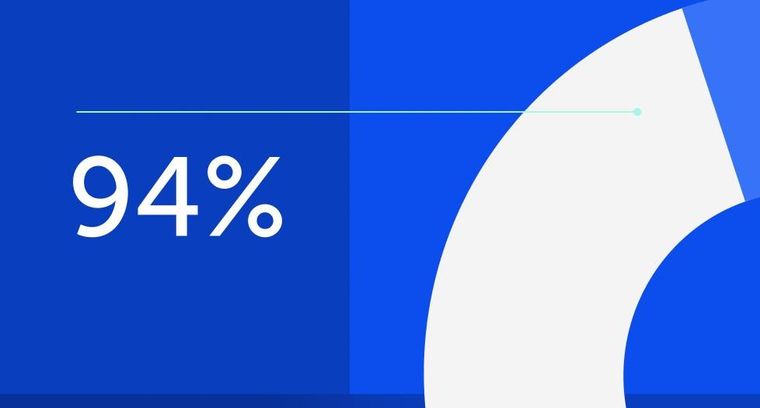
94% of researchers rate our articles as excellent or good
Learn more about the work of our research integrity team to safeguard the quality of each article we publish.
Find out more
ORIGINAL RESEARCH article
Front. Physiol., 11 October 2019
Sec. Invertebrate Physiology
Volume 10 - 2019 | https://doi.org/10.3389/fphys.2019.01282
This article is part of the Research TopicThe Role of Peptide Hormones in Insect Physiology, Biochemistry, and Molecular Biology ProcessesView all 12 articles
The immunological interaction between Drosophila melanogaster and its larval parasitoids has been thoroughly investigated, however, little is known about the interaction between the host and its pupal parasitoids. Pachycrepoideus vindemmiae, a pupal ectoparasitoid of D. melanogaster, injects venom into its host while laying eggs on the puparium, which regulates host immunity and interrupts host development. To resist the invasion of parasitic wasps, various immune defense strategies have been developed in their hosts as a consequence of co-evolution. In this study, we mainly focused on the host immunomodulation by P. vindemmiae and thoroughly investigated cellular and humoral immune response, including cell adherence, cell viability, hemolymph melanization and the Toll, Imd, and JAK/STAT immune pathways. Our results indicated that venom had a significant inhibitory effect on lamellocyte adherence and induced plasmatocyte cell death. Venom injection and in vitro incubation strongly inhibited hemolymph melanization. More in-depth investigation revealed that the Toll and Imd immune pathways were immediately activated upon parasitization, followed by the JAK/STAT pathway, which was activated within the first 24 h post-parasitism. These regulatory effects were further validated by qPCR. Our present study manifested that P. vindemmiae regulated the cellular and humoral immune system of host D. melanogaster in many aspects. These findings lay the groundwork for studying the immunological interaction between D. melanogaster and its pupal parasitoid.
Parasitoids are unique venomous organisms among hymenopteran insects, with an estimated number of species ranging from 150,000 to 600,000 (Mrinalini and Werren, 2017). There is great potential for developing parasitoids as a crucial means of biological control. They lay eggs into the hemocoel (endoparasitoids) or on the surface (ectoparasitoids) of hosts (Moreau and Asgari, 2015). To ensure the survival and development of their offspring, parasitoids circumvent the host immune system with different adaptive strategies, namely, active immune suppression and passive immune evasion (Labrosse et al., 2003). Immune suppression is usually related to virulence factors, including venom (Asgari and Rivers, 2011), polydnaviruses (PDVs) (Gundersen-Rindal et al., 2013), virus-like particles (VLPs) (Grgacic and Anderson, 2006) and ovarian secretion (Mabiala-Moundoungou et al., 2010), and they work alone or cooperate with each other to regulate the cellular and humoral immune responses of hosts (Burke and Strand, 2014). In contrast, immune evasion occurs when wasp eggs are either covered with a fibrous layer or tightly adhered to host tissue, and the hosts fail to recognize it as non-self (Asgari et al., 1998; Eslin and Prévost, 2000; Hu et al., 2003). Both strategies are how parasitic wasps outwit their hosts.
Unlike mammals, insects lack acquired immune response, however, multiple innate defense responses have been highly developed to resist the invasion of parasitoids during the long-term arms race (Hoffmann, 1995). Insect innate immunity consists in cellular and humoral innate immunity (Lemaitre and Hoffmann, 2007). Cellular immunity response is mainly mediated by hemocytes. Much of our current understanding of hemocyte-mediated resistance to wasps lies in encapsulation. The Drosophila parasitoids are supposed to be valuable models for investigating their immunological interactions at the cellular immunity level (Carton et al., 2008). In general, Drosophila melanogaster recognizes parasitoids’ eggs as non-self once parasitoids lay eggs (Russo et al., 1996), followed by recruiting and spreading of plasmatocytes. Ultimately, lamellocytes collaborate with plasmatocytes to surround the wasp eggs and melanin is then deposited to seal it off, which is indispensable for killing the invaders (Williams, 2007). As a prominent humoral immune response of Drosophila, melanization plays an important role in fighting against parasitization (Tang, 2014). Prophenoloxidase (PPO) secreted by crystal cells and lamellocytes mainly contributes to the melanization of the wasp eggs (Dudzic et al., 2015). In addition to the melanization, the Toll, immune deficiency (Imd) and Janus kinase/signal transducer and activator of transcription (JAK/STAT) immune pathways associated with humoral immune responses are other exhaustively studied areas in confronting pathogenic infection and parasitization by parasitic wasps. In the Toll pathway, Dif/Dorsal, once activated, translocates into the nucleus and initiates the expression of antimicrobial peptides (AMPs) (Lehming et al., 1995; Nicolas et al., 1998; Wu and Anderson, 1998). In contrast, caspase-mediated N-terminal cleavage of relish activates the Imd pathway (Kim et al., 2006). Subsequently, N-terminal RHD-containing fragment (Rel-68) enters the nucleus, and a battery of AMPs are robustly produced (Stöven et al., 2000; Stoven et al., 2003). Unlike the complicacy of the Toll and Imd pathways, the JAK/STAT signaling pathway is more succinct, including three cytokine-like ligands named unpaired (upd) (Harrison et al., 1998), upd2 (Hombria et al., 2005), and upd3 (Agaisse et al., 2003; Wright et al., 2011), a transmembrane receptor, JAK and a Stat transcription factor (Rawlings et al., 2004; Myllymaki and Ramet, 2014). In D. melanogaster, an increasing number of studies have revealed that there is a connection between parasitization and the Toll, Imd and JAK/STAT pathways, while the regulatory effects vary greatly in different Drosophila parasitoid models (Wertheim et al., 2005; Martinson et al., 2014; Schmid, 2014; Yang et al., 2015; Louradour et al., 2017). Moreover, reactive oxygen species (ROS), a field of humoral innate immunity that has not been exploited fully, increased sharply in posterior signaling center (PSC) cells under parasitism, which conferred Drosophila resistance to wasp parasitism (Louradour et al., 2017). In short, they elaborately exploit cellular innate immunity and humoral innate immunity to cope with successful parasitism during the long-term antagonistic interaction between Drosophila and their parasitoid wasps.
Meanwhile, parasitoids have developed corresponding defense strategies to aid their progeny’s survival by virtue of various virulence factors. Venom, the fundamental parasitic factor either in endoparasitoids or ectoparasitoids, is the topic of interest in their host immune regulation. The primary functions of venom include inducing hosts paralysis, interrupting host development, suppressing the immunity of their hosts, etc (Coudron et al., 1990; Edwards et al., 2006; Tian et al., 2010; Kryukova et al., 2011). In a larval solitary endoparasitoid Leptopilina boulardi, long gland products induced a drastic decrease and an alteration of actin cytoskeleton in lamellocyte cells to inhibit the encapsulation (Labrosse et al., 2005a, b). Additionally, venom from L. boulardi inhibited the PO cascade of the D. yakuba larval hemolymph (Colinet et al., 2009). As a previously uncharacterized Drosophila parasitoid, Ganaspis sp.1 venom suppressed plasmatocyte calcium burst, resulting in its failure to migrate toward parasitoid eggs (Mortimer et al., 2013). There is some evidence indicating that secretions from the venom gland and ovary collaborate to regulate host physiology in Asobara japonica (Mabiala-Moundoungou et al., 2010). Furthermore, PDV is another thoroughly studied virulence factor, which is equally as important as venom in host immune regulation. In Braconidae and Ichneumonidae, Bracoviruses and Ichnoviruses imitated inhibitor kB (IkB) proteins of Drosophila to regulate immune NF-kappa B signaling by virtue of ankyrins (Bitra et al., 2012; Gueguen et al., 2013). In addition to the venom and PDVs, VLPs from L. heterotoma and L. victoriae also triggered immune suppression responses and further weakened the encapsulation phenotype in the host Drosophila (Morales et al., 2005; Heavner et al., 2017). In short, the mechanism of immunological interactions between parasitoids and their hosts is extremely complex and finely modulated.
Pachycrepoideus vindemmiae (Hymenoptera: Pteromalidae) is a versatile and solitary pupal ectoparasitoid of many flies whose hosts range from Drosophilidae to Anthomyiidae, Calliphoridae, Muscidae, Sarcophagidae, Tachinidae, Tephritidae, and so on (Marchiori and Borges, 2017). Unlike the multiple virulence factors in many koinobiont parasitoids, venom is the only required parasitic factor for successful parasitism of P. vindemmiae. Although there are many lines of research addressing the physiological mechanisms of immune modulation in the larval parasitoids of Drosophila, little has been investigated about the pupal parasitoids, let alone the research of the P. vindemmiae-D. melanogaster model. The main objective of the present study is to investigate the immune response of the host D. melanogaster once parasitized by P. vindemmiae. Our results demonstrated that great changes took place in cellular and humoral immunity of the host, including cell adherence, cell viability, hemolymph melanization and the Toll, Imd, and JAK/STAT immune pathways. Further studies illustrated the necessity of venom in the process of immune modulation. In brief, our present research opens a precedent for studying the pupal parasitoid-Drosophila system, which will contribute to a better understanding of the immunological interactions between the pupal parasitoids and their hosts.
Host D. melanogaster stocks were raised on standard medium at 25°C with 60 ± 5% relative humidity and 16 h:8 h (light: dark) photoperiod. The stock w1118 originating from an indoor reared population was used as wild-type control. The following stocks were obtained from the Bloomington Stock Center (Indiana University, Bloomington, IL, United States): Dipt-lacZ, Drs-GFP (stock ID: 55707), 10∗Stat92E-GFP (stock ID: 26198), and HopTum–1 (stock ID: 8492).
The colony of P. vindemmiae was kindly provided by Prof. Yongyue Lu (South China Agricultural University, Guangzhou, China) in January 2016. Subsequently, P. vindemmiae was maintained with D. melanogaster pupae at 25°C with a photoperiod of 14 h: 10 h (light: dark) as previously described (Chen et al., 2015). Once closed, adults were held in glass containers and fed on 20% (v/v) honey solution.
We could not separate pure hemocytes from D. melanogaster pupae considering that the bled fluids contained many fat granules. As a result, third instar larvae were used to obtain the hemocytes. The cuticle of HopTum–1 third instar larvae was gently pricked by forceps. Hemocytes from three larvae were bled on a glass slide containing 30 μl 10 mM phosphate buffer (PBS), pH 7.4 or different venom reservoir equivalents (VRE) dissolved in 30 μl PBS and allowed to adhere for 1 h. Thus, 1 VRE represents protein equivalents isolated from one venom reservoir and 2 VRE represents that of two venom reservoirs. Once adhered, it was easy to distinguish plasmatocytes and lamellocytes, which constituted the majority of all blood cells in HopTum–1 Drosophlia (Hanratty and Dearolf, 1993). Namely, plasmatocytes are small spherical cells and lamellocytes are large discoid cells. The adhered cells were washed three times with PBS, fixed with 3.7% paraformaldehyde solution (Sangon Biotech, Shanghai, China) for 15 min, and then washed three times with PBS before being permeabilized for 15 min with 0.1% Triton X-100 (Sangon Biotech, Shanghai, China). Subsequently, the fixed samples were incubated with 1% bovine serum albumin (BSA) for 30–60 min once washed three times with PBS. The F-actin was visualized by staining the cells with 1:1000 phalloidin-iFluor 488 (Abcam, Cambridge, United Kingdom) diluted in 1% BSA for 1 h. After this, the cells were washed 3 times with PBS and mounted by the SlowFadeTM Gold Antifade Mountant with DAPI (Life Technologies, Carlsbad, CA, United States). Photos were taken under white light and fluorescence view, respectively, by a Nikon eclipse TS-100 (Nikon, Japan). In the following analysis, the cell area of fluorescence staining larger than 400 μm2 was considered as lamellocytes.
Hemocytes were collected into the wells of a 96-well plate (Corning, New York, NY, United States) as mentioned above and then allowed to adhere for 1 h. Thirty microliter PBS, 1 VRE or Lysis Buffer (Promega, Madison, WI, United States) was added and incubated for 30 min. Cellular mortality was monitored by using the CellToxTM Green Dye (Promega, Madison, WI, United States) according to the manufacturer’s instruction. Dead cells exhibited enhanced fluorescence as a result of the stable bond between dye and DNA, while no appreciable increase was observed in viable cells because of the integrity of the cell membrane. Photos were taken as mentioned above. The fluorescence value was measured with a setting of 485–510 nm as the excitation wavelength and 520–530 nm as the emission wavelength.
For the melanization analysis in vivo, w1118 pupae within 12 h pupation were collected and then injected with 23 nl 1 mg/ml BSA, saturated phenylthiourea (PTU) or 0.5 VRE (half dilution of 1 VRE), 1 VRE or 2 VRE, respectively. Three hours later, pupae were inspected under a Leica DFC425 Camera attached to a stereomicroscope Leica M205 A (Leica, Wetzler, Germany). The melanization analysis was performed according to the previous study with some modifications (Gregorio et al., 2002). Briefly, about 15 D. melanogaster pupae (20 mg in total) were ground in liquid nitrogen and immediately suspended in 100 μl PBS, PTU or VRE dissolved in Tris buffer (100 mM, pH 7.2) followed by 16,000 g for 20 min centrifugation, and the supernatant was transferred to a new tube. After 30 min incubation at room temperature, 10 μl aliquot was mixed with the PO assay mixture prepared as previously described and subjected to assay by measuring the optical density (OD) at 520 nm (Gregorio et al., 2002).
To determine the effects of parasitism on the Toll, Imd and JAK/STAT pathways, downstream transcription factors, AMPs or stress factors, including drosomycin, diptericin, stat92E and thioester-containing protein 1(Tep1), were used as indicators. Hence, Dipt-lacZ; Drs-GFP stocks were used for both lacZ activity analysis and fluorescence microscopy. GFP detection was also conducted in 10∗Stat92E-GFP stock. Briefly, pupae were collected and parasitized for 1 h within 12 h after pupation. It was assumed that successful parasitism occurred when the envenomation lasted for more than 1 min, and the remaining that did not meet the criteria were removed. Pupae were reared as mentioned above. Then, pupae were photographed at 1, 6, 12, 24, 48, and 72 h after parasitization with GFP fluorescence channel using Nikon AZ100M (Nikon, Tokyo, Japan). Unparasitized D. melanogaster pupae were used as control.
LacZ activity was analyzed by quantifying the enzymatic activity of β-galactosidase as the previous study (Romeo and Lemaitre, 2008). In brief, five pupae were collected into a 2.0 ml Eppendorf tube. Then, 250 μl buffer A (60 mM Na2HPO4, 60 mM NaH2PO4, 10 mM KCl, 1 mM MgSO4, and 50 mM β-mercaptoethanol, pH 8.0) was added and homogenized for 30 s followed by supplementation of 250 μl buffer A and quickly vortexed. The mixture was centrifuged at 6000 × g for 5 min and the supernatant was transferred to a new 1.5 ml Eppendorf tube. Protein concentration was determined by a Modified Bradford Protein Assay Kit (Sangon Biotech, Shanghai, China) according to the manufacturer’s protocol. Finally, 30 μl aliquot was transferred to 96-well plates and 250 μl of 0.35 mg/ml O-nitrophenyl-β-D-galactoside dissolved in buffer A was added to each well followed by 37°C incubation. The β-galactosidase activity was determined at regular time intervals (10 min) by measuring the OD420 nm, and lacZ activity was calculated as Miller’s description: [(ΔODmin)/ΔTmin]/protein concentration/0.0045 (Romeo and Lemaitre, 2008).
Both parasitized and unparasitized w1118 pupae (5 each) were homogenized in 1 ml Trizol reagent (Invitrogen, Carlsbad, CA, United States). The total RNA was extracted according to the manufacturer’s protocol and cDNA was synthesized by using a PrimeScriptTM RT Reagent Kit with gDNA Eraser (Takara, Beijing, China). Quantitative RT-PCR (qPCR) was carried out using the TB GreenTM Premix Ex TaqTM II (Tli RNaseH Plus) (Takara, Beijing, China) and run on a Bio-Rad CFX Connect (Bio-Rad, Hercules, CA, United States) instrument according to the manufacturer’s instructions. Relative expression levels of drosomycin, diptericin and Tep1 were quantified and further normalized to reference gene RPL32 (also referred as R49) using 2–ΔΔCT method (Livak and Schmittgen, 2001). All the primers used were based on the previous study (Neyen et al., 2014).
The phalloidin staining area of hemocytes and the fluorescence intensity of pupal microscopy were measured by using image processing software, Image J 1.8.0 (Image J, NIH, United States). The total corrected fluorescence (TCF) of pupal microscopy was calculated as follows: integrated fluorescence density – (area of photograph) × (mean fluorescence of background). Data for two groups or more than three groups were analyzed by unpaired two-tailed student’s t-test and one-way analysis of variance (ANOVA) with Tukey’s test, respectively. In addition, a Chi-square test was conducted to test the difference in data of Supplementary Figure S1. There was statistical significance if P < 0.05. All statistical analyses were carried out using the data processing system (DPS) package version 9.5 (Tang and Zhang, 2013). All figures were plotted using GraphPad Prism 7.0 (GraphPad, San Diego, CA, United States).
As shown in Figure 1, plasmatocytes and lamellocytes adhered to the plate within 1 h after PBS treatment. The fluorescence area of 985 cells was measured and the results indicated that total hemocyte population comprised approximately 22% lamellocytes (Supplementary Figure S1). However, compared to the PBS control (Figure 1A), the ratio of lamellocytes was significantly decreased to 16% when treated with 1 VRE (Figure 1B). With the increasing dose of VRE, fewer adherent lamellocytes were observed, and the total number of lamellocytes declined to about 4% following 2 VRE incubation (Figure 1C). In contrast, low-concentration venom (0.67 VRE, 0.33 VRE and 0.17 VRE) had limited effects on lamellocyte adherence (data not shown).
Figure 1. Immunofluorescence detection of hemocytes after venom treatment. The stained hemocytes incubated with PBS (A–A′′), 1 VRE (B–B′′), or 2 VRE (C–C′′) were photographed using fluorescence microscope. Subsequently, the staining area was counted. Plasmatocytes (P) and lamellocytes (L) are each indicated. Blue represents the nuclei stained with 4′, 6-diamidino-2-phenylindole (DAPI) and green represents the cytoskeleton marked with phalloidin 488.
To investigate whether the dysfunction in lamellocyte adherence was attributed to cell death, cell viability was detected using cellToxTM green dye staining (Figure 2). In contrast to the considerably high survival rate under PBS treatment and abrupt decline in viable hemocytes under lysis treatment (Figures 2A,C), the percentage of GFP-positive cells was about twice as high as that in the PBS control after 1 VRE incubation (Figure 2B). Similar results were obtained when fluorescence value was measured, that is, crude venom induced a significant increase (2.12-fold) in fluorescence value (P < 0.05) (Supplementary Figure S2). Surprisingly, our findings indicated that GFP-positive cells were mainly occupied by plasmatocytes rather than lamellocytes in the presence of venom. Therefore, it was inferred that the functional disturbance of adherence in lamellocytes was unrelated to the cell death.
Figure 2. Cell viability assay after venom treatment. The hemocytes were incubated with PBS (A–A′′), 1 VRE (B–B′′), and lysate (C–C′′) followed by microscopic examination under white, fluorescent, and merged view, respectively. Fluorescently labeled cells represent dead cells.
To determine whether crude venom inhibited the melaninization of hemolymph, PO activity of pupal hemolymph was determined both in vivo and in vitro. As shown in Figure 3A, there was a slight melanization at the wound after 1 VRE injection compared to the strong melanization induced by BSA injection. In addition, quantitative analysis of PO activity was also conducted. Our results showed that 0.5, 1 and 2 VRE inhibited the melanization of the host hemolymph to different degrees (Figure 3B). Thus, 2 VRE significantly blocked the blackening of the hemolymph even after 40 min incubation. By comparison, a weaker inhibitory effect was observed in 1 VRE treatment. It appeared that 0.5 VRE had a small but significant effect on melanization after 10, 20, and 30 min incubation; however, the effect disappeared after 40 min incubation. These findings demonstrated that the inhibition of hemolymph melanization by crude venom was dose-dependent. Furthermore, these results shed light on the inhibitory effect of venom components on the host PO cascade.
Figure 3. Effects of venom on melaninization of the host hemolymph. (A) Microscope inspection of w1118 pupae was performed 3 h after injection with BSA, 1 VRE, or PTU. (B) PO activity was assayed every 10 min by measuring the OD520 after hemolymph was co-incubated with BSA, PTU, or VRE. The results are shown as the mean ± standard error (n = 3); different lowercase letters above bars for the same time point indicate significant difference (P < 0.05).
To investigate the effect of parasitism on the Toll immune pathway, the expression level of drosomycin, a specific marker gene of the Toll immune pathways, was quantified. Results showed that the transcription levels of drosomycin increased sharply (46-fold) after 6 h of parasitism, and this effect lasted up to 72 h post-parasitism, although it was not shown at 48 h (Figure 4A). To further confirm the reliability of the results, protein expression level of drosomycin was quantified by measuring the fluorescence of drosomycin-GFP in Drosophila (Figure 4B). As Figure 5 showed, stronger fluorescence was generated on the parasitized Drosophila compared to unparasitized ones. Additionally, the fluorescence intensity was compared between parasitized and unparasitized Drosophila. Results showed that a higher level of drosomycin was induced both at the transcription level and the protein expression level once parasitized, whereas contrary circumstances happened at 48h post-parasitism (Figure 4). This unusual result might be explained by the smaller difference in qPCR results between unparasitized (0.52) and parasitized (0.40). Overall, it can be concluded that parasitism by P. vindemmiae activates the Toll pathway-dependent immune response.
Figure 4. Effects of parasitism on the Toll pathway. (A) mRNA expression levels of drosomycin at 1, 6, 12, 24, 48, and 72 h both in parasitized and unparasitized Drosophila (n = 3), and ribosomal protein 49 (R49) was used as internal reference. (B) Fluorescence intensity measurement of drosomycin-GFP in parasitized and unparasitized Drosophila based on the time points mentioned above (n ≥ 10). The fluorescence intensity of 1 h unparasitized was normalized to 1.0. The results are shown as the mean ± standard error; ∗P < 0.05, ∗∗P < 0.01, and ∗∗∗P < 0.001 compared to unparasitized.
Figure 5. Fluorescence microscopy of unparasitized and parasitized drosomycin-GFP in Drosophila at different times. The green fluorescence was visualized through the cuticle using a florescence microscope.
Similarly, the Imd immune pathway is equally as important as the Toll pathway in immune defense response in D. melanogaster. Once parasitized by P. vindemmiae, the expression level of the Imd pathway specific marker gene diptericin was upregulated. As shown in Figure 6A, the mRNA transcriptional level of diptericin increased more than 10-fold at 1 h post-parasitism, and the high fold change lasted to 72 h compared with the unparasitized group. At the same time, lacZ enzymatic activity was basically consistent with qPCR results. As Figure 6B shows, the enzymatic activity of parasitized Drosophila was significantly higher than unparasitized both in the early and late stage of parasitism. However, the higher enzymatic activity was observed in unparasitized Drosophila at 48 h. Regardless of this point, parasitism contributed to the activation of the Imd immune pathway both at the transcription and the protein expression levels.
Figure 6. Effects of parasitism on the Imd pathway. (A) mRNA expression levels of diptericin at 1, 6, 12, 24, 48, and 72 h in parasitized and unparasitized Drosophila (n = 3). R49 was used as internal reference. (B) Enzymatic activity assay of diptericin-lacZ both in parasitized and unparasitized Drosophila based on the time points mentioned above (n ≥ 10). The enzymatic activity of 1 h unparasitized was normalized to 1. The results are shown as the mean ± standard error; ∗∗P < 0.01 and ∗∗∗P < 0.001 compared to unparasitized.
Unlike the multifunctionality of the Toll and Imd immune pathways, the JAK/STAT immune pathway plays roles specifically in the process of immune responses against parasitoids and viruses. The Transcriptional level of Tep1, a marker gene of the JAK/STAT pathway, was detected. Similar with the previous results, the mRNA level of Tep1 increased several times in D. melanogaster pupae once parasitized by P. vindemmiae, which lasted until the later stage of parasitization (Figure 7A). To clarify the facticity of the qPCR results, the expression levels of Stat92E, a transcription factor of Tep1, were further investigated by analyzing the fluorescence of 10∗Stat92E-GFP Drosophila both in unparasitized and parasitized pupae at 1, 6, 12, 24, 48, and 72 h. Experimental results indicated that there was a stronger GFP fluorescence signal at 1, 6, and 12 h in parasitized Drosophila (Figure 7B). However, no significant difference was shown at 24 h between the parasitized and unparasitized (Figure 7B). Conversely, as shown in Figure 8, a significant inhibitory effect on the activation of Stat92E at 48h post-parasitism was revealed, that is, weaker fluorescence intensity was recorded. These results indicated that the JAK/STAT immune pathway was activated during the early stage of parasitization.
Figure 7. Effects of parasitism on the JAK/STAT pathway. (A) mRNA expression levels of Tep1 at 1, 6, 12, 24, 48, and 72 h both in parasitized and unparasitized Drosophila (n = 3), and R49 was used as internal reference. (B) Fluorescence intensity analysis of 10∗Stat92E-GFP Drosophila both in parasitized and unparasitized based on the time points mentioned above (n ≥ 10), and the fluorescence intensity of 1 h unparasitized was normalized to 1. The results are shown as the mean ± standard error; ∗P < 0.05, ∗∗P < 0.01, and ∗∗∗P < 0.001 compared to unparasitized.
Figure 8. Fluorescence microscopy of unparasitized and parasitized 10∗Stat92E-GFP in Drosophila at different times. The green fluorescence was visualized through the cuticle using a florescence microscope.
The innate immune system of host insects involves cellular and humoral aspects. They orchestrate together to resist the successful parasitism of parasitic wasps. In Drosophila, hemocytes are the key players in cellular immunity response of Drosophila, which constitute the crucial adjective weapons in cellular immunity, especially lamellocytes, and are only produced by the immune system under parasitization or aberrant conditions (Rizki and Rizki, 1992). The precondition for encapsulation is that plasmatocytes are recruited and spread over the egg surface followed by adhesion of lamellocytes to the plasmatocyte-covered wasp eggs (Anderl, 2015). It has been reported that A. japonica venom significantly disturbed the spreading behavior of D. melanogaster hemocytes (Furihata et al., 2013). In a L. Boulardi/D. melanogaster model, virulent extracts of venom reservoir induced a drastic decrease in lamellocyte counts (Labrosse et al., 2005a). More research showed that more than 80% of plasmatocytes and granular cells from the host Pseudoplusia includens were unable to spread after oviposition by Microplitis demolitor within 2 h (Strand and Noda, 1991). In a closely related species, Nasonia vitripennis, the total number of plasmatocytes declined sharply after 60 min envenomation and further in vitro experiment proved that isolated crude venom indeed blocked adhesion and spreading of hemocytes (Rivers et al., 2002). In the present study, we primarily focused on alterations in lamellocyte adherence ability following crude venom treatment. Consistent with the above studies, P. vindemmiae crude venom exerted a similar inhibitory effect on adherence of lamellocytes as that of N. vitripennis, which disturbed normal lamellocyte function involved in encapsulation. In addition, the ratio of adhered lamellocytes gradually decreased with the increasing venom dosage. To investigate whether the disability of adherence in lamellocytes was caused by cell death, cell viability was evaluated. The results showed that cell death was indeed induced by venom but mainly on plasmatocytes. Hence, the underlying mechanism for inactivation in adhesion of lamellocytes is still unknown and further investigations are still needed.
Humoral immunity, equally important as cellular immunity, is common to all insects as well. As one of the prominent humoral immunity responses, melanization plays important roles in resisting microbial infection and parasitization. During melanization, phenols are oxidized to quinones followed by the formation of melanin (Tang, 2014). Successful parasitism is greatly determined by the dysfunction in hemolymph melanization. As a counter-immune strategy, venom proteins act in inhibiting the process of PO cascades in host insects. Several lines of research have identified venom proteins that are involved in the process, such as 50-kDa serine proteinase homolog (Vn50) (Asgari et al., 2003), Serpin (Colinet et al., 2009; Yan et al., 2017), Kazal-type serine protease inhibitors (Qian et al., 2015) and defensin-like peptide (Tian et al., 2010). Given that the immune response of D. melanogaster was successfully suppressed by venom, successful parasitism occurs and the wasp eggs hatch followed by larval feeding. This is a consequence of co-evolution in the arms race between parasitoids and their hosts. In this study, we thoroughly investigated the effects of the venom cocktail on the melanization of Drosophila pupae. First, in vivo injection indicated that venom significantly inhibited the blackening of the wound. Furthermore, in contrast to BSA treatment in vitro, hemolymph incubated with venom melanized to a lesser extent, and this inhibitory effect was gradually enhanced as the concentration increased from 0.5 VRE to 2 VRE, showing a dose-dependent effect. However, it is still a black box regarding how many venom proteins really function in this process, let alone their inhibitory mechanism.
As the core portion of humoral response, the Toll, Imd and JAK/STAT pathways play important roles against parasitism. For instance, the loss-of-function mutations of the JAK/STAT and Toll pathways in Drosophila larvae exhibited inadequate capacity to encapsulate the eggs of L. boulardi (Sorrentino et al., 2004). On the contrary, transgenic Drosophila of gain-of-function in the JAK/STAT or Toll pathway led to the plentiful formation of melanotic tumors (William and Jan, 1981; Gerttula et al., 1988; Harrison et al., 1995; Luo et al., 1995). In L. boulardi, the JAK/STAT signaling was significantly activated 27 h post-parasitization (Yang et al., 2015). Different from studies about the activation of the JAK/STAT and Toll pathways on parasitization, there have been limited studies about the crosslink between parasitization and the Imd immune pathway except for several lines of research based on high-throughput omics analysis. For instance, L. heterotoma venom specifically inhibited the Toll and Imd pathway signaling in fat body by microarray analysis (Schlenke et al., 2007). In contrast, genome-wide analysis indicated that transcription factor Relish and several AMPs downstream of the Imd pathway were strongly unregulated in response to A. tabida attack (Wertheim et al., 2005). In N. vitripennis, significant increases of several immune-related genes of the Toll and Imd pathways provided evidence that venom activated certain immune responses in envenomated hosts by whole gene expression profile analysis (Martinson et al., 2014). Thus, an 8.6-fold and 2.4-fold upregulation on spätzle and relish were shown, respectively. As a closely related species of Nasonia, it is inferred that parasitization may have similar effects on Drosophila immune signaling by P. vindemmiae. As expected, here we showed that the Toll and Imd immune pathways were activated by monitoring the transcription and expression of transcription factors and AMPs in vivo after parasitization. Similarly, our findings also indicated that the JAK/STAT pathway was activated during the early stage of parasitization, lagging behind the Toll and Imd pathways during this process. One unanticipated finding was that contrary circumstances occurred between the transcription level and the protein expression level at 48h post-parasitism. This observation could be due to the fact that pupae experienced a tissue regeneration process during this period. Separation of the pupal cuticle was initiated at 24 h after puparium formation and at around 48–50 h, the adult cuticle was formed (Carol et al., 1982). The transcript levels of a set of genes changed significantly during this process (Arbeitman et al., 2002). The latter point proposed by Wright et al. also found that a set of five L71 genes encoding polypeptides resembling AMPs were activated owing to the protection of pupal cuticle from bacterial infections in the late pupal stage (Wright et al., 1996). Therefore, their transcription or protein expression levels might not truly reflect the response. After 72 h in the unparasitized pupae, the eclosion of adults begins within the next few hours. Consequently, we did not monitor the transcription level and the protein expression level beyond 72 h. In fact, it is more complicated to evaluate the definite effect of parasitism on the Toll, Imd and JAK/STAT pathways. We postulate that venom plays major roles during this process.
Previous studies on the regulation of Drosophila immune response by venom were mainly performed on larval endoparasitoids. In particular, the genera Leptopilina, Ganaspis and Asobara have been well studied in the context of immunology. Our findings provided preliminary information on the immunological interplay between D. melanogaster and its pupal ectoparasitoid P. vindemmiae for the first time (Figure 9). Based on the reported evidence, the importance of host immune regulation by ectoparasitoids could be summarized as follows. First, many immune-related proteins have been identified in ectoparasitoid venoms such as serine proteases and serine protease inhibitors (De Graaf et al., 2010; Zhu, 2016). Our unpublished results showed that immune-related proteins occupy the major categories of proteins in P. vindemmiae venom, a result consistent with these reports. It is reasonable then to believe that venom is important for ectoparasitoids to regulate the host immune response. In ectoparasitoid S. guani, venom was essential for the survival of the larvae to avoid host cellular immune attack, considering that they would contact with the hosts’ hemolymph released from the puncture wound (Li et al., 2018). Similarly, P. vindemmiae injected venom into the host hemocoel prior to laying eggs, and it is inferred that the persistently virulent effects on cellular defense of the host Drosophila also contributes to the successful development of their offspring, allowing the wasp offspring to feed more readily. More evidence revealed that envenomation by N. vitripennis induced significant increases in AMPs and their corresponding regulatory genes (Martinson et al., 2014), and our finding is consistent with the reported data. Based on this elaboration, we guess that the activation of the immune pathway of the host Drosophila is a preventative measure against bacterial or fungal infection and further enhances the nutritional quality of the host for larval feeding. In the host Sarcophaga bullata, melanization is an elaborative humoral immune response against foreign proteins such as parasitoid venom, and was inhibited by calreticulin from N. vitripennis venom (Siebert et al., 2015). Thus, it is speculated that the suppression of the host melanization is a coping strategy to avoid the dysfunction of venom for P. vindemmiae. Taken together, it is of vital importance for ectoparasitoid P. vindemmiae to regulate the host immune response. However, more research is still needed for understanding the underlying regulatory mechanisms.
Figure 9. The schematic representation of immunological interaction between P. vindemmiae and the host D. melanogaster.
As a groundbreaking research into the pupal ectoparasitoid of D. melanogaster, P. vindemmiae is attracting considerable interest in the field of biological control of fruit flies. Several attempts have been made on its potential for biological control in the last few years. One of the practical cases was that P. vindemmiae has prospects for control of olive fruit fly, Bactrocera oleae (Hoelmer et al., 2011). Additionally, a recent article reviewed that Trichopria drosophilae, a pupal parasitoid of D. suzukii, is desirable for high efficacy in biological control (Woltering et al., 2019). Similarly, as a well-known generalist pupal parasitoid of Diptera Cyclorrhapha, P. vindemmiae also possesses enormous potential on control of D. suzukii (Bonneau et al., 2019; Schlesener et al., 2019). To sum up, our explorative work on the crosstalk between ectoparasitoid P. vindemmiae and the host Drosophila will lay a foundation for providing new insights into biological control of fruit flies, and vastly propels the application of bio-control agent.
The ways that host immune systems are regulated vary greatly in different host/parasitoid systems. Here, we proposed that venom of P. vindemmiae functioned as the crucial regulator in cellular and humoral immune signaling of the host D. melanogaster. Our test results showed that the decreased cell adhesion and viability, weakened hemolymph melanization and dysfunctions of the Toll, Imd and JAK/STAT pathways were associated with the high potency of venom. However, where the actual targets of venom lied is still unknown. The data presented in this study clearly advance the knowledge of the immunological interaction between Drosophila and its pupal ectoparasitoid P. vindemmiae.
All datasets generated for this study are included in the manuscript/Supplementary Files.
We declare that appropriate ethical approval and licenses were obtained during our research.
LY, BW, and B-BW performed the experiments. LY, M-ML, and QF analyzed the data. LY, QF, Q-SS, and G-YY designed the experiments. LY, Q-SS, and G-YY wrote the manuscript. All authors gave final approval for publication.
This study was supported by grants from the Major International (Regional) Joint Research Project of National Natural Science Foundation (Grant No. 31620103915), the Key Program of National Natural Science Foundation of China (Grant No. 31830074), the National Key R&D Program of China (Grant No. 2017YFD0200400), the Program for Chinese Innovation Team in Key Areas of Science and Technology of Ministry of Science and Technology of the People’s Republic of China (2016RA4008), and the Program for Chinese Outstanding Talents in Agricultural Scientific Research of Ministry of Agriculture and Rural Affairs of the People’s Republic of China.
The authors declare that the research was conducted in the absence of any commercial or financial relationships that could be construed as a potential conflict of interest.
We greatly thank Prof. Yongyue Lu (South China Agricultural University, Guangzhou, China) for kindly providing the colony of the parasitoid, P. vindemmiae.
The Supplementary Material for this article can be found online at: https://www.frontiersin.org/articles/10.3389/fphys.2019.01282/full#supplementary-material
Agaisse, H., Petersen, U.-M., Boutros, M., Mathey-Prevot, B., and Perrimon, N. (2003). Signaling role of hemocytes in Drosophila JAK/STAT-dependent response to septic injury. Dev. Cell 5, 441–450. doi: 10.1016/s1534-5807(03)00244-2
Anderl, I. (2015). Activation of the Cellular Immune Response in Drosophila Melanogaster Larvae. Ph.D. thesis, Umeå University, Umeå.
Arbeitman, M. N., Furlong, E. E. M., Imam, F., Johnson, E., Null, B. H., Baker, B. S., et al. (2002). Gene expression during the life cycle of Drosophila melanogaster. Science 297, 2270–2275. doi: 10.1126/science.1072152
Asgari, S., and Rivers, D. B. (2011). Venom proteins from endoparasitoid wasps and their role in host-parasite interactions. Annu. Rev. Entomol. 56, 313–335. doi: 10.1146/annurev-ento-120709-144849
Asgari, S., Ulrich, T., Craig, W., and Otto, S. (1998). A protein with protective properties against the cellular defense reactions in insects. Proc. Natl. Acad. Sci. U.S.A. 95, 3690–3695. doi: 10.1073/pnas.95.7.3690
Asgari, S., Zhang, G., Zareie, R., and Schmidt, O. (2003). A serine proteinase homolog venom protein from an endoparasitoid wasp inhibits melanization of the host hemolymph. Insect Biochem. Mol. Biol. 33, 1017–1024. doi: 10.1016/s0965-1748(03)00116-4
Bitra, K., Suderman, R. J., and Strand, M. R. (2012). Polydnavirus Ank proteins bind NF-kappaB homodimers and inhibit processing of relish. PLoS Pathog. 8:e1002722. doi: 10.1371/journal.ppat.1002722
Bonneau, P., Renkema, J., Fournier, V., and Firlej, A. (2019). Ability of Muscidifurax raptorellus and other parasitoids and predators to control Drosophila suzukii populations in raspberries in the laboratory. Insects 10:E68. doi: 10.3390/insects10030068
Burke, G. R., and Strand, M. R. (2014). Systematic analysis of a wasp parasitism arsenal. Mol. Ecol. 23, 890–901. doi: 10.1111/mec.12648
Carol, J. C., Donald, J. S., and James, W. F. (1982). The cuticle proteins of Drosophila melanogaster stage specificity. Dev. Biol. 89, 379–388. doi: 10.1016/0012-1606(82)90326-8
Carton, Y., Poirié, M., and Nappi, A. J. (2008). Insect immune resistance to parasitoids. Insect Sci. 15, 67–87. doi: 10.1111/j.1744-7917.2008.00188.x
Chen, W., He, Z., Ji, X. L., Tang, S. T., and Hu, H. Y. (2015). Hyperparasitism in a generalist ectoparasitic pupal parasitoid, Pachycrepoideus vindemmiae (Hymenoptera: Pteromalidae), on its own conspecifics: when the lack of resource lead to cannibalism. PLoS One 10:e0124305. doi: 10.1371/journal.pone.0124305
Colinet, D., Dubuffet, A., Cazes, D., Moreau, S., Drezen, J. M., and Poirie, M. (2009). A serpin from the parasitoid wasp Leptopilina boulardi targets the Drosophila phenoloxidase cascade. Dev. Comp. Immunol. 33, 681–689. doi: 10.1016/j.dci.2008.11.013
Coudron, T. A., Kelly, T. J., and Puttler, B. (1990). Developmental responses of Trichoplusia ni (Lepidoptera: Noctuidae) to parasitism by the ectoparasite Euplectrus plathypenae (Hymenoptera: Eulophidae). Arch. Insect Biochem. Physiol. 13, 83–94. doi: 10.1002/arch.940130108
De Graaf, D. C., Aerts, M., Brunain, M., Desjardins, C. A., Jacobs, F. J., Werren, J. H., et al. (2010). Insights into the venom composition of the ectoparasitoid wasp Nasonia vitripennis from bioinformatic and proteomic studies. Insect Mol. Biol. 19(Suppl. 1), 11–26. doi: 10.1111/j.1365-2583.2009.00914.x
Dudzic, J. P., Kondo, S., Ueda, R., Bergman, C. M., and Lemaitre, B. (2015). Drosophila innate immunity: regional and functional specialization of prophenoloxidases. BMC Biol. 13:81. doi: 10.1186/s12915-015-0193-6
Edwards, J. P., Bell, H. A., Audsley, N., Marris, G. C., Kirkbride-Smith, A., Bryning, G., et al. (2006). The ectoparasitic wasp Eulophus pennicornis (Hymenoptera: Eulophidae) uses instar-specific endocrine disruption strategies to suppress the development of its host Lacanobia oleracea (Lepidoptera: Noctuidae). J. Insect Physiol. 52, 1153–1162. doi: 10.1016/j.jinsphys.2006.08.003
Eslin, P., and Prévost, G. (2000). Racing against host’s immunity defenses a likely strategy for passive evasion of encapsulation in Asobara tabida parasitoids. J. Insect Physiol. 46, 1161–1167. doi: 10.1016/s0022-1910(99)00227-9
Furihata, S. X., Matsumoto, H., Kimura, M. T., and Hayakawa, Y. (2013). Venom components of Asobara japonica impair cellular immune responses of host Drosophila melanogaster. Arch. Insect Biochem. Physiol. 83, 86–100. doi: 10.1002/arch.21093
Gerttula, S., Jin, Y., and Anderson, K. V. (1988). Zygotic expression and activity of the Drosophila Toll gene, a gene required maternally for embryonic dorsal-ventral pattern formation. Genetics 119, 123–133.
Gregorio, E. D., Han, S. J., Lee, W. J., Baek, M. J., Osaki, T., Kawabata, S. I., et al. (2002). An immune-responsive serpin regulates the melanization cascade in Drosophila. Dev. Cell 3, 581–592. doi: 10.1016/s1534-5807(02)00267-8
Grgacic, E. V., and Anderson, D. A. (2006). Virus-like particles: passport to immune recognition. Methods 40, 60–65. doi: 10.1016/j.ymeth.2006.07.018
Gueguen, G., Kalamarz, M. E., Ramroop, J., Uribe, J., and Govind, S. (2013). Polydnaviral ankyrin proteins aid parasitic wasp survival by coordinate and selective inhibition of hematopoietic and immune NF-kappa B signaling in insect hosts. PLoS Pathog. 9:e1003580. doi: 10.1371/journal.ppat.1003580
Gundersen-Rindal, D., Dupuy, C., Huguet, E., and Drezen, J.-M. (2013). Parasitoid polydnaviruses: evolution, pathology and applications. Biocontrol Sci. Technol. 23, 1–61. doi: 10.1080/09583157.2012.731497
Hanratty, W. P., and Dearolf, C. R. (1993). The Drosophila Tumorous lethal hematopoietic oncogene is a dominant mutation in the hopscotch locus. Mol. Gen. Genet. 238, 33–37.
Harrison, D. A., Binari, R., Nahreini, T. S., Gilman, M., and Perrimonl, N. (1995). Activation of a Drosophila Janus kinase (JAK) causes hematopoietic neoplasia and developmental defects. EMBO J. 14, 2857–2865. doi: 10.1002/j.1460-2075.1995.tb07285.x
Harrison, D. A., Mccoon, P. E., Binari, R., Gilman, M., and Perrimon, N. (1998). Drosophila unpaired encodes a secreted protein that activates the JAK signaling pathway. Genes Dev. 12, 3252–3263. doi: 10.1101/gad.12.20.3252
Heavner, M. E., Ramroop, J., Gueguen, G., Ramrattan, G., Dolios, G., Scarpati, M., et al. (2017). Novel organelles with elements of bacterial and eukaryotic secretion systems weaponize parasites of Drosophila. Curr. Biol. 27, 2869–2877. doi: 10.1016/j.cub.2017.08.019
Hoelmer, K. A., Kirk, A. A., Pickett, C. H., Daane, K. M., and Johnson, M. W. (2011). Prospects for improving biological control of olive fruit fly, Bactrocera oleae (Diptera: Tephritidae), with introduced parasitoids (Hymenoptera). Biocontrol Sci. Technol. 21, 1005–1025. doi: 10.1080/09583157.2011.594951
Hoffmann, J. A. (1995). Innate immunity of insects. Curr. Opin. Immunol. 7, 4–10. doi: 10.1016/0952-7915(95)80022-0
Hombria, J. C., Brown, S., Hader, S., and Zeidler, M. P. (2005). Characterisation of Upd2, a Drosophila JAK/STAT pathway ligand. Dev. Biol. 288, 420–433. doi: 10.1016/j.ydbio.2005.09.040
Hu, J., Zhu, X. X., and Fu, W. J. (2003). Passive evasion of encapsulation in Macrocentrus cingulum Brischke (Hymenoptera: Braconidae), a polyembryonic parasitoid of Ostrinia furnacalis Guenée (Lepidoptera: Pyralidae). J. Insect Physiol. 49, 367–375. doi: 10.1016/s0022-1910(03)00021-0
Kim, M., Lee, J. H., Lee, S. Y., Kim, E., and Chung, J. (2006). Caspar, a suppressor of antibacterial immunity in Drosophila. Proc. Natl. Acad. Sci. U.S.A. 103, 16358–16363. doi: 10.1073/pnas.0603238103
Kryukova, N. A., Dubovskiy, I. M., Chertkova, E. A., Vorontsova, Y. L., Slepneva, I. A., and Glupov, V. V. (2011). The effect of Habrobracon hebetor venom on the activity of the prophenoloxidase system, the generation of reactive oxygen species and encapsulation in the haemolymph of Galleria mellonella larvae. J. Insect Physiol. 57, 796–800. doi: 10.1016/j.jinsphys.2011.03.008
Labrosse, C., Carton, Y., Dubuffet, A., Drezen, J. M., and Poirie, M. (2003). Active suppression of D. melanogaster immune response by long gland products of the parasitic wasp Leptopilina boulardi. J. Insect Physiol. 49, 513–522. doi: 10.1016/s0022-1910(03)00054-4
Labrosse, C., Eslin, P., Doury, G., Drezen, J. M., and Poirie, M. (2005a). Haemocyte changes in D. melanogaster in response to long gland components of the parasitoid wasp Leptopilina boulardi: a Rho-GAP protein as an important factor. J. Insect Physiol. 51, 161–170. doi: 10.1016/j.jinsphys.2004.10.004
Labrosse, C., Stasiak, K., Lesobre, J., Grangeia, A., Huguet, E., Drezen, J. M., et al. (2005b). A RhoGAP protein as a main immune suppressive factor in the Leptopilina boulardi (Hymenoptera, Figitidae)-Drosophila melanogaster interaction. Insect Biochem. Mol. Biol. 35, 93–103. doi: 10.1016/j.ibmb.2004.10.004
Lehming, N., Mcguire, S., Brickman, J. M., and Ptashne, M. (1995). Interactions of a Rel protein with its inhibitor. Proc. Natl. Acad. Sci. U.S.A. 92, 10242–10246. doi: 10.1073/pnas.92.22.10242
Lemaitre, B., and Hoffmann, J. (2007). The host defense of Drosophila melanogaster. Annu. Rev. Entomol. 25, 697–743. doi: 10.1146/annurev.immunol.25.022106.141615
Li, L. F., Xu, Z. W., Liu, N. Y., Wu, G. X., Ren, X. M., and Zhu, J. Y. (2018). Parasitism and venom of ectoparasitoid Scleroderma guani impairs host cellular immunity. Arch. Insect Biochem. Physiol. 98:e21451. doi: 10.1002/arch.21451
Livak, K. J., and Schmittgen, T. D. (2001). Analysis of relative gene expression data using real-time quantitative PCR and the 2(-Delta (Delta CT)) method. Methods 25, 402–408. doi: 10.1006/meth.2001.1262
Louradour, I., Sharma, A., Morin-Poulard, I., Letourneau, M., Vincent, A., Crozatier, M. L., et al. (2017). Reactive oxygen species-dependent Toll/NF-kB activation in the Drosophila hematopoietic niche confers resistance to wasp parasitism. eLife 6:e25496. doi: 10.7554/eLife.25496
Luo, H., Hanratty, W. P., and Dearolf, C. R. (1995). An amino acid substitution in the Drosophila hopTum-l Jak kinase causes leukemia-like hematopoietic defects. EMBO J. 14, 1412–1420. doi: 10.1002/j.1460-2075.1995.tb07127.x
Mabiala-Moundoungou, A. D., Doury, G., Eslin, P., Cherqui, A., and Prevost, G. (2010). Deadly venom of Asobara japonica parasitoid needs ovarian antidote to regulate host physiology. J. Insect Physiol. 56, 35–41. doi: 10.1016/j.jinsphys.2009.09.001
Marchiori, C. H., and Borges, L. M. F. (2017). First report of the parasitoid Pachycrepoideus vindemmiae (Rondani, 1875) (Hymenoptera: Pteromalidae) parasitizing Synthesiomyia nudiseta (Van der Wulp, 1883) (Diptera: Muscidae). Braz. J. Biol. 77, 657–658. doi: 10.1590/1519-6984.03516
Martinson, E. O., Wheeler, D., Wright, J., Mrinalini, Siebert, A. L., and Werren, J. H. (2014). Nasonia vitripennis venom causes targeted gene expression changes in its fly host. Mol. Ecol. 23, 5918–5930. doi: 10.1111/mec.12967
Morales, J., Chiu, H., Oo, T., Plaza, R., Hoskins, S., and Govind, S. (2005). Biogenesis, structure, and immune-suppressive effects of virus-like particles of a Drosophila parasitoid, Leptopilina victoriae. J. Insect Physiol. 51, 181–195. doi: 10.1016/j.jinsphys.2004.11.002
Moreau, S. J., and Asgari, S. (2015). Venom proteins from parasitoid wasps and their biological functions. Toxins 7, 2385–2412. doi: 10.3390/toxins7072385
Mortimer, N. T., Goecks, J., Kacsoh, B. Z., Mobley, J. A., Bowersock, G. J., Taylor, J., et al. (2013). Parasitoid wasp venom SERCA regulates Drosophila calcium levels and inhibits cellular immunity. Proc. Natl. Acad. Sci. U.S.A. 110, 9427–9432. doi: 10.1073/pnas.1222351110
Mrinalini, and Werren, J. H. (2017). “Parasitoid wasps and their venoms,” in Evolution of Venomous Animals and their Toxins, 1 Edn, eds P. Gopalakrishnakone and A. Malhotra (Rochester: Springer Science), 187–212. doi: 10.1007/978-94-007-6458-3_2
Myllymaki, H., and Ramet, M. (2014). JAK/STAT pathway in Drosophila immunity. Scand. J. Immunol. 79, 377–385. doi: 10.1111/sji.12170
Neyen, C., Bretscher, A. J., Binggeli, O., and Lemaitre, B. (2014). Methods to study Drosophila immunity. Methods 68, 116–128. doi: 10.1016/j.ymeth.2014.02.023
Nicolas, E., Reichhart, J. M., Hoffmann, J. A., and Lemaitre, B. (1998). In vivo regulation of the Iκb homologuecactus during the immune response of Drosophila. J. Biol. Chem. 273, 10463–10469.
Qian, C., Fang, Q., Wang, L., and Ye, G. Y. (2015). Molecular cloning and functional studies of two kazal-type serine protease inhibitors specifically expressed by Nasonia vitripennis venom apparatus. Toxins 7, 2888–2905. doi: 10.3390/toxins7082888
Rawlings, J. S., Rosler, K. M., and Harrison, D. A. (2004). The JAK/STAT signaling pathway. J. Cell. Sci. 117, 1281–1283.
Rivers, D. B., Ruggiero, L., and Hayes, M. (2002). The ectoparasitic wasp Nasonia vitripennis (Walker) (Hymenoptera: Pteromalidae) differentially affects cells mediating the immune response of its flesh fly host, Sarcophaga bullata Parker (Diptera: Sarcophagidae). J. Insect Physiol. 48, 1053–1064. doi: 10.1016/s0022-1910(02)00193-2
Rizki, T. M., and Rizki, R. M. (1992). Lamellocyte differentiation in Drosophila larvae parasitized by Leptopilina. Dev. Comp. Immunol. 16, 103–110. doi: 10.1016/0145-305x(92)90011-z
Romeo, Y., and Lemaitre, B. (2008). “Methods for monitoring the activity of Toll and Imd signaling pathways,” in Methods in Molecular Biology, eds J. Ewbank and E. Vivier (Totowa, NJ: Humana Press), 379–394.
Russo, J., Dupas, S., Frey, F., Carton, Y., and Brehelin, M. (1996). Insect immunity: early events in the encapsulation process of parasitoid (Leptopilina boulardi) eggs in resistant and susceptible strains of Drosophila. Parasitology 112, 135–142. doi: 10.1017/s0031182000065173
Schlenke, T. A., Morales, J., Govind, S., and Clark, A. G. (2007). Contrasting infection strategies in generalist and specialist wasp parasitoids of Drosophila melanogaster. PLoS Pathog. 3:e158. doi: 10.1371/journal.ppat.0030158
Schlesener, D. C. H., Wollmann, J., Pazini, J. B., Padilha, A. C., Grutzmacher, A. D., and Garcia, F. R. M. (2019). Insecticide toxicity to Drosophila suzukii (Diptera: Drosophilidae) parasitoids: Trichopria anastrephae (Hymenoptera: Diapriidae) and Pachycrepoideus vindemmiae (Hymenoptera: Pteromalidae). J. Econ. Entomol. 112, 1197–1206. doi: 10.1093/jee/toz033
Schmid, M. R. (2014). Toll-Mediated Cellular Immune Response in Drosophila Melanogaster. Ph.D. thesis, Umeå University, Umeå.
Siebert, A. L., Wheeler, D., and Werren, J. H. (2015). A new approach for investigating venom function applied to venom calreticulin in a parasitoid wasp. Toxicon 107, 304–316. doi: 10.1016/j.toxicon.2015.08.012
Sorrentino, R. P., Melk, J. P., and Govind, S. (2004). Genetic analysis of contributions of dorsal group and JAK-Stat92E pathway genes to larval hemocyte concentration and the egg encapsulation response in Drosophila. Genetics 166, 1343–1356. doi: 10.1534/genetics.166.3.1343
Stöven, S., Ando, I., Kadalayil, L., Engström, Y., and Hultmark, D. (2000). Activation of the Drosophila NF-κB factor Relish by rapid endoproteolytic cleavage. EMBO Rep. 1, 347–352. doi: 10.1093/embo-reports/kvd072
Stoven, S., Silverman, N., Junell, A., Hedengren-Olcott, M., Erturk, D., Engstrom, Y., et al. (2003). Caspase-mediated processing of the Drosophila NF-kappaB factor Relish. Proc. Natl. Acad. Sci. U.S.A. 100, 5991–5996. doi: 10.1073/pnas.1035902100
Strand, M. R., and Noda, T. (1991). Alterations in the haemocytes of Pseudoplusia includens after parasitism by Microplitis demolitor. J. Insect Physiol. 37, 839–850. doi: 10.1016/0022-1910(91)90080-j
Tang, H. (2014). Regulation and function of the melanization reaction in Drosophila. Fly 3, 105–111. doi: 10.4161/fly.3.1.7747
Tang, Q. Y., and Zhang, C. X. (2013). Data Processing System (DPS) software with experimental design, statistical analysis and data mining developed for use in entomological research. Insect Sci. 20, 254–260. doi: 10.1111/j.1744-7917.2012.01519.x
Tian, C., Wang, L., Ye, G. Y., and Zhu, S. (2010). Inhibition of melanization by a Nasonia defensin-like peptide: implications for host immune suppression. J. Insect Physiol. 56, 1857–1862. doi: 10.1016/j.jinsphys.2010.08.004
Wertheim, B., Kraaijeveld, A. R., Schuster, E., Blanc, E., Hopkins, M., Pletcher, S. D., et al. (2005). Genome-wide gene expression in response to parasitoid attack in Drosophila. Genome Biol. 6:R94.
William, P. H., and Jan, S. R. (1981). A genetic melanotic neoplasm of Drosophila melanogaster. Dev. Biol. 83, 238–249. doi: 10.1016/0012-1606(81)90470-x
Williams, M. J. (2007). Drosophila hemopoiesis and cellular immunity. J. Immunol. 178, 4711–4716. doi: 10.4049/jimmunol.178.8.4711
Woltering, S. B., Romeis, J., and Collatz, J. (2019). Influence of the rearing host on biological parameters of Trichopria drosophilae, a potential biological control agent of Drosophila suzukii. Insects 10:E183. doi: 10.3390/insects10060183
Wright, L. G., Chen, T., Thummel, C. S., and Guild, G. M. (1996). Molecular characterization of the 71E late puff in Drosophila melanogaster reveals a family of novel genes. J. Mol. Biol. 255, 387–400. doi: 10.1006/jmbi.1996.0032
Wright, V. M., Vogt, K. L., Smythe, E., and Zeidler, M. P. (2011). Differential activities of the Drosophila JAK/STAT pathway ligands Upd, Upd2 and Upd3. Cell Signal. 23, 920–927. doi: 10.1016/j.cellsig.2011.01.020
Wu, L. P., and Anderson, K. V. (1998). Regulated nuclear import of Rel proteins in the Drosophila immune response. Nature 392, 93–97. doi: 10.1038/32195
Yan, Z. C., Fang, Q., Liu, Y., Xiao, S., Yang, L., Wang, F., et al. (2017). A venom serpin splicing isoform of the endoparasitoid wasp Pteromalus puparum suppresses host prophenoloxidase cascade by forming complexes with host hemolymph proteinases. J. Biol. Chem. 292, 1038–1051. doi: 10.1074/jbc.M116.739565
Yang, H., Kronhamn, J., Ekstrom, J. O., Korkut, G. G., and Hultmark, D. (2015). JAK/STAT signaling in Drosophila muscles controls the cellular immune response against parasitoid infection. EMBO Rep. 16, 1664–1672. doi: 10.15252/embr.201540277
Keywords: Drosophila melanogaster, Pachycrepoideus vindemmiae, ectoparasitoid, venom, cellular immunity, humoral immunity
Citation: Yang L, Wan B, Wang B-B, Liu M-M, Fang Q, Song Q-S and Ye G-Y (2019) The Pupal Ectoparasitoid Pachycrepoideus vindemmiae Regulates Cellular and Humoral Immunity of Host Drosophila melanogaster. Front. Physiol. 10:1282. doi: 10.3389/fphys.2019.01282
Received: 18 June 2019; Accepted: 24 September 2019;
Published: 11 October 2019.
Edited by:
Yonggyun Kim, Andong National University, South KoreaReviewed by:
Sassan Asgari, The University of Queensland, AustraliaCopyright © 2019 Yang, Wan, Wang, Liu, Fang, Song and Ye. This is an open-access article distributed under the terms of the Creative Commons Attribution License (CC BY). The use, distribution or reproduction in other forums is permitted, provided the original author(s) and the copyright owner(s) are credited and that the original publication in this journal is cited, in accordance with accepted academic practice. No use, distribution or reproduction is permitted which does not comply with these terms.
*Correspondence: Gong-Yin Ye, Y2h1QHpqdS5lZHUuY24=
†These authors have contributed equally to this work
Disclaimer: All claims expressed in this article are solely those of the authors and do not necessarily represent those of their affiliated organizations, or those of the publisher, the editors and the reviewers. Any product that may be evaluated in this article or claim that may be made by its manufacturer is not guaranteed or endorsed by the publisher.
Research integrity at Frontiers
Learn more about the work of our research integrity team to safeguard the quality of each article we publish.