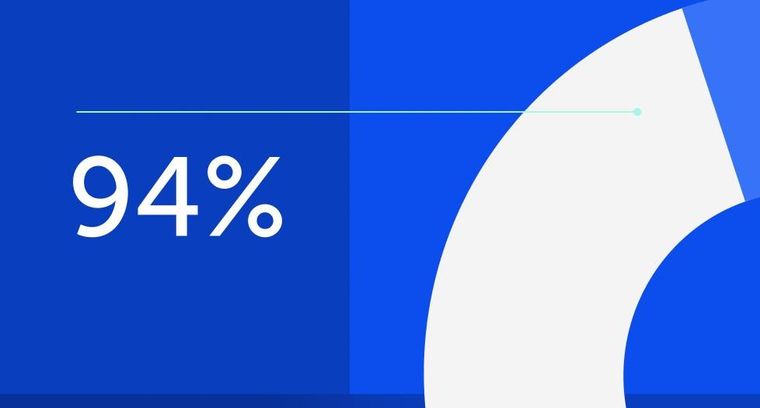
94% of researchers rate our articles as excellent or good
Learn more about the work of our research integrity team to safeguard the quality of each article we publish.
Find out more
ORIGINAL RESEARCH article
Front. Physiol., 06 April 2020
Sec. Clinical and Translational Physiology
Volume 11 - 2020 | https://doi.org/10.3389/fphys.2020.00284
Dynamic arterial elastance (Eadyn), the ratio between arterial pulse pressure and stroke volume changes during respiration, has been postulated as an index of the coupling between the left ventricle (LV) and the arterial system. We aimed to confirm this hypothesis using the gold-standard for defining LV contractility, afterload, and evaluating ventricular-arterial (VA) coupling and LV efficiency during different loading and contractile experimental conditions. Twelve Yorkshire healthy female pigs submitted to three consecutive stages with two opposite interventions each: changes in afterload (phenylephrine/nitroprusside), preload (bleeding/fluid bolus), and contractility (esmolol/dobutamine). LV pressure-volume data was obtained with a conductance catheter, and arterial pressures were measured via a fluid-filled catheter in the proximal aorta and the radial artery. End-systolic elastance (Ees), a load-independent index of myocardial contractility, was calculated during an inferior vena cava occlusion. Effective arterial elastance (Ea, an index of LV afterload) was calculated as LV end-systolic pressure/stroke volume. VA coupling was defined as the ratio Ea/Ees. LV efficiency (LVeff) was defined as the ratio between stroke work and the LV pressure-volume area. Eadyn was calculated as the ratio between the aortic pulse pressure variation (PPV) and conductance-derived stroke volume variation (SVV). A linear mixed model was used for evaluating the relationship between Ees, Ea, VA coupling, LVeff with Eadyn. Eadyn was inversely related to VA coupling and directly to LVeff. The higher the Eadyn, the higher the LVeff and the lower the VA coupling. Thus, Eadyn, an easily measured parameter at the bedside, may be of clinical relevance for hemodynamic assessment of the unstable patient.
Dynamic arterial elastance, the ratio between PPV and SVV, defines the relationship between the changes in the arterial pulse pressure and SV during a respiratory cycle (Pinsky, 2006). This index has been demonstrated to predict the arterial pressure changes associated to fluid administration in hypotensive preload-dependent patients, as well as predicting the arterial pressure response to decreasing norepinephrine dose (Monge Garcia et al., 2014; Guinot et al., 2015), and is part of some hemodynamic optimization protocols for guiding fluid and vasopressor therapy (Guinot et al., 2017; Davies et al., 2019; Guarracino et al., 2019; Maheshwari et al., 2019).
The term elastance suggests that Eadyn is related to arterial stiffness. Indeed, Eadyn was initially proposed as an index of arterial tone (Pinsky, 2006). However, as Eadyn relates relative changes in arterial pressure and SV, Eadyn is unitless and should only be considered as an indirect measure of arterial stiffness or LV afterload. As usually measured, Eadyn is defined by the dynamic arterial pressure-stroke volume relationship during respiration, as both vary (Monge Garcia et al., 2014). Moreover, when the arterial tone was directly modified, as during the use of vasoactive drugs such as phenylephrine or sodium nitroprusside, Eadyn changed in the opposite way to arterial vasomotor tone (Monge Garcia et al., 2017a; Wodack et al., 2019). Besides, other factors not related to the arterial system have been involved in the subsequent Eadyn value, for example, heart rate and blood flow acceleration have been associated with changes in Eadyn (Monge Garcia et al., 2017a). How these arterial and cardiac factors affect to PPV and SVV would define Eadyn. These potentially contradictory findings led to propose that Eadyn may represent an index of the coupling between the heart and the systemic circulation (Pinsky, 2006; Monge Garcia et al., 2017a). However, the hypothesis that Eadyn is related to VA coupling or whether Eadyn would track changes in VA coupling have not been studied. Thus, we analyzed the impact of various maneuvers known to affect vasomotor tone, contractility and volume status on both VA coupling and Eadyn.
The study was approved by the Institutional Animal Care and Use Committee (IACUC) at the Edwards Research Center, and all procedures were performed in accordance with the USDA Animal Welfare Act regulations (AWArs), and the Guide for the Care and Use of Laboratory Animals (ILAR, NAP, Washington, DC, United States, 2010, 8th edition). The ARRIVE guidelines were used for the elaboration of this manuscript (NC3Rs Reporting Guidelines Working Group, 2010).
Experiments were performed in 12 female Yorkshire crossbreed pigs of 5.5 ± 0.8 (mean ± SD) months old weighting 81 ± 6 kg. Animals were maintained in a temperature and humidity-controlled rooms with a typical light-dark cycle and given standard chow and tap water ad libitum. Before anesthesia induction, a general physical examination was performed. If found to be stable, the animal was premedicated with an intramuscular injection of telazol (4.4 mg/kg), ketamine (2.2 mg/kg), and xylazine (1.1 mg/kg). They were orally intubated, and their lungs mechanically ventilated in a volume-controlled mode (fraction of inspired oxygen of 0.6–0.8, tidal volume of 10 ml/kg plus 100 ml compensation for dead space, respiratory rate of 15 breaths/min). Ventilatory settings were maintained unchanged throughout the experiment. Following endotracheal intubation, general anesthesia was maintained with isoflurane 1.5–2.5% and a mixture of oxygen (two animals), oxygen/air (three animals) and oxygen/air/nitrous oxide (seven animals). The level of anesthesia was adjusted and kept at the lowest possible dose. No neuromuscular blocking drugs were used. Animals received a loading dose of heparin (300 I.U./kg) with additional boluses during follow-up (mean total dose: 1040 I.U./kg) for preventing coagulation. Fluid maintenance was provided intravenously by a continuous infusion of Ringer’s lactate solution at 4 ml.kg–1.h–1. Rectal temperature was monitored and kept at 37°C (mean measured value 36.6°C) using a heating pad. Arterial blood gases were monitored periodically and acid-base balance was corrected by infusing bolus sodium bicarbonate or by changing ventilator frequency if required. The depth of anesthesia was assessed throughout the study for muscular reflex by checking the jaw tone and the toe pinch assessment. Animal anesthesia was monitored and recorded approximately every 15 min for the duration of the experimentation. All the experimental procedures were performed by the same skilled operator.
Instantaneous LV PV measurements were obtained from a 7Fr-lumen dual-field conductance catheter introduced through the left external carotid artery with 12 equidistant electrodes and a high-fidelity pressure sensor (CA71083-PL, CD Leycom, Zoetermeer, Netherlands) connected to a PV signal processor (Inca®, CD Leycom, Zoetermeer, Netherlands). The catheter tip was positioned in the long axis of the LV, and the correct placement was confirmed by fluoroscopy and the examination of the individual segmental LV PV loops.
The volume signal calibration was performed via right-heart catheterization with a pulmonary artery catheter (Swan-Ganz CCOmbo 777HF8, Edwards Lifesciences, Irvine, CA, United States) inserted percutaneously through the right internal jugular vein to the pulmonary artery using the standard thermodilution method for the determination of CO. The average value of 3 to 5 thermodilution boluses randomly injected during the respiratory cycle was used for volume calibration. Correction for parallel conductance (the conductance of the surrounding tissues, which was subtracted from the raw catheter volume) was performed with the 10 ml boluses of 5% hypertonic saline through the distal port of the pulmonary artery catheter during a short period of apnea (Baan et al., 1984). The conductance signals were then converted to calibrated volume signals by considering the inter-electrode spacing, the parallel conductance correction and the CO calibration factor obtained from thermodilution (Baan et al., 1984; Kass et al., 1986). CO calibration and parallel conductance correction were performed before starting the experimental protocol and after the fluid bolus stage.
Left ventricle pressure-volume data acquisition and analysis were performed by a dedicated software system (Conduct NT, version 3.18.1, CD Leycom, Zoetermeer, Netherlands). The signals were recorded at 250 Hz and filtered using a 25 Hz low-pass filter. Before and after each experimental stage, three transient occlusions of the inferior vena cava (IVC) were performed using a 25 mm Fogarty balloon (Edwards Transfemoral Balloon Catheter, 9350BC25, Edwards Lifesciences) percutaneously introduced into the right femoral vein. All recordings were done during prolonged apnea in end-expiration. This procedure was repeated if ectopic beats were detected. End-systolic pressure, SV, CO, LV EDV, LV ESV, LV EDP, LVEF, and effective arterial elastance [Ea = Pes/SV, a lumped parameter of LV afterload accounting for pulsatile and resistive load (Sunagawa et al., 1984)] were calculated from 5 beats in steady-state conditions during the respiratory pause just before the IVC occlusion. LV Ees was calculated using the iterative regression method (Kass et al., 1986), as the slope of the ESPVR determined from the linear regression analysis of the maximal E points on each cardiac cycle, defined as E(t) = P(t)/V(t)−V0, where V0 is volume-axis intercept or the LV unstressed volume. An example of a PV loop analysis is shown in Figure 1.
Figure 1. Example of the left ventricular pressure-volume analysis. Left, left ventricular (LV) pressure-volume loops (green line). Right, simultaneous recording of LV volume (blue line) and pressure (red line), from which LV pressure-volume loops were constructed. Red points in the PV loop are the maximal elastance for each cardiac cycle. The slope of these maximum elastance values is obtained during a transient decrease in preload represents the LV end-systolic elastance (Ees). The outlined pressure-volume loops (dark green line) represent five cardiac cycles obtained just before the inferior vena cava (IVC) occlusion (marked with an asterisk above the pressure-volume waveforms) for determining end-systolic pressure, stroke volume, end-diastolic volume, end-systolic volume, and effective arterial elastance (Ea). The dashed line connects the maximal elastance during the IVC maneuver and represents the end-systolic elastance (Ees). The dotted line connects the end-systolic pressure with the end-diastolic volume representing the effective arterial elastance (Ea).
A 5-French pigtail catheter was inserted using fluoroscopy through the right carotid or femoral artery into the aortic arch for continuous measurement of central aortic pressure. The radial artery was surgically exposed and cannulated with a 5-French catheter. Arterial pressure waveforms from the ascending aortic and radial arterial artery were continuously recorded with a fluid-filled pressure transducer (FloTracIQ sensor; Edwards Lifesciences) at a sampling rate of 100 Hz, using the EV1000 monitor (Edwards Lifesciences) and then transferred onto a computer. The pressure signals were filtered to remove noise and artifacts using a 10 Hz low pass filter.
Ventriculo-arterial coupling was defined as the ratio of Ea to Ees (Sunagawa et al., 1983). LV PV area (PVA) represents the total mechanical energy and was determined as the sum of SW and PE, where SW is the area within the PV loop and PE = 0.5 × Pes × (ESV−V0). The ratio of SW/PVA represents the LVeff of converting the total mechanical energy available into SW (Burkhoff and Sagawa, 1986; Asanoi et al., 1989).
Eadyn was calculated as the ratio of PPV calculated from the aortic pressure waveform and the SVV obtained from the conductance catheter. PPV and SVV were calculated as [(PPmax−PPmin)/(PPmax + PPmin)/2] × 100, and SVV = [(SVmax−SVmin)/(SVmax + SVmin)/2] × 100. Where PPmax and SVmax, and PPmin and SVmin represent the maximal and minimal arterial pulse pressure and stroke volume determined during the same respiratory cycle, respectively. PPV and SVV values were obtained simultaneously and averaged during 1-minute just before the IVC occlusion. A peripheral Eadyn was also calculated as the ratio between PPV obtained from the radial arterial line and conductance derived SVV. Although arterial load was globally assessed by Ea, we evaluated the contribution of its main components with a two-element Windkessel model of the arterial system, consisting in the net arterial compliance (Cart = aortic pulse pressure/stroke volume) and total peripheral resistance [TPR = mean aortic pressure (MAP)/CO] as a measure of total LV pressure load (Segers et al., 2002).
Animals were initially resuscitated with colloids (Voluven®, 130/0.4, Fresenius Kabi Deutschland GmbH, Bad Homburg, Germany) until no further increase in CO. Then they were allowed to stabilize for at least 10 min. Stability was defined as heart rate and MAP variation <5% (Monge Garcia et al., 2018). The study protocol consisted of three consecutive stages with two opposite interventions: changes in afterload (phenylephrine and nitroprusside), preload (bleeding and fluid bolus), and contractility (esmolol and dobutamine). The experiment started with the afterload interventions: animals were treated with a phenylephrine infusion (30–120 mg.kg–1.min–1) to increase MAP by 40% mmHg from baseline and were allowed to recover at least for 10 min. Then they were treated with sodium nitroprusside (100–200 mg.kg–1.min–1) to decrease MAP by 40% from baseline but not below 50 mmHg for allowing an adequate hemodynamic tolerance during the IVC occlusions, followed by recovery to baseline status at least for 10 min. Subsequently, for preload interventions, the animals were submitted to stepwise bleeding of 12 ml.kg–1 (50 ml.min–1) and the blood stored into a heparinized sterile bag. Then the blood was reinfused at the same rate and a fluid bolus of 10 ml.kg–1 of a colloid was infused in 5 min. After the fluid administration, the contractility interventions followed: an esmolol infusion was started at 50 μg.kg–1.min–1 and increased until reducing LV dP/dtmax by 50% from its previous value, with a limit dose of 200 μg.kg–1.min–1. Then the esmolol infusion was stopped and, after a minimum period of 10 min for recovery, the animals were treated with a dobutamine infusion (5 μg.kg–1.min–1) to increase LV dP/dtmax by 50%. LV PV loops and arterial pressure waveforms were obtained at each baseline and after each intervention. At the end of the experiment the animals were euthanized with an intravenous dose (1 ml/4.5 kg) of a euthanasia solution (Euthasol vet, 400 mg/ml: 390 mg pentobarbital sodium and 50 mg phenytoin sodium, Virbac, Reading, Carros, France).
Data are expressed as mean ± SD. The normality of data was checked by the Shapiro-Wilk test. The intra-observer variability for thermodilution-derived CO measurements and Ees calculation was analyzed with the intraclass correlation coefficient (ICC) with its 95% confidence interval (95% CI), where a ICC > 0.9 represents an excellent agreement between repeated measurements (Koo and Li, 2016). Since we were interested in the effects of individual interventions, differences during each intervention were assessed by a paired t-test or a Wilcoxon test. Linear mixed-effects model analysis was used for assessing the relationship between continuous variables, using individual animals as a subject for random factors and sequential experimental stages as repeated measurements. This allowed us to consider the correlation between subjects and non-constant variability over time, which is not considered by the standard linear regression analysis. A heterogeneous first-order autoregressive covariance structure was selected based on the lowest corrected Akaike’s Information Criteria value (Fitzmaurice et al., 2011). Model parameters were estimated via the restricted maximum likelihood method and the estimated fixed effect of each parameter was quantified by using its estimated value (95% CI). Least-squares regression analysis and scatter plots were used for describing the relationship between continuous variables. P value < 0.05 was considered statistically significant. Statistical analyses were performed using MedCalc Statistical Software version 19.0.0 (MedCalc Software bvba, Ostend, Belgium; 2019)1 and SPPS (SPSS 21, SPPS Inc., Chicago, IL, United States).
The ICC for thermodilution CO measurements was 0.979 (95%CI: 0.948 to 0.994) at the first calibration and 0.987 (95%CI: 0.965 to 0.996) after fluid administration, respectively. The mean ICC for Ees calculation during all experimental stages was 0.903 (95% CI: 0.822 to 0.985). The mean peak inspiratory was pressure 25 ± 3 cm H2O. Blood gas analysis at the beginning of the experiment was: pH 7.53, pCO2 42 mmHg, pO2 149 mmHg, and HCO3– 32.2 mmol/L.
The evolution of the hemodynamic variables throughout different experimental conditions is detailed in Table 1 (for afterload interventions), Table 2 (for preload interventions), and Table 3 (for contractility interventions). During the afterload interventions, phenylephrine increased MAP by 39 ± 7%, while sodium nitroprusside decreased it by 33 ± 6%. During the preload interventions, bleeding reduced EDV by 10 ± 8%, while reinfusion and fluid administration increased it by 11 ± 21%. In both cases, neither CO nor SV were significantly modified. During contractility interventions, esmolol infusion decreased LV dPdtmax by 49 ± 16%, while dobutamine increased it by 89 ± 23%.
The individual changes in VA coupling and LVeff during each experimental stage are shown in Figure 2. All experimental interventions affected to a different extent both VA coupling and LVeff. These changes were related to the relative variations on Ea and Ees. Afterload interventions mainly modified VA coupling by altering Ea (Table 1), contractility interventions changed VA coupling by isolated variations in Ees (Table 3). Preload modifications, however altered VA coupling in different ways: bleeding decreased VA coupling by reducing Ea, while fluid administration increased VA coupling by decreasing Ees without changing Ea (Table 2). As expected, there was an inverse relationship between VA coupling and LVeff (Figure 3). The maximal LVeff was associated with lower VA coupling values.
Figure 2. Evolution of dynamic arterial elastance, ventricular-arterial coupling and left ventricular efficiency during different experimental interventions. Individual values of dynamic arterial elastance (Eadyn), ventricular-arterial coupling (Ea/Ees) and left ventricular mechanical efficiency during each experimental stage. Black points with bars represent the mean value and the standard deviation. Colored points represent individual changes for each animal.
Figure 3. Scatter plot: linear correlation between ventricular-arterial (VA) coupling and LV mechanical efficiency. Colors inside circles represent different experimental interventions: red, afterload; green: preload; blue: contractility.
Individual changes in Eadyn, PPV, and SVV are shown in Figures 2, 4. Eadyn was significantly modified during afterload and contractility changes, whereas it remained unchanged after bleeding and fluid administration. Sodium nitroprusside increased Eadyn, while phenylephrine decreased it. On the other hand, an improvement in contractility with dobutamine increased Eadyn, while reducing contractility with esmolol decreased Eadyn. The overall correlation between SVV and PPV during the whole study was r = 0.64 (95% CI: 0.533 to 0.729; p < 0.001). Radial and aortic PPV were highly correlated (r = 0.92; 95% CI: 0.890 to 0.941; p < 0.001). There was a significant relationship between PPV (but not SVV) and arterial system properties: a low TPR value and high compliance were associated with a higher PPV (Table 4).
Figure 4. Evolution of pulse pressure variation (PPV) and stroke volume variation (SVV) during different experimental interventions. Individual values of PPV and SVV during each experimental condition. Black points with bars represent the mean value and the standard deviation. Colored points represent individual changes for each animal.
Table 4. Relationship between arterial vascular mechanics on pulse pressure variation (PPV) and stroke volume variation (SVV) according to a mixed-effect regression model.
Table 5 shows the impact of VA coupling and LVeff on PVV, and SVV. While PPV was only inversely related to Ea, SVV was directly associated with Ees. Eadyn was significantly related to VA coupling, LVeff and its determinants (Table 6 and Figure 5). Changes in both Ea and Ees and were associated with changes in Eadyn. A 10% decrease in Ea increased Eadyn by 0.09, while a similar change in Ees was associated with an Eadyn reduction of 0.22. Accordingly, the lower the VA coupling and the better the LVeff, the higher the Eadyn. This relationship remained when using radial PPV for calculating Eadyn (Table 7), including a significant relationship between Eadyn and LVEF (estimate: 0.026, 95% CI: 0.021 to 0.032, p < 0.001).
Table 5. Estimated values of fixed effects on aortic pulse pressure variation (PPV) and stroke volume variation (SVV) according to a mixed-effect regression model.
Table 6. Relationship between ventriculo-arterial coupling and left ventricular efficiency and Eadyn according to a mixed-effect regression model.
Figure 5. Relationship between dynamic arterial elastance, ventricular-arterial (VA) coupling, left ventricular (LV) mechanical efficiency and left ventricular ejection fraction (LVEF). Colors inside circles represent different experimental interventions: red, afterload; green: preload; blue: contractility.
Table 7. Relationship between ventriculo-arterial coupling and left ventricular efficiency and peripheral Eadyn (radial PPV/SVV) according to a mixed-effect regression model.
In this study, we aimed to determine the relationship between Eadyn, VA coupling and LV mechanical efficiency while altering loading and contractile conditions in anesthetized healthy pigs. Our conclusions are twofold. First, Eadyn changes were related to both afterload and LV contractility. The relative contributions of these two factors to both PPV and SVV eventually lead to the observed Eadyn value. These findings agree with previous reports and confirm the compound nature of Eadyn (Monge Garcia et al., 2017a, b). Consequently, Eadyn does not behave as a true measure of LV afterload nor an index of vasomotor tone, but rather a variable resulting from the interaction between LV contractility and the arterial system. Second, it follows therefore that because of the impact of both LV systolic function (Ees) and arterial system properties (Ea), a significant relationship between Eadyn and VA coupling exists with higher Eadyn associated with better VA coupling. Moreover, since the LV oxygen consumption required for a given SW depends on both loading conditions and myocardial contractile state (Burkhoff and Sagawa, 1986), Eadyn was also associated with LV mechanical efficiency. Accordingly, Eadyn increases with improvements in VA coupling and LVeff and decreases when cardiovascular performance and LV mechanical efficiency worsen.
By definition, the nature of Eadyn is determined by how respiration altered both LV SV and arterial pulse pressure. Briefly, respiratory variations in LV SV are the consequence of the cyclic changes in intrathoracic pressure and lung volumes on both right and left ventricular preload and afterload. The magnitude of these variations defines the preload-dependency and depicts the slope of the cardiac function curve (Pinsky, 2012). Considering that arterial pressure is a function of LV outflow and arterial load (Sunagawa et al., 1983), variations in arterial pulse pressure should mainly reflect LV SV changes as long as both arterial system properties and LV ejection pattern remain unchanged (Pinsky, 2005). Therefore, PPV is mostly determined by SVV at a given time, making PPV a valid surrogate for assessing preload-dependency (Marquez et al., 2008; Pinsky, 2012) because variations in the arterial system and LV function during a respiratory cycle are usually negligible (Pinsky, 1997). However, significant changes in contractility and arterial load may occur over longer periods of time. Moreover, clinical conditions such as the use of vasoactive therapy (Kubitz et al., 2008; Renner et al., 2009; Hadian et al., 2011; Monge Garcia et al., 2017a; de Courson et al., 2019; Wodack et al., 2019), acute hemorrhage (Berkenstadt et al., 2005; Renner et al., 2009), fluid administration (Kim and Pinsky, 2008; Monge Garcia et al., 2011; Cecconi et al., 2014; Monge Garcia et al., 2014; Seo et al., 2015), sepsis (Cherpanath et al., 2014) or pharmacological changes in contractility (Mesquida et al., 2011), may alter the interaction between PPV and SVV and therefore modify the eventual value of Eadyn.
In our study, PPV was significantly associated with changes in Ea and related to both TPR and Cart, acting mostly as a peripheral factor, while SVV was associated with Ees, representing more as the central component of Eadyn. Thus, the nature of Eadyn was defined by the interaction between the arterial system and the LV systolic function: while increases in afterload decreases Eadyn, an increase in LV systolic function also increases Eadyn (Figure 6). Consequently, a lower Ea/Ees ratio, i.e., a better VA coupling, would therefore be associated to a higher Eadyn value, while a worse cardiovascular performance (higher VA coupling value) would be related to a lower Eadyn value. Moreover, as LV mechanical efficiency depends on the interaction of the arterial load and LV function (Sunagawa et al., 1985; Burkhoff and Sagawa, 1986), we also found a positive relationship between Eadyn and LVeff. The higher the Eadyn, the higher LVeff. So, at higher Eadyn values the interaction between the LV and the arterial system is associated to a better LV mechanical efficiency, while a decrease in Eadyn would represent a higher energy consumed by the heart to achieve the required SW (lower LVeff).
Figure 6. Representation of the relationship between effective arterial elastance (Ea, blue line), left ventricular end-systolic elastance (Ees, red line) and dynamic arterial elastance (Eadyn). Left: effects of isolated variations on left ventricular afterload (Ea) on Eadyn. Right, effects of isolated changes of left ventricular contractility (Ees). The dashed line and open circle represent the baseline condition. The intersection between Ea and Ees defines the value of Eadyn on the x-axis for each ventriculo-arterial coupling (Ea/Ees) condition.
The first application of the physiological concept of Eadyn on the clinical setting was as an index for predicting the arterial pressure response to fluid administration (Pinsky, 2002; Monge Garcia et al., 2014). The reason behind this idea is that, if variations in SV during mechanical ventilation are followed by proportional or greater changes in arterial pulse pressure, then the likelihood of increasing blood pressure when CO is increased with fluids will be high. On the contrary, if these SV changes are followed by a smaller arterial pulse pressure variations, then the fluid-induced increase in CO will be not followed by an increase in arterial pressure (Pinsky, 2002). In 25 hypotensive and preload-dependent patients receiving a fluid bolus because of acute circulatory failure (Monge Garcia et al., 2011) demonstrated that Eadyn value obtained from arterial waveform analysis was higher in patients that responded increasing both CO and MAP. This same group, using two independent techniques for estimating Eadyn (esophageal Doppler for measuring SVV and radial arterial pressure for PPV), confirmed these findings in 53 mechanically ventilated, preload-dependent patients with acute circulatory failure (Monge Garcia et al., 2014): an Eadyn ≥ 0.73 predicted the subsequent arterial pressure response to fluid administration with a sensitivity of 91% and a specificity of 92%. Seo et al. (2015) reported similar results in 39 patients during robot-assisted laparoscopic prostatectomy: an Eadyn value of 0.74 predicted a MAP increase ≥ 15% after fluid challenge with a sensitivity of 70.6% and a specificity of 86.4%. Vos et al. (2013) in 30 patients undergoing major hepatic resection, demonstrated that Eadyn allowed to predict the reduction in norepinephrine requirement at the end of fluid administration due to a significant increase in MAP. More recently, Guarracino et al. (2019) confirmed the usefulness of Eadyn for predicting the associate change in MAP in response to a CO increase after intravenous fluid infusion in septic patients, suggesting that resuscitation guided by Eadyn may result in a more rational and efficient fluid resuscitation. Under this premise, the Eadyn has been also recently included as part of hemodynamic optimization protocol for reducing intraoperative hypotension (Davies et al., 2019; Maheshwari et al., 2019).
In all previous studies, an Eadyn value > 1 usually represents the ability of increasing both arterial and CO with fluid administration in patients with acute circulatory shock. According to the current results, a high Eadyn value should be associated with a better VA coupling and LVeff. Therefore, in a hypotensive and preload-dependent patient, an Eadyn value > 1 would reflect a cardiovascular system able to increase pressure with flow changes while moving from a better LVeff (lower energy consumption) to a higher SW status (better mechanical efficacy) (Burkhoff and Sagawa, 1986; Asanoi et al., 1989). Thus, a better VA coupling and LVeff conditions would provide favorable conditions for increasing both CO and arterial pressure with fluids. That reasoning would also explain the reduction in Eadyn observed after fluid administration (Kim and Pinsky, 2008; Monge Garcia et al., 2011, 2014; Cecconi et al., 2014; Seo et al., 2015). Kim and Pinsky (2008) reported a decrease in PPV/SVV ratio from 1.47 (PPV 18.2%; SVV 12.4%) to 1.03 (PPV 7.7%; SVV 7.5%) in seven pentobarbital-anesthetized intact dogs submitted to volume loading. These results agreed with those reported in the clinical studies testing the usefulness of Eadyn in hypotensive patients. In the first study of Monge Garcia et al. (2011) Eadyn decreased from 1.34 ± 0.45 to 0.85 ± 0.21 in the group of patients with a significant increase in pressure, whereas it remained unchanged in patients with no change in arterial pressure. A similar observation was made in later studies (Monge Garcia et al., 2014; de Courson et al., 2019). As Eadyn parallels LVeff, a reduction in Eadyn should therefore reflect the transition from a higher LVeff to a better LV mechanical efficacy (Asanoi et al., 1989).
Importantly, although Eadyn appears to be related to the mechanical performance of the coupling between the left ventricle and the arterial circulation, the ability to increase pressure with fluids will depend eventually on the biventricular preload-dependency. If changes in preload are not followed by an increase in CO, then an increase in arterial pressure should not be expected (Monge Garcia et al., 2014). Moreover, as the arterial pressure is tightly regulated, if the reflex pressure control mechanisms are intact, changes in CO should not be followed by significant variations in arterial pressure in normotensive patients (Kubota et al., 1992). However, in those situations in which arterial pressure regulation has been lost, as during hypotension in circulatory shock (Kato and Pinsky, 2015), an increase in CO would result in a rise in arterial pressure depending on the baseline VA coupling. Consequently, the usefulness of Eadyn for predicting arterial pressure response to fluid administration is conditioned by the presence of biventricular preload-dependency and arterial pressure homeostasis regulatory mechanisms.
There have also been negative studies about the usefulness of Eadyn for predicting arterial pressure response to fluid administration. These studies mainly failed because Eadyn was erroneously interpreted as a measure of fluid responsiveness or used in unselected populations. For example, in the study of Stens J et al., Eadyn was evaluated non-invasively with a finger plethysmography device (Nexfin®) for predicting preload-responsiveness after positioning the patient in Trendelenburg position. They found that the degree of fluid responsiveness was not associated with baseline Eadyn values (Stens et al., 2016). Since Trendelenburg maneuvers will increase arterial pressure independent of flow, such negative results are predictable. In 51 patients scheduled for neurosurgery or elective abdominal surgery, Lanchon et al. (2017) showed that Eadyn was unable to predict the MAP increase after volume expansion. However, their analysis was performed without prejudging prior preload-responsiveness and baseline MAP was not reported. If their patients were not hypotensive, then one would not expect arterial pressure to increase if CO also increased. Wu et al. (2016) reported low performance of Eadyn for assessing the arterial pressure response after an intraoperative fluid challenge in 31 liver cirrhosis patients receiving a living donor liver transplant. However, the baseline MAP for preload-dependent patients was 75 (65 to 81 mmHg), which indicates that arterial pressure was already optimized, and the vascular system was likely operating to keep constant pressure, as reflected in the modest MAP increase (from 75 to 77 mmHg) in those 23 patients with a significant increase in SV.
Guinot et al. (2015, 2017) demonstrated that Eadyn was able to predict the MAP decrease associated with a reduction in norepinephrine dosage and, therefore, it could be used as an aid for titrating this vasoactive therapy in patients with septic shock or during vasoplegic syndrome following cardiac surgery. Patients with an Eadyn value > 0.94 were able to sustain MAP despite a decrease in vasopressor support, while patients with a lower Eadyn significantly reduced MAP after decreasing norepinephrine dose. These authors argued that Eadyn can be considered as a functional assessment of arterial tone. Considering the current results, a patient with a higher Eadyn should have better VA coupling and be able to generate an increase in SW that keeps MAP despite the decrease in the external vasopressor support and potentially vasomotor tone. Moreover, these patients showed a decrease in Eadyn after reducing norepinephrine dose, supporting the hypothesis that the cardiovascular system shifted from a better LVeff and to a higher mechanical efficacy for sustaining MAP. In concordance with this hypothesis (Guarracino et al., 2019) have recently shown that Eadyn allowed predicting the arterial pressure response to a norepinephrine infusion in patients with septic shock. An Eadyn value > 0.83, which was associated with a better LVeff and VA coupling conditions estimated by the single beat method and standard echocardiography, allowing them to predict which patients would increase MAP > 15% after the introduction of norepinephrine(Guarracino et al., 2019).
Monge Garcia et al. (2017a) reported the changes in Eadyn during isolated variations in arterial load induced by sodium nitroprusside and phenylephrine in rabbits. In this study, Eadyn behaved in a similar way to that observed in the current study. Eadyn increased during sodium nitroprusside-induced vasodilation and decreased during vasoconstriction with the phenylephrine infusion. These findings are also in agreement with the study of Wodack et al. (2019), in which MAP was stepwise increased with phenylephrine and Eadyn was reduced from 1.06 (0.53) to 0.68 (0.3). Furthermore, in a recent multicenter study (de Courson et al., 2019) have shown that the use of a phenylephrine infusion in 56 surgical patients induced a significant decrease in Eadyn from 0.67 (0.48–0.80) to 0.54 (0.37–0.68). Therefore, our results underscore the message that Eadyn does not represent an index of arterial vasomotor tone nor arterial load, although changes in arterial load may affect Eadyn. The unspoken other conclusion of these findings is that phenylephrine is a poor cardiovascular treatment for sustained hypotension because of its detrimental effects on Eadyn and LVeff.
Only a few studies have directly addressed the impact of contractility changes in the PPV and SVV relationship. Mesquida et al. (2011) studied the effects of propranolol-induced acute ventricular failure on the relation between PPV and SVV in thoracotomized mongrel dogs. According to their results, propranolol infusion decreases Eadyn from 1.16 (PPV 37 ± 24%, SVV 32 ± 16%) to 0.96 (PPV 26 ± 13%, SVV 27 ± 11%). These findings agree with those observed in the sequential changes in PPV and SVV observed by Kim and Pinsky (2008). In their study, the relationship between PPV and SVV was successively altered in anesthetized dogs with esmolol, dobutamine and fluid administration. While fluid administration and esmolol infusion were associated with low PPV/SVV ratios, dobutamine increased Eadyn. Interestingly, the effect of increasing tidal volume from 5 ml/kg to 20 ml/kg also augmented this ratio.
Although the concept of the coupling between the heart and the arterial system has been described for more than 30 years, it has resurged recently in the critical care settings (Guarracino et al., 2013, 2014). This concept offers several potential advantages for the analysis of the cardiovascular system: it defines the behavior of the heart and the arterial system as an interconnected system and not as isolated structures, but it also provides a valid method for evaluating the cardiovascular performance and cardiac energetics relating both cardiac and vascular peripheral functions (Kass and Kelly, 1992).
The cardiovascular system aims to deliver adequate blood flow and pressure to sustain normal physiological functions. Flow is important to deliver oxygen whereas pressure is important to allow for autoregulation of blood flow distribution. Because the interaction of arterial system with the heart, a given arterial pressure and CO that maintains necessary organ perfusion can be achieved by different cardiovascular configurations (Monge Garcia et al., 2016). However, an optimal combination of cardiac function and arterial system state has been described that ensures the maximal mechanical energy transfer from the ventricle to the arterial tree (Guarracino et al., 2013). Previous studies have demonstrated that the best mechanical performance of the cardiovascular system is found when the ventricle and the arterial tree are optimally matched (Sunagawa et al., 1983; Burkhoff and Sagawa, 1986; Elzinga and Westerhof, 1991). Accordingly, VA coupling has been considered as a central determinant of cardiovascular performance (Sunagawa et al., 1983; Starling, 1993).
Our data corroborate earlier reports about the compound nature of Eadyn, being affected by both Ea and Ees, and then significantly related to VA coupling and LVeff. In light of these results, the definition of Eadyn as a dynamic assessment of arterial load (Monge Garcia et al., 2011, 2014, 2017a) could be understood in the same terms that the dynamic indexes of preload-responsiveness are related to cardiac preload. While all these indexes are not actual measures of preload, they are influenced by preload changes (Pinsky, 2012). Similarly, Eadyn cannot be considered as a true measure of afterload, although variations in afterload could affect to it. Therefore, afterload is to Eadyn as what the preload is to the index of preload-dependency.
Our results are in contrast with the recent report published by Bar et al. (2018). These authors retrospectively analyzed their previous results in 28 postoperative cardiac surgery patients suffering from vasoplegic syndrome, and they failed to demonstrate any relationship between Eadyn and VA coupling or its determinants (Guinot et al., 2018). One potential reason for this disagreement is the methodology used for determining Ees. In their study, Ees was estimated by the non-invasive single-beat method described by Chen et al. (2001), which is based on empirical estimation of a normalized population-averaged elastance and the data obtained from standard echocardiography and peripheral blood pressure measurements. Our results, however, were obtained from the well-validated method of the PV loops analysis from multiple-beat recordings during a transient preload reduction.
Our assessment of SVV was based on changes in LV volume obtained by the conductance technique, which requires a correction for the parallel conductance from the surrounding structures that can be determined and subtracted by the hypertonic saline method (Baan et al., 1984). Although changes in lung volume during ventilation could potentially affect the estimation of SV altering parallel conductance independently of the actual LV volume measurements, previous experimental studies have failed to show a systemic impact in parallel dual-field conductance volume signal during positive-pressure ventilation (Szwarc and Ball, 1998; Haney et al., 2006). Therefore, respiratory variation in SV during mechanical ventilation should reflect real SVV in our study. Second, there is a close physiological relationship between SVV and PPV, as the primary determinant of arterial pressure variations is the LV stroke volume changes induced by intermittent positive-pressure ventilation. Therefore, the close numbers could lead to a higher probability of random measurement error, mostly with lower values. Moreover, while PPV is directly obtained from the measurement of arterial pressure waveform, clinically SVV is often estimated using arterial pressure pulse-contour analysis or Doppler echocardiography. Therefore, the reliability of Eadyn will eventually rely on the accuracy of the SVV estimation and PPV measurements. Third, we focused only on the impact of different hemodynamic conditions on Eadyn during mechanical ventilation, but we did not evaluate how mechanical ventilation per se or respiratory mechanics could affect to the PPV/SVV relationship. Since we kept tidal volume and respiratory rate constant during each study and the lung and chest wall mechanics of each animal were normal, we suspect that the secondary impact of mechanical ventilation of cardiovascular state, independent of specific phasic changes in venous return induced by the small cyclic change in intrathoracic pressure, would be minimal. Finally, although our experimental protocol was designed to cover a broad range of hemodynamic conditions on an intact cardiovascular system, it may not reflect the complexity of a pathophysiological process, such as septic shock. Moreover, although we kept the level of anesthesia as low as possible during different experimental conditions, the study was performed in healthy female pigs submitted to anesthetic agents with known cardiovascular effects and potential influence over the visceral reflexes, so extrapolation of our results to human cardiovascular physiology should be done cautiously. Still, our data parallels the findings of several clinical studies. Furthermore, the influence of hyperoxia on the observed hemodynamic changes was unlikely, since the oxygen level, though not hypoxic, was not overly high to alter the cardiovascular system. Moreover, gender and age differences have been described in ventriculo-arterial coupling (Najjar et al., 2004). However, although we have no reference to analyze such differences, our 5.5 ± 0.8 months old female pigs were not subjected a long-term exposure to sex hormones (Andersson et al., 1999), which potentially may lead to lasting changes in the cardiovascular function of adult animals. Therefore, the influence of the pig gender in our study was likely negligible.
Absolute Eadyn values and their change are defined by the combined effect of the LV contractility and LV afterload. Consequently, a significant relationship between Eadyn, VA coupling and LV mechanical efficiency exists. Our results not only provide the physiological background for understanding Eadyn but also explain previous clinical results. Moreover, the concept of Eadyn as a potential easily measured bedside tool for monitoring VA coupling and LVeff would allow to explore new applications of Eadyn as an index of cardiovascular performance and VA coupling.
The raw data supporting the conclusions of this article are available to any qualified researcher from the corresponding author upon reasonable request.
The study was reviewed and approved by the Institutional Animal Care and Use Committee (IACUC) at the Edwards Research Center, and all procedures were performed in accordance with the USDA Animal Welfare Act regulations (AWArs), and the Guide for the Care and Use of Laboratory Animals (ILAR, NAP, Washington, DC, 2010, 8th edition).
MMG, MP, and MC contributed to the conception of the study. MMG, ZJ, and MP contributed to the design of the study. MMG, ZJ, and FH performed experimental research. MMG, ZJ, FH, JS, and MP analyzed and interpreted the data. All authors drafted the manuscript, reviewed it, contributed significantly to its critical review, approved the final version of the manuscript and ensure the accuracy or integrity of the results of this study and will be accountable for any question related to this work.
Edwards Lifesciences provided the software, hardware, and the animals for the study.
MMG was a consultant to Edwards Lifesciences and received honoraria and or travel expenses from Deltex Medical. MP was a consultant to Edwards Lifesciences, LiDCO Ltd., and Cheetah. MC has received honoraria and/or travel expenses from Edwards Lifesciences, LiDCO, Cheetah, Bmeye, Masimo, and Deltex Medical. ZJ, JS, and FH are Edwards Lifesciences employees.
We are grateful to all laboratory staff of the Edwards Research Center for their valuable assistance in this work, especially to Mr. Fernando García, Senior Research Specialist at Edwards Lifesciences, for his valuable support during and after the experiments.
Cart, net arterial compliance; CI, confidence interval; CO, cardiac output; dP/dtmax, Maximal ventricular pressure rise; E, elastance; Ea, effective arterial elastance; Eadyn, dynamic arterial elastance; EDP, end-diastolic pressure; EDV, end-diastolic volume; Ees, end-systolic ventricular elastance; ESPVR, end-systolic pressure-volume relationship; ESV, end-systolic volume; IVC, inferior vena cava; LV, left ventricle; LVEF, left ventricular ejection fraction; LVeff, left ventricular mechanical efficiency; MAP, mean arterial pressure; P, pressure; PE, potential energy; Pes, end-systolic pressure; PPV, pulse pressure variation; PV, pressure-volume; PVA, pressure-volume area; SD, standard deviation; SV, stroke volume; SVV, stroke volume variation; SW, stroke work; TRP, total peripheral resistance; V, volume; V0, ventricular unstressed volume; VA, ventriculo-arterial.
Andersson, H. K., Hullberg, A., Malmgren, L., Lundström, K., Rydhmer, L., and Squires, J. (1999). Sexual maturity in entire male pigs: environmental effects, relations to skatole level and female puberty. Acta Agric. Scand. A Anim. Sci. 49, 103–112. doi: 10.1080/090647099424169
Asanoi, H., Sasayama, S., and Kameyama, T. (1989). Ventriculoarterial coupling in normal and failing heart in humans. Circ. Res. 65, 483–493. doi: 10.1161/01.res.65.2.483
Baan, J., van der Velde, E. T., de Bruin, H. G., Smeenk, G. J., Koops, J., van Dijk, A. D., et al. (1984). Continuous measurement of left ventricular volume in animals and humans by conductance catheter. Circulation 70, 812–823. doi: 10.1161/01.cir.70.5.812
Bar, S., Huette, P., Abou-Arab, O., Dupont, H., Lorne, E., and Guinot, P. G. (2018). Dynamic arterial elastance might not be an indicator of ventriculo-arterial coupling. Br. J. Anaesth. 121, 672–673. doi: 10.1016/j.bja.2018.03.027.
Berkenstadt, H., Friedman, Z., Preisman, S., Keidan, I., Livingstone, D., and Perel, A. (2005). Pulse pressure and stroke volume variations during severe haemorrhage in ventilated dogs. Br. J. Anaesth. 94, 721–726. doi: 10.1093/bja/aei116
Burkhoff, D., and Sagawa, K. (1986). Ventricular efficiency predicted by an analytical model. Am. J. Physiol. 250(6 Pt 2), R1021–R1027. doi: 10.1152/ajpregu.1986.250.6.R1021
Cecconi, M., Monge Garcia, M. I., Gracia Romero, M., Mellinghoff, J., Caliandro, F., Grounds, R. M., et al. (2014). The use of pulse pressure variation and stroke volume variation in spontaneously breathing patients to assess dynamic arterial elastance and to predict arterial pressure response to fluid administration. Anesth. Analg. 120, 76–84. doi: 10.1213/ANE.0000000000000442
Chen, C. H., Fetics, B., Nevo, E., Rochitte, C. E., Chiou, K. R., Ding, P. A., et al. (2001). Noninvasive single-beat determination of left ventricular end-systolic elastance in humans. J. Am. Coll. Cardiol. 38, 2028–2034. doi: 10.1016/s0735-1097(01)01651-5
Cherpanath, T. G., Smeding, L., Lagrand, W. K., Hirsch, A., Schultz, M. J., and Groeneveld, J. A. (2014). Pulse pressure variation does not reflect stroke volume variation in mechanically ventilated rats with lipopolysaccharide-induced pneumonia. Clin. Exp. Pharmacol. Physiol. 41, 98–104. doi: 10.1111/1440-1681.12187
Davies, S. J., Vistisen, S. T., Jian, Z., Hatib, F., and Scheeren, T. W. L. (2019). Ability of an arterial waveform analysis-derived hypotension prediction index to predict future hypotensive events in surgical patients. Anesth. Analg. 130, 352–359. doi: 10.1213/ANE.0000000000004121
de Courson, H., Boyer, P., Grobost, R., Lanchon, R., Sesay, M., Nouette-Gaulain, K., et al. (2019). Changes in dynamic arterial elastance induced by volume expansion and vasopressor in the operating room: a prospective bicentre study. Ann. Intensive Care 9:117. doi: 10.1186/s13613-019-0588-6
Elzinga, G., and Westerhof, N. (1991). Matching between ventricle and arterial load. An evolutionary process. Circ. Res. 68, 1495–1500. doi: 10.1161/01.res.68.6.1495
Fitzmaurice, G. M., Laird, N. M., and Ware, J. H. (2011). Modeling the Covariance. Applied Longitudinal Analysis, 2nd Edn. Hoboken, NJ: Wiley, 165–188.
Guarracino, F., Baldassarri, R., and Pinsky, M. R. (2013). Ventriculo-arterial decoupling in acutely altered hemodynamic states. Crit. Care 17:213. doi: 10.1186/cc12522
Guarracino, F., Bertini, P., and Pinsky, M. R. (2019). Cardiovascular determinants of resuscitation from sepsis and septic shock. Crit. Care 23:118. doi: 10.1186/s13054-019-2414-9
Guarracino, F., Ferro, B., Morelli, A., Bertini, P., Baldassarri, R., and Pinsky, M. R. (2014). Ventriculoarterial decoupling in human septic shock. Crit. Care 18:R80. doi: 10.1186/cc13842
Guinot, P. G., Abou-Arab, O., Guilbart, M., Bar, S., Zogheib, E., Daher, M., et al. (2017). Monitoring dynamic arterial elastance as a means of decreasing the duration of norepinephrine treatment in vasoplegic syndrome following cardiac surgery: a prospective, randomized trial. Intensive Care Med. 43, 643–651. doi: 10.1007/s00134-016-4666-z
Guinot, P. G., Bernard, E., Levrard, M., Dupont, H., and Lorne, E. (2015). Dynamic arterial elastance predicts mean arterial pressure decrease associated with decreasing norepinephrine dosage in septic shock. Crit. Care 19:14. doi: 10.1186/s13054-014-0732-5
Guinot, P. G., Longrois, D., Kamel, S., Lorne, E., and Dupont, H. (2018). Ventriculo-arterial coupling analysis predicts the hemodynamic response to norepinephrine in hypotensive postoperative patients: a prospective observational study. Crit. Care Med. 46, e17–e25. doi: 10.1097/CCM.0000000000002772
Hadian, M., Severyn, D. A., and Pinsky, M. R. (2011). The effects of vasoactive drugs on pulse pressure and stroke volume variation in postoperative ventilated patients. J. Crit. Care 26, 328.e1–e8. doi: 10.1016/j.jcrc.2010.08.018
Haney, M. F., Steendijk, P., Johansson, G., and Biber, B. (2006). The effect of lung inflation on absolute ventricular volume measurement by conductance. Clin. Physiol. Funct. Imaging 26, 220–223. doi: 10.1111/j.1475-097X.2006.00681.x
Kass, D. A., and Kelly, R. P. (1992). Ventriculo-arterial coupling: concepts, assumptions, and applications. Ann. Biomed. Eng. 20, 41–62. doi: 10.1007/bf02368505
Kass, D. A., Yamazaki, T., Burkhoff, D., Maughan, W. L., and Sagawa, K. (1986). Determination of left ventricular end-systolic pressure-volume relationships by the conductance (volume) catheter technique. Circulation 73, 586–595. doi: 10.1161/01.cir.73.3.586
Kato, R., and Pinsky, M. R. (2015). Personalizing blood pressure management in septic shock. Ann. Intensive Care 5:41. doi: 10.1186/s13613-015-0085-5
Kim, H. K., and Pinsky, M. R. (2008). Effect of tidal volume, sampling duration, and cardiac contractility on pulse pressure and stroke volume variation during positive-pressure ventilation. Crit. Care Med. 36, 2858–2862. doi: 10.1097/CCM.0b013e3181865aea
Koo, T. K., and Li, M. Y. (2016). A guideline of selecting and reporting intraclass correlation coefficients for reliability research. J. Chiropr. Med. 15, 155–163. doi: 10.1016/j.jcm.2016.02.012
Kubitz, J. C., Forkl, S., Annecke, T., Kronas, N., Goetz, A. E., and Reuter, D. A. (2008). Systolic pressure variation and pulse pressure variation during modifications of arterial pressure. Intensive Care Med. 34, 1520–1524. doi: 10.1007/s00134-008-1114-8
Kubota, T., Alexander, J. Jr., Itaya, R., Todaka, K., Sugimachi, M., Sunagawa, K., et al. (1992). Dynamic effects of carotid sinus baroreflex on ventriculoarterial coupling studied in anesthetized dogs. Circ. Res. 70, 1044–1053. doi: 10.1161/01.res.70.5.1044
Lanchon, R., Nouette-Gaulain, K., Stecken, L., Sesay, M., Lefrant, J. Y., and Biais, M. (2017). Dynamic arterial elastance obtained using arterial signal does not predict an increase in arterial pressure after a volume expansion in the operating room. Anaesth. Crit. Care. Pain Med. 36, 377–382. doi: 10.1016/j.accpm.2017.05.001
Maheshwari, K., Shimada, T., Fang, J., Ince, I., Mascha, E. J., Turan, A., et al. (2019). Hypotension prediction index software for management of hypotension during moderate- to high-risk noncardiac surgery: protocol for a randomized trial. Trials 20:255. doi: 10.1186/s13063-019-3329-0
Marquez, J., McCurry, K., Severyn, D. A., and Pinsky, M. R. (2008). Ability of pulse power, esophageal Doppler, and arterial pulse pressure to estimate rapid changes in stroke volume in humans. Crit. Care Med. 36, 3001–3007. doi: 10.1097/CCM.0b013e31818b31f0
Mesquida, J., Kim, H. K., and Pinsky, M. R. (2011). Effect of tidal volume, intrathoracic pressure, and cardiac contractility on variations in pulse pressure, stroke volume, and intrathoracic blood volume. Intensive Care Med. 37, 1672–1679. doi: 10.1007/s00134-011-2304-3
Monge Garcia, M., Gracia Romero, M., Gil Cano, A., Aya, H. D., Rhodes, A., Grounds, R., et al. (2014). Dynamic arterial elastance as a predictor of arterial pressure response to fluid administration: a validation study. Crit. Care 18:626. doi: 10.1186/s13054-014-0626-6
Monge Garcia, M. I., Gil Cano, A., and Gracia Romero, M. (2011). Dynamic arterial elastance to predict arterial pressure response to volume loading in preload-dependent patients. Crit. Care 15:R15. doi: 10.1186/cc9420
Monge Garcia, M. I., Guijo Gonzalez, P., Gracia Romero, M., Gil Cano, A., Rhodes, A., Grounds, R. M., et al. (2017a). Effects of arterial load variations on dynamic arterial elastance: an experimental study. Br. J. Anaesth. 118, 938–946. doi: 10.1093/bja/aex070
Monge Garcia, M. I., Jian, Z., Settels, J. J., Hunley, C., Cecconi, M., Hatib, F., et al. (2018). Performance comparison of ventricular and arterial dP/dtmax for assessing left ventricular systolic function during different experimental loading and contractile conditions. Crit. Care 22:325. doi: 10.1186/s13054-018-2260-1
Monge Garcia, M. I., Pinsky, M. R., and Cecconi, M. (2017b). Predicting vasopressor needs using dynamic parameters. Intensive Care Med. 43, 1841–1843. doi: 10.1007/s00134-017-4752-x
Monge Garcia, M. I., Saludes Orduna, P., and Cecconi, M. (2016). Understanding arterial load. Intensive Care Med. 42, 1625–1627. doi: 10.1007/s00134-016-4212-z
Najjar, S. S., Schulman, S. P., Gerstenblith, G., Fleg, J. L., Kass, D. A., O’Connor, F., et al. (2004). Age and gender affect ventricular-vascular coupling during aerobic exercise. J. Am. Coll. Cardiol. 44, 611–617. doi: 10.1016/j.jacc.2004.04.041
NC3Rs Reporting Guidelines Working Group (2010). Animal research: reporting in vivo experiments: the ARRIVE guidelines. J. Physiol. 588(Pt 14), 2519–2521. doi: 10.1113/jphysiol.2010.192278
Pinsky, M. R. (1997). The hemodynamic consequences of mechanical ventilation: an evolving story. Intensive Care Med. 23, 493–503. doi: 10.1007/s001340050364
Pinsky, M. R. (2002). “Functional hemodynamic monitoring: applied physiology at the bedside,” in Yearbook of Intensive Care and Emergency Medicine, ed. J. L. Vincent (Heidelberg: Springer-Verlag), 534–551.
Pinsky, M. R. (2005). The dynamic interface between hemodynamic variables and autonomic tone. Crit. Care Med. 33, 2437–2438. doi: 10.1097/01.ccm.0000182899.42273.4e
Pinsky, M. R. (2006). “Protocolized cardiovascular management based on ventricular-arterial coupling,” in Functional Hemodynamic Monitoring. Update in Intensive Care Medicine, eds M. R. Pinsky and D. Payen (Berlin: Springer-Verlag), 381–395. doi: 10.1007/3-540-26900-2_28
Pinsky, M. R. (2012). Heart lung interactions during mechanical ventilation. Curr. Opin. Crit. Care 18, 256–260. doi: 10.1097/MCC.0b013e3283532b73
Renner, J., Meybohm, P., Hanss, R., Gruenewald, M., Scholz, J., and Bein, B. (2009). Effects of norepinephrine on dynamic variables of fluid responsiveness during hemorrhage and after resuscitation in a pediatric porcine model. Paediatr. Anaesth. 19, 688–694. doi: 10.1111/j.1460-9592.2009.03017.x
Segers, P., Stergiopulos, N., and Westerhof, N. (2002). Relation of effective arterial elastance to arterial system properties. Am. J. Physiol. Heart Circ. Physiol. 282, H1041–H1046. doi: 10.1152/ajpheart.00764.2001
Seo, H., Kong, Y. G., Jin, S. J., Chin, J. H., Kim, H. Y., Lee, Y. K., et al. (2015). Dynamic arterial elastance in predicting arterial pressure increase after fluid challenge during robot-assisted laparoscopic prostatectomy: a prospective observational study. Medicine (Baltimore) 94:e1794. doi: 10.1097/MD.0000000000001794
Starling, M. R. (1993). Left ventricular-arterial coupling relations in the normal human heart. Am. Heart J. 125, 1659–1666. doi: 10.1016/0002-8703(93)90756-y
Stens, J., Oeben, J., Van Dusseldorp, A. A., and Boer, C. (2016). Non-invasive measurements of pulse pressure variation and stroke volume variation in anesthetized patients using the Nexfin blood pressure monitor. J. Clin. Monit. Comput. 30, 587–594. doi: 10.1007/s10877-015-9759-7
Sunagawa, K., Maughan, W. L., Burkhoff, D., and Sagawa, K. (1983). Left ventricular interaction with arterial load studied in isolated canine ventricle. Am. J. Physiol. 245(5 Pt 1), H773–H780.
Sunagawa, K., Maughan, W. L., and Sagawa, K. (1985). Optimal arterial resistance for the maximal stroke work studied in isolated canine left ventricle. Circ. Res. 56, 586–595. doi: 10.1161/01.res.56.4.586
Sunagawa, K., Sagawa, K., and Maughan, W. L. (1984). Ventricular interaction with the loading system. Ann. Biomed. Eng. 12, 163–189. doi: 10.1007/bf02584229
Szwarc, R. S., and Ball, H. A. (1998). Simultaneous LV and RV volumes by conductance catheter: effects of lung insufflation on parallel conductance. Am. J. Physiol. 275(2 Pt 2), H653–H661. doi: 10.1152/ajpheart.1998.275.2.H653
Vos, J. J., Kalmar, A. F., Struys, M. M., Wietasch, J. K., Hendriks, H. G., and Scheeren, T. W. (2013). Comparison of arterial pressure and plethysmographic waveform-based dynamic preload variables in assessing fluid responsiveness and dynamic arterial tone in patients undergoing major hepatic resection. Br. J. Anaesth. 110, 940–946. doi: 10.1093/bja/aes508
Wodack, K. H., Graessler, M. F., Nishimoto, S. A., Behem, C. R., Pinnschmidt, H. O., Punke, M. A., et al. (2019). Assessment of central hemodynamic effects of phenylephrine: an animal experiment. J. Clin. Monit. Comput. 33, 377–384. doi: 10.1007/s10877-018-0204-6
Wu, C. Y., Cheng, Y. J., Liu, Y. J., Wu, T. T., Chien, C. T., Chan, K. C., et al. (2016). Predicting stroke volume and arterial pressure fluid responsiveness in liver cirrhosis patients using dynamic preload variables: a prospective study of diagnostic accuracy. Eur. J. Anaesthesiol. 33, 645–652. doi: 10.1097/EJA.0000000000000479
Keywords: ventricular-arterial coupling, left ventricular efficiency, dynamic arterial elastance, arterial load, effective arterial elastance, ventricular elastance
Citation: Monge García MI, Jian Z, Hatib F, Settels JJ, Cecconi M and Pinsky MR (2020) Dynamic Arterial Elastance as a Ventriculo-Arterial Coupling Index: An Experimental Animal Study. Front. Physiol. 11:284. doi: 10.3389/fphys.2020.00284
Received: 03 January 2020; Accepted: 13 March 2020;
Published: 06 April 2020.
Edited by:
Alberto Giannoni, Gabriele Monasterio Tuscany Foundation (CNR), ItalyReviewed by:
Vincenzo Lionetti, Sant’Anna School of Advanced Studies, ItalyCopyright © 2020 Monge García, Jian, Hatib, Settels, Cecconi and Pinsky. This is an open-access article distributed under the terms of the Creative Commons Attribution License (CC BY). The use, distribution or reproduction in other forums is permitted, provided the original author(s) and the copyright owner(s) are credited and that the original publication in this journal is cited, in accordance with accepted academic practice. No use, distribution or reproduction is permitted which does not comply with these terms.
*Correspondence: Manuel Ignacio Monge García, aWduYWNpb21vbmdlQGdtYWlsLmNvbQ==
Disclaimer: All claims expressed in this article are solely those of the authors and do not necessarily represent those of their affiliated organizations, or those of the publisher, the editors and the reviewers. Any product that may be evaluated in this article or claim that may be made by its manufacturer is not guaranteed or endorsed by the publisher.
Research integrity at Frontiers
Learn more about the work of our research integrity team to safeguard the quality of each article we publish.