- 1Shelf Sea System Ecology, Alfred-Wegener-Institut Helmholtz-Zentrum für Polar- und Meeresforschung, Helgoland, Germany
- 2Institute for Sea Fisheries, Thünen Institute, Bremerhaven, Germany
- 3Nature and Biodiversity Conservation Union, Berlin, Germany
Oxygen Minimum Zones prevail in most of the world’s oceans and are particularly extensive in Eastern Boundary Upwelling Ecosystems such as the Humboldt and the Benguela upwelling systems. In these regions, euphausiids are an important trophic link between primary producers and higher trophic levels. The species are known as pronounced diel vertical migrators, thus facing different levels of oxygen and temperature within a 24 h cycle. Declining oxygen levels may lead to vertically constrained habitats in euphausiids, which consequently will affect several trophic levels in the food web of the respective ecosystem. By using the regulation index (RI), the present study aimed at investigating the hypoxia tolerances of different euphausiid species from Atlantic, Pacific as well as from Polar regions. RI was calculated from 141 data sets and used to differentiate between respiration strategies using median and quartile (Q) values: low degree of oxyregulation (0.25 < RI median < 0.5); high degree of oxyregulation (0.5 < RI median < 1; Q1 > 0.25 or Q3 > 0.75); and metabolic suppression (RI median, Q1 and Q3 < 0). RI values of the polar (Euphausia superba, Thysanoessa inermis) and sub-tropical (Euphausia hanseni, Nyctiphanes capensis, and Nematoscelis megalops) species indicate a high degree of oxyregulation, whereas almost perfect oxyconformity (RI median ≈ 0; Q1 < 0 and Q3 > 0) was identified for the neritic temperate species Thysanoessa spinifera and the tropical species Euphausia lamelligera. RI values of Euphausia distinguenda and the Humboldt species Euphausia mucronata qualified these as metabolic suppressors. RI showed a significant impact of temperature on the respiration strategy of E. hanseni from oxyregulation to metabolic suppression. The species’ estimated hypoxia tolerances and the degree of oxyconformity vs. oxyregulation were linked to diel vertical migration behavior and the temperature experienced during migration. The results highlight that the euphausiid species investigated have evolved various strategies to deal with different levels of oxygen, ranging from species showing a high degree of oxyconformity to strong oxyregulation. Neritic species may be more affected by hypoxia, as these are often short-distance-migrators and only adapted to a narrow range of environmental conditions.
Introduction
Oxygen concentration and water temperature are two important abiotic factors influencing several physiological processes, such as metabolic rate, energy expenditure, as well as the horizontal and vertical distribution of animals living in the world’s oceans (Torres and Childress, 1983; Claireaux and Lagardère, 1999; Ekau et al., 2010). However, both factors are not evenly distributed and temperature and oxygen levels at the surface area are usually higher, compared to deeper water layers. Water temperature is influenced by solar radiation, i.e., latitude and water turbulence. In contrast, oxygen concentration is affected by physical replenishment (mixing), bacterial decomposition and animal respiration. Temperature and oxygen profiles of the water column show a more or less steady decline from upper to deeper water layers (weak thermo- and oxycline) or a more saltatory pattern (strong thermo- and oxycline). In the oceans, the depth and strength of the thermocline vary between season and year. It is semi-permanent in the tropics, variable in temperate regions, and shallow to non-existent in Polar regions. High oxygen concentrations are found at high latitudes, whereas at mid-latitudes, in particular off the western coasts of the continents, oxygen-deficient zones, so-called Oxygen Minimum Zones (OMZs), prevail. Consequently, the ecosystems in the world’s oceans are characterized by distinct oxygen and temperature regimes shaping the different species’ behavior, distribution and physiological processes.
In the anticipated future, anthropogenic induced changes, such as rising nutrient loads coupled with climate change, will cause regional declines in oceanic dissolved oxygen, mainly due to increased stratification and reduced mixing, and an increase in water temperature (Diaz and Rosenberg, 2008; Keeling et al., 2010). Increasing temperature is known to negatively impact the hypoxia tolerance of animals and at the same time raise their energy expenditures. Furthermore, as water temperature rises, oxygen solubility decreases. Thus, decreasing oxygen levels accompanied by increasing temperatures may affect key processes and trophic interactions including community composition, energy flows, migration patterns, and consequently biogeochemical processes (Ekau et al., 2018) and will exert significant pressure on pelagic communities. This applies particularly to planktonic species, such as euphausiids, which cannot, or only to a very limited degree, escape unfavorable environmental conditions (Verheye and Ekau, 2005). As a consequence, it is expected that some areas may experience a shift from an abundant and diverse regime to one that is lean and dominated by vertical migrators (Wishner et al., 2013; Elder and Seibel, 2015).
Evaluation of time series already revealed vertical expansion of OMZs during the last decades (Stramma et al., 2008). It is assumed that these OMZs will further expand, which can happen horizontally into areas previously not experiencing hypoxic conditions, or consist of vertical expansion of an existing OMZ, while coastal hypoxia will increase in extent and severity (Levin, 2018). Compared to other hypoxic habitats, the particular nature of such an OMZ is that it is characterized by moderate to severe hypoxia (<2 mg O2 L–1) over very large areas (∼8% of total oceanic area; Paulmier and Ruiz-Pino, 2009) and over long time periods. They differ from the “dead zones” phenomena caused by anthropogenic coastal eutrophication found, e.g., in the Gulf of Mexico (Rabalais et al., 2002; Diaz and Rosenberg, 2008). OMZs are permanent midwater features occurring at intermediate depth (300–2,500 m) in most of the oceans (Emelyanov, 2005). The largest and most pronounced OMZs are located in the Northern Indian Ocean, the Eastern Atlantic off northwest Africa, and the Eastern Tropical Pacific (ETP) (Wyrtki, 1962; Kamykowski and Zentara, 1990; Olson et al., 1993). Notably, the OMZ of the ETP and the Eastern Atlantic off northwest Africa have expanded to higher latitudes during the past 50 years (Stramma et al., 2008), suggesting changes in zoogeographic distribution patterns, compression of habitats, and restricted zones of biomass production (Prince and Goodyear, 2006; Koslow et al., 2011; Stramma et al., 2011; Gilly et al., 2013). The shallow and severe OMZ in the ETP is due to the poor lateral ventilation of surface waters (Reid, 1965; Luyten et al., 1983) and the formation of a strong thermocline, which limits O2 diffusion into the deeper layers of the ocean (Lavín et al., 2006). Very high temperatures at the surface result in strong stratification, at which the zooplankton aggregate and locally increase the oxygen consumption (Bianchi et al., 2013). At this depth, oxygen is consumed faster than it is replaced by the horizontal mixing of the water mass (Wyrtki, 1962; Fiedler and Talley, 2006; Karstensen et al., 2008), creating the shallow OMZ. The oxygen utilization is particularly enhanced during El Niño-Southern Oscillation and inter-annual changes in upwelling conditions, thus partly explaining the vertical OMZ expansion of the ETP since the 1980s (Ito and Deutsch, 2013).
Compared to Eastern Boundary Upwelling Systems (EBUEs), such as the California, Humboldt, and Benguela Current ecosystems with their pronounced OMZs, the oxygen levels of Polar regions are higher and water temperatures are much lower. No real OMZs exist in these areas and species living there may not be forced to develop adaptations to cope with low oxygen levels. However, mild-hypoxia (50% air saturation) was reported in the Indian sector of the Southern Ocean at depth greater than 500 m (Dehairs et al., 1990) and deoxygenation in the Southern Ocean is currently taking place at 200–400 m depth between 50 and 60° of latitude (Matear et al., 2000; Aoki, 2005). In the Artic, the potential effects of global warming and changes in deep-sea circulation on the oxygenation of the deep ocean is monitored continuously in Fram Strait, West Spitsbergen, the only deep connection between the central Arctic Ocean and the Nordic Seas (Friedrich et al., 2014). The Arctic ecosystem is far from being classified as hypoxic, but strong increase in the annual mean net heat transport within the waters of the West Spitsbergen Current could potentially affect oxygen levels to less than 80% air saturation.
Euphausiids, or krill, are distributed ubiquitously across the globe and often dominate zooplankton communities in terms of abundance and biomass throughout the world‘s oceans. Euphausiids form a pivotal component of many food webs and are known as pronounced diel vertical migrators, thereby contributing to the vertical flux of carbon and facing different levels of oxygen and temperature within a 12 h period. During diel vertical migration (DVM), many euphausiid species cross pronounced gradients of temperature, salinity, and oxygen indicating that these species must be of a broad ecophysiological plasticity. In this regard, euphausiids are ideal model organisms for studying the interactions between organismal and environmental variability (Mangel and Nicol, 2000). Euphausiids and other taxa living in areas with pronounced OMZs have to physiologically and/or behaviorally adapt to low oxygen levels or will be excluded from these areas or at least their vertical distribution ranges will be limited. A typical euphausiid DVM pattern consists of an upward migration at dusk to feed in the upper, productive layers of the oceans, and a downward movement at dawn to avoid visual predators (Zaret and Suffern, 1976; Ohman, 1984), decreasing at the same time their metabolic rates due to the lower water temperature and O2 concentrations (McLaren, 1963; Enright, 1977). Euphausiids channel energy from lower (phytoplankton, small zooplankton) to higher (fish, birds, and even whales) trophic levels. Accordingly, as varying oxygen and temperature levels will likely alter these species’ vertical and horizontal distribution ranges, this may impact a larger part of the whole food web, and even impinge on fisheries yield.
Adaptations of animals to low dissolved oxygen concentrations are driven by strong selective pressures to maintain aerobic metabolism (Seibel, 2011). Most animals facing low oxygen concentrations respond either by decreasing their oxygen consumption rates, known as oxyconformity, or by maintaining a constant oxygen uptake irrespective of the ambient oxygen levels, known as oxyregulation. However, as analyzed mathematically by Cobbs and Alexander (2018) using/applying seven different functions and as discussed by Wood (2018), animals seldom show perfect oxyconformity or oxyregulation. Accordingly, species’ metabolic responses to declining oxygen levels lay somewhere between the two ends of this continuum (Mueller and Seymour, 2011). Furthermore, at a certain species-specific oxygen pressure, animals are unable to maintain their normoxic metabolic rate and have to goose anaerobic metabolism. This point is called ‘critical oxygen partial pressure’ (Pcrit) and can be determined by analyzing the response of the metabolic rate (respiration) to declining oxygen concentrations. Oxyconformers do not regulate their oxygen demand as, physiologically, these species do not need to enhance the transport of oxygen to the metabolizing tissues when oxygen is decreasing. Thus, the capability of an animal to either regulate its oxygen uptake in combination with the Pcrit value or reduce its respiration rate when ambient oxygen levels decrease provides meaningful information about their ability to survive hypoxic events and represent an important ecological tipping point to understand the resilience of populations to declining levels of oxygen (Mueller and Seymour, 2011). A third strategy called metabolic suppression entails the suppression of total energy consumption by shutting down intensive energy demanding processes (Seibel, 2011; Seibel et al., 2016). This strategy has been observed in euphausiid species inhabiting regions where oxygen decline was faster than euphausiid oxygen demands (Seibel et al., 2016).
In this paper, we aim to characterize the hypoxia tolerance of 10 dominant euphausiid species from the Atlantic and the Pacific Ocean, including three prominent EBUEs (Benguela, California, and the Humboldt Current system), and both Polar regions at in situ temperatures by analyzing the regulation index (RI) to explain the DVM behavior in their habitat.
Materials and Methods
Ten euphausiid species were collected between 2010 and 2013 during several small- or large-scale expeditions to the Benguela, California, and Humboldt Current systems (BCS, CCS, and HCS), the Eastern Tropical Pacific (ETP), the Arctic and the Antarctic (details compiled in Table 1). Polar (Antarctic and Arctic: between −0.5°C and 5.5°C), temperate (NCCS and HCS: between 6.5°C and 15.1°C), sub-tropical (BCS: between 8.0°C and 21°C), and tropical (ETP: between 14.6°C and 30.2°C) temperature gradients as well as different hypoxic conditions (severe and shallow: ETP and HCS; severe and deep: BCS; moderate: NCCS; and non-existent: Antarctica and Arctic) are thus covered by the habitat of the species studied (Figure 1).
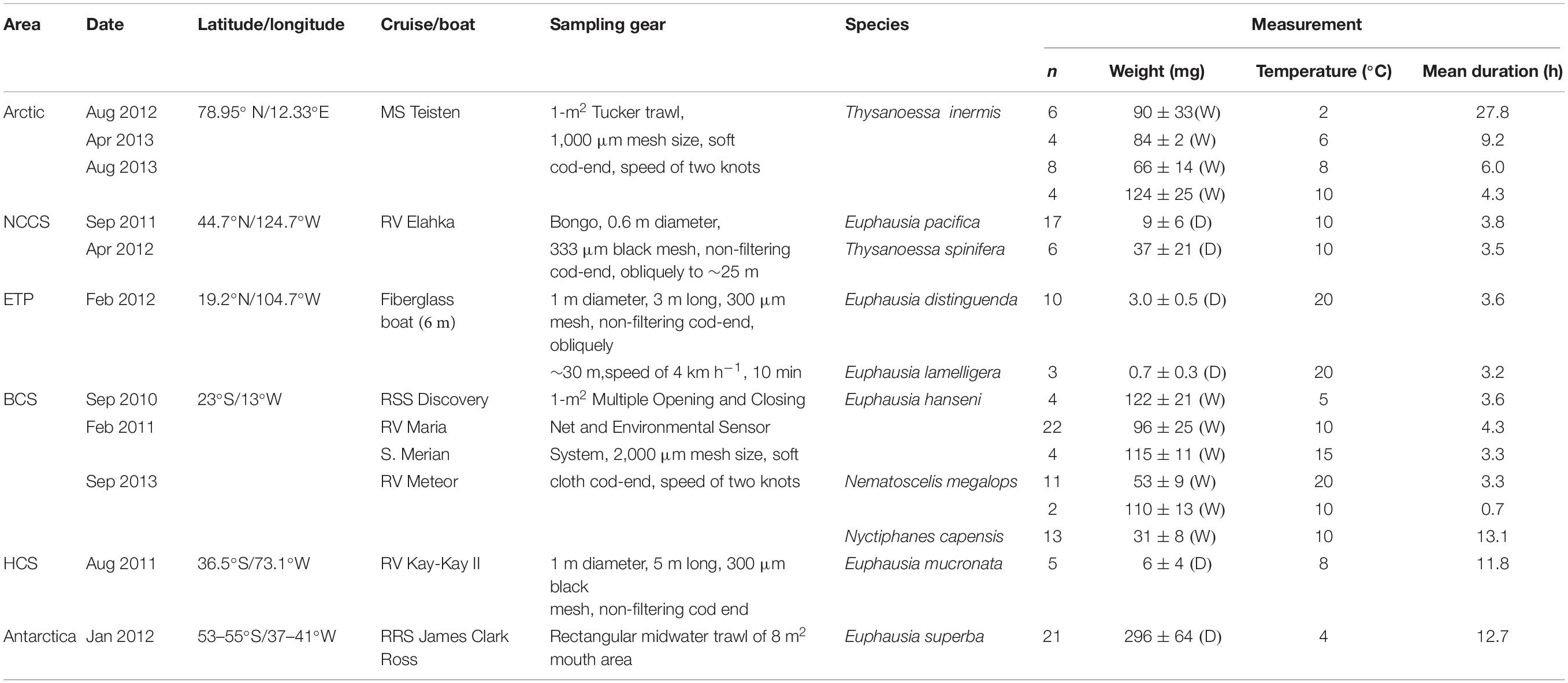
Table 1. From North to South: Sampling areas (latitude/longitude), species names, number of individuals analyzed (n), specimens mean weight (±standard deviation; W, wet weight; D, dry weight) and respiration measurement information in the Benguela, Northern California, and Humboldt Current systems (BCS, CCS, and HCS), in the Eastern Tropical Pacific (ETP), in the Arctic (Kongsfjord, Spitsbergen) and in Antarctica (South Georgia).
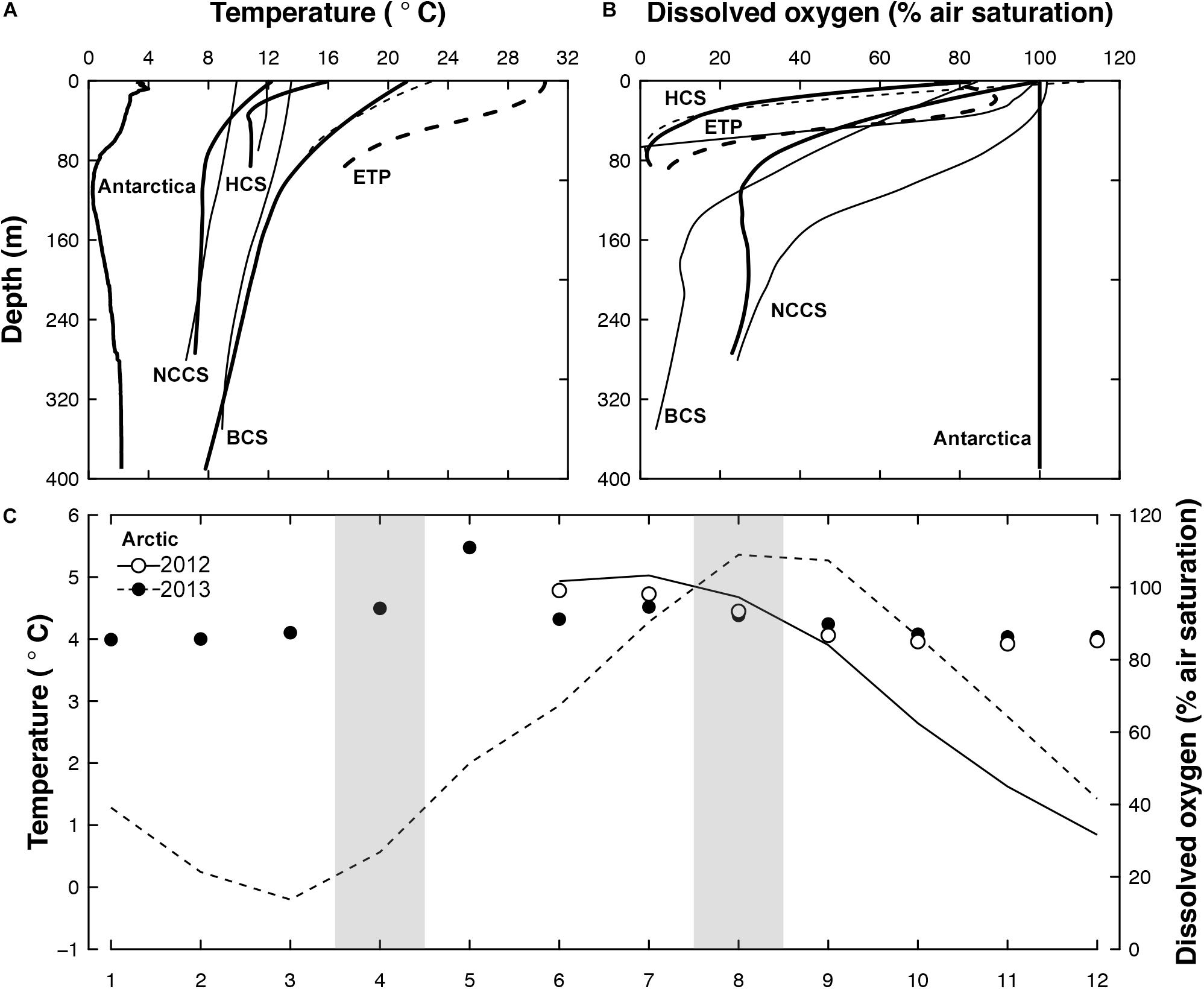
Figure 1. Temperature and oxygen during sampling periods. Depth profiles of (A) mean water temperature and (B) dissolved oxygen in Antarctica, the Humboldt current system [HCS], the Eastern Tropical Pacific [ETP], the Benguela Current System [BCS], and the Northern California Current System [NCCS]. Bold lines represent summer conditions and dashed lines the ETP area. (C) Monthly mean temperature (line) and dissolved oxygen (dots) in the Arctic with sampling periods shaded in gray. Data were compiled from the AWIPEV underwater observatory located at 12 m water depth (Fischer et al., 2018a, b).
All samplings were executed during night time, when euphausiids are more abundant at the surface, to avoid overstressing the experimental animals by reducing catch time. Live adult euphausiids, in healthy condition (showing a lot of movement and with no visible damage), were manually sorted into bins filled with filtered seawater at in situ temperature and acclimated for at least 6 (CCS, HCS, ETP, and Antarctica) or 12 h (BCS and Arctic) prior to starting respirometry procedures to make sure that all animals are in a post-absorptive state.
Respirometry
The measurements were conducted in the dark to mimic the conditions of the time of the day when euphausiids should be in deeper water and hypoxic conditions when hypoxia applied to the area. The same closed configuration system, chamber volume (20 mL; except for Antarctica where chamber volume was 250 mL to account for the larger size of Euphausia superba) and measurement method were used in all areas. The oxygen level within the chamber decreased as the effect of respiration. Measurements were carried out at in situ temperature for the 10 species, and at four different temperatures for Thysanoessa inermis (Arctic; 2, 4, 8, and 10°C) and Euphausia hanseni (BCS; 5, 10, 15, and 20°C) to assess how temperature modulates intraspecific hypoxia tolerance (Table 1). Both species were acclimated at a rate of 1°C h–1 to colder and warmer temperatures for at least 12 h after completion of the 12 h post-capture acclimation. The thermal ramp steepness and amplitude took into consideration the vertical migration temperature gradient that E. hanseni experience during DVM (Werner and Buchholz, 2013) and the Arrhenius breakpoint temperature (12°C) of T. inermis (Huenerlage and Buchholz, 2015; Huenerlage et al., 2016).
OXY-4 or -10 channel PreSens Oxygen Measurement system (Regensburg, Germany) was used with dipping probes DP-PSt3 or planar oxygen-sensitive foils PSt3 integrated in the chambers. Probes and foils were calibrated at in situ temperature prior to measurements at 0% air saturation with sodium sulfite (Na2SO3; 1 g in 100 mL water) and at 100% air saturation with air-saturated water (10 min after air injection in stirred water for 20 min). The system was equipped with four (OXY-4) or ten (OXY-10) chambers including respectively one or two blanks (for seawater bacterial oxygen demand). All chambers were filled with filtered local seawater at 100% air saturation and the oxygen concentration in each chamber was measured every 15 or 30 s. The first 30 min of each measurement were discarded to allow acclimation to chamber. Movements of the pleopods and/or heartbeats of the animals were visually monitored to make sure that they were alive during the entire duration of the measurement. Wet or dry (48 h at 50°C) weight of the preserved animal was measured after completion of the respiration measurement (information provided in Table 1). All respiration rates were reported as mL O2 h–1g wet weight–1. For some species, only the dry weight was available and it was converted to wet weight using the euphausiids conversion equation of Kiørboe (2013) to allow comparison among the 10 species. The programming environment for data analyses and graphics R (R Core Team1) was used to calculate the RI [see section “Regulation Index (RI)”] [script provided as Supplementary Material (see Supplementary Data Sheet 1)].
Regulation Index (RI)
Mueller and Seymour (2011) were the first to propose the use of the RI to assess regulation ability of aquatic organisms that do not present a clear critical oxygen partial pressure (Pcrit) in their respiration pattern. The authors advised to fit a curve (straight line, quadratic or one-phase association) with the highest r2 to the respiration rate data for each individual plotted against the whole oxygen concentration range measured within the respiration chamber (ideally from 100 to 0% air saturation). RI corresponded to the proportion of the area bounded by a linear regression that represented how respiration rates would decline if the animals showed complete oxyconformity (perfect oxyconformity; RI = 0) and a horizontal line at maximum oxygen consumption (perfect regulation; RI = 1). The perfect oxyconformity linear regression assumes zero respiration rate at 0% air saturation (Figure 2A).
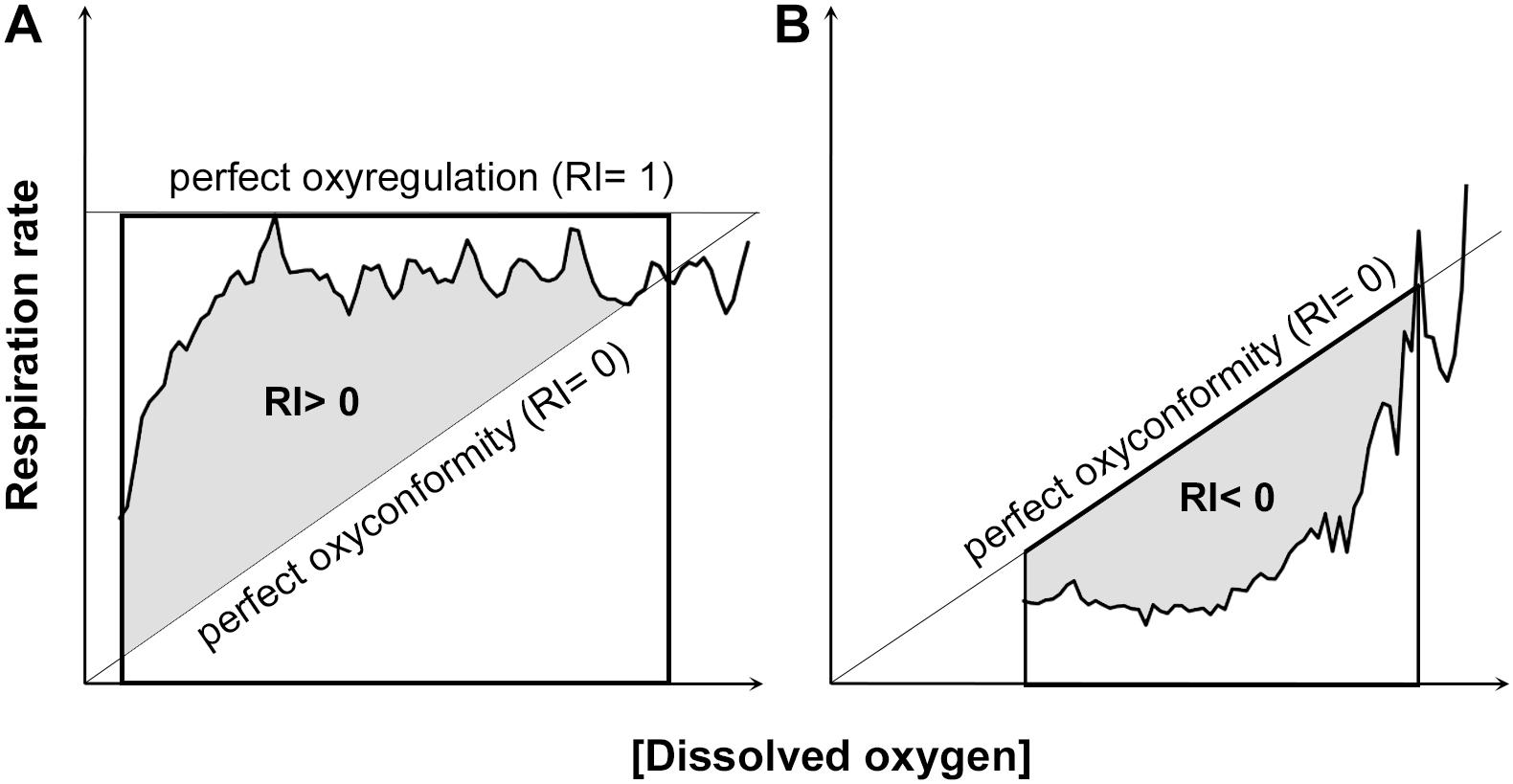
Figure 2. Examples of regulation index (RI) calculations. As shown by Mueller and Seymour (2011), the area under the curve and above the linear regression of perfect oxyconformity was used to calculate a positive RI (A). In contrast, a negative RI was calculated as the area bounded by the area above the curve and the linear regression of perfect oxyconformity (B).
The present work used 141 respiration data sets [available in Supplementary Material (see Supplementary Data Sheet 1)], in which the experimental oxygen concentration dropped to ≤50% of the respective experiments’ start concentration. In order to reduce user interpretation in calculating RI by mean of the best fitted curve, no parametric model was fitted to the original data sets. The area under curve was computed using R package “MESS” (Ekstrøm, 2018) with the natural spline interpolation (loess) for dissolved oxygen concentration as x-values and respiration rates as y-values. The same procedure was conducted with the linear regression that represents perfect oxyconformity and perfect oxyregulation. When the natural spline interpolation of the data was below the linear regression of perfect oxyconformity, RI became negative and was calculated from the area bounded by the horizontal line at y = 0 and the linear regression that represented perfect oxyconformity (Figure 2B). A negative RI value can thus be interpreted as hypoxia-sensitivity (Alexander and McMahon, 2004), but could be an indication of metabolic suppression, as respiration rates are significantly reduced. Respiration strategies using median and quartile values were defined as: low degree of oxyregulation (0.25 < RI median < 0.5); high degree of oxyregulation (0.5 < RI median < 1; Q1 > 0.25 or Q3 > 0.75); oxyconformity (RI median≈0; Q1 < 0 and Q3 > 0) and metabolic suppression (RI median, Q1 and Q3 < 0).
Statistical Analysis
All statistics and figures were done with R (R Core Team, 2020). For interspecific (in situ temperature) and intraspecific (among temperature for E. hanseni and T. inermis) hypoxia tolerance comparison, the non-parametric Kruskal–Wallis test was conducted (normality and variance homogeneity were not met). Significant level of all comparisons was fixed at 95% (p < 0.05). For post hoc comparison a multiple comparison test from the package “pgirmess” (Giraudoux, 2018) was applied.
Results
The overall view of the euphausiids’ respiration rates over decreasing dissolved oxygen concentration at in situ temperature shows different magnitude and patterns (Figure 3). Comparing this magnitude by area, the highest respiration rates were observed in Euphausia pacifica (NCCS), Euphausia lamelligera (ETP), and Nematoscelis megalops (BCS). Different magnitude and patterns were also seen intraspecifically when T. inermis and E. hanseni were acclimated at lower or higher temperatures (Figures 4, 5). The respiration rates of T. inermis increased at 8 and 10°C (Figures 4C,D) in comparison to 2 and 6°C (Figures 4A,B) during the whole oxygen range measured. For E. hanseni, respiration rates were similar at all temperatures in the high-oxygen levels between 80 and 100% air saturation (Figure 5).
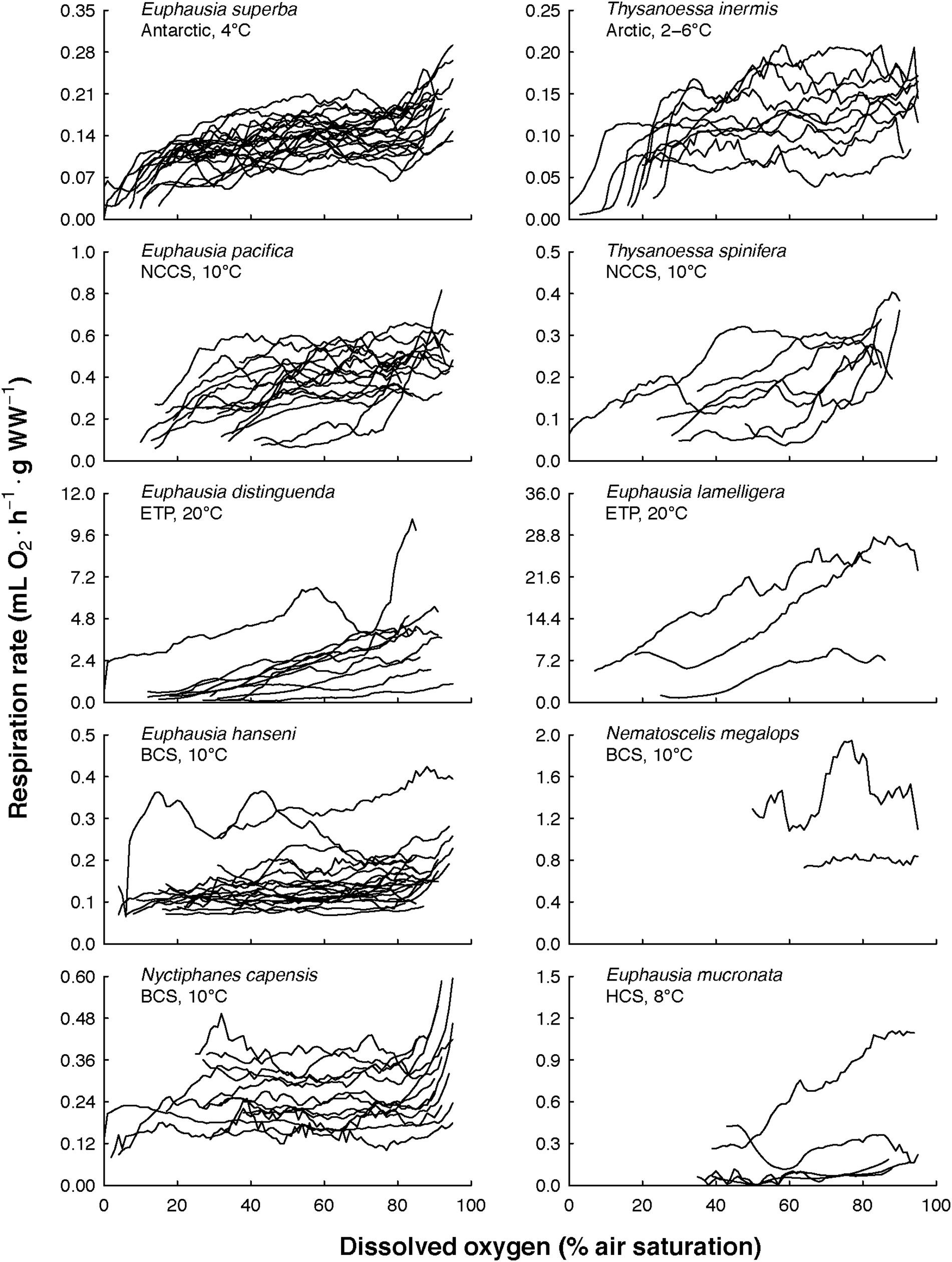
Figure 3. Euphausiids’ respiration rates over decreasing dissolved oxygen concentration at in situ temperature. The 10 euphausiids species were from both Polar regions, three major Eastern Boundary Upwelling Systems (NCCS, Northern California Current System; BCS, Benguela Current System; HCS, Humboldt Current System), and one tropical region (ETP, Eastern Tropical Pacific).
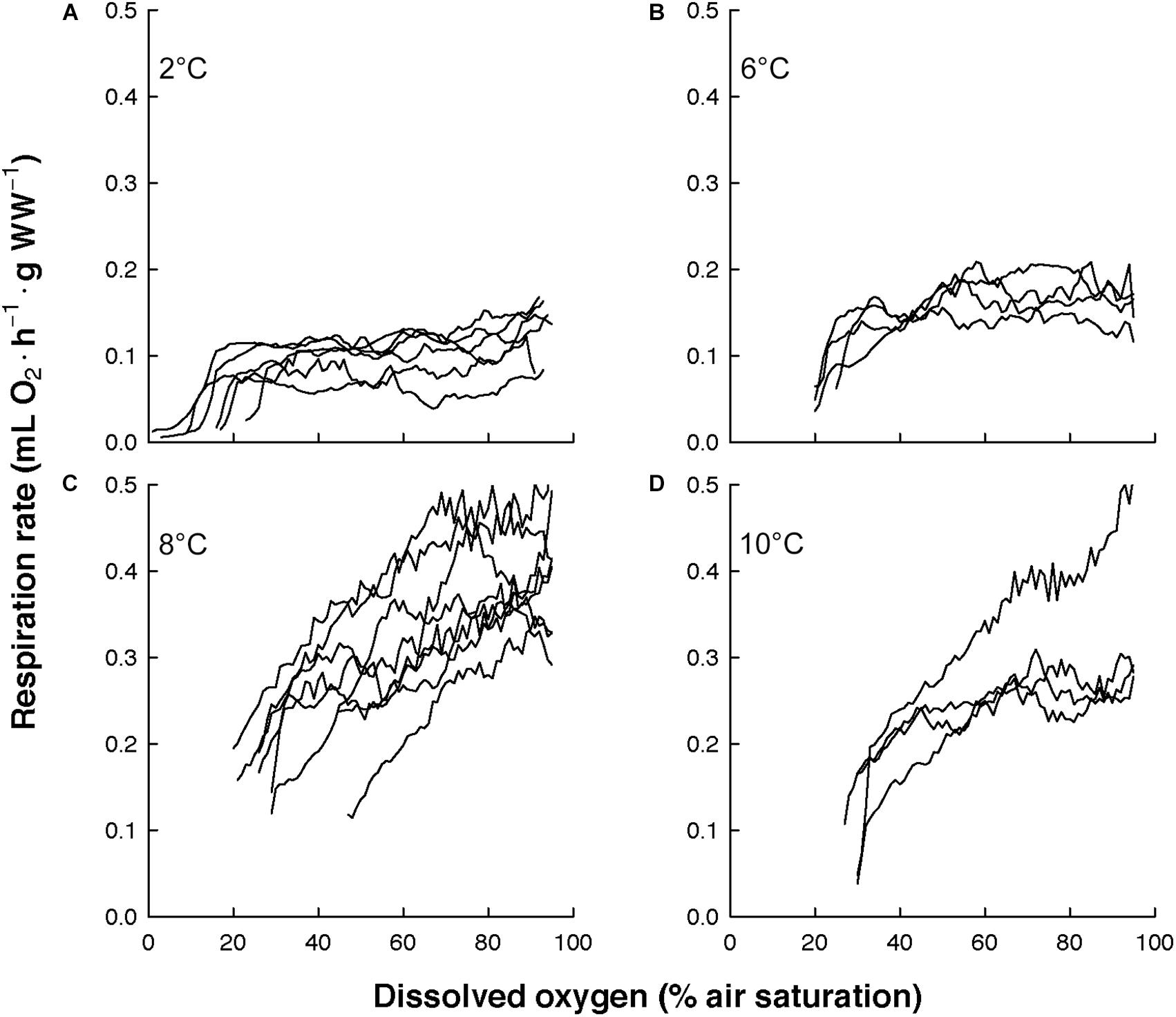
Figure 4. Respiration rates of Thysanoessa inermis over decreasing dissolved oxygen concentration at four temperatures. (A) at 2°C (n = 6), (B) at 6°C (n = 4), (C) at 8°C (n = 8), and (D) at 10°C (n = 4).
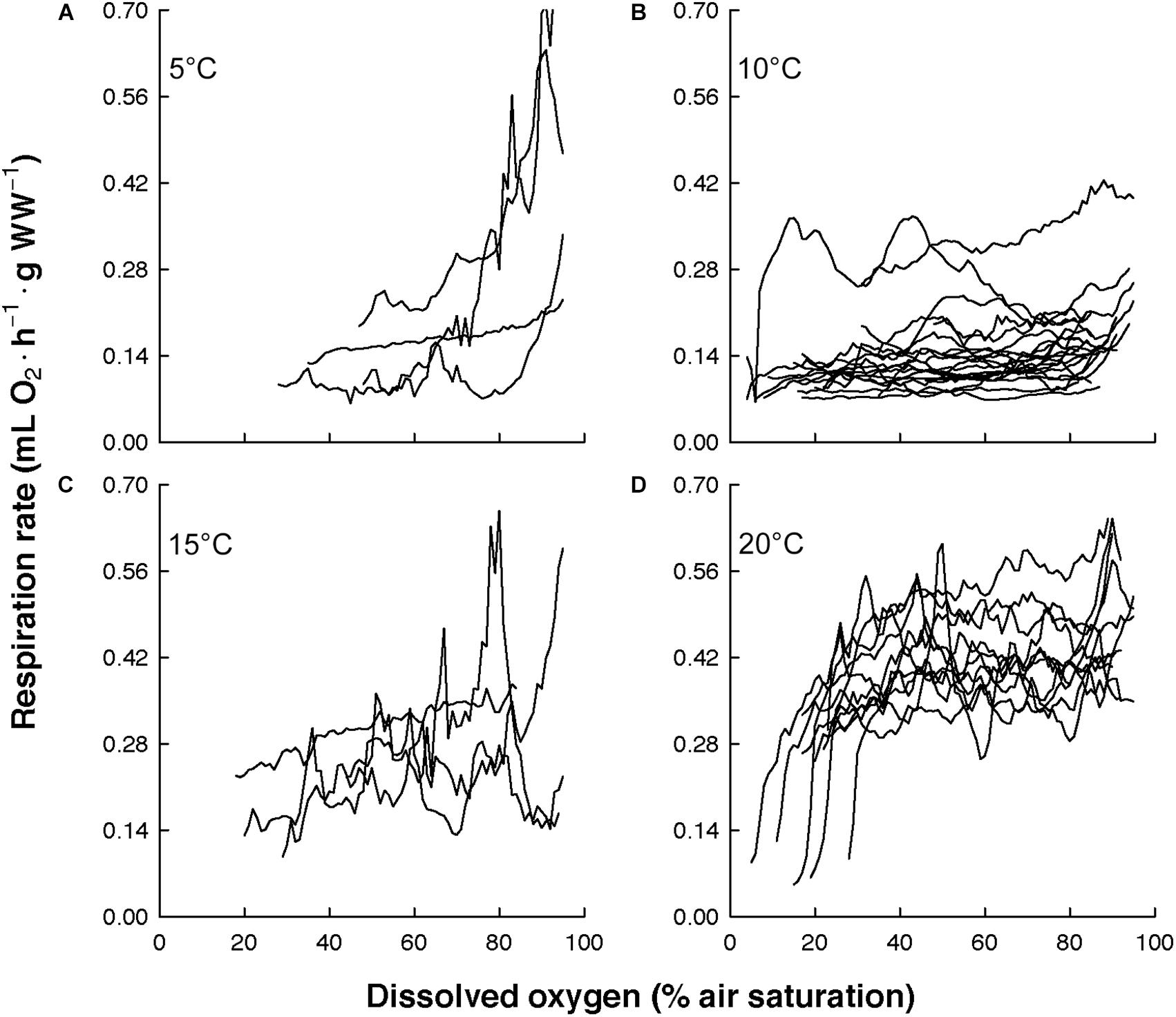
Figure 5. Respiration rates of Euphausia hanseni over decreasing dissolved oxygen concentration at four temperatures. (A) at 5°C (n = 4), (B) at 10°C (n = 22), (C) at 15°C (n = 4), and (D) at 20°C (n = 11).
The RI of Euphausia superba (Antarctica), Thysanoessa inermis (Arctic), Euphausia hanseni (BCS), and Nyctiphanes capensis (BCS) were significantly higher than the RI of the tropical and temperate species Euphausia distinguenda (ETP) and Euphausia mucronata (HCS), respectively (Figure 6A, χ2 = 56.05, p < 0.000, Table 2). Median RI values ≥0.5 of the polar (E. superba, T. inermis), temperate (E. pacifica), and sub-tropical (E. hanseni, N. megalops, and N. capensis) species indicated a high degree of oxyregulation, whereas the neritic temperate (T. spinifera) and tropical (E. lamelligera) species showed a low regulation ability as RI values fluctuated between −0.25 and 0.25 (Figure 6A and Table 2). Quartiles values below and above 0 of T. spinifera and E. lamelligera indicate almost perfect oxyconformity of these species. The oceanic tropical species E. distinguenda and the Humboldt endemic species E. mucronata were qualified as metabolic suppressors with RI median and quartile values well below 0 (Figure 6A and Table 2).
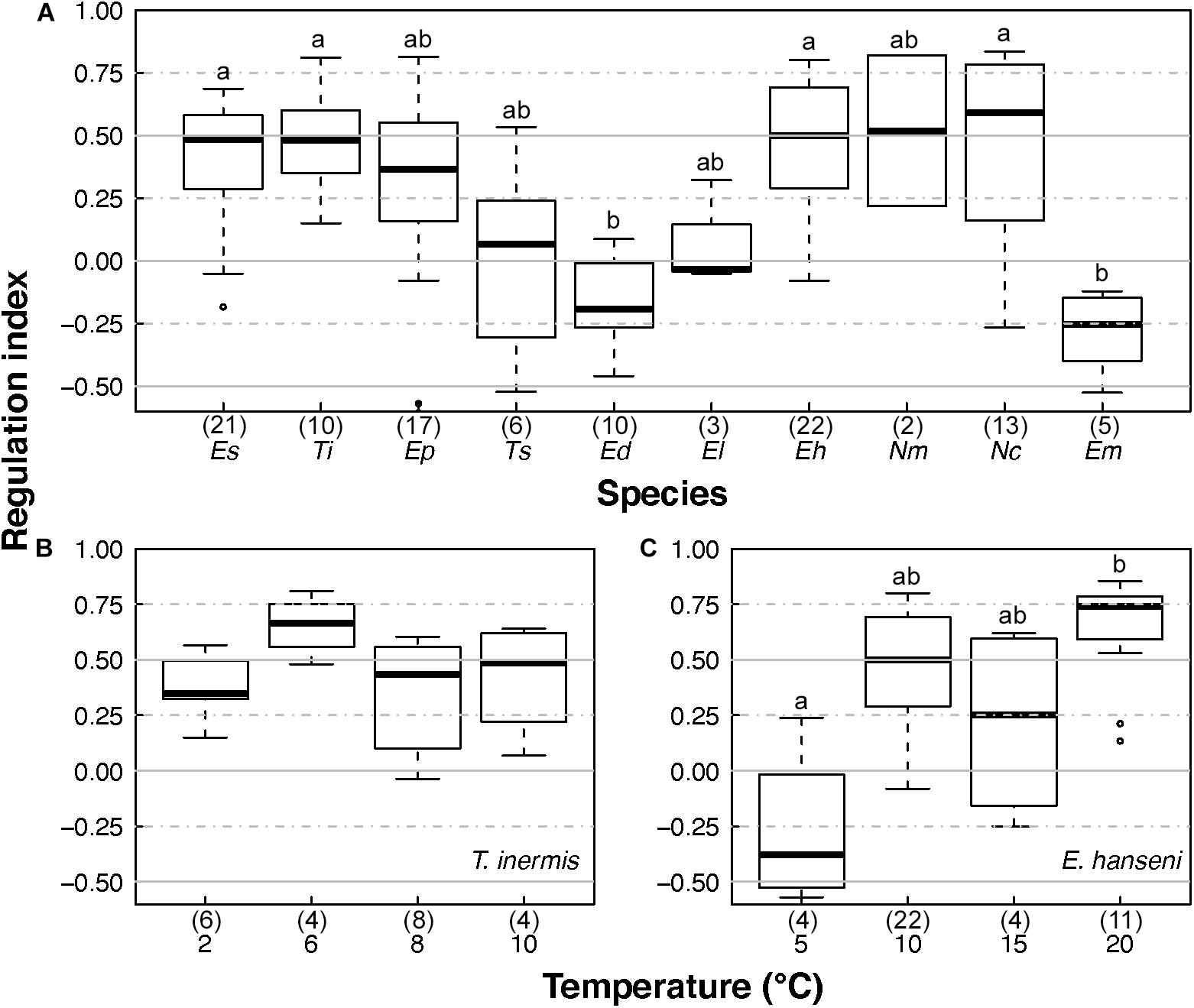
Figure 6. Euphausiids’ regulation indices at in situ temperature and after temperature acclimation. (A) Regulation indices of the 10 euphausiid species investigated at in situ temperature, (B) regulation indices of T. inermis acclimated at 2, 6, 8, and 10°C, and (C) regulation indices of E. hanseni acclimated at 5, 10, 15, and 20°C. Es, E. superba; Ti, T. inermis; Ep, E. pacifica; Ts, T. spinifera; Ed, E. distinguenda; El, E. lamelligera; Eh, E. hanseni; Nm, N. megalops; Nc, N. capensis; Em, E. mucronata. Numbers in parentheses give the numbers of samples analyzed. Horizontal bars in the box plots indicate the median. The upper and lower edges of the rectangles show the first and third quartiles, respectively. Vertical error bars extend to the lowest and highest values in a 1.5-fold inter-quartile range (R Core Team, 2020). Lower case letters indicate significant differences.
Regulation index did not change significantly with temperature for T. inermis (Figure 6B), but it did in E. hanseni from high oxyregulation to metabolic suppression, when acclimation temperature was decreased to 5°C (compared to 20°C; χ2 = 14.53, p = 0.002; Figure 6C).
Discussion
Euphausiids and other zooplankton taxa perform DVM to feed on the phytoplankton-rich upper water layers during night time and to reduce mortality from visual predation during the day. These benefits are counteracted by higher energy demands due to increased swimming speeds and higher water temperatures in upper water layers and reduced growth and reproduction rates in deeper, cold water layers. Thus, animals performing DVM have to compensate with increased energy expenditures. Furthermore, they must have evolved physiological and behavioral adaptations to the strong gradients of oxygen and temperature in the water column. As some species suppress their metabolism (Seibel et al., 2016), determination of metabolic rates of diel vertical migrators is crucial to assess the role and quantify the contribution of these animals to the downward transport of carbon and thus carbon fluxes in the oceans. The environmental conditions prevailing in the different ecosystems in terms of oxygen availability and vertical temperature profiles seem to have caused specific physiological adaptations in euphausiids – mostly irrespective of the actual oxygen and temperature level and the time spent in the OMZs. Species which come across the shallowest severe hypoxia levels during their DVM show metabolic suppression [in the Humboldt Current System (HCS) and ETP]. In contrast, the three species from the Benguela Current System (BCS), characterized by a deeper OMZ, maintain constant oxygen uptakes irrespective of the ambient oxygen levels. These differences may indicate that a steep decline in oxygen levels constitutes a physiological threshold at which euphausiids must significantly shut down their metabolic functions (Seibel et al., 2016).
Shallow OMZs
The hypoxia tolerance of the euphausiid species adapted to the OMZs of the HCS and the ETP was assessed using the RI. In the literature, typical low Pcrit between 0.6 and 1.7 kPa at 13°C and 23°C (corresponding to 3% and 8% air saturation) were obtained by Teal and Carey (1967) and Kiko et al. (2016), who were working on Euphausia mucronata from the HCS. This euphausiid species performs extensive DVM down to 250 m into the OMZ in all seasons (Escribano et al., 2000; Antezana, 2002b). However, highest abundances of this species occur in areas where the upper boundary of the OMZ is deeper (Escribano et al., 2000). During the warm season at 12°C and 13°C, E. mucronata maintains the same rate regardless of whether exposed to surface pO2 (70% air saturation or 17 kPa), or to pO2 typical for OMZ layers (20% air saturation or 4 kPa; Antezana, 2002a; Donoso and Escribano, 2014). The temperature used by the authors cited above represents the warmer range in subsurface water of the area (50–200 m), while the present study was simulating the coldest temperature that can be encountered in the same water depth or at surface during “normal” or cold (La Niña) years between 40°S and 17°S off Chile (Strub et al., 1998). As seen from the changes in RI following the decreasing temperature in Euphausia hanseni from the BCS, the OMZ-adapted species of the genus Euphausia may regulate their metabolic rates when exposed to warmer surface temperature and tend to conform or suppress their metabolism when exposed to the colder thermal limit of their deeper habitat. This may explain why no oxyregulation pattern at all was observed at 8°C in E. mucronata, despite the long duration of the measurement.
The tendency to conform or suppress the metabolism at colder temperature may be also true for Euphausia distinguenda from the ETP (corresponding to sub-surface temperature) as shown here with our measurement at 20°C. From field samples collected at different depths above and into the OMZ of the ETP off Mexico, Herrera et al. (2019) observed the highest specific Electron Transfer System (ETS) activity between 3.20 and 3.93 mL O2 L–1 (48 to 58% air saturation) at 25°C, meaning that the species was still relying on aerobic processes half-way within the oxycline. This ETP species is also reported in the OMZ of the HCS (Antezana, 2009). Both E. distinguenda and E. mucronata possess larger gills relative to their body size (Antezana, 2002a), increasing contact surface for O2 diffusion from the hypoxic environment. Antezana (2009) also observed that both were among the last OMZ species to begin their ascent to the surface at dusk in the HCS, thus extending the deep hypoxic residence time to a maximum. Habitat segregation was suggested to explain this behavior, which consists in avoiding spatial and temporal co-occurrence with other species within the same area. This finding was based on body and gills size analysis, feeding appendages, and HCS food resources. Euphausia lamelligera, the other ETP species also endemic to the OMZ, dominates the neritic zone while E. distinguenda distributes more in oceanic waters (Brinton, 1962, 1979; Färber-Lorda et al., 1994, 2004, 2010). Because of its neritic preference, E. lamelligera does not migrate as much as E. distinguenda, explaining probably why this species is almost a perfect oxyconformer rather than a metabolic suppressor. Both species are thus highly hypoxia tolerant, reflected mainly by their low RI. As high temperature pushes physiological limits, a small sub-mesoscale oxygen variability in the ETP of ≤1% could affect their vertical and horizontal distribution (Wishner et al., 2018). Accordingly, even small changes in oxygen availability may exert strong pressure on these animals, leading to unexpected changes in ecosystem structure and functioning in the near future. Species of the ETP are adapted to low oxygen and high temperature, but as they live at the edge of their maximum thermal limit, further warming could have negative impact on the fitness of both species as their higher brood size depends on the coastal upwelling dynamics between January and June (Ambriz-Arreola et al., 2015, 2018). A negative RI was initially not proposed by Mueller and Seymour (2011) when developing the RI as a new method to assess hypoxia tolerance of aquatic ectotherms. However, as shown for weak oxyregulating (or perfect oxyconformers) and metabolic suppressing species presented in this study, a negative RI is relevant and thus presents a further development for the use of this index. The corroboration of the presence of metabolism suppression associated with a negative RI remains to be shown looking at physiological metabolic markers (e.g., enzymatic activity, ATP production, anaerobic end-products). This pattern was previously described as hypoxia-sensitivity by Alexander and McMahon (2004).
Deep OMZ
In the BCS, E. hanseni and Nematoscelis megalops dominate the shelf break surroundings, i.e., partly sharing one habitat in this upwelling region (Barange and Stuart, 1991; Barange et al., 1991). The species E. hanseni performs extensive DVM from 0 to 200 and even 1,000 m water depth (Barange, 1990; Barange and Stuart, 1991; Barange and Pillar, 1992; Werner and Buchholz, 2013), while N. megalops is characterized as a weak migrator (Werner and Buchholz, 2013). In contrast to E. hanseni, N. megalops has a broader distribution and can be found at both sides of the equator: in the mid-latitude zones of the subtropical-temperate North Atlantic (10–60°N), in the warm-temperate belts of the South Atlantic, the Indian Ocean and the South Pacific (35–50°S), in the Mediterranean Sea (e.g., Gopalakrishnan, 1974), in subarctic regions (Zhukova et al., 2009), even up to 79°N in the high Arctic Kongsfjord (Buchholz et al., 2009; Huenerlage and Buchholz, 2015). Morphologically and ecologically, N. megalops is very similar to Nematoscelis difficilis from the ETP and California Current System (Karedin, 1971; Gopalakrishnan, 1974, 1975). Those species are observed within the OMZ, but in its upper boundary (Tremblay et al., 2010; Werner and Buchholz, 2013), probably taking advantage of the accumulation of organisms to actively feed. The third species Nyctiphanes capensis shows extraordinarily high abundances over the Namibian shelf in water <200 m depth (Barange and Stuart, 1991; Barange and Pillar, 1992).
E. hanseni and N. capensis have one of the highest RI values of all species analyzed meaning that they cover their energy requirements at low oxygen levels in the coldest temperature experienced in their habitat. RI values were enhanced in E. hanseni acclimated at 20°C compared to 10°C, which is similar to results of Teal and Carey (1967) and Kiko et al. (2016) at sub-surface temperature conditions with the species E. mucronata from the HCS. However, the respiration rate of N. megalops investigated here was 10-fold higher than of E. hanseni and N. capensis, which is not consistent with what was reported before by Werner et al. (2012). The number of individuals here reported is small, and the specimens were probably stressed as the duration of the measurement was short (less than 1 h) compared to other nine species analyzed. Despite this fast decrease in oxygen, it is possible to say that N. megalops was regulating its respiration rate. This ability may explain its persistence in the OMZ 24 h a day. Consequently, N. megalops must have evolved efficient adaptations to deal with low oxygen levels, such as, e.g., a high respiratory surface (gills) and/or a general low oxygen demand due to its smaller vertical migration movement. Thus, the ability of an animal to either maintain a constant oxygen uptake irrespective of the ambient oxygen levels or decrease its oxygen consumption rates when ambient oxygen levels decrease seems not to be influenced by its DVM behavior in the first place.
Seasonal OMZ
Off Oregon (United States), in the Northern California Current System (NCCS), two euphausiid species dominate the macrozooplankton community: the oceanic Euphausia pacifica (Brinton, 1962) with DVM between the surface and depths of at least 250 m (Brinton, 1967) and the neritic cold upwelling-associated Thysanoessa spinifera (Brinton, 1962; Smith and Adams, 1988; Lavaniegos and Ambriz-Arreola, 2012). Because of its neritic lifestyle, T. spinifera does not migrate as deep as E. pacifica, but, instead, remain within the upper 100 m during day and night and swarm in summer at surface for reproduction (Brinton, 1962; Smith and Adams, 1988). This species is also known for its narrow plasticity when facing changes in the physical oceanographic conditions (Brinton, 1979). Indeed, T. spinifera is strongly influenced by the North Pacific Gyre Oscillation (Di Lorenzo et al., 2008; Sydeman et al., 2013). This oscillation is connected with the winds and upwelling responses (Chenillat et al., 2012) and corroborates the upwelling preference of this species. Important changes in both species’ distribution occurred during the El Niño event of 1992–1993, after which biomass of T. spinifera fell by more than 70% off Oregon and British-Columbia (Tanasichuk, 1999). In the southern part of the CCS (at approximately 30°N; Off Baja California), E. pacifica took some time to recover after the El Niño event of 1997–1998, but was abundant again during summers of 2000, 2002, and 2005. These high abundances were linked to La Niña in 2000, a sub-Arctic water intrusion in 2002 and to high upwelling conditions in 2005 (Lavaniegos and Ambriz-Arreola, 2012). It is clear that El Niño brings low upwelling conditions (low food availability) and warmer deoxygenated water, which are not optimal for the temperate species of the NCCS.
A different pattern within the respiratory response to declining pO2 was observed between T. spinifera and E. pacifica. The neritic lifestyle, short vertical migration distance, and strong association with upwelling areas (high nutrients, cold temperature, and lower oxygen concentration) match the comparatively low metabolic rate of T. spinifera compared to oceanic E. pacifica. The strong association of T. spinifera with upwelling conditions likely signifies an oxyconformity strategy to tolerate the typical low oxygen concentration of upwelled water. So far, no acoustic or direct observations of hypoxia and warming effects on T. spinifera have been reported. However, massive stranding events in several bays on the US West Coast over an area of approximately 400 km between Oregon and California were observed in summer of 2013 and related to the strongly hypoxic conditions prevailing regionally (Leising et al., 2014). This hypoxic zone was extending into the upper 50–100 m of the water column. A similar situation was observed in the Gulf of California (Mexico) with the subtropical species N. difficilis (López-Cortés et al., 2006), the counterpart in the Pacific of N. megalops. The authors proposed that high unusual upwelling conditions promoted a phytoplankton bloom, which indirectly depleted the oxygen concentration with the sinking of organic matter. This would have forced the mesopelagic N. difficilis to migrate upward toward more oxygenated waters and then to be washed out by the surface currents. N. difficilis was shown to be relatively tolerant to hypoxic conditions, but less than the tropical species Euphausia eximia (Tremblay et al., 2010; Seibel et al., 2016) and Nemastocelis gracilis (Seibel et al., 2016).
High tolerance to hypoxia was assumed in the past for E. pacifica because of its low critical oxygen partial pressure (Pcrit = 18 mm Hg, 2 kPa or 11% air saturation at 10°C), lower than what the species experiences in situ at 350 m depth in its habitat (off South California; Childress, 1975). In fjords and bays their downward migration is often reduced (Bollens et al., 1992), sometimes limited by seasonal hypoxic or anoxic conditions in bottom water layers (Kunze et al., 2006). In these environments, Pcrit values of E. pacifica were higher (Pcrit = 4 kPa or 20% air saturation at 10°C), showing less hypoxia tolerance (Ikeda, 1977). The RI of E. pacifica indicated that this species is not an outstanding oxyregulator as BCS and polar species. The high standard deviation of RI may speak for a lack of a consistent strategy when dissolved oxygen concentration decrease at in situ temperature.
Alternation between El Niño and La Niña events maintains the abundance of krill across time in the NCCS, but it is clear that if strong El Niño event like the one of 1997–1998 last longer or occurs more often, both T. spinifera and E. pacifica stocks would probably disappear from the NCCS and continue their life cycle at higher latitudes in the Gulf of Alaska, where they are not so affected by the El Niño event. This would have strong consequences for the higher trophic levels of the NCCS.
Cold Regions and OMZ-Free
The polar species Euphausia superba and Thysanoessa inermis exhibit also one of the highest RI among the 10 species assessed. The Antarctic krill E. superba is a central constituent of Antarctic food webs and forms large biomasses in the Southern Ocean (Atkinson et al., 2004; Murphy et al., 2007). Cumulative impacts of sea ice decline and ocean warming have negatively modified the abundance, distribution and life cycle of this species (Flores et al., 2012). The species T. inermis is restricted to the North Atlantic, North Pacific and the shelf region around Spitsbergen, continuously advected to the Arctic by the ocean currents from the Barents Sea where they have their major spawning ground. Both polar species are known as pronounced vertical migrators with some flexibility depending on food availability and predation risk (Kaartvedt et al., 1996; Cresswell et al., 2009).
As oxygen levels in Polar regions are relatively high, it appears that there is no compelling need to evolve adaptations to low oxygen levels. However, both species are well known for their dense swarming behavior and may experience reduced oxygen levels in these dense aggregations (Brierley and Cox, 2010). According to Brierley and Cox (2010), the oxygen concentration in a median packed E. superba swarm (40 m diameter, 111 ind. m–3) can fall from 6.8 to 5.8 mL O2 L–1 (76 to 65% air saturation or 16 to 14 kPa in South Georgia) after approximately 3 min spent in the middle. Swarm density can reach 25,000 ind m–3 (Hamner and Hamner, 2000) or spread over hundreds km–2 (Nowacek et al., 2011), so it can be easily envisaged that the reduction in oxygen availability in the middle of these biological features may be dramatically higher. This is probably the reason why E. superba and T. inermis deploy unexpected high hypoxia tolerance at in situ temperatures. As temperature generates higher energy demands in T. inermis (>three-fold), temperature rise in the North-Arctic of 3°C above the current summer conditions could lead to increased competition with other warmer adapted species, like Meganyctiphanes norvegica and N. megalops (Huenerlage and Buchholz, 2015). So far, this Arcto-boreal species seems to benefit from the current higher water temperatures in the Arctic as it seems to reproduce successfully in the Kongsfjorden (Buchholz et al., 2012).
RI or Others?
Even though temperature increases the metabolic activity, hence the energy demands of an animal, the present study suggests that the ability to cope with low oxygen levels is not always worse at higher temperatures for hypoxia-adapted species. A possible explanation could be that despite higher energy expenditure other processes such as diffusion rates are also enhanced, providing sufficient oxygen for an animal. As a consequence, we suggest that it is of crucial importance to measure respiration rates at in situ temperatures when comparing the hypoxia tolerances of various species within and between ecosystems.
Furthermore, the RI value, as a proxy for the capability of an animal to withstand low oxygen levels, seems to be indicative for the oxygen tolerance for its own. Low RI values were possible to determine for species such as E. mucronata, E. distinguenda, and E. lamelligera as they were showing oxyconformity or metabolic suppression patterns. In contrast, E. hanseni and N. capensis occurring in the BCS show high RI values. However, all species are known to withstand comparably low oxygen levels. This highlights the need to analyze the RI, additionally to Pcrit, to get a wider understanding of the species-specific adaptation strategies. Standardization to calculate RI is important as its determination depend on the model used (Cobbs and Alexander, 2018). In the present work, in order to reduce user interpretation in calculating RI by mean of the best fitted curve, we used the area under curve of the original data sets.
The analysis of the ETS activity and the contribution of the alternative oxidase (AOX) pathway are parameters that could be implemented to understand other metabolic adaptations related to vertical oxygen and temperature gradients. High specific ETS activities were observed in zooplankton collected in the Equatorial-Subtropical Atlantic mesopelagic zone (Hernández-León et al., 2019), also coinciding with a previous observation in the Eastern Equatorial Pacific (Herrera et al., 2019). The authors discussed this observation as an adaptation of migrant zooplankton to endure the adverse conditions of low temperature and low oxygen in deep waters. The AOX pathway is also a promising avenue to explore in response to temperature and oxygen vertical gradients as it has been identified and expressed in the copepod Tigriopus californicus in response to cold and heat stress compared to normal rearing temperature (Tward et al., 2019). This pathway could be an important player to support partial electron transport in order to stabilize mitochondrial membrane potential during metabolic suppression of OMZ-adapted species when residing for some hours in hypoxic conditions, as seen in the gills of freshwater bivalves adapted to hypoxia (Yusseppone et al., 2018).
It is known that species or populations of species confined to one hemisphere or a particular part of the ocean (neritic vs. oceanic) become often specialists (Jones and Cheung, 2017). They are in most cases neither widely distributed nor physiologically versatile, and can be predicted to especially suffer from the effects of ocean warming and OMZs’ expansion. This may be also true for euphausiid species, but this study clearly illustrates that most euphausiids, using different strategies, cope with a range of different oxygen and temperature levels – showing high physiological plasticity – and hence, explaining why this successful taxon is predominate in all the world’s oceans. However, species from the NCCS, ETP and the Arctic may be more vulnerable to future environmental conditions with increased water temperatures and decreased oxygen levels.
Data Availability Statement
All datasets generated for this study are included in the article/Supplementary Material.
Author Contributions
All authors participated in the concept of the study. NT conducted the experiments, analyzed the results, draw the figures, wrote, and revised the manuscript. KH conducted the experiments and contributed to data analysis, writing and revision of the manuscript. TW conducted the experiments, analyzed the results, and contributed to writing and revision of the manuscript.
Funding
This study was funded by the GENUS project, Bundesministerium für Bildung und Forschung (BMBF, 03F0497F, Germany) and supported by the Alfred-Wegner-Institute for Polar and Marine Research (PACES, WP2T2) as well as the French-German AWIPEV project KOP 124, RIS ID 3451. We acknowledge support by the Open Access Publication Funds of Alfred-Wegener-Institut Helmholtz-Zentrum für Polar- und Meeresforschung.
Conflict of Interest
The authors declare that the research was conducted in the absence of any commercial or financial relationships that could be construed as a potential conflict of interest.
Acknowledgments
We thank the Captains and crew of the RV Meteor, the RV Maria S. Merian, RV Kay-Kay II, RV Elahka, RRS James Clark Ross (JR260, supported by the Ecosystems programme at the British Antarctic Survey, funded by the Natural Environment Research Council), and the RSS Discovery for excellent support on-board. We are further grateful for the professional support by the AWIPEV station leaders, (logistic) engineers, and the captains of the Kings Bay AS workboat MS Teisten, Ny-Alesund, Spitsbergen. We thank the scientists Carmen Franco-Gordo, Israel Ambriz-Arreola, and Eva Kozak from the Centro de Ecología Costera de la Universidad de Guadalajara (San Patricio de Melaque, Mexico), Rubén Escribano, Pamela Hidalgo, Ramiro Riquelme-Bugueño from the Pelagic and Mesozooplankton Laboratory of the Centro de Investigación Oceanográfica del Pacífico Sur-Oriental de la Universidad de Concepción (Concepción, Chile), C. Tracy Shaw, William T. Peterson (deceased 08/2017), Jay Peterson from the Hatfield Marine Science Center of Oregon State University (Newport, United States) and Sophie Fielding and Geraint A. Tarling from the British Antarctic Survey (Cambridge, United Kingdom) for the record of environmental information, and their support in collecting zooplankton samples. We thank the reviewers for their critical comments that improved the quality of the article. This work was presented as “Krill worldwide: A comparison of hypoxia tolerances of euphausiid species from Atlantic, Pacific and Polar regions” in the Third International Symposium on Effects of Climate Change on the World’s Oceans held in Santos City (Brazil) on March 23–27th, 2015 (Werner et al., 2015). Finally, we are deeply grateful to our Ph.D. supervisors, Doris Abele and Friedrich Buchholz, for providing us the opportunity to work in these projects. The idea was developed and partly presented in the doctoral thesis of NT and TW.
Supplementary Material
The Supplementary Material for this article can be found online at: https://www.frontiersin.org/articles/10.3389/fphys.2020.00248/full#supplementary-material
Footnotes
References
Alexander, J. E. Jr., and McMahon, R. F. (2004). Respiratory response to temperature and hypoxia in the zebra mussel Dreissena polymorpha. Comp. Biochem.Physiol. A 137, 425–434. doi: 10.1016/j.cbpb.2003.11.003
Ambriz-Arreola, I., Gómez-Gutiérrez, J., Franco-Gordo, M. C., and Kozak, E. R. (2015). Reproductive biology, embryo and early larval morphology, and development rates of krill (Euphausia lamelligera and Euphausia distinguenda), endemic to the Eastern Tropical Pacific. Sex. Early Dev. Aquat. Org. 1, 143–161. doi: 10.3354/sedao00014
Ambriz-Arreola, I., Gómez-Gutiérrez, J., Franco-Gordo, M. C., Plascencia-Palomera, V., Gasca, R., Kozak, E. R., et al. (2018). Seasonal succession of tropical community structure, abundance, and biomass of five zooplankton taxa in the central Mexican Pacific. Cont. Shelf Res. 168, 54–67. doi: 10.1016/j.csr.2018.08.007
Antezana, T. (2002a). “Adaptive behaviour of Euphausia mucronata in relation to the oxygen minimum layer of the Humboldt Current,” in Oceanography of the eastern Pacific II, ed. J. F. ärber-Lorda (Mexico: Editorial CICESE), 29–40.
Antezana, T. (2002b). “Vertical distribution and diel migration of Euphausia mucronata in the oxygen minimum layer of the Humboldt Current,” in Oceanography of the eastern Pacific II, ed. J. F. ärber-Lorda (Mexico: Editorial CICESE), 29–40.
Antezana, T. (2009). Species-specific patterns of diel migration into the oxygen minimum zone by euphausiids in the humboldt current ecosystem. Progr. Oceanogr. 83, 228–236. doi: 10.1016/j.pocean.2009.07.039
Aoki, S. (2005). Interdecadal water mass changes in the Southern Ocean between 30°E and 160°E. Geophys. Res. Lett. 32:L07607.
Atkinson, A., Siegel, V., Pakhomov, E., and Rothery, P. (2004). Long-term decline in krill stock and increase in salps within the Southern Ocean. Nature 432, 100–103. doi: 10.1038/nature02996
Barange, M. (1990). Vertical migration and habitat partitioning of six euphausiid species in the northern Benguela upwelling system. J. Plank. Res. 12, 1223–1237. doi: 10.1093/plankt/12.6.1223
Barange, M., Gibbons, M. J., and Carola, M. (1991). Diet and feeding of Euphausia hanseni and Nematoscelis megalops (Euphausiacea) in the northern Benguela Current: ecological significance of vertical space partitioning. Mar. Ecol. Progr. Ser. 73, 173–181. doi: 10.3354/meps073173
Barange, M., and Pillar, S. C. (1992). Cross-Shelf Circulation, zonation and maintenance mechanisms of Nyctiphanes capensis and Euphausia hanseni (Euphausiacea) in the Northern Benguela Upwelling System. Cont. Shelf Res. 12, 1027–1042. doi: 10.1016/0278-4343(92)90014-b
Barange, M., and Stuart, V. (1991). Distribution patterns, abundance and population dynamics of the euphausiids Nyctiphanes capensis and Euphausia hanseni in the Northern Benguela Upwelling System. Mar. Biol. 109, 93–101. doi: 10.1007/bf01320235
Bianchi, D., Galbraith, E. D., Carozza, D. A., Mislan, K. A. S., and Stock, C. A. (2013). Intensification of open-ocean oxygen depletion by vertically migrating animals. Nat. Geosci. 6, 545–548. doi: 10.1038/ngeo1837
Bollens, S. M., Frost, B. W., and Lin, T. S. (1992). Recruitment, growth, and diel vertical migration of Euphausia pacifica in a temperate fjord. Mar. Biol. 114, 219–228. doi: 10.1007/bf00349522
Brierley, A. S., and Cox, M. J. (2010). Shapes of krill swarms and fish schools emerge as aggregation members avoid predators and access oxygen. Curr. Biol. 20, 1758–1762. doi: 10.1016/j.cub.2010.08.041
Brinton, E. (1962). The distribution of Pacific euphausiids. Bull. Scripps Inst. Oceanogr. 8, 51–269.
Brinton, E. (1967). Vertical migration and avoidance capability of euphausiids in the California Current. Limnol. Oceanogr. 12, 451–483. doi: 10.4319/lo.1967.12.3.0451
Brinton, E. (1979). Parameters relating to the distributions of planktonic organisms, especially euphausiids in the eastern tropical Pacific. Progr. Oceanogr. 8, 125–168.
Buchholz, F., Buchholz, C., and Weslawski, J. M. (2009). Ten years after: krill as indicator of changes in the macro-zooplankton communities of two Arctic fjords. Polar Biol. 33, 101–113. doi: 10.1007/s00300-009-0688-0
Buchholz, F., Werner, T., and Buchholz, C. (2012). First observation of krill spawning in the high Arctic Kongsfjorden, west Spitsbergen. Polar Biol. 35, 1273–1279. doi: 10.1007/s00300-012-1186-3
Chenillat, F., Rivière, P., Capet, X., Di Lorenzo, E., and Blanke, B. (2012). North Pacific Gyre Oscillation modulates seasonal timing and ecosystem functioning in the California Current upwelling system. Geophys. Res. Lett. 39:L01606.
Childress, J. (1975). The respiratory rates of midwater crustaceans as a function of depth of occurrence and relation to the oxygen minimum layer off southern California. Comp. Biochem. Physiol. A 50, 787–799. doi: 10.1016/0300-9629(75)90146-2
Claireaux, G., and Lagardère, J. P. (1999). Influence of temperature, oxygen and salinity on the metabolism of the European sea bass. J. Sea Res. 42, 157–168. doi: 10.1016/s1385-1101(99)00019-2
Cobbs, G. A., and Alexander, J. E. (2018). Assessment of oxygen consumption in response to progressive hypoxia. PLoS ONE 13:e208836–e208820.
Cresswell, K. A., Tarling, G. A., Thorpe, S. E., Burrows, M. T., Wiedenmann, J., et al. (2009). Diel vertical migration of Antarctic krill (Euphausia superba) is flexible during advection across the Scotia Sea. J. Plank. Res. 31, 1265–1281. doi: 10.1093/plankt/fbp062
Dehairs, F., Goeyens, L., Stroobants, N., Bernard, P., Goyet, C., Poisson, A., et al. (1990). On suspended barite and the oxygen minimum in the Southern Ocean. Global Biogeochem. Cycles 4, 85–102. doi: 10.1029/gb004i001p00085
Di Lorenzo, E., Schneider, N., Cobb, K. M., Franks, P. J. S., Chhak, K., Miller, A. J., et al. (2008). North pacific gyre oscillation links ocean climate and ecosystem change. Geophys. Res. Lett. 35:L08607.
Diaz, R. J., and Rosenberg, R. (2008). Spreading dead zones and consequences for marine ecosystems. Science 321, 926–929. doi: 10.1126/science.1156401
Donoso, K., and Escribano, R. (2014). Mass-specific respiration of mesozooplankton and its role in the maintenance of an oxygen-deficient ecological barrier (BEDOX) in the upwelling zone off Chile upon presence of a shallow oxygen minimum zone. J. Mar. Syst. 129, 166–177. doi: 10.1016/j.jmarsys.2013.05.011
Ekau, W., Auel, H., Hagen, W., Koppelmann, R., Wasmund, N., Bohata, K., et al. (2018). Pelagic key species and mechanisms driving energy flows in the northern Benguela upwelling ecosystem and their feedback into biogeochemical cycles. J. Mar. Syst. 188, 49–62. doi: 10.1016/j.jmarsys.2018.03.001
Ekau, W., Auel, H., Pörtner, H.-O., and Gilbert, D. (2010). Impacts of hypoxia on the structure and processes in pelagic communities (zooplankton, macro-invertebrates and fish). Biogeoscience 7, 1669–1699. doi: 10.5194/bg-7-1669-2010
Ekstrøm, C. T. (2018). MESS: Miscellaneous Esoteric Statistical Scripts. R Package Version 0.5.2. Available online at: https://CRAN.R-project.org/package=MESS (accessed February 19, 2020).
Elder, L. E., and Seibel, B. A. (2015). The thermal stress response to diel vertical migration in the hyperiid amphipod Phronima sedentaria. Comp. Biochem. Physiol. A 187, 20–26. doi: 10.1016/j.cbpa.2015.04.008
Enright, J. T. (1977). Diurnal vertical migration: adaptive significance and timing. Part 1. Selective advantage: a metabolic model. Limnol. Oceanogr 22, 856–872. doi: 10.4319/lo.1977.22.5.0856
Escribano, R., Marín, V., and Irribarren, C. (2000). Distribution of Euphausia mucronata at the upwelling area of Peninsula Mejillones, northern Chile: the influence of the oxygen minimum layer. Sci. Mar. 64, 69–77. doi: 10.3989/scimar.2000.64n169
Färber-Lorda, J., Lavín, M. F., and Guerrero-Ruiz, M. A. (2004). Effects of wind forcing on the trophic conditions, zooplankton biomass and krill biochemical composition in the Gulf of Tehuantepec. Deep Sea Res. Pt. II 51, 601–614. doi: 10.1016/j.dsr2.2004.05.022
Färber-Lorda, J., Lavín, M. F., Zapatero, M. A., and Robles, J. M. (1994). Distribution and abundance of euphausiids in the Gulf of Tehuantepec during wind forcing. Deep-Sea Res. Pt. I 41, 359–367. doi: 10.1016/0967-0637(94)90008-6
Färber-Lorda, J., Trasviña, A., and Cortés-Verdín, P. (2010). Summer distribution of euphausiids in the entrance of the Sea of Cortés in relation to hydrography. Deep-Sea Res. Pt. II 57, 631–641. doi: 10.1016/j.dsr2.2009.10.012
Fiedler, P., and Talley, L. (2006). Hydrography of the eastern tropical Pacific: a review. Progr. Oceanogr. 69, 143–180. doi: 10.1016/j.pocean.2006.03.008
Fischer, P., Schwanitz, M., Brand, M., Posner, U., Brix, H., and Baschek, B. (2018a). Hydrographical Time Series Data of the Littoral Zone of Kongsfjorden, Svalbard 2012. Helgoland: Alfred Wegener Institute - Biological Institute Helgoland, PANGAEA. doi: 10.1594/PANGAEA.896828
Fischer, P., Schwanitz, M., Brand, M., Posner, U., Brix, H., and Baschek, B. (2018b). Hydrographical Time Series Data of the Littoral Zone of Kongsfjorden, Svalbard 2013. Helgoland: Alfred Wegener Institute - Biological Institute Helgoland, PANGAEA doi: 10.1594/PANGAEA.896822
Flores, H., Atkinson, A., Kawaguchi, S., Krafft, B. A., Milinevsky, G., Nicol, S., et al. (2012). Impact of climate change on Antarctic krill. Mar. Ecol. Progr. Ser. 458, 1–19.
Friedrich, J., Janssen, F., Aleynik, D., Bange, H. W., Boltacheva, N., Çagatay, M. N., et al. (2014). Investigating hypoxia in aquatic environments: diverse approaches to addressing a complex phenomenon. Biogeoscience 11, 1215–1259. doi: 10.5194/bg-11-1215-2014
Gilly, W. F., Beman, J. M., Litvin, S. Y., and Robison, B. H. (2013). Oceanographic and biological effects of shoaling of the oxygen minimum zone. Ann. Rev. Mar. Sci. 5, 393–420. doi: 10.1146/annurev-marine-120710-100849
Giraudoux, P. (2018). Pgirmess: Spatial analysis and Data Mining for Field Ecologists. R Package Version 1.6.9. Available online at: https://CRAN.R-project.org/package=pgirmess (accessed February 19, 2020).
Gopalakrishnan, K. (1974). Zoogeography of the genus Nematoscelis (Crustacea: Euphausiacea). Fish. Bull. 72, 1039–1074.
Gopalakrishnan, K. (1975). Biology and taxonomy of the genus Nematoscelis (Crustacea: Euphausiacea). Fish. Bull. 73, 797–814.
Hamner, W., and Hamner, P. (2000). Behavior of Antarctic krill (Euphausia superba): schooling, foraging, and antipredatory behavior. Can. J. Fish. Aqua. Sci. 57, 192–202. doi: 10.1139/f00-195
Hernández-León, S., Calles, S., and Fernánde and de Puelles, M. L. (2019). The estimation of metabolism in the mesopelagic zone: disentangling deep-sea zooplankton respiration. Progr. Oceanogr. 178:102163. doi: 10.1016/j.pocean.2019.102163
Herrera, I., Yebra, L., Antezana, T., Giraldo, A., Färber-Lorda, J., and Hernández-León, S. (2019). Vertical variability of Euphausia distinguenda metabolic rates during diel migration into the oxygen minimum zone of the Eastern Tropical Pacific off Mexico. J. Plank. Res. 41, 165–176. doi: 10.1093/plankt/fbz004
Huenerlage, K., and Buchholz, F. (2015). Thermal limits of krill species from the high-Arctic Kongsfjord (Spitsbergen). Mar. Ecol. Progr. Ser. 535, 89–98. doi: 10.3354/meps11408
Huenerlage, K., Cascella, K., Corre, E., Toomey, L., Lee, C.-Y., Buchholz, F., et al. (2016). Responses of the arcto-boreal krill species Thysanoessa inermis to variations in water temperature: coupling Hsp70 isoform expressions with metabolism. Cell Stress Chaperon. 6, 969–981. doi: 10.1007/s12192-016-0720-6
Ikeda, T. (1977). The effect of laboratory conditions on the extrapolation of experimental measurements to the ecology of marine zooplankton II. Effect of oxygen saturation on the respiration rate. Bull. Plank. Soc. Jap. 24, 19–28.
Ito, T., and Deutsch, C. (2013). Variability of the oxygen minimum zone in the tropical North Pacific during the late twentieth century. Global Biogeochem. Cycles 27, 1119–1128. doi: 10.1002/2013gb004567
Jones, M. C., and Cheung, W. W. L. (2017). Using fuzzy logic to determine the vulnerability of marine species to climate change. Glob. Change Biol. 24, e719–e731. doi: 10.1111/gcb.13869
Kaartvedt, S., Melle, W., Knutsen, T., and Skjoldal, H. R. (1996). Vertical distribution of fish and krill beneath water of varying optical properties. Mar. Ecol. Progr. Ser. 136, 51–58. doi: 10.3354/meps136051
Kamykowski, D., and Zentara, S. J. (1990). Hypoxia in the world ocean as recorded in the historical data set. Deep Sea Res. Pt I 37, 1861–1874. doi: 10.1016/0198-0149(90)90082-7
Karedin, E. P. (1971). About identity between Nematoscelis megalops G.O. Sars, 1885, N. difficilis Hansen 1911 (Euphausiacea, Crustacea) and validity of distinguishing N. difficilis Hansen. Izv. tikhookean. nauchnoissled. Inst. ryb. Khoz. Okeanogr. 75, 121–129.
Karstensen, J., Stramma, L., and Visbeck, M. (2008). Oxygen minimum zones in the eastern tropical Atlantic and Pacific oceans. Progr. Oceanogr. 77, 331–350. doi: 10.1016/j.pocean.2007.05.009
Keeling, R., Körtzinger, A., and Gruber, N. (2010). Ocean deoxygenation in a warming world. Annu. Rev. Mar. Sci. 2, 199–229. doi: 10.1146/annurev.marine.010908.163855
Kiko, R., Hauss, H., Buchholz, F., and Melzner, F. (2016). Ammonium excretion and oxygen respiration of tropical copepods and euphausiids exposed to oxygen minimum zone conditions. Biogeoscience 13, 2241–2255. doi: 10.5194/bg-13-2241-2016
Kiørboe, T. (2013). Zooplankton body composition. Limnol. Oceanogr. 58, 1843–1850. doi: 10.4319/lo.2013.58.5.1843
Koslow, J. A., Goericke, R., Lara-Lopez, A., and Watson, W. (2011). Impact of declining intermediate-water oxygen on deepwater fishes in the California current. Mar. Ecol. Progr. Ser. 436, 207–218. doi: 10.3354/meps09270
Kunze, E., Dower, J. F., Beveridge, I., Dewey, R., and Bartlett, K. P. (2006). Observations of biologically generated turbulence in a coastal inlet. Science 313, 1768–1770. doi: 10.1126/science.1129378
Lavaniegos, B. E., and Ambriz-Arreola, I. (2012). Interannual variability in krill off Baja California in the period 1997–2005. Progr. Oceanogr. 97, 164–173. doi: 10.1016/j.pocean.2011.11.008
Lavín, M. F., Fiedler, P. C., Amador, J. A., Ballance, L. T., Farber-Lorda, J., and Mestas-Nuñez, A. M. (2006). A review of eastern tropical Pacific oceanography: summary. Progr. Oceanogr. 69, 391–398. doi: 10.1016/j.pocean.2006.03.005
Leising, A. W., Schroeder, I. D., Bograd, S. J., Bjorkstedt, E. P., Field, J., Sakuma, K., et al. (2014). State of the California Current 2013–2014: El Nino looming. California. Coop. Ocean. Fish. Investig. Rep. 55, 51–87.
Levin, L. A. (2018). Manifestation, drivers, and emergence of open ocean deoxygenation. Annu. Rev. Mar. Sci. 10, 229–260. doi: 10.1146/annurev-marine-121916-063359
López-Cortés, D., Bustillos-Guzmán, J., and Gárate-Lizárraga, I. (2006). Unusual mortality of krill (Crustacea: Euphausiacea) in Bahía de La Paz, Gulf of California. Pac. Sci. 60, 235–242. doi: 10.1353/psc.2006.0010
Luyten, J. R., Pedlosky, J., and Stommel, H. (1983). The ventilated thermocline. J. Phys. Oceanogr. 13, 292–309.
Mangel, M., and Nicol, S. (2000). Krill and the unity of biology. Can. J. Fish. Aquat. Sci. 57, 1–5. doi: 10.1139/f00-203
Matear, R., Hirst, A., and McNeil, B. (2000). Changes in dissolved oxygen in the Southern Ocean with climate change. Geochem. Geophys. Geosyst. 1:1050.
McLaren, I. A. (1963). Effects of temperature on growth of zooplankton, and the adaptive value of vertical migration. J. Fish. Board Canada 20, 685–727. doi: 10.1139/f63-046
Mueller, C. A., and Seymour, R. S. (2011). The regulation index: a new method for assessing the relationship between oxygen consumption and environmental oxygen. Physiol. Biochem. Zool. 84, 522–532. doi: 10.1086/661953
Murphy, E. J., Watkins, J. L., Trathan, P. N., Reid, K., Meredith, M. P., Thorpe, S. E., et al. (2007). Spatial and temporal operation of the Scotia Sea ecosystem: a review of large-scale links in a krill centred food web. Philos. Trans. R. Soc. B 362, 113–148. doi: 10.1098/rstb.2006.1957
Nowacek, D. P., Friedlaender, A. S., Halpin, P. N., Hazen, E. L., Johnston, D. W., Read, A. J., et al. (2011). Super-aggregations of krill and humpback whales in Wilhelmina Bay, Antarctic Peninsula. PLoS ONE 6:e19173. doi: 10.1371/journal.pone.0019173
Ohman, M. D. (1984). Omnivory by Euphausia pacifica: the role of copepod prey. Mar. Ecol. Progr. Ser. 19, 125–131. doi: 10.3354/meps019125
Olson, D. B., Hitchcock, G. L., Fine, R. A., and Warren, B. A. (1993). Maintenance of the low-oxygen layer in the central Arabian Sea. Deep-Sea Res. Pt II 40, 673–685. doi: 10.1016/0967-0645(93)90051-n
Paulmier, A., and Ruiz-Pino, D. (2009). Oxygen minimum zones (OMZs) in the modern ocean. Prog.Oceanogr. 80, 113–128. doi: 10.1016/j.pocean.2008.08.001
Prince, E. D., and Goodyear, C. P. (2006). Hypoxia-based habitat compression of tropical pelagic fishes. Fish. Oceanogr. 15, 451–464. doi: 10.1111/j.1365-2419.2005.00393.x
R Core Team (2020). R: A Language and Environment for Statistical Computing. Vienna:R Core Team. Available online at: https://www.R-project.org/ (accessed February 19, 2020).
Rabalais, N. N., Turner, R. E., and Wiseman, W. J. (2002). Gulf of Mexico hypoxia, A.K.A. “The dead zone”. Ann. Rev. Ecol. Syst. 33, 235–263. doi: 10.1146/annurev.ecolsys.33.010802.150513
Reid, J. L. (1965). Intermediate waters of the Pacific Ocean. Johns Hopkins Oceanogr. Study 2, 1–85.
Seibel, B. A. (2011). Critical oxygen levels and metabolic suppression in oceanic oxygen minimum zones. J. Exp. Biol. 214, 326–336. doi: 10.1242/jeb.049171
Seibel, B. A., Schneider, J. L., Kaartvedt, S., Wishner, K. F., and Daly, K. L. (2016). Hypoxia tolerance and metabolic suppression in oxygen minimum zone euphausiids: implications for ocean deoxygenation and biogeochemical cycles. Integr. Comp. Biol. 56, 510–523. doi: 10.1093/icb/icw091
Smith, S. E., and Adams, P. B. (1988). Daytime surface swarms of Thysanoessa spinifera (Euphausiacea) in the Gulf of the Farallones, California. Bull. Mar. Sci. 42, 76–84.
Stramma, L., Johnson, G. C., Sprintall, J., and Mohrholz, V. (2008). Expanding oxygen-minimum zones in the tropical oceans. Science 320, 655–658. doi: 10.1126/science.1153847
Stramma, L., Prince, E. D., Schmidtko, S., Luo, J., Hoolihan, J. P., Visbeck, M., et al. (2011). Expansion of oxygen minimum zones may reduce available habitat for tropical pelagic fishes. Nat. Clim. Change 2, 33–37. doi: 10.1038/nclimate1304
Strub, P. T., Mesías, J. M., Montecino, V., Rutllant, J., and Salinas, S. (1998). Coastal ocean circulation off western South America. Sea 11, 273–313.
Sydeman, W. J., Santora, J. A., Thompson, S. A., Marinovic, B., and Lorenzo, E. D. (2013). Increasing variance in North Pacific climate relates to unprecedented ecosystem variability off California. Glob. Change Biol. 19, 1662–1675. doi: 10.1111/gcb.12165
Tanasichuk, R. W. (1999). Interannual variation in the availability and utilization of euphausiids as prey for Pacific hake (Merluccius productus) along the south-west coast of Vancouver Island. Fish. Oceanogr. 8, 150–156. doi: 10.1046/j.1365-2419.1999.00100.x
Teal, J. M., and Carey, F. G. (1967). Respiration of a euphausiid from the oxygen minimum layer. Limnol. Oceanogr. 12, 548–550. doi: 10.4319/lo.1967.12.3.0548
Torres, J. J., and Childress, J. J. (1983). Relationship of oxygen consumption to swimming speed in Euphausia pacifica. Mar. Biol. 74, 79–86. doi: 10.1007/bf00394278
Tremblay, N., Gómez-Gutiérrez, J., Zenteno-Savín, T., Robinson, C. J., and Sánchez-Velasco, L. (2010). Role of oxidative stress in seasonal and daily vertical migration of three krill species in the Gulf of California. Limnol. Oceanogr. 55, 2570–2584. doi: 10.4319/lo.2010.55.6.2570
Tward, C. E., Singh, J., Cygelfarb, W., and McDonald, A. E. (2019). Identification of the alternative oxidase gene and its expression in the copepod Tigriopus californicus. Comp. Biochem.Physiol. B 228, 41–50. doi: 10.1016/j.cbpb.2018.11.003
Verheye, H. M., and Ekau, W. (2005). Maintenance mechanisms of plankton populations in frontal zones in the Benguela and Angola Current systems: a preface. Afr. J. Mar.e Sci. 27, 611–615. doi: 10.2989/18142320509504121
Werner, T., and Buchholz, F. (2013). Diel vertical migration behaviour in Euphausiids of the northern Benguela current: seasonal adaptations to food availability and strong gradients of temperature and oxygen. J. Plank. Res. 35, 792–812. doi: 10.1093/plankt/fbt030
Werner, T., Hünerlage, K., Verheye, H., and Buchholz, F. (2012). Thermal constraints on the respiration and excretion rates of krill, Euphausia hanseni and Nematoscelis megalops, in the northern Benguela upwelling system off Namibia. Afr. J. Mar. Sci. 34, 391–399. doi: 10.2989/1814232x.2012.689620
Werner, T., Tremblay, N., Hünerlage, K., and Buchholz, F. (2015). “Krill worldwide: A comparison of hypoxia tolerances of euphausiid species from Atlantic, Pacific and Polar Regions,” in Proceedings of the Third International Symposium on Effects of Climate Change on the World‘s Oceans, Santos City. Available online at: https://epic.awi.de/id/eprint/37766/ (accessed February 19, 2020).
Wishner, K. F., Outram, D. M., Seibel, B. A., Daly, K. L., and Williams, R. L. (2013). Zooplankton in the eastern tropical north pacific: boundary effects of oxygen minimum zone expansion. Deep-Sea Res. Pt. I 79, 122–140. doi: 10.1016/j.dsr.2013.05.012
Wishner, K. F., Seibel, B. A., Roman, C., Deutsch, C., Outram, D., Shaw, C. T., et al. (2018). Ocean deoxygenation and zooplankton: Very small oxygen differences matter. Sci. Adv. 4:eaau5180. doi: 10.1126/sciadv.aau5180
Wood, C. M. (2018). The fallacy of the Pcrit– are there more useful alternatives? J. Exp. Biol. 221, jeb163717–jeb163719.
Wyrtki, K. (1962). The oxygen minima in relation to ocean circulation. Deep Sea Res. Oceanogr. Abst. 9, 11–23. doi: 10.1016/0011-7471(62)90243-7
Yusseppone, M. S., Rocchetta, I., Sabatini, S. E., Luquet, C. M., Ríos, de Molina, M. D. C., et al. (2018). Inducing the alternative oxidase forms part of the molecular strategy of anoxic survival in freshwater bivalves. Front. Physiol. 9:100.
Zaret, T. M., and Suffern, J. S. (1976). Vertical migration in zooplankton as a predator avoidance mechanism. Limnol. Oceanogr. 21, 804–813. doi: 10.4319/lo.1976.21.6.0804
Keywords: oxygen minimum zones, diel vertical migration, krill, respiration rate, regulation index
Citation: Tremblay N, Hünerlage K and Werner T (2020) Hypoxia Tolerance of 10 Euphausiid Species in Relation to Vertical Temperature and Oxygen Gradients. Front. Physiol. 11:248. doi: 10.3389/fphys.2020.00248
Received: 28 June 2019; Accepted: 04 March 2020;
Published: 24 March 2020.
Edited by:
Silvia Franzellitti, University of Bologna, ItalyReviewed by:
Folco Giomi, University of Padova, ItalySantiago Hernández-León, University of Las Palmas de Gran Canaria, Spain
Copyright © 2020 Tremblay, Hünerlage and Werner. This is an open-access article distributed under the terms of the Creative Commons Attribution License (CC BY). The use, distribution or reproduction in other forums is permitted, provided the original author(s) and the copyright owner(s) are credited and that the original publication in this journal is cited, in accordance with accepted academic practice. No use, distribution or reproduction is permitted which does not comply with these terms.
*Correspondence: Nelly Tremblay, TmVsbHkudHJlbWJsYXlAYXdpLmRl