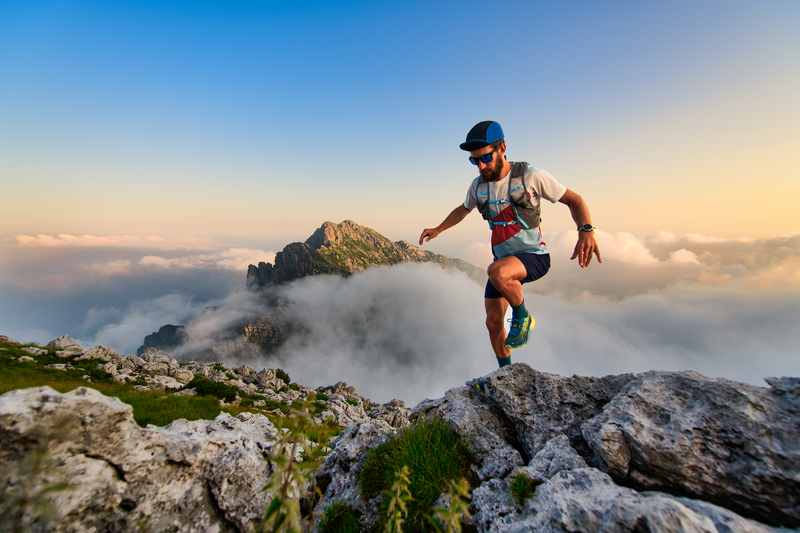
95% of researchers rate our articles as excellent or good
Learn more about the work of our research integrity team to safeguard the quality of each article we publish.
Find out more
ORIGINAL RESEARCH article
Front. Physiol. , 09 October 2019
Sec. Exercise Physiology
Volume 10 - 2019 | https://doi.org/10.3389/fphys.2019.01260
This article is part of the Research Topic Muscle and Tendon Plasticity and Interaction in Physiological and Pathological Conditions View all 27 articles
We compared the effects of aerobic high-intensity training (HIT) and isoinertial resistance training (IRT) on the strength, mass, architecture, intermuscular adipose tissue (IMAT) quality, and neuromuscular activation of the quadriceps in elderly subjects. Twelve healthy men (69.3 ± 4.2 years; 77.8 ± 10.4 kg; 1.72 ± 0.05 m) were exposed to 8 weeks of HIT (7 × 2-min cycling repetitions at 90% of O2peak, 3 times/week) and, after 4 months (detraining), to IRT (4 × 7 maximal concentric–eccentric knee extensions, 3 times/week). Before and after trainings, we measured knee extension isometric (TMVC) and dynamic (TC) maximal concentric torque, anatomical cross-sectional area (ACSA) at 25, 50, and 75% of femur length, quadriceps volume (Vol), IMAT, pennation angle (θp) of the fibers from the vastus lateralis, and voluntary activation (%Act). TMVC and TC were significantly larger only after IRT (P = 0.008); IRT was able to elicit a greater increase of ACSA than HIT; Vol increases similarly and significantly after HIT and IRT (P = 0.003–0.001); IMAT at 50% of femur length decreased after both HIT and IRT (P = 0.001–0.003); physiological cross-sectional area (PCSA) was larger after IRT than before (P = 0.025); specific torque did not change throughout the study (45.5 N cm–2 ± 12.0); %Act of the quadriceps was significantly affected only by IRT (P = 0.011). Both HIT and IRT are able to elicit beneficial modifications of muscular mass, architecture, and quality (reducing IMAT) in elderly subjects in connection with an amelioration of strength. HIT and IRT caused a homogeneous increase of ACSA and of Vol of the quadriceps. PCSA increases, but specific strength per unit of PCSA did not change. The increases of functional torque seemed to be attributed to a parallel increase of %Act and muscle hypertrophy only after IRT. Data suggest that IMAT may be a prominent indicator to track metabolic-dependent activity and skeletal muscle quality.
In elderly people, the loss of muscle mass and strength has a negative impact on their daily life autonomy, balance, and gait (Narici and Maffulli, 2010). Sarcopenia (the progressive loss of muscle mass and strength with age), however, has also functional and metabolic consequences: the progressive decrease of lean body mass is mirrored by the decay of resting metabolic rate (Narici and Maffulli, 2010), and it also implies a decrease of daily physical activities and of total energy expenditure (Vaughan et al., 1991; Goran and Poehlman, 1992). Both these factors predispose elderly people to accumulate visceral and total body fat (Kohrt and Holloszy, 1995) and to develop poor insulin sensitivity and increased post-prandial hyperglycemia.
Furthermore, also, the quality of the muscles decays in the elderly because of the substantial increase of the so-called intermuscular adipose tissue (IMAT) (Marcus et al., 2010; Zoico et al., 2010). Unlike subcutaneous adipose tissue, IMAT, which might be viewed as a peripheral ectopic fat depot, surrounds and infiltrates muscle groups with which it shares a direct vascular connection. This anatomic relationship is analogous to that of visceral liver and abdominal fat, suggesting that IMAT might have a functional negative influence on skeletal muscle metabolism analogous to that of visceral adipose tissue on liver metabolism (Durheim et al., 2008). In spite of its role, thigh IMAT has not been widely studied.
It is commonly observed that long-term heavy strength training increases anatomical cross-sectional area (ACSA) and muscle volume (Vol) mainly because of preferential Type-II fiber hypertrophy (Tesch, 1988). Yet, there seems to be a selective growth within the muscles involved in training (Housh et al., 1992; Narici et al., 1996) that may depend on the magnitude of their activation due to the load imposed by the mechanic gain of each single muscle belly (Folland and Williams, 2007). However, the data are not completely consistent, since it has not been clarified whether selective hypertrophy is more pronounced, for instance, in the region of the maximal ACSA of the quadriceps (Häkkinen et al., 2000) or in the more distal and proximal regions of the muscles (Narici et al., 1996), also as a consequence of different training modalities and, therefore, regional loads. Differential adaptations are likely to alter the moment of inertia of the thigh and the functional consequences (Earp et al., 2015). The architectural changes must be properly taken into account when we aim to estimate the possible changes of specific tension elicited by training, since force must be normalized by the so-called physiological cross-sectional area (PCSA) and not by the ACSA. In this regard, it is not clear whether specific torque (i.e., the torque per unit of PCSA) may change following resistance training. In addition, any decrease in IMAT content brought about by training would, however, modify the specific strength calculated as the ratio between torque and ACSA.
In the elderly, the progressive loss of muscle mass and strength (sarcopenia) is associated with metabolic alterations. Furthermore, the peripheral changes that compromise gas exchanges contribute to the progressive decrease in the maximum cardiovascular oxygen transport observed in aging. It is therefore ecological to propose strength training. It is useful to counteract the loss of muscle mass by promoting or enhancing the beneficial adaptations induced also by aerobic training on the main risk factors leading to metabolic syndrome. Even though traditional calisthenic workout (whole-body exercises, 3 sets of 10–15 reps, 3 days/week) has been described to improve health-related parameters as biomarkers (Tomeleri et al., 2016) and body fat (Cunha et al., 2017), resistance training is mainly prescribed in elderly people to promote the increase of muscle strength and mass (Hurley et al., 1994; Hunter et al., 2004). Since resistance training is highly effective when concentric and eccentric contractions are repeatedly applied (Berg and Tesch, 1994; Franchi et al., 2017), the so-called isoinertial resistance training (IRT) performed with the YoYo® ergometer, which implements the flywheel principle and is able to generate resistance force during both the lengthening and shortening actions of the contraction thanks to eccentric overload (Tesch et al., 2017), seems to be a promising and effective way to induce a fast increase of muscle mass and strength also in elderly people (Maroto-Izquierdo et al., 2017).
The improvement in muscle strength following resistance training is also the result of beneficial neuromuscular adaptions. The main neurological adaptations, which seem to establish already in the early phases of training, include a decrease of the activation of the antagonist muscles and enhanced agonist muscle activation due to increased motor recruitment, firing frequency, and synchronization of the motor units (Gabriel et al., 2006; Folland and Williams, 2007). By using the interpolated twitch technique to measure the level of muscular activation during maximal, voluntary isometric contraction (Shield and Zhou, 2004), several studies have indeed shown that increased muscle activation follows strength training (Shield and Zhou, 2004). Even if a high-intensity interval training (HIT) program induced beneficial muscular neural adaptations in young adults (Vera-Ibañez et al., 2017), no evidence exists of any amelioration in muscle activation in the elderly population.
HIT has gained popularity as a safe and efficient exercise method with the potential to influence several health-related parameters: in various populations, HIT has been shown not only to increase maximal oxygen consumption (O2max) but also to decrease fat mass and increase insulin sensitivity (Gibala et al., 2006; Bruseghini et al., 2015). Moreover, it has been observed that HIT can lead to an increase of skeletal muscle oxidative and buffering capacity, muscle protein synthesis, and mitochondrial biogenesis (Scalzo et al., 2014). Despite the aforementioned data that suggest a potential positive impact of HIT on muscle remodeling and growth, there are limited data on the effect of HIT on muscle mass, strength, architecture, and quality in elderly (Astorino et al., 2011). HIT has been described to contrast the progressive loss of muscle mass (Harber et al., 2012) and 8 weeks of HIT were followed by the decrease of the percentage of adipose tissue and by the hypertrophic adaptation of the muscle involved in training (Bruseghini et al., 2015). If HIT induces also a substantial decrease of IMAT, this would result in a substantial improvement of muscle quality and function (Addison et al., 2014). In this regard, no clear data exist on the efficacy of HIT to reduce IMAT in elderly, active, healthy men, even though it has been shown that moderate physical activity (e.g., walking) was able to prevent the increase of IMAT in older subjects (Goodpaster et al., 2008).
On the basis of all these premises, we tested the hypothesis that muscular mass-volume, quality, morphology, and function can be increased/improved by both types of training intervention. Therefore, we evaluated the effects of 8 weeks of HIT and IRT on muscle quality, morphology, strength, and neuromuscular activation of healthy, elderly men. Also, we test the hypothesis that structural adaptations would differ between training modalities because of the differences in muscular and regional activation. The results would help to obtain a deeper insight into the specific effectiveness of these two types of training on muscular quality, morphology, and function in elderly subjects considering that these features have a substantial impact on the metabolic and locomotor roles of the skeletal muscle.
Therefore, we aimed to assess (i) whether HIT elicited any substantial increase of muscle strength and mass; (ii) whether muscle hypertrophy elicited by training was selectively more pronounced in specific areas of the quadriceps; (iii) if HIT and IRT were able to induce significant amelioration of the quality of the muscles and, in turn, modification of the strength-to-ACSA ratio; (iv) whether muscle morphology was substantially modified by the two training interventions; and (v) the relative contribution of muscle hypertrophy and muscular increased activation in eliciting the observed increments of strength.
Twelve moderately active Caucasian men (age: 69.3 ± 4.2 years, range, 65–75; body weight: 77.8 ± 10.4 kg; height: 1.72 ± 0.05 m; BMI: 26.5 ± 2.8 kg m–2; IPAQ score: 4333 ± 1750 MET min week–1) were recruited through local advertisements in the Verona, Italy, metropolitan area and volunteered to participate in the study. Main physiological and health-related outcomes (e.g., O2max, body composition) have been previously published for this sample of participants (Bruseghini et al., 2015; Tam et al., 2018). All the subjects had filled in the IPAQ questionnaire and then they underwent a preliminary medical examination to evaluate exclusion criteria [abnormal EKG at rest and during exercise, uncontrolled hypertension, diagnosis of cardiovascular, respiratory and metabolic diseases, moderate-severe renal failure, neurological and orthopedic diseases limiting mobility and exercise, anti-coagulants and anti-aggregant therapy, alcohol and drug abuse and common contraindications to MRI (i.e., pacemakers, metallic clips)] and pathological responses to exercise. The study was conducted in accordance with ethical standards, the provisions of the Declaration of Helsinki, and national and international guidelines. The protocol and the methods of the study were approved by the Regional Review Board (approval on June 18, 2013), and written informed consent was obtained from each subject before entering the study.
A two-factor within-subject design was planned in which each subject received all the combinations of treatment that originated by crossing the two factors: one fixed factor was training modality (two levels, HIT and IRT); the second fixed factor was time (two levels, Pre- and Post-training) in which all subjects were exposed to all the two conditions (Keppel and Wickens, 2004). All the subjects were evaluated four times: before training for baseline values (Pre-HIT) and immediately after 8 weeks of HIT (Post-HIT), after 12 weeks of recovery before IRT (Pre-IRT), and, finally, after 8 weeks of resistance training (Post-IRT). Before the first data collection, a familiarization session was conducted, during which the experimental procedures were thoroughly explained and a simplified version of them was carried out.
During each experimental session, the tests were performed in the morning, at the same time of the day and in the environmentally controlled conditions, on three consecutive days: on the first day, the main anthropometric measurements were carried out and the O2max of each subject was measured. The second day was devoted to functional strength test and ultrasound scan acquisition. The third day was dedicated to MRI scans. Twenty-four hours before the tests, participants abstained from strenuous physical activity and alcohol and caffeine consumption.
The subjects were asked to perform each supervised training session at the same time of the day on alternate days. Compliance to training was high with subjects in each training period completing all of the exercise sessions. No injuries or health disorders were reported during the exercise program, and no modification in the planned protocol had to be introduced. Between and during HIT and IRT sessions, the subjects were asked to maintain their habitual lifestyle: to evaluate physical activity, all subjects wore a portable monitor SenseWear Armband Mini (BodyMedia, Inc., Pittsburgh, United States) continuously for a 1-week period (Mackey et al., 2011). This has been done 1 month before each training period and during both training periods.
Volunteers trained three times a week for 8 weeks. Training consisted of seven 2-min bouts of cycling exercise (915 E, Monark, Varberg, Sweden) at 85–95% of individual O2max interspersed by 2-min recovery intervals at about 40% of O2max (Buchheit and Laursen, 2013a, b). Each series was preceded by 10 min of active warm-up. The entire supervised training session lasted from 45 to 50 min, including the post-training cooling-down phase. The mechanical workloads related to the percentage of O2max were calculated using the individual oxygen consumption/load ratio of the warm-up before the incremental test and created using the oxygen consumption values measured in the last minute of each load. Heart rate/load (HR/W) ratio was also computed in order to control responses to exercise and to adjust workloads every 14 days, according to changes in the HR/W relationship assessed during three submaximal workloads at steady state.
Resistance exercise was performed on a seated knee extension flywheel ergometer (Berg and Tesch, 1994) (YoYo Technology AB, Stockholm, Sweden) 3 times a week for 8 weeks. Each supervised session consisted of four sets of seven maximal, coupled concentric extensions and eccentric flexions of the knee from about 90° to 160°–170° knee joint angle. Subjects received verbal encouragement to push as harder as they could and direct feedback was provided during exercise by shoving force production. The increase in the maximum force applied during each training session has made it possible to enhance and adapt the workload constantly during the 8 weeks of training. The sets were interspersed by 3-min rest periods and initiated immediately after performing two submaximal actions. Each exercise session was preceded by 10 min of active warm-up, including three sets of seven submaximal actions with progressively increased effort. Training was performed using a polymer flywheel (4.2 kg). Each exercise session, including warm-up and rest periods, was completed in about 30 min.
Body weight and stature were measured and BMI was also calculated. O2max was measured using a metabolic cart (Quark b2, Cosmed, Rome, Italy) at the end of incremental ramp tests to exhaustion on a cycle ergometer (Excalibur Sport, Lode, Groningen, Netherlands), as described in detail previously (Bruseghini et al., 2015).
To determine the volumes and ACSA of the total quadriceps femoris (QF), rectus femoris (RF), vastus lateralis (VL), vastus intermedius (VI), and vastus medialis (VM), MRI scans in a 1.5-T GE scanner (General Electric, Milwaukee, WI) were obtained following the protocol described by Trappe et al. (2001). Briefly, a coronal scout scan [repetition time/echo time (TR/TE) 5 300/14 ms, field of view 48 cm, 256 × 160 matrix] of five slices 5 cm thick with 5-mm spacing was completed to establish orientation of the femur. Then, interleaved transaxial images of 1 cm thick (TR/TE 633/20 ms, field of view 274 × 480 mm, 256 × 256 matrix) were obtained along the entire length of the femur. The procedure has already been discussed previously (Bruseghini et al., 2015) and only the most salient details will be explained here. Analyses of the magnetic resonance images of dominant limb began with the first proximal slice not containing gluteal muscle and continued distally to the last slice containing RF (Castro et al., 1999). The average ACSA (cm2) was taken as the average of all the analyzed slices for an individual muscle and determined for the RF, VL, VI, and VM and summed for the total QF. ACSA was drawn manually in correspondence of slice obtained at 75, 50, and 25% of the length between the greater trochanter to the upper border of the patella (LF). The volume of muscle tissue per slice was calculated by multiplying the ACSA area by the inter-slice distance. The volumes of each of the QF components were calculated as the sum of all corresponding slice volumes. The volume of the quadriceps (Vol) was then computed as the sum of the single muscular volume.
MRI scans of dominant limb at 75, 50, and 25% femur length were examined to determine IMAT and subcutaneous adipose tissue using SliceOmatic image analysis software (version 4.2; TomoVision, Montreal, Quebec, Canada). IMAT was defined as adipose tissue area visible between quadriceps muscle groups. The gray-level intensity (threshold value) of the adipose tissue in the subcutaneous adipose tissue region was determined. This value was reduced by 20% to identify the quadriceps IMAT threshold (Rossi et al., 2010).
Isometric and dynamic strength produced by the knee extensors of the dominant limb was evaluated with an isometric–isokinetic dynamometer (CMSi Cybex Humac Norm Dynamometer, Stoughton, MA, United States) at 90° of knee angle during maximal voluntary contraction in isometric condition (TMVC) and during concentric (TC) isokinetic contractions at an angular velocity of 120° s–1. Before the strength test, the subjects completed 10 min of warm-up exercise on a stationary bike, and they performed several practice trials while sitting on the reclining chair of dynamometer. The lower part of the leg was strapped to the end of the lever arm of the dynamometer and the center of rotation of the knee was aligned with the axis of the dynamometer. Three maximal trials (30 s of rest was provided between each trials) were performed for each condition with 3 min of recovery between each condition (Connelly and Vandervoort, 2000; Power et al., 2013). Visual feedback was provided to participants and verbal encouragement was standardized throughout both testing protocols (McNair et al., 1996). The highest torque values were recorded for further analysis.
Real-time B-mode ultrasonography (ACUSON P50 ultrasound system, 12L5 linear probe) was used to measure fascicle pennation angle (θp) and fascicle length (Lf) of the VL. Participants were sitting with the knee angle fixed at 90° (Raj et al., 2012). Images were obtained at mid-belly of the dominant VL muscle by using a linear-array probe. The probe was positioned perpendicular to the dermal surface of the VL muscle and oriented along the median longitudinal plane of the muscle. Mid-belly was defined as the point along the median longitudinal axis of the muscle at 50% of the distance between the proximal and distal apexes of the myotendinous junctions. The center of the probe was aligned to this position. The probe was coated with a water-soluble transmission gel to provide acoustic contact without depressing the dermal surface. Three images at rest were obtained within the same experimental session in each individual (Narici et al., 2003). Scans were analyzed with an open source software OsiriX (Pixmeo, Geneva, Switzerland). VL muscle thickness was defined as the distance between the superficial and deep aponeurosis. θp was measured as the angle between the muscle fascicles and the deep aponeurosis. Lf was measured as the length of a fascicle between its insertions at the superficial and deep aponeurosis. Where the fascicles extended beyond the recorded image, Lf was estimated from muscle thickness (Tm) and θp using the following equation (Raj et al., 2012):
Then, the PCSA of the quadriceps (PCSA) was calculated as follows (Gans, 1982):
θp and Lf measured on the VL were assumed to be representative of the mean θp and Lf of the entire quadriceps (Erskine et al., 2009). Quadriceps specific tension (Ts) was subsequently calculated using the following formula:
where TMVCcorr is the tension in N calculated from TMVC at 90° of knee flexion corrected by the patellar tendon moment arm length obtained from the literature (Smidt, 1973; Narici et al., 1992).
To determine the level of voluntary muscle activation and contractile proprieties of the dominant quadriceps muscle and biceps muscle of the arm (control condition), the interpolated twitch technique was used (Shield and Zhou, 2004). During experiments on lower limb, the participants sat in a standardized position with hip and knee at 90° of flexion, on a customized testing system’s chair and tightly secured to it with hip and torso straps. During experiments on upper limb, the elbow of the subjects was accommodated in a standardized position on a customized dynamometer placed on a table; the dominant forearm was then positioned vertically and connected to the load cell rigidly attached to the customized testing system. Electrical stimulation was administered via two 5 cm × 10 cm self-adhesive electrodes, placed distally (anode) and proximally (cathode) over the quadriceps (Kufel et al., 2002). The quadriceps and the biceps brachii were stimulated in a relaxed state with 50-mA pulses of 100 μs, which were increased in 30-mA increments (Digitimer High Voltage Stimulator model DS7A, Digitimer Ltd., Welwyn Garden City) until no further increase in twitch force was observed. This current was used 2 min later to elicit a single twitch during three maximal voluntary contractions (MVC) lasting 5 s each and a second twitch in the resting state 5 s after the MVC.
Force was measured by means of a calibrated load cell (DBBE, Applied Measurements Ltd., Aldermaston, Reading, United Kingdom) connected to a non-compliant strap that was placed around the subject’s dominant leg just superior to the ankle malleoli and around the forearm close to the wrist of the dominant arm. Torque signals and electrical stimuli collected with the help of PowerLab data acquisition (PowerLab 16/35 AD Instruments Ltd., Australia) at a sampling frequency of 1 kHz and analyzed by Labchart 6.0 software (AD Instruments Ltd., Australia).
Voluntary activation of the stimulated muscles (%Act) was quantified applying the following equation:
where the superimposed twitch is the force increment noted during a maximal voluntary contraction at the time of stimulation and the control twitch is that evoked in the relaxed muscle (Shield and Zhou, 2004).
All values in the text are presented as mean ± standard deviation. Sample size was determined using G∗Power software (ver 3.1.9.2) (Faul et al., 2007) to ensure there was sufficient power (1–β = 0.80) to detect significant differences within factors. Normality of data distribution was evaluated by means of the Shapiro–Wilk test (StatPlus: mac Version v6, AnalystSoft, CA, United States). When criteria for normality were not met, inferential analysis was always performed on the log-transformed data. Overall analysis of the data was carried out according to Keppel and Wickens (2004) for two-fixed factors within-subjects design with Training type (HIT and IRT) and Time (Pre and Post) as fixed. In particular, (i) F values were calculated taking into account the possible violation of sphericity by using the correction of the degree of freedom, as suggested by Geisser and Greenhouse; (ii) effect size in terms of population variability was evaluated by computing ω2, which expresses the variability of the effect over the sum of that variability and the error variability and total variability that affects it; (iii) single planned contrasts time (Pre vs. Post) and Training modalities, HIT vs. IRT were evaluated; (iv) effect size (d) of the differences between the contrasted values was also calculated. Calculations were carried out using an Excel spreadsheet (MO 2010, Microsoft Corp. Seattle, WA, United States) programed for this purpose.
The differences between the percent increase of the cross-sectional areas of the quadriceps between Pre- and Post-interventions were evaluated by using a 2-ANOVA analysis for repeated measurements; post-hoc analysis was carried out between families of pairwise comparisons by using the Šidák–Bonferroni procedure to correct for the family-wise Type I error. Multiple linear regressions between a dependent variable and two independent explanatory variables were calculated by using least squares approach (Motulsky and Christopoulos, 2004).
Correlation analyses were conducted on ACSA, IMAT, %Act, with Pearson’s product–moment correlation, and correlation coefficients (r) were classified as small (0.1 < r ≤ 0.3), moderate (0.3 < r ≤ 0.5), high (0.5 < r ≤ 0.7), very high (0.7 < r ≤ 0.9), and almost perfect (r > 0.9) (Hopkins et al., 2009).
The results have been reported synthetically (mean ± SD) in Supplementary Table S1 for greater clarity and commented in the following sessions.
HIT was followed by a significant increase of ACSA at all the three femur lengths [at 25%: plus 3.09 cm2 ± 1.38; (P = 0.001; 95% CI of diff: 2.21–4.0; d: 2.24); at 50%: plus 2.27 cm2 ± 2.52 (P = 0.010; 95% CI of diff: 0.67–3.87; d: 0.90); at 75%: plus 2.65 cm2 ± 3.04 (P = 0.011; 95% CI of diff: 0.72–4.58; d: 0.87)] (Figure 1).
Figure 1. Anatomical cross-sectional area (ACSA, cm2) of the quadriceps assessed before (filled symbols) and after (empty symbols) high-intensity interval training (HIT, A) and isoinertial resistance training (IRT, B) at 75, 50, and 25% of the length between the greater trochanter to the upper border of the patella (LF) (∗P < 0.05; ∗∗P < 0.01).
Also, IRT was followed by a significant increase of ACSA at all the three evaluated % of femur length [at 25%: plus 3.19 cm2 ± 1.24; (P = 0.001; 95% CI of diff: 2.40–3.99; d: 2.57); at 50%: plus 3.03 cm2 ± 3.04 (P = 0.005; 95% CI of diff: 1.10–4.96; d: 1.00); at 75%: plus 3.40 cm2 ± 3.21 (P = 0.004; 95% CI of diff: 1.36–5.44; d: 1.06)] (Figure 1).
ANOVA analysis revealed a significant effect of factor Time on the increase of ACSA at 25% of femur length (F = 0.001; ω2 = 0.59), 50% of LF (F = 0.001; ω2 = 0.64), and 75% (F = 0.002; ω2 = 0.25). At 25% and at 75% of femur length, also an effect of factor Training was present (F = 0.028; ω2 = 0.10): at 25%, ACSA at Post-IRT was larger than at Post-HIT (P = 0.024; d = 0.753); at 75%, ACSA at Post-IRT was larger at Post-HIT (P = 0.008; d = 0.929) than at Pre-HIT (P = 0.011; d = 1.291). In none of the cases, however, significant interactions between factors were present.
The highest quadriceps ACSA was found at 50% of femur length. However, for both trainings, the percent increases of quadriceps ACSA at the 3% of femur length were rather homogeneous and they were not significantly different. The percent increases of quadriceps ACSA at the 3% of femur length were not different between HIT and IRT.
Finally, the percent increase of quadriceps ACSA observed after trainings at the three levels of femur length was negatively and moderately related with the absolute area at Pre-HIT and Pre-IRT: r = -0.37; P = 0.001; n = 72.
ANOVA analysis revealed a significant effect of time on the increase of Vol (F = 0.001; ω2 = 0.848). Vol was significantly larger at Post-HIT than at Pre-HIT: plus 42.2 cm3 ± 38.3 (P = 0.003; d = 1.11), and at Post-IRT than at Pre-IRT: plus 68.2 cm3 ± 38.3 (P = 0.001; d = 1.40) (Figure 2).
Figure 2. (A) Box and whiskers plot of the volumes of the quadriceps (Vol, cm3) before and after HIT and IRT. Asterisks indicate the significant difference between Pre- and Post-conditions (∗∗P < 0.01). (B) Box and whiskers plots of the percent increases (Vol, %) of the entire quadriceps and of each of its belly observed during HIT and IRT. The box extends from the 25th to 75th percentiles. The line in the middle of the box is plotted at the median and “+” at the mean. Whiskers range from a Min to Max value.
The percent increase of the total Vol of the quadriceps was not significantly different between HIT and IRT.
ANOVA analysis on the data of IMAT at 50% of femur length was performed after log transformation of the data as they failed the test for assessing normal distribution. The analysis revealed a significant effect of time (F = 0.001; ω2 = 0.78) and of training (F = 0.001; ω2 = 0.66).
There was a progressive decrease of the IMAT area during the study. In particular, IMAT was significantly lower at Post-HIT than at Pre-HIT (P = 0.001; d = 1.57). As the analysis was performed on log-transformed data, the 95% CI of the ratio between the Pre-HIT and Post-HIT were calculated: 0.70–1.15. IMAT significantly decreased also after IRT in respect to Pre-IRT (P = 0.003; d = 1.08), the 95% CI of the ratio being equal to 0.51–1.49. IMAT after the strength training was also significantly smaller than at Post-HIT (P = 0.001; d = 1.64) with 95% CI of the ratio equal to 0.42–1.40) (Figure 3).
Figure 3. (A) Box and whiskers plots of intermuscular adipose tissue (IMAT, cm2). (B) Box and whiskers plots of ACSA (cm2) measured at 50% of the length between the greater trochanter to the upper border of the patella (% LF) excluding IMAT. Lines and asterisks indicate the significant differences between the mean values (∗∗P < 0.01). The box extends from the 25th to 75th percentiles. The line in the middle of the box is plotted at the median and “+” at the mean. Whiskers range from a Min to Max value.
Also, for subcutaneous adipose tissue at 50% of femur length, it was possible to show an effect of time (F = 0.001; ω2 = 0.63): subcutaneous adipose area significantly decreased after HIT in respect to the initial control condition (P = 0.001; d = 1.31, 95% CI of diff: –5.97 to –7.73). An effect of the type of training was also evident (F = 0.001; ω2 = 0.66). In addition, significant interactions between time and training effects were also demonstrated (F = 0.003; ω2 = 0.56), as the differences between Pre-HIT and Post-HIT values were significantly different from those observed before and after IRT (P = 0.010; d = 0.99).
The absolute and percent changes of ACSA at 50% of femur length were also calculated net of IMAT contribution. The subtraction of IMAT, however, did not alter the pattern of the changes observed after the two training interventions: ACSA at Post-HIT was significantly larger than at Pre-HIT: plus 3.95 cm2 ± 3.17 (P = 0.001; d: 1.25; 95% CI of diff: 1.93–5.96) and at Post-IRT than at Post-IRT: plus 4.47 cm2 ± 2.63 (P = 0.001; d: 1.697; 95% CI of diff: 2.79–6.14). Interestingly, the percent increases of total ACSA neglecting IMAT were significantly larger than the ones measured including IMAT both for HIT (7.71% ± 6.21 vs. 4.00% ± 4.29; P = 0.001, 95% CI of discrepancy from 0: 2.1–5.3) and for IRT (8.38% ± 5.47 vs. 5.65% ± 6.14; P = 0.006, 95% CI of discrepancy from 0: 0.97–4.48) (Figure 3).
For the sake of completeness, also the analysis of IMAT at 25% and at 75% of femur length was carried out after log transformation of the data. The data generally confirmed the ones obtained at 50%: the analysis revealed a significant effect of time (F = 0.001; ω2 = 0.70) at 25% of femur length. In details, IMAT was significantly lower at Post-HIT than at Pre-HIT (P = 0.003; d = 1.12) and at Post-IRT than at Pre-IRT (P = 0.008; d = 0.92). At 75% of femur length, however, IMAT turned out to be significantly lower only after IRT than at Pre-IRT (P = 0.001; d = 1.244).
Finally, the net decrement of IMAT observed in the two training interventions was highly correlated (r = –0.71) with the initial absolute value of IMAT.
Only IRT training was followed by a significant increase of TMVC measured at 90° of knee joint flexion: plus 11.5 N m ± 17.1 (P = 0.040; d = 0.67): the percent of increase amounted to 7.0% ± 9.8 (Figure 4).
Figure 4. Box and whiskers plots of isometric torque at 90° of knee flection (A) and isokinetic concentric torque (TC, N × m) at 120° s–1 of angular velocity (B). Lines and asterisks indicate the significant differences between the mean values (∗P < 0.05; ∗∗P < 0.01). The box extends from the 25th to 75th percentiles. The line in the middle of the box is plotted at the median and “+” at the mean. Whiskers range from a Min to Max value.
Training significantly affected TC at 120° s–1 (F = 0.014; ω2 = 0.137), but isokinetic strength was only significantly larger after IRT than before IRT: plus 8.8 N m ± 13.0 (P = 0.008; d = 0.93). For strength values, the baseline conditions Pre-HIT are comparable to baseline conditions Pre-IRT (Figure 4).
Percent increases of TC were not linearly related with the corresponding initial torque values (P between 0.050 and 0.705). They ranged, on average, from 2.44 to 10.4% (grand mean at 120° s–1: 6.6% ± 11.3).
ANOVA analysis revealed a significant effect of time (F = 0.019; ω2 = 0.410) and of training (F = 0.001; ω2 = 0.119) on θp, which turned out to be significantly greater after HIT than before (P = 0.001; d = 1.93) and after IRT than before strength training (P = 0.004; d = 1.03) (Figure 5).
Figure 5. Pennation angle (θp, °) of the fibers of vastus lateralis (A) and physiological cross-sectional area (PCSA) of quadriceps in cm2 (B) assessed before (Pre) and after (Post) HIT and IRT. Lines and asterisks indicate the significant differences between the mean values (∗P < 0.05; ∗∗P < 0.01). The box extends from the 25th to 75th percentiles. The line in the middle of the box is plotted at the median and “+” at the mean. Whiskers range from a Min to Max value.
PCSA at 50% of femur length was significantly affected by training (F = 0.041; ω2 = 0.083), but was only larger at Post-IRT than at Pre-IRT (P = 0.025; d = 0.741) (Figure 5).
However, when specific isometric strength was calculated as the ratio between strength and ACSA, we obtained a different pattern if we corrected or did not correct ACSA for IMAT (Figure 6). When IMAT is included, torque per square centimeter of ACSA did not change during the study, and its grand average amounted to 59.8 N cm–2 ± 7.1, 95% CI 61.8–57.7. When ACSA was corrected for the contribution of IMAT, torque per squared cm of ACSA at Post-HIT (60.8 N cm–2 ± 7.5) was smaller than at Pre-HIT (66.4 N cm–2 ± 6.1), P = 0.007, 95% CI of the difference from 0: 1.9–9.3 N cm–2. Conversely, it did not change from Pre-IRT (63.8 N cm–2 ± 5.6) to Post-IRT (63.0 N cm–2 ± 9.1). Finally, specific strength measured as the ratio between TMVC and PCSA remained constant during the study, and its grand average amounted to 45.5 N cm–2 ± 12.0, 95% CI 49.0–42.0.
Figure 6. Box and whiskers plots of specific isometric strength (90° of knee flection) per unit of area of ACSA (A) and PCSA (B) in N cm–2. Lines and asterisks indicate the significant differences between the mean values (∗∗P < 0.01). The box extends from the 25th to 75th percentiles. The line in the middle of the box is plotted at the median and “+” at the mean. Whiskers range from a Min to Max value.
ANOVA analysis for the two fixed effects demonstrated that %Act of the quadriceps was affected by training (F = 0.019; ω2 = 0.119), being larger at Post-IRT than at Pre-IRT (P = 0.011; d = 0.897) (Figure 7). Arm activation remained identical throughout the study.
Figure 7. Box and whiskers plots of percent of voluntary activation (%Act, %) of the lower limb extensors assessed before (Pre) and after (Post) HIT and IRT. Lines and asterisks indicate the significant differences between the mean values (∗P < 0.05). The box extends from the 25th to 75th percentiles. The line in the middle of the box is plotted at the median and “+” at the mean. Whiskers range from a Min to Max value.
Moreover, %Act of the quadriceps was negatively correlated with the absolute levels of IMAT both before (r = 0.21, small correlation) and after (training (r = 0.53, high correlation).
The percent increases of isokinetic and isometric torque selected as explanatory variables (Y) were fitted to a multiple linear regression with the percent increases of ACSA at 50% of femur length (X1) and of %Act (X2) as predictive variables for both HIT and IRT conditions, forcing the functions to pass through the origin of the axis:
where Y, X1, and X2 represent the percent increases of torque, ACSA, and %Act, respectively.
However, in HIT, only the percent of increase of ACSA significantly predicted the increase of strength (t = 2.25; P = 0.03); in IRT, both percent of increases of ACSA (t = 3.03; P = 0.004) and %Act (t = 2.031; P = 0.048) significantly predicted the percent increase of Torque.
The purpose of this investigation was to compare the effects of HIT and IRT on muscular strength, mass, morphology, muscle quality, i.e., IMAT, and neuromuscular activation in a group of active, healthy elderly subjects.
The main findings showed that:
i) Tc and TMVC turned out to be significantly larger only after IRT; ACSA of quadriceps significantly increased both after HIT and after IRT at three evaluated femur lengths; the efficacy of training was also confirmed by the analysis of the changes of Vol;
ii) IMAT at 50% of femur length decreased after both trainings, in particular after IRT, and moreover, although the removal of IMAT did not change the pattern of the percent change of ACSA after trainings, it turned out to be larger than the ones calculated including IMAT area;
iii) θp increased both after HIT and IRT; however, PCSA turned out to be larger only after IRT; the changes in PCSA and in torque resulted in constant value of specific strength per unit of PCSA;
iv) %Act of the quadriceps was only greater after the IRT and the increments of strength observed after IRT seem to be predicted by the consensual increases of ACSA and %Act.
Therefore, both HIT and IRT seemed to be able to induce significant and remarkable changes in muscle mass, morphology, and quality, but strength turned out to be significantly and positively affected only by IRT. The overall analysis of physical activity during the intervention period shows that subjects were able to maintain the same lifestyle, and they have not changed their total daily energy expenditure during the two training programs (before HIT: 2318 ± 315 kcal/day; during HIT: 2255 ± 314 kcal/day; before IRT: 2253 ± 243 kcal/day; during IRT: 2344 ± 220 kcal/day).
Both HIT and IRT were able to induce muscle hypertrophy, since ACSA increased at all the three femur lengths, as shown in Figure 1, and Vol was augmented after the two training interventions (Figure 2). The findings after HIT are in agreement with the ones reported by other investigators (Harber et al., 2012; Estes et al., 2017) who showed a significant increase of Vol in older and young men after moderate-to-vigorous aerobic training. The percent increase reported in those studies is of the same order of magnitude as the one found in the present investigation, i.e., 5.5% ± 4.6. Harber et al. (2012) attributed the observed hypertrophy to the specific increase of ACSA of MHC Type 1 fibers.
Strength training has been shown to induce remarkable increases of muscle mass in elderly subjects (Cadore et al., 2014) and 10 to 12 weeks of heavy resistance training resulted in increase of ACSA of the quadriceps ranging from 6% (Kraemer et al., 1999) to 11–14% (Häkkinen et al., 2000). In the present study, the average percent increase of ACSA at 50% of LF was 5.7% ± 6.4, a value that might be explained by the shorter duration of the intervention and by the different training protocols applied in the studies. In this respect, IRT with eccentric overload has been claimed to be more effective and capable to induce larger gains in muscle mass and strength (Maroto-Izquierdo et al., 2017) than traditional weight lifting. However, a recent review (Vincens-Bordas et al., 2018) has refuted this conclusion, highlighting that the available data do not allow us to conclude that IRT is superior to traditional weight lifting and gravity-dependent strength training in increasing muscle mass and strength. Yet, it is worth noting that the majority of the available data were collected on young or adult men and women and conclusive data concerning elderly individuals on the comparison of the two training modalities are scarce.
The largest ACSA was found at 50% of femur length in agreement with the findings of Narici et al. (1996). However, in contrast with their findings, and with the ones of Mangine et al. (2018), the increase of ACSA found after the two trainings was uniform at the three levels of femur length. The discrepancy between the data obtained in this investigation and the ones reported in the quoted paper deserves, of course, a comment. First, our study concerned elderly subjects, whereas others investigated adult young volunteers. We know that different increases of ACSA have been found between young and elderly subjects (Kraemer et al., 1999), although this has not been consistently confirmed (Cadore et al., 2014). Therefore, we may speculate that the less pronounced response of the elderly subjects to the training stimulus may have somehow blunted and made less evident the selective growth of the four bellies of the quadriceps. Secondly, IRT, with its eccentric overload, may lead to a massive activation of all the bellies of the quadriceps, thus overriding the limitation of the gravity-dependent training that may induce activations of different magnitude as a consequence of the different loads imposed to each singular muscle by their mechanical gains (Folland and Williams, 2007).
Muscular morphological adaptations are also paralleled by substantial changes in muscle architecture. There is a general agreement that the pennation angle (θp) of the muscle fibers increases with hypertrophy (Kawakami et al., 1993; Folland and Williams, 2007). The increase of θp would allow a larger packing of fibers for the same ACSA and lead to the increase of the PCSA, i.e., the area perpendicular to the line of application of the force produced by the fibers. However, an increase of θp would bring about a decrease of the force applied to the tendon because the angle between the fibers and the line along which the force is projected decreases. Yet, it can be geometrically demonstrated that if θp stays below 45°, its increase is compensated by the increase of PCSA so that an augmentation of force results after training (Alexander and Vernon, 1975). Indeed, it has been shown that an increase of θp from 8.0° to 10.7° (+36%) increased PCSA and force (+16%) more than ACSA (+10%) (Aagard et al., 2001).
Many physiological/pathological conditions (e.g., aging, sedentary lifestyle, but also the augmentation of inflammatory cytokines, reduced anabolic hormonal response, and general metabolic disorders) lead to muscle deconditioning, a phenomenon characterized by a loss of muscle strength and power and an increase of fatty infiltration. Data suggest that IMAT may be a prominent indicator to track metabolic-dependent activity and skeletal muscle quality. The IMAT increase and accumulation are linked to muscle dysfunction and is largely attributable to inactivity, but it is also associated with increasing age (Marcus et al., 2010). IMAT negatively affects muscle quality and muscle function by decreasing absolute and specific strength levels leading to muscle weakness. The latter have been associated with high levels of IMAT in adults with other comorbidities (Marcus et al., 2010) and/or characterized by low levels of physical activity (Addison et al., 2014).
The efficacy of resistance training to decrease IMAT in adult and elderly subjects has already been documented (Nicklas et al., 2015), although the intensity of the training remains crucial: no decrease in thigh IMAT occurs if the eccentric intervention is carried out at submaximal intensity (Jacobs et al., 2014). Less clear and definitive data exist on the efficacy of endurance training, let alone HIT, to abate IMAT infiltration in healthy elderly subjects. The benefits of low-intensity endurance training have been evaluated (Ikenaga et al., 2017); yet, the effectiveness of HIT is still unknown. Notwithstanding that the present study has been conducted on a small number of subjects, the data suggest that even short periods of high-intensity aerobic training may be effective to reduce IMAT in elderly healthy subjects.
The improvement in the muscle quality was also highlighted by finding that the percent increases of ACSA obtained when IMAT was neglected were significantly larger than the ones obtained when IMAT was included in the planimetric calculation of ACSA.
It has been suggested that only individuals with a low infiltration of IMAT are able to significantly ameliorate their muscle quality (Marcus et al., 2010). In the present study, however, we observed a negative and very high correlation (r = 0.71) between the IMAT content before the training and the net decrease of IMAT observed after HIT and IRT. The regression analysis also implied that the two training modalities were able to induce an average percent decay of IMAT of about 25% (95% CI 51.2-1.3). These results highlight the efficacy of HIT and IRT training programs in reducing the contribution of non-functional IMAT.
Muscle strength significantly increased only after IRT. This type of training has been found to be effective for increasing muscular performances of the limb extensors in elderly people (Onambélé et al., 2008), and the average percent increase of TMVC found in the present investigation (7.0% ± 9.8) is close to the one reported in the quoted paper. One unexpected finding consisted in the absence of any substantial and significant increase of strength after HIT, in spite of documented increases of ACSA and Vol. The dissociation between functional and morphological adaptations requires some extended comment.
As we know, the increase of muscular strength is a functional adaptation that results from several morphological and neural mechanisms that intervene during training (Folland and Williams, 2007). In this regard, the changes of muscular architecture are of paramount importance in order to understand how they may affect the improvement of strength consequent to the increase of muscle mass. As we have already outlined, there is a large bulk of evidence that shows that the θp of muscle fibers is increased in strength-trained muscles (Kawakami et al., 1993) and it augments with muscle hypertrophy (Aagard et al., 2001). This morphological change is beneficial because it will allow a greater packing of fibers for the same ACSA increasing PCSA, i.e., the area normal to the line of application of the force produced by the fibers. Of course, an increased θp will also decrease the force applied by the muscle along the tendon. Therefore, there is a sort of trade-off between the increase of muscle mass and the widening of θp on the strength measured at the ending of the tendon. Even though it has been geometrically demonstrated that any increase of θp that stays below 45° is compensated by the increase of PCSA, wherefrom an increase of strength, however, occurs, in the present case, the increase of θp after HIT was not probably sufficient to induce a substantial augmentation of PCSA (Figure 5). We must also consider that PCSA of the quadriceps was calculated by assuming an identical angle of θp measured at the level of the VL, which, in turn, was considered representative of the mean θp of the entire quadriceps. Of course, this assumption may have introduced an unpredictable error in the calculated PCSA of the quadriceps.
Secondly, the level of neuromuscular activation increased only after IRT in respect to the control condition before strength training. Even admitting that the interpolated twitch technique is not freed of several technical and methodological issues (Folland and Williams, 2007) and that activation is muscle specific (Belanger and McComas, 1981) and angle specific (Becker and Awiszus, 2001), one must acknowledge that recent studies confirm that strength training is followed by the increase of muscular activation in elderly humans (Reeves et al., 2004). Flywheel resistance training elicits a greater muscle activation of the involved muscles during isometric maximal voluntary contraction; in addition, muscular activation during both eccentric and concentric contraction seems to be maximal even in the early phases of training over the entire range of movement (Norrbrand et al., 2010). The capability of flywheel resistance training of inducing a greater neuromuscular activation may well explain the findings, after IRT, of a higher level of activation of the trained quadriceps. By incidence, the empirical model proposed by calculating the multiple linear regression between the percent increases of isokinetic torque and the percent increases of ACSA at 50% of femur length (X1) and of %Act (X2) seems to suggest that the gain in strength achieved with IRT was more ascribed to the increase of muscle mass than to the amelioration of muscle activation.
HIT was not followed, though, by any substantial increase of neuromuscular activation. It has been shown that, during cycling, the four bellies of the quadriceps are activated with different timing: VL and VM show the highest activation in the pushing phase from the top dead center to about 90°, or the first quadrant of the cycle; RF, a bi-articular muscle, shows bursts of biphasic activation in the first (0°–90°) and fourth (270°–360°) quadrants (Lima da Silva et al., 2016). We can therefore suggest that flywheel training seems to be able to induce greater neuromuscular activation of the involved muscles along the entire range of motion in respect of cycling and, hence, contribute substantially to the increase of strength.
The investigation is not freed from methodological weaknesses. The primary limitation to the generalization of our results is the absence of a control group: considering the experimental design, the study was not counterbalanced. As such, it suffered from intrinsic limitations, since the results were not freed from incidental effects other than the one directly induced by the interventions, and a longer washout period between training sessions could be considered in future studies. Furthermore, the effects of training on the antagonist muscles or on all the thigh muscles could be evaluated.
In this investigation, we evaluated for the first time the effect of HIT and of IRT on the IMAT and muscle quality of the quadriceps in elderly subjects. In order to unveil which changes occurred in muscle tissue due to these types of trainings, a group of elderly, although healthy, untrained volunteers were investigated, since, in this category of subjects, an age-related impaired muscular response has been frequently described. Despite initial doubts about the feasibility of carrying out high-intensity workouts with elderly subjects, we found that HIT and IRT, when performed with care and customizations, are absolutely safe and well tolerated by the subjects. Therefore, the meaning and the applicability of the results obtained in this study may be relevant to address training interventions in elderly subjects: our study protocol has been applied on healthy subjects; however, the effects of HIT and IRT on IMAT must also be evaluated in sarcopenic subjects.
Although these results must be interpreted with caution and the limitations of the study should be borne in mind, our results indicate that HIT and IRT seem to be able to elicit beneficial modifications of skeletal muscular mass, architecture, and quality in active elderly subjects in connection with an amelioration of the functional performances (strength, power, neuromuscular activation, etc.). In particular, a significant reduction of IMAT was evident after the two training interventions, a fact that led to the amplification of the percent changes of ACSA of the muscles when they were calculated without considering IMAT.
IMAT is an important predictor of muscle metabolism and also appears to be a modifiable muscle risk factor: adipose tissue stored in ectopic locations, as in the muscle, is connected with impaired glucose tolerance, chronic inflammation, and increased total cholesterol (Prior et al., 2007; Durheim et al., 2008; Koster et al., 2010). Physical activity and resistance or endurance training appear to be effective countermeasures against increases in IMAT. The exercise protocol proposed in our study has positively influenced IMAT: we can therefore speculate that the exercise carried out at high intensity reduces modifiable muscle risk factors.
The two training modalities caused a homogeneous increase of ACSA of the quadriceps at different percentages of the total muscle length. By the same token, the percent increase of Vol of the different bellies of the quadriceps turned out to be homogeneous. θp underwent an expected increase with both training modalities with a consensual increase of PCSA in IRT. However, specific strength per unit of PCSA did not change and the observed increases of strength seemed to be attributed to a parallel improvement of neuromuscular activation and muscle hypertrophy only after IRT.
We can therefore consider that both HIT and, especially, IRT induce beneficial modification on different systems with the final effect to counteract most of the causes of the morphological and functional consequences of sarcopenia.
The raw data supporting the conclusions of this manuscript will be made available by the authors, without undue reservation, to any qualified researcher.
The protocol and the methods of the study were approved by the Regional Review Board (approval on June 18, 2013) and written informed consent was obtained from each subject before entering the study.
PB, ET, and CC conceived the study. PB, ET, and EC collected the data. PB, ET, EC, and AR analyzed the data. PB and CC wrote the first draft of the manuscript. All authors approved final version of the manuscript.
This study was financially supported by the European Space Agency (ESA) Contract #4000102580 MAP Project “Astronaut Exercise Prescriptions Promoting Health and Fitness on Earth,” allocated to CC for the project “Cardiovascular and Skeletal Muscle Responses to Chronic Concurrent Exercise Using Flywheel Technology in Old Men.”
The authors declare that the research was conducted in the absence of any commercial or financial relationships that could be construed as a potential conflict of interest.
The authors would like to acknowledge Full Prof. Dr. Roberto Pozzi Mucelli, Dr. Andrea Pezzato, and Dr. Eugenio Oliboni for their technical support.
The Supplementary Material for this article can be found online at: https://www.frontiersin.org/articles/10.3389/fphys.2019.01260/full#supplementary-material
%Act, percent neuromuscular activation; θp, pennation angle of muscle fibers; ACSA, anatomical cross-sectional area; BMI, body mass index; HIT, high-intensity interval training; IMAT, intermuscular adipose tissue; IRT, isoinertial resistance training; Lf, fascicle length; MVC, maximal voluntary contraction; O2, oxygen uptake; O2max, maximal oxygen uptake; PCSA, physiological cross-sectional area; QF, quadriceps femoris; RF, rectus femoris; RT, resistance training; TC, TMVC, Maximal concentric and isometric muscular torques; Tm, muscle thickness; TMVCcorr, corrected maximal isometric force exerted on the patellar tendon calculated from TMVC at 90° of knee flection; VI, vastus intermedius; VL, vastus lateralis; VM, vastus medialis; Vol, muscle volume
Aagard, P., Andersen, J. L., Dyrhe-Poulsen, P., Leffers, A. M., Wagner, A., Magnusson, S. P., et al. (2001). A mechanism for increased contractile strength training: changes in muscle architecture. J. Physiol. 534, 613–623. doi: 10.1111/j.1469-7793.2001.t01-1-00613.x
Addison, O., Marcus, R. L., LaStayo, P. C., and Ryan, A. S. (2014). Intermuscular fat: a review of the consequences and causes. Int. J. Endocrinol. 2014:309570. doi: 10.1155/2014/309570
Alexander, R. M., and Vernon, A. (1975). The dimensions of knee and ankle muscles and the forces they exert. J. Hum. Mov. Stud. 1, 115–123.
Astorino, T. A., Allen, R. P., Roberson, D. W., Jurancich, M., Lewis, R., McCarthy, K., et al. (2011). Adaptations to high-intensity training are independent of gender. Eur. J. Appl. Physiol. 111, 1279–1286. doi: 10.1007/s00421-010-1741-y
Becker, R., and Awiszus, F. (2001). Physiological alterations of maximal voluntary quadriceps activation by changes of knee joint angle. Muscle Nerve 24, 667–672. doi: 10.1002/mus.1053
Belanger, A. Y., and McComas, A. J. (1981). Extent of motor units activation during effort. J. Appl. Physiol. 51, 1131–1135. doi: 10.1152/jappl.1981.51.5.1131
Berg, H., and Tesch, P. A. (1994). A gravity-independent ergometer to be used for resistance training in space. Aviat. Space. Environ. Med. 65, 752–756.
Bruseghini, P., Calabria, E., Tam, E., Milanese, C., Oliboni, E., Pezzato, A., et al. (2015). Effects of eight weeks of aerobic interval training and of isoinertial resistance training on risk factors of cardiometabolic diseases and exercise capacity in healthy elderly subjects. Oncotarget 6, 16998–17015. doi: 10.18632/oncotarget.4031
Buchheit, M., and Laursen, P. B. (2013a). High-intensity interval training, solutions to the programming puzzle: part I: cardiopulmonary emphasis. Sports Med. 43, 313–338. doi: 10.1007/s40279-013-0029-x
Buchheit, M., and Laursen, P. B. (2013b). High-intensity interval training, solutions to the programming puzzle. Part II: anaerobic energy, neuromuscular load and practical applications. Sports Med. 43, 927–954. doi: 10.1007/s40279-013-0066-5
Cadore, E. L., Silveira Pinto, R., Bottaro, M., and Izquierdo, M. (2014). Strength and endurance training prescription in healthy and frail elderly. Aging Dis. 5, 183–195. doi: 10.14336/AD.2014.0500183
Castro, M. J., Apple, D. F. Jr., Hillegass, E. A., and Dudley, G. A. (1999). Influence of complete spinal cord injury on skeletal muscle cross sectional area within the first 6 months of injury. Eur. J. Appl. Physiol. 80, 373–378. doi: 10.1007/s004210050606
Connelly, D. M., and Vandervoort, A. A. (2000). Effects of isokinetic strength training on concentric and eccentric torque development in the ankle dorsiflexors of older adults. J. Gerontol. A. Biol. Sci. Med. Sci. 55, 465–472.
Cunha, P. M., Ribeiro, A. S., Tomeleri, C. M., Schoenfeld, B. J., Silva, A. M., Souza, M. F., et al. (2017). The effects of resistance training volume on osteosarcopenic obesity in older women. J. Sports Sci. 10, 1–8. doi: 10.1080/02640414.2017.1403413
Durheim, M. T., Slentz, C. A., Bateman, L. A., Mabe, S. K., and Kraus, W. E. (2008). Relationships between exercise-induced reductions in thigh intermuscular adipose tissue, changes in lipoprotein particle size, and visceral adiposity. Am. J. Physiol. Endocrinol. Metab. 295, E407–E412. doi: 10.1152/ajpendo.90397.2008
Earp, J. E., Newton, R. U., Cormie, P., and Blazevich, A. J. (2015). Inhomogeneous quadriceps femoris hypertrophy in response to strength and power training. Med. Sci. Sport. Exerc. 47, 2389–2397. doi: 10.1249/MSS.0000000000000669
Erskine, R. M., Jones, D. A., Maganaris, C. N., and Degens, H. (2009). In vivo specific tension of the human quadriceps femoris muscle. Eur. J. Appl. Physiol. 106, 827–838. doi: 10.1007/s00421-009-1085-7
Estes, R. R., Malinowski, A., Piacentini, M., Thrush, D., Salley, E., Losey, C., et al. (2017). The effect of high intensity interval run training on cross-sectional area of the vastus lateralis in untrained college students. Int. J. Exerc. Sci. 10, 137–145.
Faul, F., Erdefelder, E., Lang, A. G., and Buchner, A. (2007). G∗Power 3: a flexible statistical power analysis program for the social, behavioral, and biomedical sciences. Behav. Res. Method 39, 175–191. doi: 10.3758/bf03193146
Folland, J. P., and Williams, A. G. (2007). The adaptations to strength training. Morphological and neurological contributions to increased strength. Sports Med. 37, 145–168. doi: 10.2165/00007256-200737020-00004
Franchi, M. V., Reeves, N. D., and Narici, M. V. (2017). Skeletal muscle remodeling in response to eccentric vs. concentric loading: morphological, molecular, and metabolic adaptations. Front. Physiol. 8:447. doi: 10.3389/fphys.2017.00447
Gabriel, D. A., Kamen, G., and Frost, G. (2006). Neural adaptations to resistive exercise. Mechanisms and recommendations for training practices. Sports Med. 36, 133–149. doi: 10.2165/00007256-200636020-00004
Gibala, M. J., Little, J. P., van Essen, M., Wilkin, G. P., Burgomaster, K. A., Safdar, A., et al. (2006). Short-term sprint interval versus traditional endurance training: similar initial adaptations in human skeletal muscle and exercise performance. J. Physiol. 575, 901–911. doi: 10.1113/jphysiol.2006.112094
Goodpaster, B. H., Chomentowski, P., Ward, B. K., Rossi, A., Glynn, N. W., Delmonico, M. J., et al. (2008). Effects of physical activity on strength and skeletal muscle fat infiltration in older adults: a randomized controlled trial. J. Appl. Physiol. 105, 1498–1503. doi: 10.1152/japplphysiol.90425.2008
Goran, M. I., and Poehlman, E. T. (1992). Total energy expenditure and energy requirements in healthy elderly persons. Metabolism 41, 744–753. doi: 10.1016/0026-0495(92)90315-2
Häkkinen, K., Alen, M., Kallinen, M., Newton, R. U., and Kraemer, W. J. (2000). Neuromuscular adaptation during prolonged strength training, detraining and re-strength-training in middle-aged and elderly people. Eur. J. Appl. Physiol. 83, 51–62. doi: 10.1007/s004210000248
Harber, M. P., Konopka, A. R., Undem, M. K., Hinkley, J. M., Minchev, K., Kaminsky, L. A., et al. (2012). Aerobic exercise training induces skeletal muscle hypertrophy and age-dependent adaptations in myofiber function in young and older men. J. Appl. Physiol. 113, 1495–1504. doi: 10.1152/japplphysiol.00786.2012
Hopkins, W. G., Marshall, S. W., Batterham, A. M., and Hanin, J. (2009). Progressive statistics for studies in sports medicine and exercise science. Med. Sci. Sports Exerc. 41, 3–13. doi: 10.1249/MSS.0b013e31818cb278
Housh, D. J., Housh, T. J., Johnson, G. O., and Chu, W. K. (1992). Hypertrophic response to unilateral concentric isokinetic resistance training. J. Appl. Physiol. 73, 65–70. doi: 10.1152/jappl.1992.73.1.65
Hunter, G. R., McCarthy, J. P., and Bamman, M. M. (2004). Effects of resistance training on older adults. Sports Med. 34, 329–348. doi: 10.2165/00007256-200434050-00005
Hurley, B. F., Redmond, R. A., Pratley, R. E., Treuth, M. S., Rogers, M. A., and Goldbeg, A. P. (1994). Effects of strength training on muscle hypertrophy and muscle cell disruption in older men. Int. J. Sports Med. 16, 378–384. doi: 10.1055/s-2007-973024
Ikenaga, M., Yamada, Y., Higaki, Y., and Kiyonaga, A. (2017). Effects of a 12-week, short-interval, intermittent, low-intensity, slow-jogging program on skeletal muscle, fat infiltration, and fitness in older adults: randomized controlled trial. Eur. J. Appl. Physiol. 117, 7–15. doi: 10.1007/s00421-016-3493-9
Jacobs, J. L., Marcus, R. L., Morrell, G., and LaStayo, P. (2014). Resistance exercise with older fallers: its impact on intermuscular adipose tissue. Biomed Res. Int. 2014:398960. doi: 10.1155/2014/398960
Kawakami, Y., Abe, T., and Fukunaga, T. (1993). Muscle-fibre pennation angles are greater in hypertrophied than in normal muscles. J. Appl. Physiol. 74, 2740–2744. doi: 10.1152/jappl.1993.74.6.2740
Keppel, G., and Wickens, T. D. (2004). Design and Analysis. A Researcher’s Handbook, 4th Edn. Upper Saddle River, NJ: Pearson Prentice Hall, 400–431.
Kohrt, W. M., and Holloszy, J. O. (1995). Loss of skeletal muscle mass with aging: effect on glucose tolerance. J. Gerontol. A. Biol. Sci. Med. Sci. 50, 68–72. doi: 10.1093/gerona/50a.special_issue.68
Koster, A., Stenholm, S., Alley, D. E., Kim, L. J., Simonsick, E. M., Kanaya, A. M., et al. (2010). Body fat distribution and inflammation among obese older adults with and without metabolic syndrome. Obesity 18, 2354–2361. doi: 10.1038/oby.2010.86
Kraemer, W. J., Häkkinen, K., Newton, R. U., Nindl, B. C., Volek, J. S., Mccormick, M., et al. (1999). Effects of heavy resistance training on hormonal response patterns in younger vs. older men. J. Appl. Physiol. 87, 982–992. doi: 10.1152/jappl.1999.87.3.982
Kufel, T. J., Pineda, L. A., and Mador, M. J. (2002). Comparison of potentiated and unpotentiated twitches as an index of muscle fatigue. Muscle Nerve 25, 438–444. doi: 10.1002/mus.10047
Lima da Silva, J. C., Tarassova, O., Ekblom, M. M., Andersson, E., Rönquist, G., and Arndt, A. (2016). Quadriceps and hamstrings muscle activity during cycling as measured with intramuscular electromyography. Eur. J. Appl. Physiol. 116, 1807–1817. doi: 10.1007/s00421-016-3428-5
Mackey, D. C., Manini, T. M., Schoeller, D. A., Koster, A., Glynn, N. W., Goodpaster, B. H., et al. (2011). Validation of an armband to measure daily energy expenditure in older adults. J. Gerontol. A Biol. Sci. Med. Sci. 66, 1108–1113. doi: 10.1093/gerona/glr101
Mangine, G. T., Redd, M. J., Gonzalez, A. M., Townsend, J. R., Wells, A. J., Jajtner, A. R., et al. (2018). Resistance training does not induce uniform adaptations to quadriceps. PLoS One 13:e0198304. doi: 10.1371/journal.pone.0198304
Marcus, R. L., Addison, O., Kidde, J. P., Dibble, L. E., and Lastayo, P. C. (2010). Skeletal muscle fat infiltration: impact of age, inactivity, and exercise. J. Nutr. Health Aging 14, 362–366. doi: 10.1007/s12603-010-0081-2
Maroto-Izquierdo, S., García-López, D., Fernandez-Gonzalo, R., Moreira, O. C., González-Gallego, J., and de Paz, J. A. (2017). Skeletal muscle functional and structural adaptations after eccentric overload flywheel resistance training: a systematic review and meta-analysis. J. Sci. Med. Sport 20, 943–951. doi: 10.1016/j.jsams.2017.03.004
McNair, P., Depledge, J., Brettkelly, M., and Stanley, S. (1996). Verbal encouragement: effects on maximum effort voluntary muscle action. Br. J. Sports Med. 30, 243–245. doi: 10.1136/bjsm.30.3.243
Motulsky, H. Y., and Christopoulos, A. (2004). Fitting Models to Biological Data using Linear and Nonlinear Regression. New York, NY: Oxford University Press, 104–108.
Narici, M., and Maffulli, N. (2010). Sarcopenia: characteristics, mechanisms and functional significance. Br. Med. Bull. 95, 139–159. doi: 10.1093/bmb/ldq008
Narici, M., Maganaris, C., Reeves, N., and Capodaglio, P. (2003). Effect of aging on human muscle architecture. J. Appl. Physiol. 95, 2229–2234. doi: 10.1152/japplphysiol.00433.2003
Narici, M. V., Hoppeler, H., Kayser, B., Landoni, L., Claassen, H., Gavardi, C., et al. (1996). Human quadriceps cross-sectional area, torque and neural activation during 6 months strength training. Acta. Physiol. Scand. 157, 175–186. doi: 10.1046/j.1365-201X.1996.483230000.x
Narici, M. V., Landoni, L., and Minetti, A. E. (1992). Assessment of human knee extensor muscles stress from in vivo physiological cross-sectional area and strength measurements. Eur. J. Appl. Physiol. 65, 438–444. doi: 10.1007/BF00243511
Nicklas, B. J., Chmelo, E., Delbono, O., Carr, J. J., Lyles, M. F., and Marsh, A. P. (2015). Effects of resistance training with and without caloric restriction on physical function and mobility in overweight and obese older adults: a randomized controlled trial. Am. J. Clin. Nutr. 101, 991–999. doi: 10.3945/ajcn.114.105270
Norrbrand, L., Pozzo, M., and Tesch, P. A. (2010). Flywheel resistance training calls for greater eccentric muscle activation than weight training. Eur. J. Appl. Physiol. 110, 997–1005. doi: 10.1007/s00421-010-1575-7
Onambélé, G. L., Maganaris, C. N., Mian, O. S., Tam, E., Rejc, E., McEwan, I. M., et al. (2008). Neuromuscular and balance responses to flywheel inertial versus weight training in older persons. J. Biomech. 41, 3133–3138. doi: 10.1016/j.jbiomech.2008.09.004
Power, G., Makrakos, D., Rice, C., and Vandervoort, A. (2013). Enhanced force production in old age is not a far stretch: an investigation of residual force enhancement and muscle architecture. Physiol. Rep. 1:e00004. doi: 10.1002/phy2.4
Prior, S. J., Joseph, L. J., Brandauer, J., Katzel, L. I., Hagberg, J. M., and Ryan, A. S. (2007). Reduction in midthigh low-density muscle with aerobic exercise training and weight loss impacts glucose tolerance in older men. J. Clin. Endocrinol. Metab. 92, 880–886. doi: 10.1210/jc.2006-2113
Raj, I., Bir, S., and Shield, A. (2012). Reliability of ultrasonographic measurement of the architecture of the vastus lateralis and gastrocnemius medialis muscles in older adults. Clin. Physiol. Funct. Imaging 32, 65–70. doi: 10.1111/j.1475-097X.2011.01056.x
Reeves, N. D., Narici, M. V., and Maganaris, C. N. (2004). Effect of resistance training on skeletal muscle-specific force in elderly humans. J. Appl. Physiol. 96, 885–892. doi: 10.1152/japplphysiol.00688.2003
Rossi, A., Zoico, E., Goodpaster, B. H., Sepe, A., Di Francesco, V., Fantin, F., et al. (2010). Quantification of intermuscular adipose tissue in the erector spinae muscle by MRI: agreement with histological evaluation. Obesity 18, 2379–2384. doi: 10.1038/oby.2010.48
Scalzo, R. L., Peltonen, G. L., Binns, S. E., Shankaran, M., Giordano, G. R., Hartley, D. A., et al. (2014). Greater muscle protein synthesis and mitochondrial biogenesis in males compared with females during sprint interval training. FASEB J. 28, 2705–2714. doi: 10.1096/fj.13-246595
Shield, A., and Zhou, S. (2004). Assessing voluntary muscle activation with the Twitch interpolation technique. Sports Med. 34, 253–267. doi: 10.2165/00007256-200434040-00005
Smidt, G. L. (1973). Biomechanical analysis of knee flexion and extension. J. Biomech. 6, 79–92. doi: 10.1016/0021-9290(73)90040-7
Tam, E., Bruseghini, P., Capelli, C., Oliboni, E., Pezzato, A., Pogliaghi, S., et al. (2018). Effect of endurance and strength training on the slow component of VO2 kinetics in elderly humans. Front. Physiol. 9:1353. doi: 10.3389/fphys.2018.01353
Tesch, P. A. (1988). Skeletal-muscle adaptations consequent to long-term heavy resistance exercise. Med. Sci. Sports Exerc. 20, S132–S134.
Tesch, P. A., Fernandez-Gonzalo, R., and Lundberg, T. R. (2017). Clinical applications of iso-inertial, eccentric-overload (YoYoTM) resistance exercise. Front. Physiol. 27:241. doi: 10.3389/fphys.2017.00241
Tomeleri, C. M., Ribeiro, A. S., Souza, M. F., Schiavoni, D., Schoenfeld, B. J., Venturini, D., et al. (2016). Resistance training improves inflammatory level, lipid and glycemic profiles in obese older women: a randomized controlled trial. Exp. Gerontol. 84, 80–87. doi: 10.1016/j.exger.2016.09.005
Trappe, T. A., Lindquist, D. M., and Carrithers, J. A. (2001). Muscle-specific atrophy of the quadriceps femoris with aging. J. Appl. Physiol. 90, 2070–2074. doi: 10.1152/jappl.2001.90.6.2070
Vaughan, L., Zurlo, F., and Ravussin, E. (1991). Aging and energy expenditure. Am. J. Clin. Nutr. 53, 821–825. doi: 10.1093/ajcn/53.4.821
Vera-Ibañez, A., Colomer-Poveda, D., Romero-Arenas, S., Viñuela-García, M., and Márquez, G. (2017). Neural adaptations after short-term wingate-based high-intensity interval training. J. Musculoskelet Neuronal Interact. 17, 275–282.
Vincens-Bordas, J., Esteve, E., Fort-Vanmeerhæge, A., and Bandholm, T. (2018). Is inertial flywheel resistance training superior to gravity-dependent resistance training in improving muscle strength? A systematic review with meta-analysis. J. Sci. Med. Sport 21, 75–83. doi: 10.1016/j.jsams.2017.10.006
Zoico, E., Rossi, A., Di Francesco, V., Sepe, A., Olioso, D., Pizzini, F., et al. (2010). Adipose tissue infiltration in skeletal muscle of healthy elderly men: relationship with body composition, insulin resistance, and inflammation at the systemic and tissue level. J. Gerontol. A. Biol. Sci. Med. Sci. 65, 295–299. doi: 10.1093/gerona/glp155
Keywords: aging, high-intensity interval training, isoinertial resistance training, muscle volume, muscle architecture, muscle activation, intermuscular adipose tissue
Citation: Bruseghini P, Capelli C, Calabria E, Rossi AP and Tam E (2019) Effects of High-Intensity Interval Training and Isoinertial Training on Leg Extensors Muscle Function, Structure, and Intermuscular Adipose Tissue in Older Adults. Front. Physiol. 10:1260. doi: 10.3389/fphys.2019.01260
Received: 11 April 2019; Accepted: 17 September 2019;
Published: 09 October 2019.
Edited by:
Adamantios Arampatzis, Humboldt University of Berlin, GermanyReviewed by:
David Tomlinson, Manchester Metropolitan University, United KingdomCopyright © 2019 Bruseghini, Capelli, Calabria, Rossi and Tam. This is an open-access article distributed under the terms of the Creative Commons Attribution License (CC BY). The use, distribution or reproduction in other forums is permitted, provided the original author(s) and the copyright owner(s) are credited and that the original publication in this journal is cited, in accordance with accepted academic practice. No use, distribution or reproduction is permitted which does not comply with these terms.
*Correspondence: Carlo Capelli, Y2FybG8uY2FwZWxsaUB1bml2ci5pdA==
Disclaimer: All claims expressed in this article are solely those of the authors and do not necessarily represent those of their affiliated organizations, or those of the publisher, the editors and the reviewers. Any product that may be evaluated in this article or claim that may be made by its manufacturer is not guaranteed or endorsed by the publisher.
Research integrity at Frontiers
Learn more about the work of our research integrity team to safeguard the quality of each article we publish.