Silva, A., Brito, G., Bustamante, S., Ponce, H., and Paeile, C. (1995). Vasorelaxant effect of the analgesic clonixin on rat aorta. Gen. Pharmacol. 26, 425–430.
Morales-Soto, N., Dunham, S. J. B., Baig, N. F., Ellis, J. F., Madukoma, C. S., Bohn, P. W., et al. (2018). Spatially dependent alkyl quinolone signaling responses to antibiotics in Pseudomonas aeruginosa swarms. J. Biol. Chem. 293, 9544—9552. doi: 10.1074/jbc.RA118.002605
Moreira, I. S. (2014). Structural features of the G-protein/GPCR interactions. Biochim. Biophys. Acta Gen. Subj. 1840, 16–33.
Mueller, K. L., Hoon, M. A., Erlenbach, I., Chandrashekar, J., Zuker, C. S., and Ryba, N. J. (2005). The receptors and coding logic for bitter taste. Nature 434, 225–229.
Mukerji, V., Alpert, M. A., Flaker, G. C., Beach, C. L., and Weber, R. D. (1986). Cardiac conduction abnormalities and atrial arrhythmias associated with salicylate toxicity. Pharmacotherapy 6, 41–43.
Nakanishi, M., Ando, H., Watanabe, N., Kitamura, K., Ito, K., Okayama, H., et al. (2000). Identification and characterization of human Wee1B, a new member of the Wee1 family of Cdk-inhibitory kinases. Genes Cells 5, 839–847.
Nia, A. M., Fuhr, U., Gassanov, N., Erdmann, E., and Er, F. (2010). Torsades de pointes tachycardia induced by common cold compound medication containing chlorpheniramine. Eur. J. Clin. Pharmacol. 66, 1173–1175.
Nishino, T., Wakai, S., Aoki, H., and Inokuchi, S. (2018). Cardiac arrest caused by diphenhydramine overdose. Acute Med. Surg. 5, 380–383.
Nowak, S., Di Pizio, A., Levit, A., Niv, M. Y., Meyerhof, W., and Behrens, M. (2018). Reengineering the ligand sensitivity of the broadly tuned human bitter taste receptor TAS2R14. Biochim. Biophys. Acta Gen. Subj. 1862, 2162–2173.
Nugraha, B., Buono, M. F., Von Boehmer, L., Hoerstrup, S. P., and Emmert, M. Y. (2019). Human cardiac organoids for disease modeling. Clin. Pharmacol. Ther. 105, 79–85.
Okeahialam, B. N. (2015). Cardiac dysrhythmia resulting from antibiotic abuse. Niger. Med. J. 56, 429–432.
Orsmark-Pietras, C., James, A., Konradsen, J. R., Nordlund, B., Soderhall, C., Pulkkinen, V., et al. (2013). Transcriptome analysis reveals upregulation of bitter taste receptors in severe asthmatics. Eur. Respir. J. 42, 65–78.
Ortiz, M., Martin, A., Arribas, F., Coll-Vinent, B., Del Arco, C., Peinado, R., et al. (2017). Randomized comparison of intravenous procainamide vs. intravenous amiodarone for the acute treatment of tolerated wide QRS tachycardia: the PROCAMIO study. Eur. Heart J. 38, 1329–1335.
Osadchii, O. E. (2018). Effects of antiarrhythmics and hypokalemia on the rate adaptation of cardiac repolarization. Scand. Cardiovasc. J. 52, 218–226.
Ozeck, M., Brust, P., Xu, H., and Servant, G. (2004). Receptors for bitter, sweet and umami taste couple to inhibitory G protein signaling pathways. Eur. J. Pharmacol. 489, 139–149.
Papageorgiou, N., Briasoulis, A., Lazaros, G., Imazio, M., and Tousoulis, D. (2017). Colchicine for prevention and treatment of cardiac diseases: a meta-analysis. Cardiovasc. Ther. 35, 10–18.
Pedretti, R. F., Colombo, E., Sarzi Braga, S., Ballardini, L., and Caru, B. (1995). Effects of oral pirenzepine on heart rate variability and baroreceptor reflex sensitivity after acute myocardial infarction. J. Am. Coll Cardiol. 25, 915–921.
Perera, R. K., Fischer, T. H., Wagner, M., Dewenter, M., Vettel, C., Bork, N. I., et al. (2017). Atropine augments cardiac contractility by inhibiting cAMP-specific phosphodiesterase type 4. Sci. Rep. 7:15222.
Perkins, A., and Marill, K. (2012). Accelerated AV nodal conduction with use of procainamide in atrial fibrillation. J. Emerg. Med. 42, e47–e50.
Pinto-Scognamiglio, W. (1968). Effects of thujone on spontaneous activity and on conditioned behavior of rats. Boll. Chim. Farm. 107, 780–791.
Pluznick, J. L., Protzko, R. J., Gevorgyan, H., Peterlin, Z., Sipos, A., Han, J., et al. (2013). Olfactory receptor responding to gut microbiota-derived signals plays a role in renin secretion and blood pressure regulation. Proc. Natl.Acad. Sci. 110:4410.
Ponraj, L., Mishra, A. K., Koshy, M., and Carey, R. A. B. (2017). A rare case report of Strychnos nux-vomica poisoning with bradycardia. J. Fam. Med. Prim. Care 6, 663–665.
Postma, D. F., Spitoni, C., Van Werkhoven, C. H., Van Elden, L. J. R., Oosterheert, J. J., and Bonten, M. J. M. (2019). Cardiac events after macrolides or fluoroquinolones in patients hospitalized for community-acquired pneumonia: post-hoc analysis of a cluster-randomized trial. BMC Infect. Dis. 19:17.
Rainer, P. P., Primessnig, U., Harenkamp, S., Doleschal, B., Wallner, M., Fauler, G., et al. (2013). Bile acids induce arrhythmias in human atrial myocardium–implications for altered serum bile acid composition in patients with atrial fibrillation. Heart 99, 1685–1692.
Reed, D. R., Zhu, G., Breslin, P. A. S., Duke, F. F., Henders, A. K., Campbell, M. J., et al. (2010). The perception of quinine taste intensity is associated with common genetic variants in a bitter receptor cluster on chromosome 12. Hum. Mol. Genet. 19, 4278–4285.
Reichling, C., Meyerhof, W., and Behrens, M. (2008). Functions of human bitter taste receptors depend on N-glycosylation. J. Neurochem. 106, 1138–1148.
Risso, D., Morini, G., Pagani, L., Quagliariello, A., Giuliani, C., De Fanti, S., et al. (2014). Genetic signature of differential sensitivity to stevioside in the Italian population. Genes Nutr. 9:401.
Risso, D. S., Giuliani, C., Antinucci, M., Morini, G., Garagnani, P., Tofanelli, S., et al. (2017). A bio-cultural approach to the study of food choice: the contribution of taste genetics, population and culture. Appetite 114, 240–247.
Rohatgi, G., Rissmiller, D. J., and Gorman, J. M. (2005). Treatment of carisoprodol dependence: a case report. J. Psychiatr. Pract. 11, 347–352.
Roudnitzky, N., Risso, D., Drayna, D., Behrens, M., Meyerhof, W., and Wooding, S. P. (2016). Copy number variation in TAS2R bitter taste receptor genes: structure. origin, and population genetics. Chem. Sen. 41, 649–659.
Rozengurt, N., Wu, S. V., Chen, M. C., Huang, C., Sternini, C., and Rozengurt, E. (2006). Colocalization of the alpha-subunit of gustducin with PYY and GLP-1 in L cells of human colon. Am. J. Physiol. Gastrointest Liver Physiol. 291, G792–G802.
Ruiz-Avila, L., Wong, G. T., Damak, S., and Margolskee, R. F. (2001). Dominant loss of responsiveness to sweet and bitter compounds caused by a single mutation in α-gustducin. Proc. Natl. Acad. Sci. U.S.A. 98:8868.
Russo, V., Puzio, G., and Siniscalchi, N. (2006). Azithromycin-induced QT prolongation in elderly patient. Acta Biomed. 77, 30–32.
Salem, J. E., Alexandre, J., Bachelot, A., and Funck-Brentano, C. (2016). Influence of steroid hormones on ventricular repolarization. Pharmacol. Ther. 167, 38–47.
Santone, D. J., Shahani, R., Rubin, B. B., Romaschin, A. D., and Lindsay, T. F. (2008). Mast cell stabilization improves cardiac contractile function following hemorrhagic shock and resuscitation. Am. J. Physiol. Heart Circ. Physiol. 294, H2456–H2464.
Schoenwald, P. K., Sprung, J., Abdelmalak, B., Mraovic, B., Tetzlaff, J. E., and Gurm, H. S. (1999). Complete atrioventricular block and cardiac arrest following intravenous famotidine administration. Anesthesiology 90, 623–626.
Schroeter, H., Heiss, C., Balzer, J., Kleinbongard, P., Keen, C. L., Hollenberg, N. K., et al. (2006). (-)-Epicatechin mediates beneficial effects of flavanol-rich cocoa on vascular function in humans. Proc. Natl. Acad. Sci. US.A. 103, 1024–1029.
Serban, M. C., Sahebkar, A., Zanchetti, A., Mikhailidis, D. P., Howard, G., Antal, D., et al. (2016). Effects of quercetin on blood pressure: a systematic review and meta-analysis of randomized controlled trials. J. Am. Heart Assoc. 5:e002713.
Shah, A. S., Ben-Shahar, Y., Moninger, T. O., Kline, J. N., and Welsh, M. J. (2009). Motile cilia of human airway epithelia are chemosensory. Science 325, 1131–1134.
Shaw, L., Mansfield, C., Colquitt, L., Lin, C., Ferreira, J., Emmetsberger, J., et al. (2018). Personalized expression of bitter ‘taste’ receptors in human skin. PLoS One 13:e0205322. doi: 10.1371/journal.pone.0205322
Shiffman, D., Ellis, S. G., Rowland, C. M., Malloy, M. J., Luke, M. M., Iakoubova, O. A., et al. (2005). Identification of four gene variants associated with myocardial infarction. Am. J. Hum. Genet. 77, 596–605.
Shiffman, D., O’meara, E. S., Bare, L. A., Rowland, C. M., Louie, J. Z., Arellano, A. R., et al. (2008). Association of gene variants with incident myocardial infarction in the Cardiovascular Health Study. Arterioscler. Thromb. Vasc. Biol. 28, 173–179.
Shimamura, M., Hazato, T., Ashino, H., Yamamoto, Y., Iwasaki, E., Tobe, H., et al. (2001). Inhibition of angiogenesis by humulone, a bitter acid from beer hop. Biochem. Biophys. Res. Commun. 289, 220–224.
Singer, M., Deutschman, C. S., Seymour, C. W., Shankar-Hari, M., Annane, D., Bauer, M., et al. (2016). The third international consensus definitions for sepsis and septic shock (Sepsis-3). Jama 315, 801–810.
Singh, N., Chakraborty, R., Bhullar, R. P., and Chelikani, P. (2014). Differential expression of bitter taste receptors in non-cancerous breast epithelial and breast cancer cells. Biochem. Biophys. Res. Commun. 446, 499–503.
Soong, P. L., Tiburcy, M., and Zimmermann, W. H. (2012). Cardiac differentiation of human embryonic stem cells and their assembly into engineered heart muscle. Curr. Protoc. Cell Biol. Chapter 23:Unit23.28.
Soranzo, N., Bufe, B., Sabeti, P. C., Wilson, J. F., Weale, M. E., Marguerie, R., et al. (2005). Positive selection on a high-sensitivity allele of the human bitter-taste receptor TAS2R16. Curr. Biol. 15, 1257–1265.
Stas, P., Faes, D., and Noyens, P. (2008). Conduction disorder and QT prolongation secondary to long-term treatment with chloroquine. Int. J. Cardiol. 127, e80–e82.
Stern, L., Giese, N., Hackert, T., Strobel, O., Schirmacher, P., Felix, K., et al. (2018). Overcoming chemoresistance in pancreatic cancer cells: role of the bitter taste receptor T2R10. J. Cancer 9, 711–725.
Suarez, C. R., and Ow, E. P. (1992). Chloramphenicol toxicity associated with severe cardiac dysfunction. Pediatr. Cardiol. 13, 48–51.
Sutherland, J. M. (1959). Fatal cardiovascular collapse of infants receiving large amounts of chloramphenicol. JAMA Pediatr. 97, 761–767.
Teloh, J. K., Dohle, D. S., Petersen, M., Verhaegh, R., Waack, I. N., Roehrborn, F., et al. (2016). Histidine and other amino acids in blood and urine after administration of Bretschneider solution (HTK) for cardioplegic arrest in patients: effects on N-metabolism. Amino Acids 48, 1423–1432.
Tepper, B. J., Koelliker, Y., Zhao, L., Ullrich, N. V., Lanzara, C., D’adamo, P., et al. (2008). Variation in the bitter-taste receptor gene TAS2R38, and adiposity in a genetically isolated population in Southern Italy. Obesity 16, 2289–2295.
Thompson, P. L. (2019). Colchicine in cardiovascular disease: repurposing an ancient gout drug. Clin. Ther. 41, 8–10.
Tirziu, D., Giordano, F. J., and Simons, M. (2010). Cell communications in the heart. Circulation 122, 928–937.
Tonnesmann, E., Kandolf, R., and Lewalter, T. (2013). Chloroquine cardiomyopathy - a review of the literature. Immunopharmacol. Immunotoxicol. 35, 434–442.
Tran, H. T. T., Herz, C., Ruf, P., Stetter, R., and Lamy, E. (2018). Human t2r38 bitter taste receptor expression in resting and activated lymphocytes. Front. Immunol. 9:2949. doi: 10.3389/fimmu.2018.02949
Trifiro, G., De Ridder, M., Sultana, J., Oteri, A., Rijnbeek, P., Pecchioli, S., et al. (2017). Use of azithromycin and risk of ventricular arrhythmia. Cmaj 189, E560–E568.
Tsygankova, V. O., Lozhkina, G. N., Khasanova, H. M., Kuimov, D. A., Ragino, I. Y., Maksimov, N. V., et al. (2017). Multifactorial prognostication of remote outcomes in patients with Non-ST Elevation acute coronary syndrome. Kardiologiia 57, 28–33.
Ueda, T., Ugawa, S., Yamamura, H., Imaizumi, Y., and Shimada, S. (2003). Functional interaction between T2R taste receptors and G-protein alpha subunits expressed in taste receptor cells. J. Neurosci. 23, 7376–7380.
Uhlen, M., Fagerberg, L., Hallstrom, B. M., Lindskog, C., Oksvold, P., Mardinoglu, A., et al. (2015). Proteomics. Tissue-based map of the human proteome. Science 347:1260419.
Upadhyaya, J., Singh, N., Bhullar, R. P., and Chelikani, P. (2015). The structure–function role of C-terminus in human bitter taste receptor T2R4 signaling. Biochim. Biophys. Acta Biomembr. 1848, 1502–1508.
Upadhyaya, J. D., Singh, N., Sikarwar, A. S., Chakraborty, R., Pydi, S. P., Bhullar, R. P., et al. (2014). Dextromethorphan mediated bitter taste receptor activation in the pulmonary circuit causes vasoconstriction. PLoS One 9:e110373. doi: 10.1371/journal.pone.0110373
Vanherweghem, J. L. (1997). Association of valvular heart disease with Chinese-herb nephropathy. Lancet 350:1858.
Verbeurgt, C., Veithen, A., Carlot, S., Tarabichi, M., Dumont, J. E., Hassid, S., et al. (2017). The human bitter taste receptor T2R38 is broadly tuned for bacterial compounds. PLoS One 12:e0181302. doi: 10.1371/journal.pone.0181302
Vesely, P., Stracina, T., Hlavacova, M., Halamek, J., Kolarova, J., Olejnickova, V., et al. (2019). Haloperidol affects coupling between QT and RR intervals in guinea pig isolated heart. J. Pharmacol. Sci. 139, 23–28.
Vo, K. T., Horng, H., Smollin, C. G., and Benowitz, N. L. (2017). Severe carisoprodol withdrawal After a 14-year addiction and acute overdose. J. Emerg. Med. 52, 680–683.
Vukajlovic, D. D., Guettler, N., Miric, M., and Pitschner, H. F. (2006). Effects of atropine and pirenzepine on heart rate turbulence. Ann. Noninvasive Electrocardiol. 11, 34–37.
Wang, J., Gareri, C., and Rockman Howard, A. (2018). G-protein–coupled receptors in heart disease. Circ. Res. 123, 716–735.
Wang, Y., Yu, X., Wang, F., Wang, Y., Wang, Y., Li, H., et al. (2013). Yohimbine promotes cardiac NE release and prevents LPS-induced cardiac dysfunction via blockade of presynaptic alpha2A-adrenergic receptor. PLoS One 8:e63622. doi: 10.1371/journal.pone.0063622
Wasserman, A. J., Horgan, J. H., Ul-Hassan, Z., and Proctor, J. D. (1975). Diphenidol treatment of arrhythmias. Chest 67, 422–424.
Weaver, L. C., Alexander, W. M., Abreu, B. E., Richards, A. B., Jones, W. R., and Begley, R. W. (1958). The mechanism of the hypotensive action of narcotine hydrochloride. J. Pharmacol. Exp. Ther. 123, 287–295.
Wolfle, U., Elsholz, F. A., Kersten, A., Haarhaus, B., Schumacher, U., and Schempp, C. M. (2016). Expression and functional activity of the human bitter taste receptor TAS2R38 in human placental tissues and JEG-3 Cells. Molecules 21:306.
Wolfle, U., Haarhaus, B., Kersten, A., Fiebich, B., Hug, M. J., and Schempp, C. M. (2015). Salicin from willow bark can modulate neurite outgrowth in human neuroblastoma SH-SY5Y cells. Phytother. Res. 29, 1494–1500. doi: 10.1002/ptr.5400
Wood, D., Webster, E., Martinez, D., Dargan, P., and Jones, A. (2002). Case report: survival after deliberate strychnine self-poisoning, with toxicokinetic data. Crit. Care 6, 456–459.
Wooding, S. P., Atanasova, S., Gunn, H. C., Staneva, R., Dimova, I., and Toncheva, D. (2012). Association of a bitter taste receptor mutation with Balkan Endemic Nephropathy (BEN). BMC Med. Genet.ics 13:96.
Wu, T. C., Chao, C. Y., Lin, S. J., and Chen, J. W. (2012). Low-dose dextromethorphan, a NADPH oxidase inhibitor, reduces blood pressure and enhances vascular protection in experimental hypertension. PLoS One 7:e46067. doi: 10.1371/journal.pone.0046067
Yan, W., Sunavala, G., Rosenzweig, S., Dasso, M., Brand, J. G., and Spielman, A. I. (2001). Bitter taste transduced by PLC-beta(2)-dependent rise in IP(3) and alpha-gustducin-dependent fall in cyclic nucleotides. Am. J. Physiol. Cell Physiol. 280, C742–C751.
Yan, Y., Hu, Y., North, K. E., Franceschini, N., and Lin, D. (2009). Evaluation of population impact of candidate polymorphisms for coronary heart disease in the Framingham Heart Study Offspring Cohort. BMC Proc. 3(Suppl. 7):S118.
Yang, C. C., and Deng, J. F. (1998). Clinical experience in acute overdosage of diphenidol. J. Toxicol. Clin. Toxicol. 36, 33–39.
Yang, P. C., Kurokawa, J., Furukawa, T., and Clancy, C. E. (2010). Acute effects of sex steroid hormones on susceptibility to cardiac arrhythmias: a simulation study. PLoS Comput. Biol. 6:e1000658. doi: 10.1371/journal.pcbi.1000658
Yu, J. H., Chen, D. Y., Chen, H. Y., and Lee, K. H. (2016). Intravenous lipid-emulsion therapy in a patient with cardiac arrest after overdose of diphenhydramine. J. Formos. Med. Assoc. 115, 1017–1018.
Yucel, A., Ozyalcin, S., Talu, G. K., Yucel, E. C., and Erdine, S. (1999). Intravenous administration of caffeine sodium benzoate for postdural puncture headache. Reg. Anesth. Pain Med. 24, 51–54.
Zeng, M., Jiang, W., Tian, Y., Hao, J., Cao, Z., Liu, Z., et al. (2017). Andrographolide inhibits arrhythmias and is cardioprotective in rabbits. Oncotarget 8, 61226–61238.
Zhang, C.-H., Lifshitz, L. M., Uy, K. F., Ikebe, M., Fogarty, K. E., and Zhuge, R. (2013). The cellular and molecular basis of bitter tastant-induced bronchodilation. PLoS Biol. 11:e1001501. doi: 10.1371/journal.pbio.1001501
Zhang, L., Ma, J., Li, S., Xue, R., Jin, M., and Zhou, Y. (2015). Fatal diphenidol poisoning: a case report and a retrospective study of 16 cases. Forensic. Sci. Med. Pathol. 11, 570–576.
Zhang, X., Bedigian, A. V., Wang, W., and Eggert, U. S. (2012). G-protein-coupled receptors participate in cytokinesis. Cytoskeleton (Hoboken) 69, 810–818. doi: 10.1002/cm.21055
Zhang, Y., Hoon, M. A., Chandrashekar, J., Mueller, K. L., Cook, B., Wu, D., et al. (2003). Coding of sweet, bitter, and umami tastes: different receptor cells sharing similar signaling pathways. Cell 112, 293–301.
Zheng, K., Lu, P., Delpapa, E., Bellve, K., Deng, R., Condon, J. C., et al. (2017). Bitter taste receptors as targets for tocolytics in preterm labor therapy. FASEB J. 31, 4037–4052. doi: 10.1096/fj.201601323RR
Zheng, M., Simon, R., Mirlacher, M., Maurer, R., Gasser, T., Forster, T., et al. (2004). TRIO amplification and abundant mRNA expression is associated with invasive tumor growth and rapid tumor cell proliferation in urinary bladder cancer. Am. J. Pathol. 165, 63–69.
Zhou, E., Parikh, P. S., Kanchuger, M. S., and Balsam, L. B. (2019). Intraoperative anaphylaxis to chlorhexidine during LVAD and transplant surgery. J. Cardiothorac. Vasc. Anesth. 33, 169–172.
Keywords: taste receptors, G protein-coupled receptors, cardiac physiology, signaling, polymorphisms, bitter ligands
Citation: Bloxham CJ, Foster SR and Thomas WG (2020) A Bitter Taste in Your Heart. Front. Physiol. 11:431. doi: 10.3389/fphys.2020.00431
Received: 21 February 2020; Accepted: 08 April 2020;
Published: 08 May 2020.
Edited by:
Ronghua ZhuGe, University of Massachusetts Medical School, United StatesReviewed by:
Wenkuan Xin, Southwest University, ChinaCheng-Hai Zhang, Harvard Medical School, United States
Copyright © 2020 Bloxham, Foster and Thomas. This is an open-access article distributed under the terms of the Creative Commons Attribution License (CC BY). The use, distribution or reproduction in other forums is permitted, provided the original author(s) and the copyright owner(s) are credited and that the original publication in this journal is cited, in accordance with accepted academic practice. No use, distribution or reproduction is permitted which does not comply with these terms.
*Correspondence: Walter G. Thomas, dy50aG9tYXNAdXEuZWR1LmF1