- 1Departamento de Biologia, Faculdade de Filosofia, Ciências e Letras de Ribeirão Preto, Universidade de São Paulo, Ribeirão Preto, Brazil
- 2Instituto de Ciências Biológicas, Universidade Federal do Rio Grande, Rio Grande, Brazil
- 3Departamento de Química, Faculdade de Filosofia, Ciências e Letras de Ribeirão Preto, Universidade de São Paulo, Ribeirão Preto, Brazil
- 4Centro Austral de Investigaciones Científicas, CADIC-CONICET, Ushuaia, Argentina
- 5Centro de Biologia Marinha, Universidade de São Paulo, São Sebastião, Brazil
Temperature is an important abiotic factor that drives the evolution of ectotherms owing to its pervasive effects at all levels of organization. Although a species’ thermal tolerance is environmentally driven within a spatial cline, it may be constrained over time due to differential phylogenetic inheritance. At the limits of thermal tolerance, hemolymph oxygen is reduced and lactate formation is increased due to mismatch between oxygen supply and demand; imbalance between enzyme flexibility/stability also impairs the ability to generate energy. Here, we characterized the effects of lower (LL50) and upper (UL50) critical thermal limits on selected descriptors of aerobic and anaerobic metabolism in 12 intertidal crab species distributed from northern Brazil (≈7.8°S) to southern Patagonia (≈53.2°S), considering their phylogeny. We tested for (i) functional trade-offs regarding aerobic and anaerobic metabolism and LDH kinetics in shaping thermal tolerance; (ii) influence of shared ancestry and thermal province on metabolic evolution; and (iii) presence of evolutionary convergences and adaptive peaks in the crab phylogeny. The tropical and subtropical species showed similar systemic and kinetic responses, both differing from the sub-Antarctic crabs. The lower UL50’s of the sub-Antarctic crabs may reflect mismatch between the evolution of aerobic and anaerobic metabolism since these crabs exhibit lower oxygen consumption but higher lactate formation than tropical and subtropical species also at their respective UL50’s. LDH activity increased with temperature increase, while KmPyr remained fairly constant; catalytic coefficient correlated negatively with thermal niche. Thermal tolerance may rely on a putative evolutionary trade-off between aerobic and anaerobic metabolism regarding energy supply, while temperature compensation of kinetic performance is driven by thermal habitat as revealed by the LDH affinity/efficiency equilibrium. The overall physiological evolution revealed two homoplastic adaptive peaks in the sub-Antarctic crabs with a further shift in the tropical/subtropical clade. The physiological traits at UL50 have evolved in a phylogenetic manner while all others were more plastic. Thus, shared inheritance and thermal environment have driven the crabs’ thermal tolerance and metabolic evolution, revealing physiological transformations that have arisen in both colder and warmer climes, especially at higher levels of biological organization and phylogenetic diversity.
Introduction
Biological systems are thermodynamically open, owing to the continuous flux of energy and matter with the surrounding environment (Cannon, 1929; Recordati and Bellini, 2004). Temperature is the main abiotic factor that drives the biogeographical evolution of ectotherms (Pörtner, 2002; Johns and Somero, 2004; Somero, 2004, 2010; Dong et al., 2008; Stevens et al., 2010; Tomanek and Zuzow, 2010) since it affects all levels of organization, from the subcellular to the systemic, including the intact organism. The deleterious effects of temperature often exceed the regulatory capability of an organism’s homeostatic mechanisms, and thus limit the distribution of life, imposing constraints on biogeographical distributions.
In aquatic decapod crustaceans at usual habitat temperatures, the capacity for oxygen uptake by ventilation and its delivery by circulation matches the biochemical demands of the tissues for oxygen: the fully oxygenated hemolymph thus can sustain optimal aerobic metabolism in the tissues (Pörtner and Gutt, 2016; Verberk et al., 2016). However, at the critical thermal limits, hemolymph oxygen levels are reduced due to the mismatch between oxygen supply and demand, imposing limitations on temperature tolerance (Pörtner, 2002). This limitation to systemic oxygen availability creates a hypoxic state, which is unable to sustain tissue demand, leading to anaerobic metabolism at the critical thermal limits as a consequence of elevated oxygen demand or insufficient aerobic capacity (Giomi and Pörtner, 2013). The recruitment of chaperone molecules and anti-oxidant defenses may extend survival, albeit with reduced performance at the molecular level, reverberating at the whole animal level and leading to death. This unifying principle known as “oxygen and capacity limitation of thermal tolerance” (OCLTT) characterizes sensitivity to thermal stress in aquatic invertebrates (Pörtner and Gutt, 2016; Verberk et al., 2016). During aerial exposure, however, air-breathers and amphibious species can attain higher hemolymph oxygen concentrations and increase oxygen diffusion, which may enhance their thermal tolerance by extending aerobic scope (Giomi et al., 2014).
To illustrate, the spider crab Maja squinado exhibits highly oxygenated hemolymph between 8 and 17°C, showing decreased oxygen partial pressures below 8°C and increased hemolymph lactate above 17°C (Frederich and Pörtner, 2000). The shore crabs Carcinus maenas and Cancer irroratus also accumulate hemolymph lactate at temperatures above 32 and 28°C, respectively, after direct transfer from 12°C (Jost et al., 2012). The transition to a predominantly anaerobic metabolism in the lobster Homarus americanus takes place gradually and continuously, accompanying a progressive increase in hemolymph lactate, reaching a maximum at 30°C (Jost et al., 2012). Clearly, the limitation of oxygen supply to the tissues is linked to the onset of anaerobiosis due to a decreased capacity for oxygen uptake and transport. Further, patent patterns of increased oxygen demand and lactate formation are evident when temperature becomes life limiting.
Considering the subcellular environment, kinetic adjustments can result from oxygen- and capacity-limited thermal tolerance (Hochachka and Somero, 2002). Enzymes exhibit an inherent structural flexibility that guarantees their molecular function. However, they must also preserve the molecular rigidity that maintains their three-dimensional structure (Zavodszky et al., 1998). The balance between flexibility and stability in enzyme kinetics is temperature dependent, and is particularly evident with regard to lactate dehydrogenase (LDH) in vertebrate species. LDH converts pyruvate to lactate during anaerobic metabolism, reflecting the ability to produce energy under critical thermal conditions as predicted by the OCLTT principle (Zakhartsev et al., 2004).
Comparative studies of LDH have explored certain lineages of ectothermal vertebrates and invertebrates from various thermal niches, although a phylogenetic approach has only been implemented in the latter. In response to increased temperature, vertebrate kinetic patterns show decreased binding capacity and increased catalytic efficiency (Fields and Somero, 1998; Hochachka and Somero, 2002; Somero, 2003, 2004). LDH activity tends to increase at higher temperatures as a consequence of the augmented energy available for the conformational changes required during catalysis (Dunn et al., 1991; Fields, 2001; Hochachka and Somero, 2002). These kinetic properties of ectothermal vertebrate enzymes reflect a highly competent or occasionally a marginally functional LDH, depending on thermal regime. In contrast, in invertebrates, particularly exemplified by species of the anomuran genus Petrolisthes, LDH thermal stability is not affected by maximum habitat temperature, suggesting likely phylogenetic constraints on interspecific variability in LDH stability (Stillman and Somero, 2001).
Considering environments that offer a wide thermal landscape, the continental shelves of the Americas are divided into sixteen zoogeographic provinces. These have been defined based on “part of the neritic zone with a relatively narrow range of temperatures where the fauna shows certain homogeneity,” and particularly on oceanographic characteristics like water temperature and ocean currents (Boschi, 2000a,b; Boschi and Gavio, 2005). The eastern coast of South America embraces a significant thermal gradient and includes three provinces (Figure 1). The Brazilian province, between the mouth of the Orinoco river, Venezuela (9°N) and Cabo Frio, Rio de Janeiro, southeastern Brazil (23°S) (Briggs, 1974; Boschi, 2000a,b) in which mean surface water temperatures vary between 20.7 and 30.5°C (median = 26°C). The Argentinian province, between Cabo Frio and Rawson, Chubut, central Argentina (43–44°S) (Cooke, 1895; Boschi, 2000a), which exhibits temperatures between 7.0 and 27.5°C (median = 15°C). And the Magellanic province, between Rawson and Tierra del Fuego (55°S), Patagonian Argentina (Carcelles and Williamson, 1951; Balech, 1954; Boschi, 2000a,b) in which temperatures vary between 4.0 and 18.0°C (median = 9°C). These ranges represent the minimum and maximum mean monthly temperatures registered between 2009 and 2019 at the northern and southern geographical limits of each province (National Oceanic and Atmospheric Administration [NOAA] and U.S. Department of Commerce, 2019).
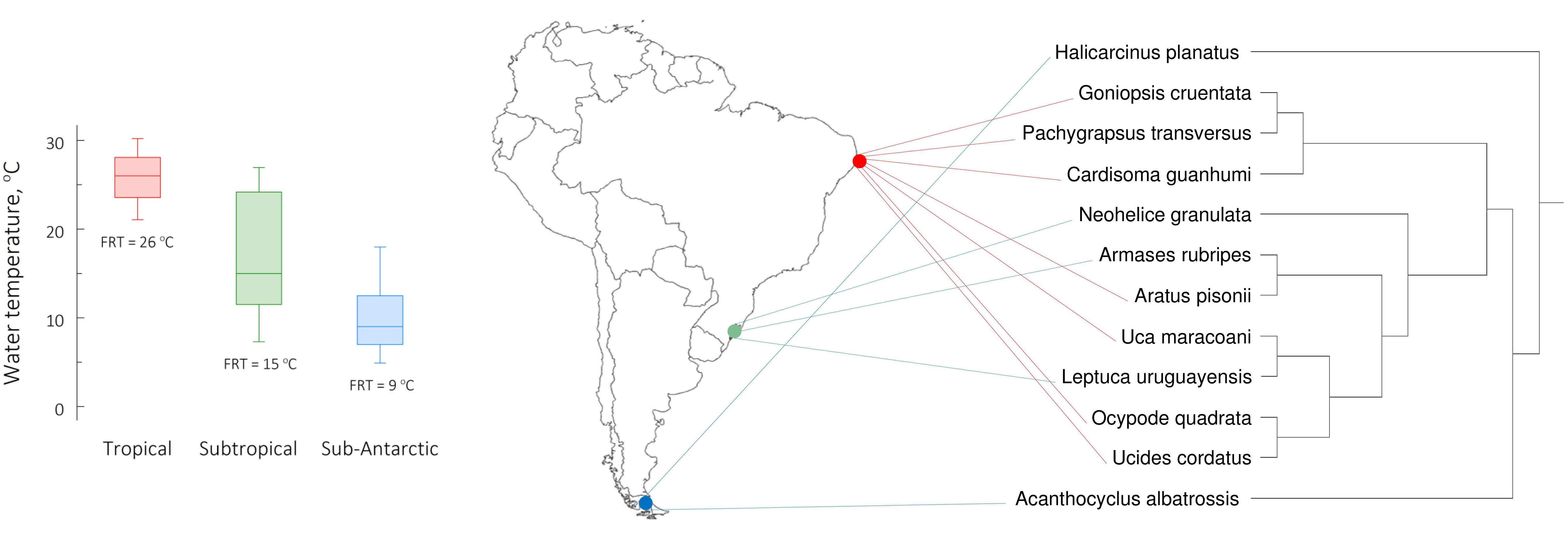
Figure 1. Mean, monthly, maximum and minimum surface seawater temperatures registered between 2009 and 2019 (National Oceanic and Atmospheric Administration [NOAA] and U.S. Department of Commerce, 2019) (left panel) at the northern and southern geographical limits, respectively, of each zoogeographical province along the eastern Atlantic coast of South America (sensu Boschi, 2000a). Surface seawater temperatures varied between 20.7 and 30.5°C for the Brazilian province, 7.0 and 27.5°C for the Argentinian province, and 4.0 and 18.0°C for the Magellanic province. The horizontal line within each box indicates the median temperature while the box boundaries show the interquartile values. Whiskers indicate the lowest and highest temperatures (range). Collecting site locations are indicated within each zoogeographical province (middle panel). The temperatures 26, 15, and 9°C represent the field reference temperatures (FRT) at which the crabs from each province were acclimated. The tropical species (Aratus pisonii, Cardisoma guanhumi, Goniopsis cruentata, Ocypode quadrata, Pachygrapsus transversus, Uca maracoani, and Ucides cordatus) were collected from the Brazilian province (northeastern Brazil, ≈7.8°S, 34.8°W, red). The subtropical species (Armases rubripes, Neohelice granulata and Leptuca uruguayensis) were collected from the Argentinian province (southern Brazil, ≈32.1°S, 52.1°W, green). The sub-Antarctic species (Acanthocyclus albatrossis and Halicarcinus planatus) were collected from the Magellanic province (southern Patagonia, ≈53.2°S, 67.2°W, blue). The phylogenetic relationships among the species investigated (right panel) were generated employing a maximum likelihood search method using the mitochondrial 16S ribosomal gene as a marker (see Faria et al., 2017).
We have shown (Faria et al., 2017) that the micro-habitat temperatures (MHT) of some crab species from the Brazilian and Argentinian zoogeographical provinces are similar, but are higher than those of the Magellanic species. The upper (UL50) and lower (LL50) critical thermal limits of these crabs are subject to different environmental pressures, and exhibit unique evolutionary histories. The UL50 show phylogenetic signal and correlate with micro-habitat temperature, while the LL50 are more plastic and differ markedly among all provinces, revealing an asymmetrical evolutionary history of the critical thermal limits.
In the present study, we now investigate physiological and evolutionary patterns of thermal tolerance in this crab clade (Faria et al., 2017), characterizing the effects of critical thermal limits (LL50 and UL50) on selected descriptors of aerobic and anaerobic metabolism at the systemic and kinetic levels. Using 12 intertidal crab species distributed across the three thermal provinces from northern Brazil to southern Patagonia, we tested for: (i) the influence of shared ancestry and thermal province on metabolic evolution; (ii) the existence of functional trade-offs among aerobic and anaerobic metabolism and LDH kinetics in shaping thermal tolerance; and (iii) the presence of evolutionary convergences and adaptive peaks in the crab phylogeny. This study considers that species’ traits are linked to each other through space and time, embracing most dimensions of physiological evolution.
Materials and Methods
Crab Sampling and Laboratory Maintenance
Approximately 70 adult, intermolt, male or female crabs of each of the 12 species were collected during the morning, at low tide, from mangroves, salt marshes, and sandy or rocky beaches, at the ends of the summers of 2013 and 2014. Collection sites included the Brazilian, Argentinian and Magellanic zoogeographical provinces (Boschi and Gavio, 2005; Figure 1), which correspond roughly to the Tropical, Subtropical and sub-Antarctic physiographical zones.
In the laboratory, the crabs were maintained prior to experiments for 5 days in plastic boxes, each containing a thin layer of seawater (≈3 mm deep, 30 ‰S) to allow gill wetting, in incubators or in water baths (Fanem 347 DCG; Solab SL 224; Polystat 12002, Palmer Instrument Company) at the respective field reference temperatures (FRT) of each zoogeographical province (see above). These temperatures represent the median of the mean monthly minimum and maximum temperatures registered at the northern and southern geographical limits of each province (sensu Boschi, 2000a; National Oceanic and Atmospheric Administration [NOAA] and U.S. Department of Commerce, 2019). This protocol also minimized possible intrinsic physiological variability related to the species’ vertical positions within the intertidal habitat. The crabs were not fed during the experiments, and the seawater was replaced daily in the morning.
The tropical species (Aratus pisonii H. Milne Edwards, 1837; Cardisoma guanhumi Latreille, 1825; Goniopsis cruentata Latreille, 1803; Ocypode quadrata Fabricius, 1787; Pachygrapsus transversus Gibbes, 1850; Uca maracoani Latreille 1802–1803; and Ucides cordatus Linnaeus, 1763) were collected from beaches or mangroves on Ilha de Itamaracá or Itapissuma, Pernambuco (northeastern Brazil, ≈7.8°S, 34.8°W). They were held at the FRT of 26°C (Figure 1) on a 12 h light/12 h dark photoperiod at the Universidade Federal de Pernambuco (Recife, Brazil).
The subtropical species (Armases rubripes Rathbun, 1897; Neohelice granulata Dana, 1851; and Leptuca uruguayensis Nobili, 1901) were collected from beaches near Rio Grande, Rio Grande do Sul (southern Brazil, ≈32.1°S, 52.1°W). They were held at the FRT of 15°C (Figure 1) on a 14 h light/10 h dark photoperiod at the Universidade Federal do Rio Grande (Rio Grande, Brazil).
The sub-Antarctic representatives (Acanthocyclus albatrossis Rathbun, 1898; and Halicarcinus planatus Fabricius, 1775) were collected from stony beaches near Ushuaia, Tierra del Fuego (southern Argentina, ≈53.2°S, 67.2°W). They were held at the FRT of 9°C (Figure 1) on a 14 h light/10 h dark photoperiod, at the Centro Austral de Investigaciones Científicas, Consejo Nacional de Investigaciones Científicas y Técnicas (Ushuaia, Tierra del Fuego, Argentina).
Critical Thermal Limits
Lower (LL50) and upper (UL50) critical thermal limits and microhabitat temperatures for each of the species evaluated here have been published previously (Table 1; Faria et al., 2017). Both limits define the temperatures at which “the animal loses its ability to escape from conditions that will promptly lead to its death” (Cowles and Bogert, 1944). At each limit, 50% of the crabs were unable to right themselves when placed in a supine position. This inability reflects the loss of key motor functions and/or potentially lethal, disorganized locomotory activity (Faria et al., 2017).
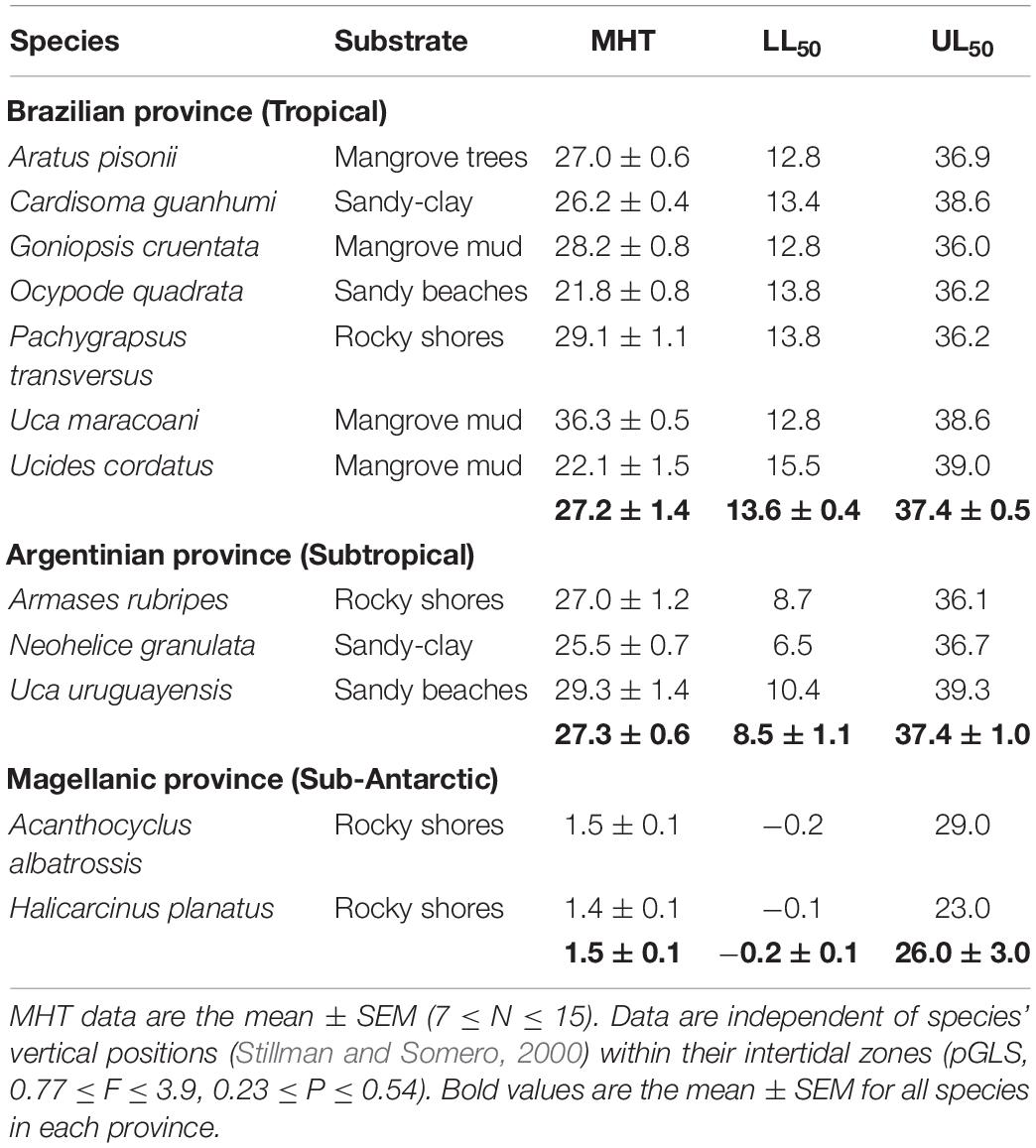
Table 1. Crab species collected from different zoogeographical provinces along the eastern Atlantic coast of South America, providing their microhabitat temperatures (MHT) and lower (LL50) and upper (UL50) critical thermal limits (all in °C) (modified from Faria et al., 2017).
In brief, LL50 and UL50 were established (8 ≤ N ≤ 10 crabs per temperature and species) by direct transfer of the crabs to various lower or higher temperatures, respectively, from their respective FRT’s (26°C for the tropical, 15°C for the subtropical, and 9°C for the sub-Antarctic species). The crabs were examined for 30 s every 30 min during a 6 h period. This duration of exposure represents “the mean emergence time of a crab at low tide” (Faria et al., 2017) and constitutes the mean duration of exposure to aerial temperatures during low tide.
This experimental design, i.e., direct exposure from the respective FRT to the LL50 or UL50 of each species, enabled quantitative measurement of the critical thermal limits of each species, in line with Faria et al.’s (2017) protocol. It thus allowed direct comparison and straightforward correlations between the critical thermal limits and the physiological traits evaluated.
Mass-Specific Oxygen Consumption
Individual crabs (10 ≤ N ≤ 15) were placed into acrylic respirometer chambers containing a 3 mm deep layer of seawater (30 ‰S), constructed specifically to accommodate each species with regard to diameter and volume. The respirometers were held in incubators or water baths (Fanem 347 DCG; Solab SL 224; Polystat 12002, Palmer Instrument Company) at the respective FRT of each species for a 12 h adjustment period prior to beginning the measurements. Each respirometer was coupled by plastic Crystal tubing to a multiplexing manometer and a gas analyzer (FoxBox-C Field Oxygen & Carbon Dioxide Analysis System, Sable Systems International Inc.), and perfused with an airflow of from 150 to 600 mL min–1, depending on respirometer volume, standardized for each species. The standardized airflow provided a baseline percentage of atmospheric oxygen (20.8% O2) in the absence of ambient pressure variation owing to the automated, barometric pressure compensation system.
After the 12 h adjustment period at the FRT, the respirometers were transferred to an incubator or water bath to measure O2 consumption at the LL50 or UL50 temperatures established previously for each species, using a stop-flow respirometric protocol (Steffensen, 1989). After a 4 h period of continuous airflow, the respirometers were sealed by closing their respective manometer valves for a ≈2 h period. The O2 respired by each crab in each respirometer was then measured by recording the decrease in percentage oxygen compared to the pre-established baseline reading while renewing the airflow during a 6 min period of flushing to the gas analyzer. Since measurements were conducted at the critical thermal limits, some crabs died during the measurements. Only the lowest O2 consumption measurements recorded from at least 5–7 crabs alive at the end of each experiment were used.
After each experiment, each crab was cryoanesthetized in a freezer and killed by immersion for 20 min in crushed ice. The crabs were then dried in an oven at 60°C for up to 72 h, and their dry masses were measured using an analytical balance (±1 mg precision).
Data acquisition software (Expedata, Sable Systems International Inc.) was used to calculate the integral of the area corresponding to the decrease in percentage oxygen (IA) in the flushed air. The individual mass-specific oxygen consumption rate (QO2) for each crab was obtained from the formula:
where IA is oxygen percentage area; f, airflow rate (150–600 mL min–1 through each respirometer during the open phase); m, dry mass; t, duration of sealed phase. The factors “100” and “60” convert O2 percentage to O2 volume, and minutes to hours, respectively.
The effect of body mass on QO2 was removed by transforming the QO2 measurements into log10 values, which were then regressed against the respective dry masses. The residues of this regression were then employed, thus ensuring mass-free data.
Extraction of Hemolymph and Abdominal Muscle Samples
Specimens (10 ≤ N ≤ 15) of each crab species were transferred from their respective FRT’s to their respective LL50 and UL50 temperatures in incubators or water baths. After a 6 h exposure period at each thermal limit, a 50 μL hemolymph sample was drawn through the arthrodial membrane at the base of the last pereiopod of the surviving crabs using a #27-5 gauge needle coupled to an insulin syringe. Approximately 50 mg of abdominal muscle were also dissected. All samples were frozen immediately in liquid nitrogen and transferred in dry ice to the Laboratory of Crustacean Physiology at the University of São Paulo in Ribeirão Preto where they were stored at −80°C until analyses could be performed.
Hemolymph Lactate
Perchloric acid (6%) was added to each hemolymph sample (3:1, v/v), followed by centrifugation at 10,000 g for 5 min at 4°C. The supernatant was neutralized with 5 M K2CO3 (10:1, v/v) and re-centrifuged. For microplate reading, an aliquot of each sample was added to a buffer containing 0.5 M glycine, 0.4 M hydrazine and 10 mM EDTA at pH 9.0. 1 mM NAD+ (final concentration) and 20 U/mL LDH (final concentration) were added to each well, and the microplate was incubated at 25°C for 1 h in the dark and read at 340 nm (Bergmeyer and Bernt, 1974; Santos and Keller, 1993; Henry et al., 1994). The lactate concentration (mg L–1) was obtained from the formula:
where A, Absorbance; 0.3, final fluid volume in each well; 6.22, molar extinction coefficient of NADH at 340 nm; 0.874, well optical path; 0.015, sample volume in each well; 3.4, sample dilution factor; and 90.1, conversion factor from mmol L–1 to mg L–1.
Temperature Coefficient
The effect of temperature on QO2 (aerobic sensitivity) and on lactate concentration (anaerobic sensitivity) was expressed using the formula Q10 = (k1/k2)10/(t1–t2), where k1 and k2 are the mass specific oxygen consumption rates at temperatures t1 and t2, respectively, where t1 > t2. Q10O2 and Q10Lac represent the temperature coefficients for changes in QO2 and hemolymph lactate, respectively.
Muscle Lactate Dehydrogenase Kinetics
Each abdominal muscle sample was homogenized (Eurostar Power-B, IKO Labortechnik) on crushed ice at 1,500 rpm for 60 s in 50 mM K2HPO4 buffer (7:1 v/m, mL g–1, unadjusted pH) (modified from Zietara et al., 1996). The homogenate was then centrifuged at 14,000 g for 40 min at 4°C and the supernatant was separated for enzyme kinetic assays. The LDH assays (1 ≤ N ≤ 4, pools of samples from 2 to 3 individual crabs) were performed using 100 mL of supernatant diluted from 10 to 2,000 times, depending on condition and species. This assay evaluates the oxidation of NADH to NAD+ assuming steady-state conditions such as the linear relationship between NAD+ formation and time (Segel, 1976). Controls without added homogenate were conducted to evaluate spontaneous NADH oxidation.
LDH activity was measured continuously, accompanying the oxidation of NADH to NAD+ at 340 nm in thermostatted cells (ε340 nm, pH 6.9 = 5,860 mM–1cm–1; Cary 60 UV-Vis Spectrophotometer, Agilent Technologies) at both 25°C and at the respective FRT, LL50, and UL50 temperatures of each crab species for up to 5 min. LDH activity was not measured at the LL50’s of Acanthocyclus albatrossis and Halicarcinus planatus owing to the inability of the spectrophotometer to read at these low temperatures (≈-1°C).
For the kinetic assays, up to 14 pyruvate concentrations ranging from 1 μM to 7 mM were used in 80 mM Imidazole-HCl buffer at pH 6.9, containing 150 μM NADH in a final volume of 1 mL (modified from Stillman and Somero, 2001). The pH of the Imidazole buffer was adjusted according to the temperature of each assay. The kinetic parameters, i.e., maximum LDH activity (Vmax, U mg–1), the Michaelis-Menten constant for pyruvate (KmPyr, mM) and the catalytic coefficient (min–1 103) were calculated using the saturation curve for LDH activity against pyruvate concentration, employing the SigrafW application (Leone et al., 2005). KmPyr describes the enzyme-substrate affinity, representing the pyruvate concentration at which LDH activity is Vmax/2, indicating the ability to form an enzyme-substrate complex. The catalytic coefficient was measured at the FRT and was calculated for 25°C using a Q10 of 2, reflecting a measure of the rates of enzyme-substrate formation and dissociation (Segel, 1976; Northrop, 1998) and, thus, the rate constant for transforming pyruvate (substrate) into lactate (product). Vmax also was calculated for 25°C, using a Q10 of 2. One enzyme unit (U) is defined as the amount of LDH that hydrolyzes 1 mmol of NADH per minute. Assays were performed in duplicate aliquots.
Total protein titers in the homogenates were measured following Read and Northcote (1981), using bovine serum albumin as the standard.
Statistical Analyses
For the intra-specific analyses, the effects of critical thermal limits on the systemic parameters QO2, lactate concentration and their respective Q10’s, and on the kinetic parameters Vmax and KmPyr, for each species, were evaluated using a one-way Analysis of Variance followed by the Student-Newman-Keuls (SNK) multiple means comparison procedure to detect differences among groups (P ≤ 0.05). Data are expressed as the mean ± SEM.
Inter-specific comparisons were conducted based on a molecular phylogenetic analysis of 36 species, performed by a maximum likelihood procedure using the 16S ribosomal gene (Faria et al., 2017), a marker commonly used in phylogenetic studies of decapod Crustacea (e.g., Schubart et al., 2000; Mantelatto et al., 2009a,b; Pileggi and Mantelatto, 2010). Briefly, the phylogenetic analysis was undertaken using the Maximum Likelihood search method (Felsenstein, 1973, 1981) developed in RAxML 7.2.7 (Stamatakis, 2006) and implemented on the CIPRES system, employing the GTR + ∫ + I substitution model (Tavaré, 1986). A rapid bootstrap method (1,000 replicates) (Stamatakis et al., 2008) was used to evaluate topology consistency, only confidence values greater than 50% being considered. The original tree was pruned, maintaining only those species physiologically evaluated here. Branch lengths reflect genetic divergence.
The hypothesis that zoogeographical province may have affected the evolution of the physiological traits at the FRT, LL50, or UL50 was tested using Phylogenetic Generalized Least Squares (PGLS) models. PGLS assumes that the residual variation among species is correlated owing to their shared ancestry (Grafen, 1989; Garland and Ives, 2000; Lavin et al., 2008), following a hierarchical disposition through time. Since character evolution is mathematically modeled along each branch of the phylogeny, we tested two evolutionary models simultaneously for each PGLS, employing the α parameter. The α-value is a measure of the selection strength that constrains a trait toward an optimum value: unconstrained character evolution (α = 0) is best fitted by the “Brownian motion” evolutionary model, while trait evolution with weakly limited or unlimited minimum and maximum values is best represented by the “Ornstein-Uhlenbeck” (O-U) model of evolution (Revell, 2010). This procedure was performed to ensure the best-adjusted evolutionary correlation for each set of inter-specific data.
Phylogenetic signal, a measure of the tendency of closely related species to resemble each other more than those drawn randomly from a clade, was calculated using Abouheif’s test (Abouheif, 1999; Pavoine et al., 2008). An independent Monte Carlo test was conducted for each trait to provide its Moran’s I index and respective P-value. Moran’s I varies from −1 to +1, revealing physiological dissimilarities and similarities, respectively, between closely related species (Gittleman and Kot, 1990; Diniz-Filho, 2001).
Overall physiological variability was analyzed using a phylogenetic principal components analysis (pPCA; Jombart et al., 2010). The phylogenetic structure of the multivariate comparative data was obtained by including all measured traits simultaneously (except Vmax and KmPyr for the sub-Antarctic species where data were unavailable), including microhabitat temperature and both critical thermal limits, for all species. The scores of the two main dimensions (PC1 and PC2) for each species were then plotted against the phylogeny.
We also tested for the presence of convergent evolutionary events using the SURFACE method (Ingram and Mahler, 2013) to evaluate whether the hypothesis of physiological shifts among the three zoogeographic provinces is more likely than that expected by chance. Comparisons among several simulated O-U models were performed using the stepwise Akaike Information Criterion (AIC) procedure, and all improvements in AIC values were considered.
Comparative analyses were conducted using the R environment (R Development Core Team, 2019), specifically ape (Paradis et al., 2004), nlme (Pinheiro et al., 2015), phytools (Revell, 2012), adephylo (Jombart et al., 2010), and SURFACE (Ingram and Mahler, 2013) packages. P-values were set at 0.05.
Our findings can be considered free of bias from the species’ vertical positions within their intertidal zones since this parameter had no effect on any physiological trait (pGLS ANOVA, 0.77 ≤ F ≤ 3.9, 0.23 ≤ P ≤ 0.54). Our characterization of the vertical distributions assumed the five categories distinguished by Stillman and Somero (2000).
Results
Thermal Effects on Systemic Metabolism: QO2, Hemolymph Lactate and Q10
The subtropical species Neohelice granulata and Armases rubripes exhibited the lowest (0.01 ± 0.00 mLO2 g–1 h–1) and highest QO2’s (0.97 ± 0.08 mLO2 g–1 h–1) at their respective LL50 (6.5°C) and UL50 (36.1°C) (Table 2 and Figure 2). Both these species also showed the most sensitive QO2’s (Q10O2’s of 3.7 and 3.1, respectively). Interestingly, the sub-Antarctic species Acanthocyclus albatrossis and Halicarcinus planatus at the FRT of 9°C exhibited higher QO2’s (≈0.11 mLO2 g–1 h–1) than all the subtropical representatives (Armases rubripes, N. granulata and Leptuca uruguayensis [≈0.06 mLO2 g–1 h–1]) at the FRT of 15°C (Table 2).
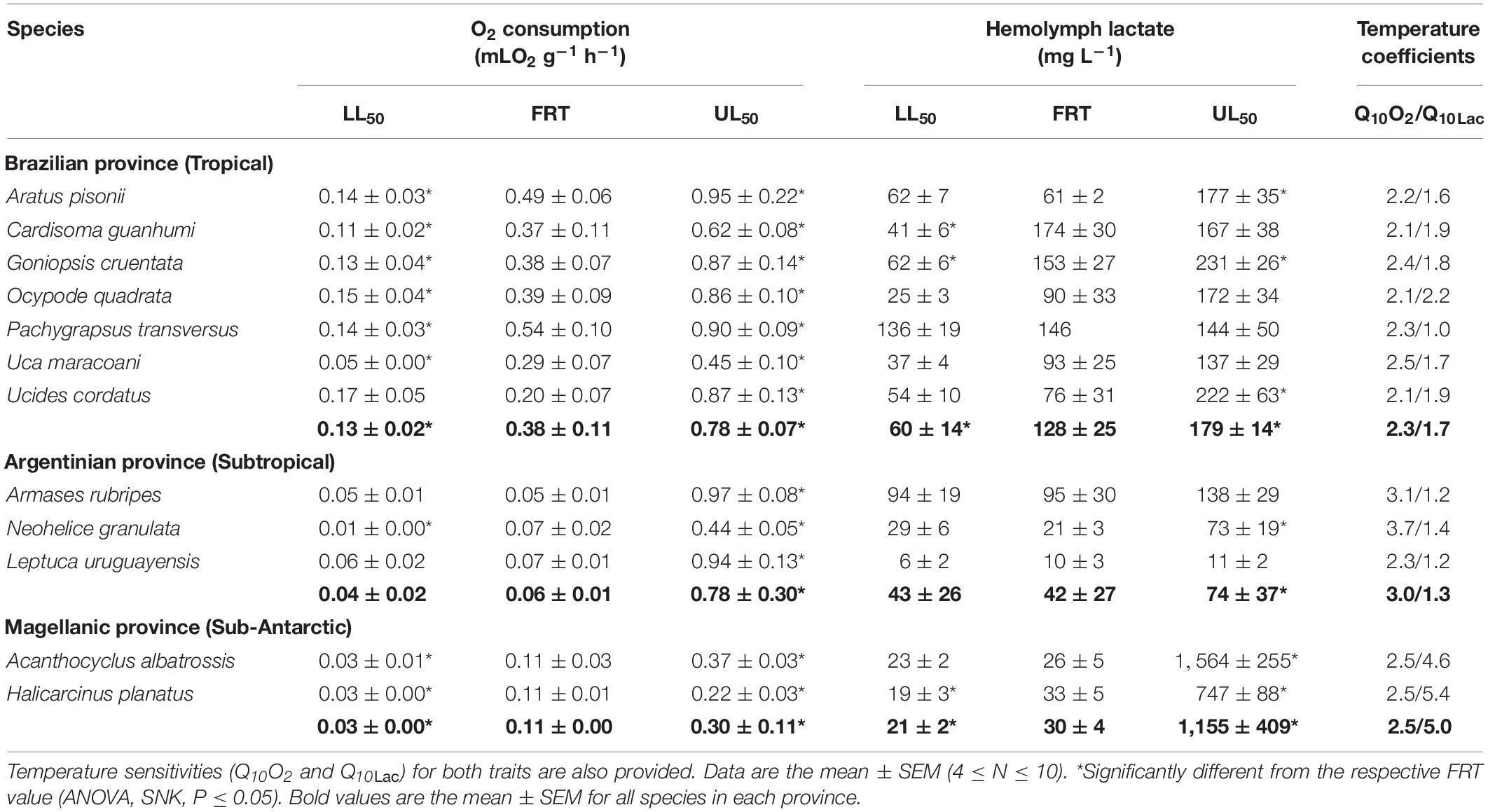
Table 2. Crab species and their systemic physiological parameters, mass-specific oxygen consumption rate (mL O2 g–1 h–1) and hemolymph lactate concentration (mg L–1), measured at their respective field reference temperatures (FRT) and lower (LL50) and upper (UL50) critical thermal limits.
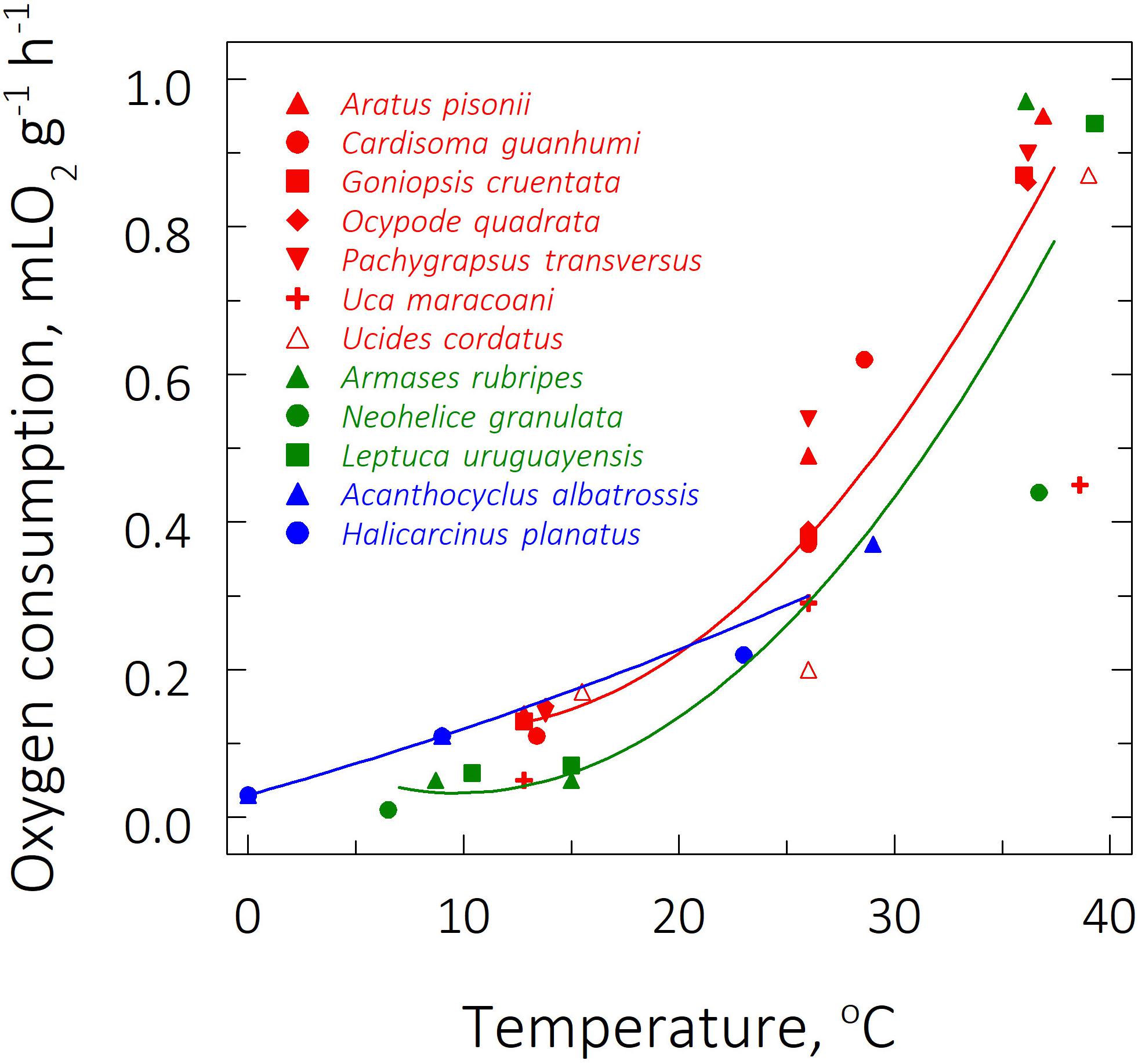
Figure 2. Effect of direct transfer of tropical (red), subtropical (green), and sub-Antarctic (blue) crab species on mass-specific oxygen consumption rate (QO2) after 6 h exposure to their respective upper and lower critical thermal limits, from their corresponding field reference temperatures of 26, 15, and 9°C. Data (mL O2 g–1 h–1) are mean values (6 ≤ N ≤ 9) and were adjusted to a second degree polynomial curve.
The lowest hemolymph lactate concentration ([Lac]) was found in the subtropical species Leptuca uruguayensis (6 ± 2 mg/L) at its LL50 of 10.4°C. The sub-Antarctic species A. albatrossis showed the highest concentrations (1,564 ± 255 mg/L, respectively) at its UL50 of 29°C (Figure 3). Q10Lac values consistently fell between 1.2 and 1.9, the highest values being found in the sub-Antarctic species, particularly H. planatus (5.4) (Table 2).
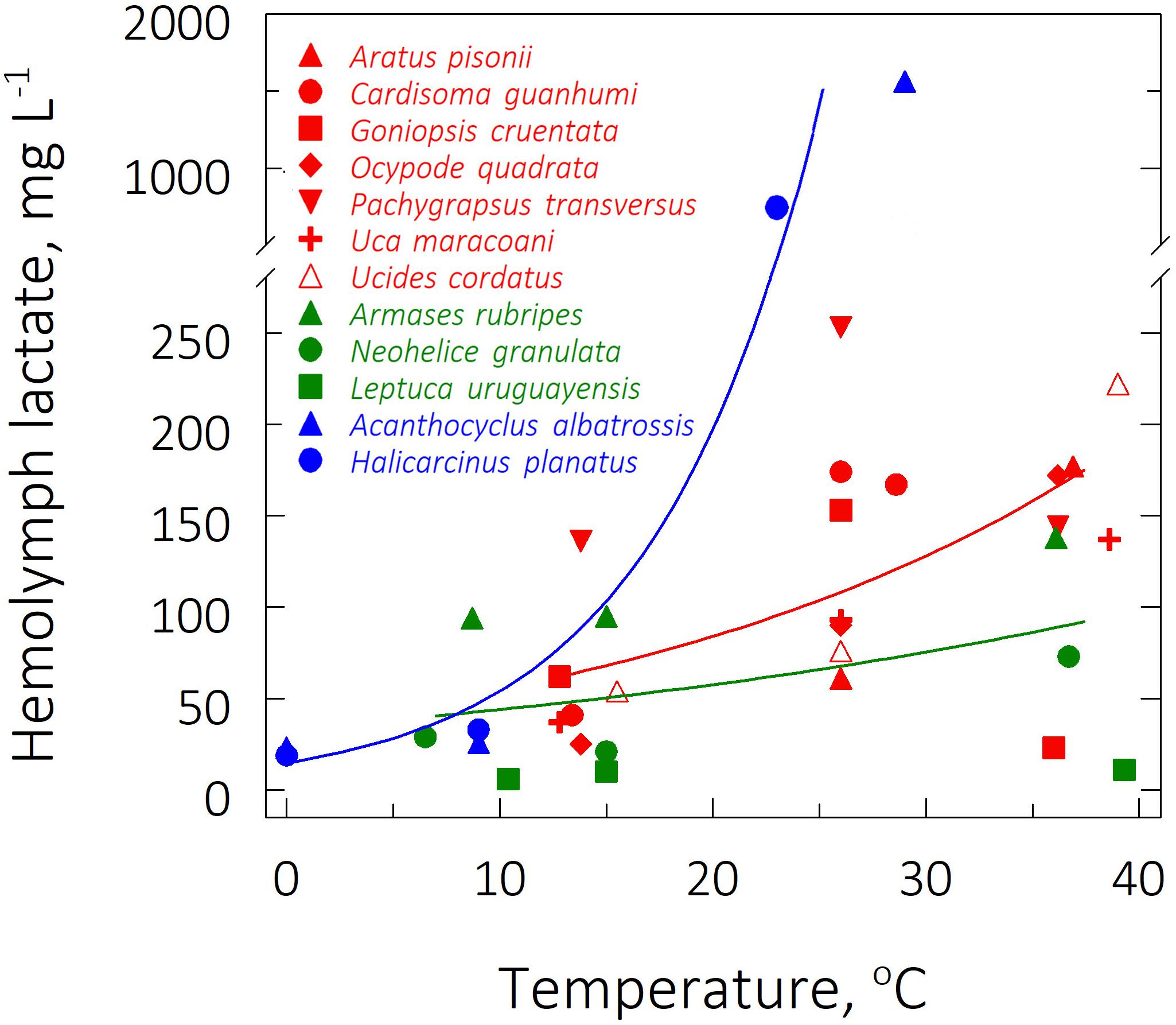
Figure 3. Effect of direct transfer of tropical (red), subtropical (green) and sub-Antarctic (blue) crab species on hemolymph lactate concentration after 6 h exposure to their respective upper and lower critical thermal limits, from their corresponding field reference temperatures of 26, 15, and 9°C. Data (mg L–1) are mean values (4 ≤ N ≤ 7), and were adjusted to a second degree polynomial curve.
Critical temperatures affected mean QO2 (ANOVA 20 ≤ F ≤ 46, P ≤ 0.004) and mean hemolymph [Lac] (ANOVA, 16 ≤ F ≤ 25, P ≤ 0.05) in the tropical and Sub-Antarctic species. These traits increased at the respective mean UL50’s of ≈37 and ≈26°C and diminished at the mean LL50’s of ≈14°C and ≈0°C, both compared to the FRT’s of 26 and 9°C, respectively (SNK, P ≤ 0.05) (Table 2). However, for the subtropical representatives, the mean QO2’s and mean hemolymph [Lac] were similar when comparing these rates at the FRT of 15°C and the mean LL50 of ≈9°C (SNK, P = 0.23), demonstrating that metabolism was unchanged during cooling. Values were higher, however, at the mean UL50 of ≈37°C (SNK, P ≤ 0.05).
Evolutionary and Comparative Patterns
Zoogeographical province affected the evolution of QO2 under all three thermal regimes (pGLS ANOVA, 9 ≤ F ≤ 18, P ≤ 0.02, 0.14 ≤ α ≤ 21). At the FRT of 26°C, tropical species showed a higher mean QO2 (0.38 ± 0.11 mLO2 g–1 h–1) than subtropical (0.06 ± 0.01 mLO2 g–1 h–11) and sub-Antarctic (0.11 ± 0.00 mLO2 g–1 h–1) species, which also differed between their respective FRT’s of 15 and 9°C, respectively (phyHolm-Bonferroni, P = 0.05) (Table 2). Further, mean QO2’s at the critical temperature limits showed different evolutionary patterns. Mean QO2 at the mean UL50 was lower in the sub-Antarctic species (0.30 ± 0.11 mLO2 g–1 h–1) than in the tropical (0.88 ± 0.13 mLO2 g–1 h–1) and subtropical (0.78 ± 0.3 mLO2 g–1 h–1) representatives (phyHolm-Bonferroni, P = 0.02) (Figure 4). However mean QO2 was similar (≈0.07 mLO2 g–1 h–1) for all provinces at the mean LL50 (phyHolm-Bonferroni, P ≥ 0.68). The evolution of Q10 was not driven by thermal regime (pGLS ANOVA, F ≤ 3.3, P ≥ 0.1), showing a mean sensitivity of 2.5 (Table 2).
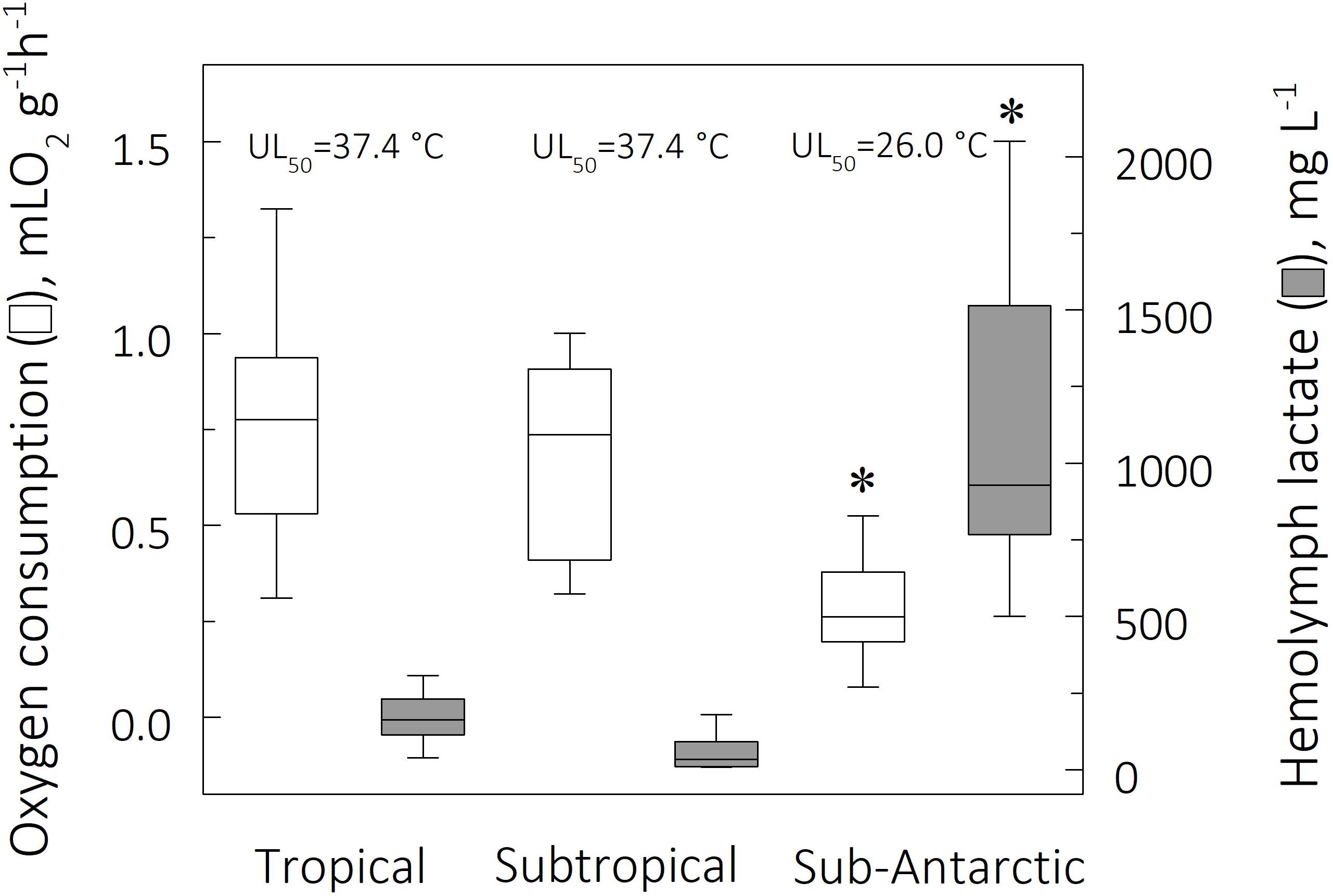
Figure 4. Mean mass-specific oxygen consumption (QO2, mL O2 g–1 h–1) and mean hemolymph lactate concentrations (mg L–1) for tropical, subtropical and sub-Antarctic crab species at their respective UL50, after 6 h direct transfer from their corresponding field reference temperatures of 26, 15, and 9°C. The horizontal line within each box indicates the median QO2 while the box boundaries show the interquartile values. Whiskers indicate the lowest and highest QO2’s (range). Specimens (12 ≤ N ≤ 41 per species) for all species sampled from each zoogeographical province were used to provide the plots. *Significantly different from tropical and subtropical species (phyHolm-Bonferroni, P ≤ 0.05).
In contrast, zoogeographical province affected the evolution of hemolymph [Lac] only at the mean UL50 (pGLS ANOVA, F = 7.9, P ≤ 0.05, 44 ≤ α ≤ 47). Mean hemolymph [Lac] for the sub-Antarctic species was greater (1,155 ± 409 mg L–1) than those of the tropical (179 ± 14 mg L–1) and subtropical (74 ± 37 mg/L) representatives (phyHolm-Bonferroni, P ≤ 0.05), the values for the latter groups being similar (phyHolm-Bonferroni, P ≥ 0.05) (Table 2 and Figure 4). Mean hemolymph [Lac] at the mean LL50 (49 ± 11 mg L–1, all species) and at the FRT (90 ± 21 mg L–1, all species) were similar among provinces (phyHolm-Bonferroni, P ≥ 0.05). Mean Q10’s for hemolymph lactate mirrored this similarity (pGLS ANOVA, 7.5 ≤ F ≤ 7.8, P ≤ 0.05), being greater in the sub-Antarctic species (3.9 ± 1.5) than in the tropical (1.7 ± 0.1) and subtropical species (1.3 ± 0.1).
Thermal Effects on Muscle LDH Kinetics: Vmax, KmPyr and Catalytic Coefficient
The highest Vmax was 7.43 ± 0.39 U mg–1 for the tropical species Ucides cordatus at its UL50 of 39.0°C while the lowest activity was 0.09 ± 0.00 U mg–1 in the sub-Antarctic species Halicarcinus planatus at the FRT of 9.0°C (Table 3 and Figure 5). At the zoogeographical province level, the mean Vmax’s for the tropical and subtropical species were strongly sensitive to critical temperature exposure: Vmax’s were higher at the mean UL50 and lower at the mean LL50 (ANOVA; 11.5 ≤ F ≤ 15.2; P ≤ 0.01). The mean Vmax for the sub-Antarctic species was thermally insensitive (ANOVA; F = 1.0; P = 0.4). At an extrapolated temperature of 25°C, mean Vmax values were affected by zoogeographical province (pGLS ANOVA, F = 6.0, P = 0.05, α = 16.9) with calculated values of 1.7 ± 0.3, 1.1 ± 0.1 and 0.6 ± 0.3 U mg–1 for the tropical, subtropical and sub-Antarctic species, respectively (Table 3 and Figure 5, insert).
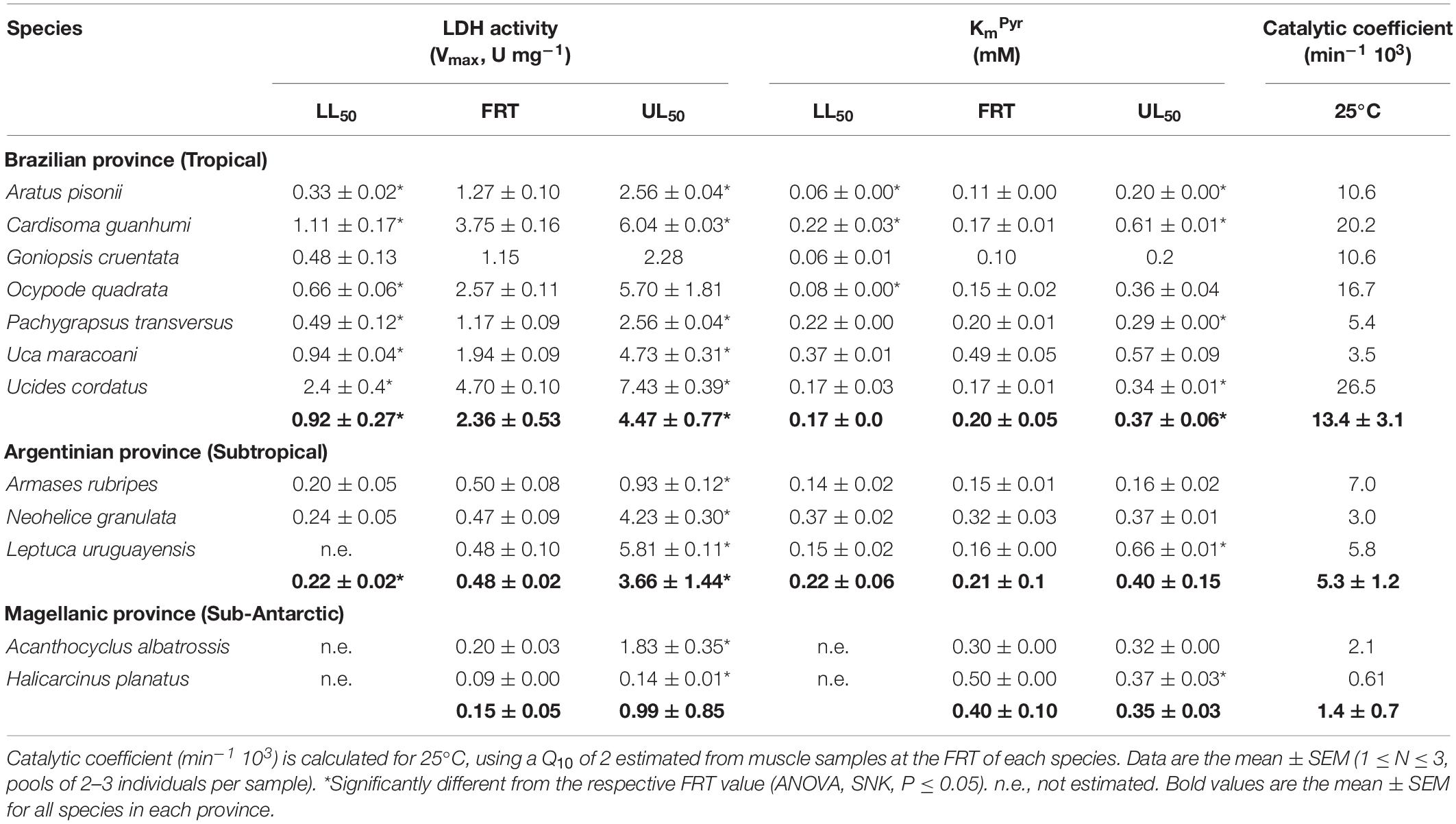
Table 3. Crab species and their kinetic parameters, LDH activity (Vmax, U mg–1) and KmPyr (mM), measured at their respective field reference temperatures (FRT) and lower (LL50) and upper (UL50) critical thermal limits.
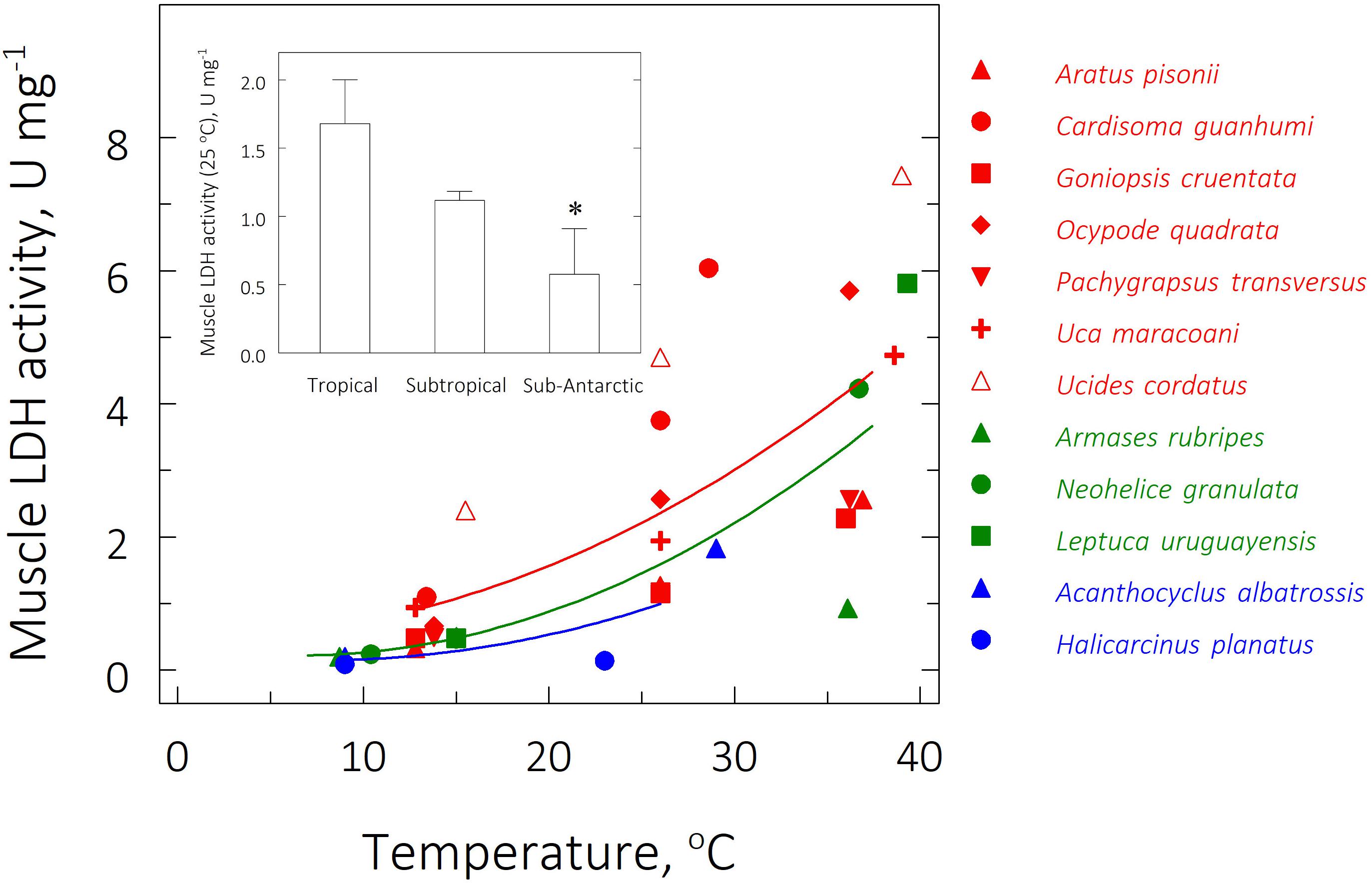
Figure 5. Effect of direct transfer of tropical (red), subtropical (green), and sub-Antarctic (blue) crab species on muscle lactate dehydrogenase (LDH) activity (Vmax, U mg–1) after 6 h exposure to their respective upper and lower critical thermal limits from their corresponding field reference temperatures (FRT) of 26, 15, and 9°C. Data are mean values (2 ≤ N ≤ 3, pools of 3 individuals each) and were adjusted to a second degree polynomial curve. Vmax was measured continuously in duplicate aliquots at the same experimental temperatures. Activities could not be measured for the sub-Antarctic species at their lower critical thermal limits. Insert: Mean (± SEM) muscle LDH activity at 25°C for all species from each province (2 ≤ N ≤ 3, pools of 3 individuals each); muscle samples taken at the FRT of each species. *Significantly different from tropical crab species (phyHolm-Bonferroni, P ≤ 0.05).
Mean KmPyr for the subtropical and sub-Antarctic crab species were not affected by exposure at their critical thermal limits (ANOVA, 0.62 ≤ F ≤ 0.95; P ≥ 0.43) (Table 3). However, mean KmPyr for the tropical species was affected by exposure at the mean UL50 (ANOVA, F = 4.6, P ≤ 0.05; SNK, P ≤ 0.05). The mean KmPyr for the three provinces under all thermal regimes was 0.3 mM (Figure 6). In contrast, the catalytic coefficients calculated for 25°C (3.0–26.5 min–1 103; Figure 5, insert) did regress on microhabitat temperatures (pGLS, −0.62 ≤ slope ≤ −0.64, 5.4 ≤ F ≤ 8.8, P ≤ 0.05, α = 22.9), when the sub-Antarctic species A. albatrossis and H. planatus are excluded from the analysis (see also Table 3).
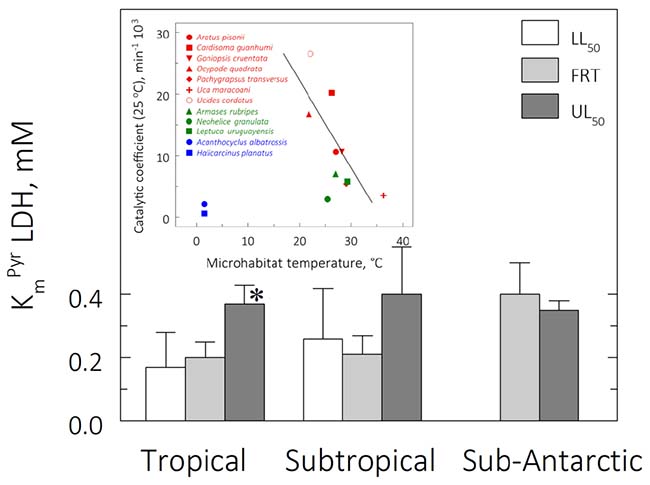
Figure 6. Michaelis-Menten constants (KmPyr, mM) for muscle lactate dehydrogenase (LDH) in tropical, subtropical and sub-Antarctic crab species at their respective lower (LL50) and upper (UL50) critical thermal limits after 6 h direct transfer from the corresponding field reference temperatures (FRT) of 26, 15, and 9°C. Data are the mean ± SEM (2 ≤ N ≤ 3, pools of 3 individuals each). LDH activity was measured continuously in duplicate aliquots at the same experimental temperatures. Measurements could not be made for the sub-Antarctic species at their lower critical thermal limits. *Significantly different from tropical and subtropical crabs (phyHolm-Bonferroni, p ≤ 0.05). Insert: Catalytic coefficient (min–1 103) calculated for 25°C, assuming a Q10 of 2, plotted against the microhabitat temperature.
Evolutionary and Comparative Patterns
Zoogeographic province affected the evolution of muscle LDH activity at the FRT (pGLS ANOVA, 6.1 ≤ F ≤ 7.2, P ≤ 0.03, α = 11.5). Tropical species showed a mean Vmax (2.4 ± 0.5 U mg–1) greater than that of the subtropical (0.5 ± 0.0 U mg–1) and sub-Antarctic (0.2 ± 0.1 U mg–1) species (phyHolm-Bonferroni, P ≤ 0.05) (Table 3). However, there was no effect of province on Vmax at either mean critical limit (pGLS ANOVA, 1.1 ≤ F ≤ 2.6, P ≥ 0.06). Tropical (0.9 ± 0.3 U mg–1) and subtropical representatives (0.2 ± 0.0 U mg–1) showed similar mean Vmax’s at their mean LL50’s (phyHolm-Bonferroni, P ≥ 0.14) and at their mean UL50’s (4.5 ± 0.8 U mg–1 and 3.7 ± 1.4 U mg–1, respectively) (phyHolm-Bonferroni, P ≥ 0.14).
The evolution of KmPyr was not affected by province under any thermal regime (pGLS ANOVA, 0.009 ≤ F ≤ 2.5, P ≥ 0.14) (Figure 6). Mean KmPyr was 0.2 mM at the mean FRT, 0.21 mM at the mean LL50 and 0.37 mM at the mean UL50. However, catalytic coefficient was associated with microhabitat temperature (pGLS ANOVA; −0.62 ≤ slope ≤ −0.64, 5.4 ≤ F ≤ 8, P ≤ 0.05, ΔAIC ≤ 2, α = 22.9), excluding the two outliers from the Magellanic province (Figure 6, insert).
Phylogenetic Signal and Multi-Variate/Dimensional Patterns
With regard to the upper critical limit (UL50), all traits, i.e., QO2, [Lac], Vmax and KmPyr, as well as UL50 itself, showed significant phylogenetic signal (I > 0.28, P < 0.05), thus their inter-specific variability exhibits a phylogenetic pattern. However, all other traits regarding exposure to FRT and LL50 were unrelated to phylogeny (−0.22 ≤ I ≤ 0.19, P ≥ 0.05).
Phylogenetic principal components analysis, grouping all traits, revealed that the first two dimensions accounted for 54.3% of the total physiological variance, specifically 38.1% for PC1 and 16.2% for PC2 (pPCA, Figure 7): PC1 is more related to the sub-Antarctic species while PC2 to the tropical/subtropical clade. This scenario is in agreement with the two adaptive peaks detected in the phylogeny: (i) at the root, and maintained in A. albatrossis and H. planatus; and (ii) a shift at the outset of the tropical and subtropical species (SURFACE analysis, Figure 7). Microhabitat temperature, LL50 and UL50 were the traits that most strongly correlated with the more relevant pPCA dimension (R ≥ 0.30), and also contributed most to the adaptive peaks (−14 < AICc < −8) as evaluated using SURFACE.
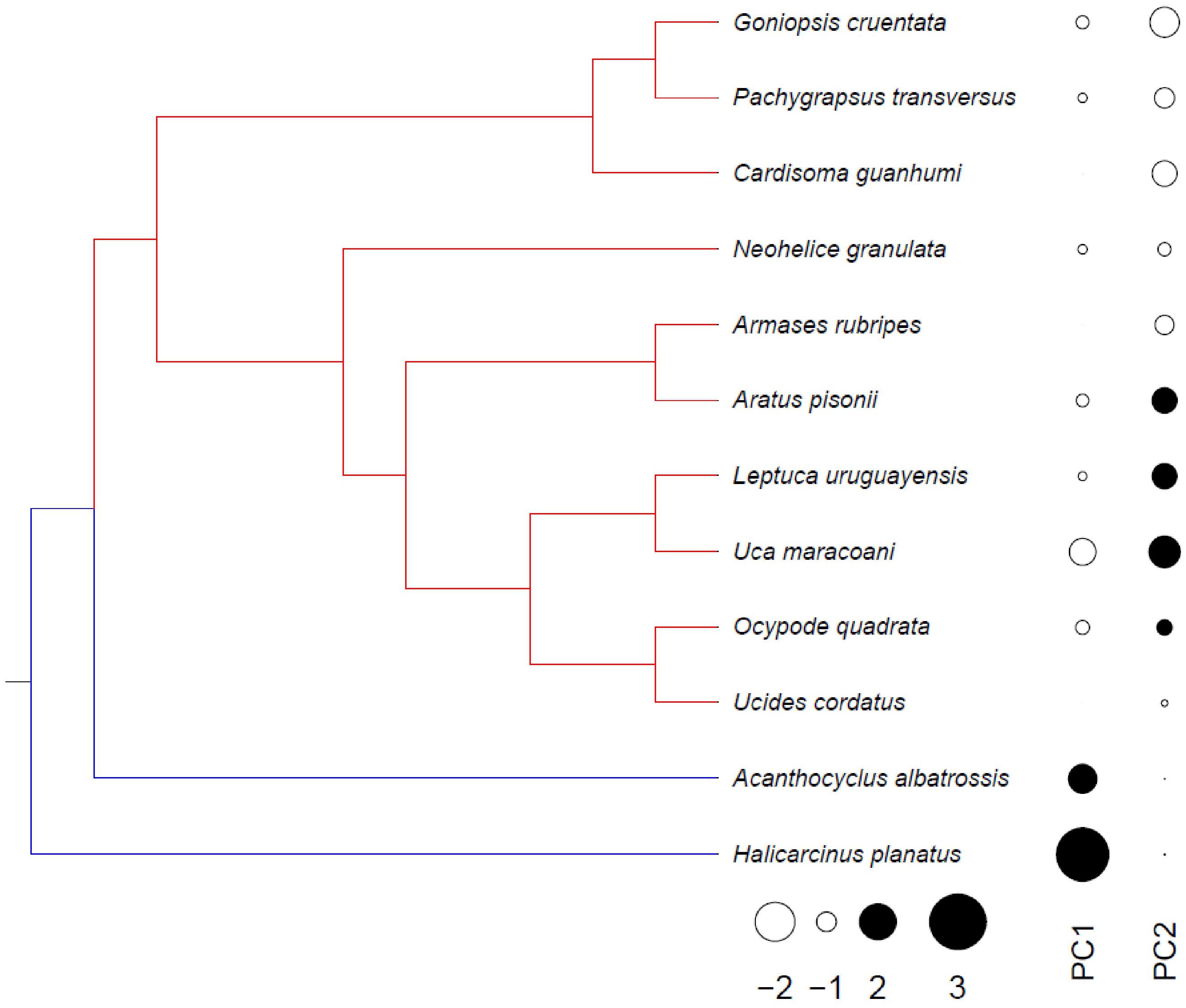
Figure 7. Overall physiological evolution of thermal tolerance, grouping systemic and kinetic traits in intertidal crabs distributed from northern Brazil to southern Patagonia, mapped onto a phylogeny modified from Faria et al. (2017). Different colors represent adaptive peaks identified using the SURFACE method: the first optimum (blue) was detected for the sub-Antarctic species while the second (red) was found in the tropical and subtropical representatives. Using a phylogenetic PCA, the first two dimensions accounted for 54.3% of total physiological variance, specifically 38.1% for PC1 and 16.2% for PC2. The scores of both dimensions follow the same phylogenetic pattern as the adaptive peaks. Microhabitat temperature, LL50 and UL50 were the most important parameters in both analyses (R ≥ 0.30 for pPCA, −14 < AIC < −8 for SURFACE).
Discussion
We found that tropical and subtropical intertidal crab species showed similar subcellular and organismic responses to acute thermal stress, differing from their sub-Antarctic relatives. Oxygen consumption (QO2) and hemolymph lactate ([Lac]) increased with increasing temperature in all species from the three zoogeographical provinces, revealing an apparent trade-off with the evolution of upper critical thermal limit (UL50). While maximum LDH activity (Vmax) also increased with temperature, KmPyr tended to remain constant, whereas the catalytic coefficient is driven by micro-habitat temperature in most cases. Interestingly, all physiological traits at the UL50 have evolved in a phylogenetic fashion, while the others linked to LL50 and FRT were more plastic. Overall metabolic evolution at both critical limits has taken place by two independent adaptive pathways in the sub-Antarctic lineages, however one adaptive peak has been inherited by the northernmost species. These physiological patterns and evolutionary history appear to reflect the species’ ability to function at higher temperatures, together with a lesser capacity to confront lower temperatures, since the crabs were likely acclimatized to the southern summer. The discussion that follows should be considered in this light.
Systemic Metabolism
The systemic metabolism complied with the paradigm that higher temperatures lead to greater energy demands through increased QO2 and [Lac]. The highest values for these parameters were seen at the UL50 while the lowest values occurred at the LL50, both compared to the FRT. At the FRT, the tropical crab species exhibited a mean QO2 6-fold greater than the mean value for the subtropical crabs, and 3-fold greater than the sub-Antarctic representatives. The same phenomenon has occurred in the Echinodermata, Annelida, other Crustacea, and Mollusca: warmer water species show higher rates of oxygen consumption than do colder water species when measured at their respective usual environmental temperatures (Fox, 1936), reaching up to 10-fold greater in crustaceans from tropical waters than in polar species (Scholander et al., 1953). Further, the sub-Antarctic crab species showed a 2-fold higher QO2 at the FRT of 9°C than did the subtropical crabs at the FRT of 15°C, which reveals temperature compensation of aerobic metabolism in cold regions, an adaptive peak probably maintained by strong stabilizing selection as revealed by the higher α-value.
The evolution of temperature compensation of aerobic capacity as observed here may result from the differential regulation of hemolymph Mg2+ as seen in some decapod crustaceans (Frederich et al., 2000; Wittmann et al., 2010, 2012) or may be due to selective pressure on cellular energy demand, as demonstrated in fish (Peck et al., 2002; Pörtner, 2002; Morley et al., 2009). Hemolymph Mg2+ hypo-regulation is associated with higher activity levels in decapods from low temperatures since higher Mg2+ concentrations diminish activity by inhibiting Ca2+ influx across neuronal membranes, slowing down neuromuscular transmission and decreasing muscle contraction (Parnas et al., 1994; Dunn and Mercier, 2003). Elevated activity levels in decapods from naturally or experimentally cold temperatures are due to fairly high heart rates, ventilation frequencies and oxygen consumption, as a consequence of lower Mg2+ levels (Frederich and Pörtner, 2000). Increased mitochondrial densities also contribute to elevated aerobic metabolism in fish acclimated to cold temperatures revealing ultrastructural adjustments (Johnston and Maitland, 1980; Tyler and Sidell, 1984). Both hypotheses, in combination or independently, may explain the higher QO2 values seen in the sub-Antarctic species.
With regard to phylogenetic signal for oxygen consumption, closely related species do not show similar QO2’s at the FRT and LL50, which is also supported by the effect of zoogeographical province on QO2 under both thermal regimes together with a significant effect of selection strength (17 ≤ α ≤ 20). Given the physical dependence of QO2 on the kinetic energy of the molecules, this scenario suggests adaptive evolution strongly driven by thermal regime, regardless of the phylogenetic position of each species. To illustrate, the fiddler crabs sampled, i.e., the tropical species U. maracoani and the subtropical species L. uruguayensis, although closely related, exhibit distinct QO2’s under their natural temperature conditions and on cold challenge. Conversely, aerobic metabolism at the UL50 is similar among closely related species, also reinforced by the absence of a province effect, signifying that inter-specific variability in QO2 at higher temperatures is constrained by shared inheritance, regardless of a significant effect of thermal regime. The maximum aerobic capacities at higher temperature challenge vary among species in a phylogenetic fashion.
With respect to anaerobic metabolism, mean [Lac] in the subtropical crab species did not vary as a function of their respective FRT and LL50, while the tropical and sub-Antarctic crabs showed a reduction of ≈50 and 70%, respectively, in mean [Lac] at the mean LL50 compared to FRT. Since the subtropical species encounter a eurythermic environment owing to the mixing of warm water from the Brazilian Current with colder water from the Malvinas Current (Boschi, 2000a), their stable anaerobic metabolism suggests the capability for cold acclimatization and energy production even during rapid temperature changes such as might occur during exposure at low tide. In contrast, the tropical and sub-Antarctic crabs hail from more stenothermic provinces, dominated respectively by the South Equatorial Current and by sub-Antarctic waters (Boschi, 2000a; Thurman et al., 2013), suggesting that rapid cooling effects are more pervasive and more able to reduce anaerobic energy production. However, species from all three zoogeographical provinces showed an increase in mean [Lac] during exposure at their UL50 compared to the FRT. Mean [Lac] was 1.4- and 1.8-fold higher at the mean UL50 of ≈39°C for the tropical and subtropical species, respectively, but 17-fold higher at the mean UL50 of 26°C for the sub-Antarctic species, revealing a stronger effect of higher temperatures in species acclimatized to a colder province. The lack of mobilization of lactate at the lower critical limits is possibly due to a lower cellular energy demand, which is compatible with the lower kinetic energy of the molecules.
The high [Lac] at the upper critical temperatures indicates recruitment of anaerobiosis owing to high tissue energy demand, while the high QO2’s suggest some cardiorespiratory capability in supplying tissues with oxygen. For example, the spider crab Maja squinado shows changes in [Lac] in some tissues in tandem with a reduction in aerobic capacity. High (33°C) and low (0°C) temperatures provoke decoupling between O2 supply and demand, since mitochondrial O2 consumption is consistent with oxygen delivery by the cardiorespiratory system (Frederich and Pörtner, 2000). Further, in decapod crustaceans subjected to hypoxia or exercise, lactate affects hemocyanin affinity for oxygen, increasing oxygen extraction by the gills and transport by the hemolymph to the tissues as seen in the brachyuran crabs Cancer, Carcinus, and Hyas (Truchot, 1980; Morris and Bridges, 1989), Homarus lobsters (Nies et al., 1992) and Macrobrachium shrimps (Ern et al., 2013).
Since aerobic and anaerobic metabolism are both linked to the energy supply of a biological system (see Pörtner et al., 2017), the lower mean UL50 for the sub-Antarctic crabs (≈26°C) compared to the mean UL50 for the tropical and subtropical species (≈37°C) may reflect a mismatch between aerobic and anaerobic energy production at the upper critical limits. In fact, under high thermal challenge, the sub-Antarctic species have evolved a lower aerobic metabolism (0.4-fold), albeit circumvented by an augmented anaerobic capacity (9.1-fold) when compared to the mean values for the tropical and subtropical species (Figure 4). Since aerobic capacity ensures higher ATP production (potentially up to 38 ATP molecules per glucose molecule) than does anaerobic metabolism (only 2 ATP molecules), the lower energy budget available to the sub-Antarctic species could impair overall cellular activities such as anti-oxidant defenses and heat-shock responses, reducing thermal tolerance. The acute stress encountered at the upper critical temperatures constrains aerobic capacity and shifts the energy generating system to a predominantly anaerobic metabolism in crabs from colder climates, suggesting an evolutionary metabolic trade-off during thermal niche diversification.
LDH Kinetics
For each zoogeographical province, mean Vmax tends to become reduced at the LL50 and to increase at the UL50, both compared to the respective FRT. Interestingly, at the fixed temperature of 25°C, there was an effect of zoogeographical province: mean Vmax for the sub-Antarctic species was 3-fold lower than that for tropical species. This tendency for lower activities at the same temperature for species from colder regions contrasts with previous data for tropical/subtropical and Antarctic fish (Kawall et al., 2002) that show thermal compensation of LDH specific activity. In this case, brain LDH activity was up to 2.5-fold higher in the Antarctic species, while muscle LDH activities were similar between the two thermal groups, both measured at the same temperature of 10°C (Kawall et al., 2002).
The absence of thermal compensation in LDH activity in the intertidal, neotropical crabs evaluated here corroborates findings for another intertidal decapod clade distributed from Northern to Central America, anomuran crabs Petrolisthes. No effect of maximum habitat temperature was seen on the evolution of LDH thermal stability in 22 congeneric species sampled over a ≈35° range of latitude, suggesting that the diversity of LDH stabilities also is not adaptive (Stillman and Somero, 2001). Further, similar stabilities at maximum temperatures for some sibling Petrolisthes groups were suggested, which is reinforced here for neotropical crabs, owing to the phylogenetic signal seen for Vmax at UL50. Although maximum and critical temperatures, respectively, do not constitute a selective pressure on the evolution of LDH stability (anomurans) and activity (brachyurans), the inter-specific variability in these mechanistic bases demonstrates the importance of ancestral inertia in the evolutionary history of different intertidal decapod lineages.
While Vmax does not suggest LDH thermal compensation when comparing activities at the same temperature, the catalytic coefficient (also at 25°C) correlated negatively with micro-habitat temperatures (MHT). Species sampled from higher MHT tend to have lower catalytic coefficients and vice-versa (except for the sub-Antarctic species A. albatrossis and H. planatus). In fact, the lower MHT values (21–26°C) show a mean catalytic coefficient 2.5-fold higher than the mean value for the species from warmer habitats (28–36°C), which means that species from colder MHT’s show more flexible LDH molecules, while conversely, those from warmer habitats show more rigid enzymes. In contrast, mean KmPyr tends to be constant (≈0.27 mM), reflecting retention of LDH affinity for pyruvate and, consequently, its binding efficiency.
The temperature compensation of LDH activity has evolved through the transformation of catalytic efficiency, which is in agreement with maintenance of binding capacity for pyruvate (see Hochachka and Somero, 2002). Such a biochemical trade-off has been documented for several fish species from various thermal niches, such as Sphyraena barracudas (Holland et al., 1997), Chromis Pacific fish (Johns and Somero, 2004) and four families of Antarctic notothenioids (Fields and Somero, 1998). The maintenance of the LDH binding property demonstrates conservation of the catalytic site. Variability in enzyme efficiency results from alterations to the amino acid sequence in regions that undergo conformational changes during formation of the enzyme-substrate complex (see Hochachka and Somero, 2002). The reasons why LDH in tropical species has lost its affinity (higher KmPyr) during exposure at UL50, and why sub-Antarctic crabs have not retained a higher catalytic efficiency at their natural MHT, remain conjectural and require investigation at the molecular level.
Overall physiological evolution, when considered simultaneously for all systemic and kinetic traits, including MHT and both critical limits, consists of two steps. The first took place at the outset of the present crab phylogeny, and the second at the outset of the tropical + subtropical lineage, owing to the shift between the two main dimensions, PC1 for the sub-Antarctic species and PC2 for the tropical and subtropical crabs (Figure 7). Interestingly, these findings are in agreement with the two adaptive peaks detected in this crab phylogeny: one at the root, and maintained in A. albatrossis and H. planatus, and another at the outset of the tropical and subtropical species. However, since the sub-Antarctic A. albatrossis and H. planatus are a paraphyletic grouping, also belonging to different eubrachyuran families (Bellidae and Hymenosomatidae, respectively, see Faria et al., 2017), the adaptive peak revealed here should be interpreted as the result of adaptive convergence. The adaptive peaks detected using SURFACE are also in concert with most evolutionary associations detected using PGLS since they were better adjusted by the O-U model, with higher selection strengths that limit minimum and maximum physiological capabilities. The reason why the most important traits that contribute to this macroevolutionary landscape are the thermal niche, and the upper and lower critical thermal limits, may depend on their underlying biological organization, since such traits integrate lower hierarchical levels, including lesser physiological differences, following the synergistic principle of the “part-whole” relationship.
In summary, the metabolic physiology of crabs living at the edge of their thermal windows accompanies the asymmetry of their critical limits. The extension of life to higher temperatures depends on a putative trade-off between the evolution of aerobic and anaerobic metabolism with respect to energy supply, while temperature compensation of kinetic performance is driven by thermal habitat, as revealed by the LDH affinity/efficiency equilibrium. In fact, shared inheritance and the thermal environment both affect the overall physiological history of these intertidal crabs, revealing that certain evolutionary transformations have arisen both in warmer and in colder climes, particularly at higher levels of biological organization and phylogenetic diversity.
Data Availability Statement
The datasets generated for this study are available on request to the corresponding author.
Ethics Statement
Crab sampling in Brazil was authorized by the Ministry for the Environment (MMA/ICMBio permit #29594-8 to JM), and in Argentina by the Ministry for the Environment, Sustainable Development and Climate Change of Tierra del Fuego (permit #0116/2014 to MR).
Author Contributions
SF and JM contributed to the conceptualization, the data curation, the project administration, the funding acquisition, and the visualization. SF, ML, AZ, FT, MR, AB, and JM contributed to the methodology, writing, review, and the editing. SF contributed to the software, writing, and the initial draft. SF, ML, and JM contributed to the formal analysis. SF, ML, FT, MT, and JM contributed to the investigation. SF, AB, FT, MR, and JM contributed to the resources. JM contributed to the supervision.
Funding
This work was supported by the Fundação de Amparo à Pesquisa do Estado de São Paulo (FAPESP scholarship #2011/08852-0 to SF, and grants #2011/22537-0 and #2020/04135-1 to JM), the Conselho Nacional de Desenvolvimento Científico e Tecnológico (CNPq #450320/2010-3 and #300662/2009-2 to JM) and the Coordenação de Aperfeiçoamento de Pessoal de Nível Superior (CAPES 33002029031P8, finance code 001, to JM). SF was a post-doctoral investigator (FAPESP #2018/17252-6).
Conflict of Interest
The authors declare that the research was conducted in the absence of any commercial or financial relationships that could be construed as a potential conflict of interest.
Acknowledgments
We are grateful to P. Barreto, R. Faleiros, M. Sotelano, O. Florentin, R. Lorenzo, and R. Padilha for assisting with fieldwork or preparing dry ice. We also thank Drs. Luiz Carlos Alves and Fábio Brayner for generously offering their facilities in Pernambuco, Drs. Douglas Masui, Eduardo Oliveira and Luana Meleiro for assistance with kinetic assays, and Dr. Wilfried Klein for helping set up the FoxBox system. SF is especially thankful to Dr. Rosa Furriel for supervising the biochemical kinetic assays and for her rare mixture of humanity and science. This investigation is part of a Ph.D. thesis submitted by SF to the Graduate Program in Comparative Biology, Departamento de Biologia, FFCLRP, Universidade de São Paulo.
References
Abouheif, E. (1999). A method for testing the assumption of phylogenetic independence in comparative data. Evol. Ecol. Res. 1, 895–909.
Balech, E. (1954). División zoogeográfica del litoral sudamericano. Rev. Biol. Mar. Dep. Oceanol. Univ. Chile 4, 184–195.
Bergmeyer, H. U., and Bernt, E. (1974). ““L-lactate analyses”,” in Methods of Enzymatic Analysis, 2nd Edn, Vol. II, ed. H. U. Bergmeyer (New York: Academic Press), 574–579.
Boschi, E. E. (2000a). Species of decapod crustaceans and their distribution in the American marine zoogeographic provinces. Rev. Invest. Des. Pesq. 13, 7–136.
Boschi, E. E. (2000b). Biodiversity of marine decapod brachyurans of the Americas. J. Crust. Biol. 20, 337–342. doi: 10.1163/1937240x-90000036
Boschi, E. E., and Gavio, M. A. (2005). On the distribution of decapod crustaceans from the Magellan Biogeographic Province and the Antarctic region. Sci. Mar. 69, 195–200. doi: 10.3989/scimar.2005.69s2195
Carcelles, A. R., and Williamson, S. I. (1951). Catalogo de los moluscos de la provincia magallanica. Revta. Lnst. Nac. Invest. Cienc. Nat. 2, 255–383.
Cooke, A. H. (1895). “Mollusca,” in The Cambridge Natural History, eds S. F. Harmer and A. E. Shipley (London: MacMillan).
Cowles, R. B., and Bogert, C. M. (1944). A preliminary study of the thermal requirements of desert reptiles. Bull. Am. Mus. Nat. Hist. 83, 265–296.
Diniz-Filho, J. A. F. (2001). Phylogenetic autocorrelation under distinct evolutionary processes. Evolution 55, 1104–1109. doi: 10.1111/j.0014-3820.2001.tb00630.x
Dong, Y., Miller, L. P., Sanders, J. G., and Somero, G. N. (2008). Heat-shock protein 70 (Hsp70) expression in four limpets of the genus Lottia: interspecific variation in constitutive and inducible synthesis correlates with in situ exposure to heat stress. Biol. Bull. 215, 173–181. doi: 10.2307/25470698
Dunn, C. R., Wilks, H. M., Halsall, D. J., Atkinson, T., Clarke, A. R., Muirhead, H., et al. (1991). Design and synthesis of new enzymes based on the lactate dehydrogenase framework. Phil. Trans. R. Soc. B 332, 177–184. doi: 10.1098/rstb.1991.0047
Dunn, T. W., and Mercier, A. J. (2003). Synaptic modulation by a neuropeptide depends on temperature and extracellular calcium. J. Neurophysiol. 89, 1807–1814. doi: 10.1152/jn.00710.2002
Ern, R., Huong, D. T. T., Nguyen, V. C., Wang, T., and Bayley, M. (2013). Effects of salinity on standard metabolic rate and critical oxygen tension in the giant freshwater prawn (Macrobrachium rosenbergii). Aquat. Res. 44, 1259–1265. doi: 10.1111/j.1365-2109.2012.03129.x
Faria, S. C., Faleiros, R. O., Brayner, F. A., Alves, L. C., Bianchini, A., Romero, C., et al. (2017). Macroevolution of thermal tolerance in intertidal crabs from Neotropical provinces: a phylogenetic comparative evaluation of critical limits. Ecol. Evol. 7, 3167–3176. doi: 10.1002/ece3.2741
Felsenstein, J. (1973). Maximum likelihood and minimum-steps methods for estimating evolutionary trees from data on discrete characters. Syst. Biol. 22, 240–249. doi: 10.1093/sysbio/22.3.240
Felsenstein, J. (1981). Evolutionary trees from DNA sequences: a maximum likelihood approach. J. Mol. Evol. 17, 368–376. doi: 10.1007/bf01734359
Fields, P. A. (2001). Protein function at thermal extremes: balancing stability and flexibility. Comp. Biochem. Physiol. 129, 417–431. doi: 10.1016/s1095-6433(00)00359-7
Fields, P. A., and Somero, G. N. (1998). Hot spots in cold adaptation: localized increases in conformational flexibility in lactate dehydrogenase A4 orthologs of Antarctic notothenioid fishes. Proc. Natl. Acad. Sci. 95, 11476–11481. doi: 10.1073/pnas.95.19.11476
Fox, H. M. (1936). The activity and metabolism of poikilothermal animals in different latitudes. Proc. Zool. Soc. London. 1936, 945–955. doi: 10.1111/j.1469-7998.1936.tb06295.x
Frederich, M., and Pörtner, H. O. (2000). Oxygen limitation of thermal tolerance defined by cardiac and ventilatory performance in the spider crab Maja squinado. Am. J. Physiol. 279, 1531–1538.
Frederich, M., Sartoris, F. J., Arntz, W. E., and Pörtner, H. O. (2000). Haemolymph Mg2+ regulation in decapod crustaceans: physiological correlates and ecological consequences in polar areas. J. Exp. Biol. 203, 1383–1393.
Garland, T. Jr., and Ives, I. R. (2000). Using the past to predict the present: confidence intervals for regression equations in phylogenetic comparative methods. Am. Nat. 155, 346–364. doi: 10.1086/303327
Giomi, F., Fusi, M., Barausse, A., Mostert, B., Pörtner, H. O., and Cannicci, S. (2014). Improved heat tolerance in air drives the recurrent evolution of air-breathing. Proc. R. Soc. Lond. B. 281, 20132927. doi: 10.1098/rspb.2013.2927
Giomi, F., and Pörtner, H. O. (2013). A role for haemolymph oxygen capacity in heat tolerance of eurythermal crabs. Front. Physiol 4:110. doi: 10.3389/fphys.2013.00110
Gittleman, J. L., and Kot, M. (1990). Adaptation: statistics and a null model for estimating phylogenetic effects. Syst. Zool. 39, 227–241.
Henry, R. P., Booth, C. E., Lallier, F. H., and Walsh, P. J. (1994). Post-exercise lactate production and metabolism in three species of aquatic and terrestrial decapod crustaceans. J. Exp. Biol. 186, 215–234.
Hochachka, P. W., and Somero, G. N. (2002). Biochemical Adaptation: Mechanism and Process in Physiological Evolution. New York, NY: Oxford University Press.
Holland, L. Z., McFall-Ngai, M., and Somero, G. N. (1997). Evolution of lactate dehydrogenase-A homologues of barracuda fishes (genus Sphyraena) from different thermal environments: differences in kinetic properties and thermal stability are due to amino acid substitutions outside the active site. Biochemistry 36, 3207–3215. doi: 10.1021/bi962664k
Ingram, T., and Mahler, D. L. (2013). SURFACE: detecting convergent evolution from comparative data by fitting Ornstein-Uhlenbeck models with stepwise Akaike Information Criterion. Methods Ecol Evol 4, 416–425. doi: 10.1111/2041-210x.12034
Johns, G. C., and Somero, G. N. (2004). Evolutionary convergence in adaptation of proteins to temperature: A4-lactate dehydrogenases of Pacific damselfishes (Chromis spp.). Mol. Biol. Evol. 21, 314–320. doi: 10.1093/molbev/msh021
Johnston, I. A., and Maitland, B. (1980). Temperature acclimation in crucian carp (Carassius carassius L): morphometric analysis of muscle fibre ultrastructure. J. Fish. Biol. 17, 113–125. doi: 10.1111/j.1095-8649.1980.tb02746.x
Jombart, T., Balloux, F., and Dray, S. (2010). Adephylo: new tools for investigating the phylogenetic signal in biological traits. Bioinformatics 26, 1907–1909. doi: 10.1093/bioinformatics/btq292
Jost, J. A., Podolski, S. M., and Frederich, M. (2012). Enhancing thermal tolerance by eliminating the pejus range: a comparative study with three decapod crustaceans. Mar. Ecol. Prog. Ser. 444, 263–274. doi: 10.3354/meps09379
Kawall, H., Torres, J., Sidell, B., and Somero, G. (2002). Metabolic cold adaptation in Antarctic fishes: evidence from enzymatic activities of brain. Mar. Biol. 140, 279–286. doi: 10.1007/s002270100695
Lavin, S. R., Karasov, W. H., Ives, A. R., Middleton, K. M., and Garland, T. Jr. (2008). Morphometrics of the avian small intestine, compared with nonflying mammals: a phylogenetic perspective. Physiol. Biochem. Zool. 81, 526–550. doi: 10.1086/590395
Leone, F. A., Baranauskas, J. A., Furriel, R. P. M., and Borin, I. A. (2005). SigrafW: an easy-to-use program for fitting enzyme kinetic data. Biochem. Mol. Biol. Educ. 33, 399–403. doi: 10.1002/bmb.2005.49403306399
Mantelatto, F. L., Pardo, L. M., Pileggi, L. G., and Felder, D. L. (2009a). Taxonomic re-examination of the hermit crab species Pagurus forceps and Pagurus comptus (Decapoda: Paguridae) by molecular analysis. Zootaxa 2133, 20–32. doi: 10.11646/zootaxa.2133.1.2
Mantelatto, F. L., Robles, R., Schubart, C. D., and Felder, D. L. (2009b). “Molecular phylogeny of the genus Cronius Stimpson, 1860, with reassignment of C. tumidulus and several American species of Portunus to the genus Achelous De Haan, 1833 (Brachyura: Portunidae),” in Crustacean issues: Decapod crustacean phylogenetics, eds J. M. Martin, K. A. Crandall, and D. L. Felder (Boca Raton: Taylor & Francis/CRC Press), 537–551.
Morley, S. A., Lurman, G. L., Skepper, J. N., Pörtner, H. O., and Peck, L. S. (2009). Thermal plasticity of mitochondria: A latitudinal comparison between Southern Ocean molluscs. Comp. Biochem. Physiol. 152, 423–430. doi: 10.1016/j.cbpa.2008.11.015
Morris, S., and Bridges, C. R. (1989). Interactive effects of temperature and L-lactate on the binding of oxygen by the hemocyanin of two arctic boreal crabs, Hyas araneus and Hyas coarctatus. Physiol. Zool. 62, 62–82. doi: 10.1086/physzool.62.1.30159998
National Oceanic and Atmospheric Administration [NOAA], and U. S. Department of Commerce (2019). Satellite Data Products for Understanding and Managing Our Oceans and coasts. Avaliable at: https://coastwatch.noaa.gov/cw/index.html (accessed July 2019).
Nies, A., Zeis, B., Bridges, C. R., and Grieshaber, M. K. (1992). Allosteric modulation of haemocyanin oxygen-affinity by lactate and urate in the lobster Homarus vulgaris: II. Characterization of specific effector binding sites. J. Exp. Biol. 168, 111–124.
Northrop, D. B. (1998). On the meaning of Km and V/K in enzyme kinetics. J. Chem. Educ. 75:1153. doi: 10.1021/ed075p1153
Paradis, E., Claude, J., and Strimmer, K. (2004). Ape: analyses of phylogenetics and evolution in R language. Bioinformatics 20, 289–290. doi: 10.1093/bioinformatics/btg412
Parnas, H., Parnas, I., Ravin, R., and Yudelevitch, B. (1994). Glutamate and N-methyl-D-aspartate affect release from crayfish axon terminals in a voltage-dependent manner. Proc. Nat. Acad. Sci. U.S.A. 91, 11586–11590. doi: 10.1073/pnas.91.24.11586
Pavoine, S., Ollier, S., Pontier, D., and Chessel, D. (2008). Testing for phylogenetic signal in phenotypic traits: new matrices of phylogenetic proximities. Theor. Popul. Biol. 73, 79–91. doi: 10.1016/j.tpb.2007.10.001
Peck, L. S., Pörtner, H. O., and Hardewig, I. (2002). Metabolic demand, oxygen supply and critical temperatures in the Antarctic bivalve Laternula elliptica. Physiol. Biochem. Zool. 75, 123–133. doi: 10.1086/340990
Pileggi, L. G., and Mantelatto, F. L. (2010). Molecular phylogeny of the freshwater prawn genus Macrobrachium (Decapoda, Palaemonidae), with emphasis on the relationships among selected American species. Invertebr. Syst. 24, 194–208.
Pinheiro, J., Bates, D., DebRoy, S., and R Core Team (2015). nlme: Linear and Nonlinear Mixed Effects Models. R Package Version 3. 1–137.
Pörtner, H. O. (2002). Climate variations and the physiological basis of temperature dependent biogeography: systemic to molecular hierarchy of thermal tolerance in animals. Comp. Biochem. Physiol. 132, 739–761. doi: 10.1016/s1095-6433(02)00045-4
Pörtner, H. O., Bock, C., and Mark, F. C. (2017). Oxygen-and capacity-limited thermal tolerance: bridging ecology and physiology. J. Exp. Biol. 220, 2685–2696. doi: 10.1242/jeb.134585
Pörtner, H. O., and Gutt, J. (2016). Impacts of climate variability and change on (marine) animals: physiological underpinnings and evolutionary consequences. Integr. Comp. Biol. 56, 31–44. doi: 10.1093/icb/icw019
R Development Core Team (2019). R: A Language and Environment for Statistical Computing. Vienna: R Foundation for Statistical Computing. doi: 10.1093/icb/icw019
Read, S. M., and Northcote, D. H. (1981). Minimization of variation in the response to different protein of the Coomassie blue G dye-binding assay for protein. Anal. Biochem. 116, 53–64. doi: 10.1016/0003-2697(81)90321-3
Recordati, G., and Bellini, T. G. (2004). A definition of internal constancy and homeostasis in the context of non-equilibrium thermodynamics. Exp. Physiol. 89, 27–38. doi: 10.1113/expphysiol.2003.002633
Revell, L. J. (2010). Phylogenetic signal and linear regression on species data. Methods Eco.l Evol. 1, 319–329. doi: 10.1111/j.2041-210x.2010.00044.x
Revell, L. J. (2012). Phytools: an R package for phylogenetic comparative biology (and other things). Method Ecol. Evol. 3, 217–223. doi: 10.1111/j.2041-210x.2011.00169.x
Santos, E. A., and Keller, R. (1993). Effect of exposure to atmospheric air on blood glucose and lactate concentrations in two crustacean species: a role of the crustacean hyperglycemic hormone (CHH). Comp. Biochem. Physiol. 106, 343–347. doi: 10.1016/0300-9629(93)90523-7
Scholander, P. F., Flagg, W., Walters, V., and Irving, L. (1953). Climatic adaptation in arctic and tropical poikilotherms. Physiol. Zool. 26, 67–92. doi: 10.1086/physzool.26.1.30152151
Schubart, C. D., Cuesta, J. A., Diesel, R., and Felder, D. L. (2000). Molecular phylogeny, taxonomy, and evolution of nonmarine lineages within the American grapsoid crabs (Crustacea: Brachyura). Mol. Phylogenet. Evol. 15, 179–190. doi: 10.1006/mpev.1999.0754
Somero, G. N. (2003). Protein adaptations to temperature and pressure: complementary roles of adaptive changes in amino acid sequence and internal milieu. Comp. Biochem. Physiol. 136, 577–591. doi: 10.1016/s1096-4959(03)00215-x
Somero, G. N. (2004). Adaptation of enzymes to temperature: searching for basic ‘strategies’. Comp. Biochem. Physiol. 139, 321–333. doi: 10.1016/j.cbpc.2004.05.003
Somero, G. N. (2010). The physiology of climate change: how potentials for acclimatization and genetic adaptation will determine ‘winners’ and ‘losers.’. J. Exp. Biol. 213, 912–920. doi: 10.1242/jeb.037473
Stamatakis, A. (2006). RAxML-VI-HPC: maximum likelihood-based phylogenetic analyses with thousands of taxa and mixed models. Bioinformatics 22, 2688–2690. doi: 10.1093/bioinformatics/btl446
Stamatakis, A., Hoover, P., and Rougemont, J. (2008). A rapid bootstrap algorithm for the RAxML web servers. Syst. Biol. 57, 758–771. doi: 10.1080/10635150802429642
Steffensen, J. F. (1989). Some errors in respirometry of aquatic breathers: how to avoid and correct them. Fish. Physiol. Biochem. 6, 49–59. doi: 10.1007/bf02995809
Stevens, M. M., Jackson, S., Bester, S. A., Terblanche, J. S., and Chown, S. L. (2010). Oxygen limitation and thermal tolerance in two terrestrial arthropod species. J. Exp. Biol. 213, 2209–2218. doi: 10.1242/jeb.040170
Stillman, J. H., and Somero, G. N. (2000). A comparative analysis of the upper thermal tolerance limits of eastern Pacific porcelain crabs, genus Petrolisthes: influences of latitude, vertical zonation, acclimation, and phylogeny. Physiol. Biochem. Zool. 73, 200–208. doi: 10.1086/316738
Stillman, J. H., and Somero, G. N. (2001). A comparative analysis of the evolutionary patterning and mechanistic bases of lactate dehydrogenase thermal stability in porcelain crabs, genus Petrolisthes. J. Exp. Biol. 204, 767–776.
Tavaré, S. (1986). “Some probabilistic and statistical problems in the analysis of DNA sequences,” in Lectures on Mathematics in the Life Sciences, ed. R. M. Miura (Providence, RI: American Mathematical Society,), 57–86.
Thurman, C. L., Faria, S. C., and McNamara, J. C. (2013). The distribution of fiddler crabs (Uca) along the coast of Brazil: implications for biogeography of the western Atlantic ocean. Mar. Biodivers. Records. 6, 1–21.
Tomanek, L., and Zuzow, M. (2010). The proteomic response of the mussel congeners Mytilus galloprovincialis and M. trossulus to acute heat-stress: implications for thermal tolerance limits and metabolic costs of thermal stress. J. Exp. Biol. 213, 3559–3574. doi: 10.1242/jeb.041228
Truchot, J. P. (1980). Lactate increases the oxygen affinity of crab hemocyanin. J. Exp. Zool. 214, 205–208. doi: 10.1002/jez.1402140212
Tyler, S., and Sidell, B. D. (1984). Changes in mitochondrial distribution and diffusion distances in muscle of goldfish upon acclimation to warm and cold temperatures. J. Exp. Zool. 232, 1–9. doi: 10.1002/jez.1402320102
Verberk, W. C., Overgaard, J., Ern, R., Bayley, M., Wang, T., Boardman, L., et al. (2016). Does oxygen limit thermal tolerance in arthropods? A critical review of current evidence. Comp. Biochem. Physiol. 192, 64–78. doi: 10.1016/j.cbpa.2015.10.020
Wittmann, A. C., Held, C., Pörtner, H. O., and Sartoris, F. J. (2010). Ion regulatory capacity and the biogeography of Crustacea at high southern latitudes. Polar Biol. 33, 919–928. doi: 10.1007/s00300-010-0768-1
Wittmann, A. C., Pörtner, H. O., and Sartoris, F. J. (2012). A role for oxygen delivery and extracellular magnesium in limiting cold tolerance of the sub-antarctic stone crab Paralomis granulosa? Physiol. Biochem. Zool. 85, 285–298. doi: 10.1086/665328
Zakhartsev, M. V., Johansen, T., Pörtner, H. O., and Blust, R. (2004). Effects of temperature acclimation on lactate dehydrogenase of cod (Gadus morhua): genetic, kinetic and thermodynamic aspects. J. Exp. Biol. 207, 95–112. doi: 10.1242/jeb.00708
Zavodszky, P., Jozsef, K., Svingor, A., and Petsko, G. A. (1998). Adjustment of conformational flexibility is a key event in the thermal adaptation of proteins. Proc. Nat. Acad. Sci. U.S.A. 98, 7406–7411. doi: 10.1073/pnas.95.13.7406
Keywords: evolutionary physiology, thermal adaptation, critical limits, oxygen consumption, lactate, LDH, Brachyura
Citation: Faria SC, Bianchini A, Lauer MM, Zimbardi ALRL, Tapella F, Romero MC and McNamara JC (2020) Living on the Edge: Physiological and Kinetic Trade-Offs Shape Thermal Tolerance in Intertidal Crabs From Tropical to Sub-Antarctic South America. Front. Physiol. 11:312. doi: 10.3389/fphys.2020.00312
Received: 30 May 2019; Accepted: 19 March 2020;
Published: 24 April 2020.
Edited by:
Peter John Fraser, University of Aberdeen, United KingdomReviewed by:
Carl L. Thurman, University of Northern Iowa, United StatesWolfgang Stein, Illinois State University, United States
Carolina Madeira, Associação para a Inovação e Desenvolvimento da FCT (NOVA.ID.FCT), Portugal
Copyright © 2020 Faria, Bianchini, Lauer, Zimbardi, Tapella, Romero and McNamara. This is an open-access article distributed under the terms of the Creative Commons Attribution License (CC BY). The use, distribution or reproduction in other forums is permitted, provided the original author(s) and the copyright owner(s) are credited and that the original publication in this journal is cited, in accordance with accepted academic practice. No use, distribution or reproduction is permitted which does not comply with these terms.
*Correspondence: John Campbell McNamara, bWNuYW1hcmFAZmZjbHJwLnVzcC5icg==
†present address: Samuel Coelho Faria, Centro de Biologia Marinha, Universidade de São Paulo, São Sebastião, Brazil