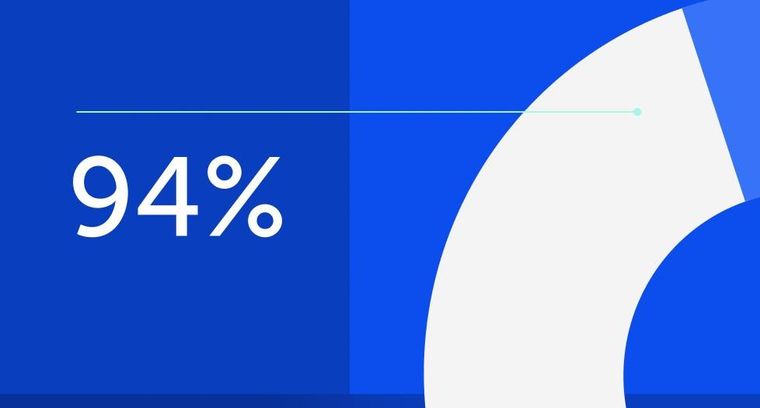
94% of researchers rate our articles as excellent or good
Learn more about the work of our research integrity team to safeguard the quality of each article we publish.
Find out more
ORIGINAL RESEARCH article
Front. Physiol., 28 August 2019
Sec. Environmental, Aviation and Space Physiology
Volume 10 - 2019 | https://doi.org/10.3389/fphys.2019.01032
This article is part of the Research TopicTime Domains of Hypoxia Adaptation: Evolutionary Insights and ApplicationsView all 24 articles
Autonomic innervation of the pulmonary vasculature triggers vasomotor contractility predominately through activation of alpha-adrenergic receptors (α-ARs) in the fetal circulation. Long-term hypoxia (LTH) modulates pulmonary vasoconstriction potentially through upregulation of α1-AR in the vasculature. Our study aimed to elucidate the role of α-AR in phenylephrine (PE)-induced pulmonary vascular contractility, comparing the effects of LTH in the fetal and adult periods on α-AR subtypes and PE-mediated Ca2+ responses and contractions. To address this, we performed wire myography, Ca2+ imaging, and mRNA analysis of pulmonary arteries from ewes and fetuses exposed to LTH or normoxia. Postnatal maturation depressed PE-mediated contractile responses. α2-AR activation contracted fetal vessels; however, this was suppressed by LTH. α1A- and α1B-AR subtypes contributed to arterial contractions in all groups. The α1D-AR was also important to contractility in fetal normoxic vessels and LTH mitigated its function. Postnatal maturity increased the number of myocytes with PE-triggered Ca2+ responses while LTH decreased the percentage of fetal myocytes reacting to PE. The difference between myocyte Ca2+ responsiveness and vessel contractility suggests that fetal arteries are sensitized to changes in Ca2+. The results illustrate that α-adrenergic signaling and vascular function change during development and that LTH modifies adrenergic signaling. These changes may represent components in the etiology of pulmonary vascular disease and foretell the therapeutic potential of adrenergic receptor antagonists in the treatment of pulmonary hypertension.
The pulmonary circulation is regulated to optimize respiratory gas exchange. Various factors affect pulmonary vascular reactivity, including neural, hormonal, inflammatory, and local mediators. Autonomic innervation of the pulmonary vasculature has been documented in various mammals although there is significant variation in the distribution of the nerves (Barnes and Liu, 1995). Considerable evidence also indicates there is a greater density of autonomic nerve fibers in larger vessels and vascular branching points (Daly and Hebb, 1966). The functional significance of sympathetic innervation of the human lung is not well understood although both α- and β-adrenergic receptors (ARs) are expressed in the pulmonary vascular bed. α-AR function predominates, however, in the fetal circulation with a higher basal vasomotor tone and greater reactivity to α-adrenergic stimulation (Mandel and Taichman, 2006). Notably, long-term hypoxia (LTH) results in upregulation of α1-AR gene transcription (Salvi, 1999).
Pulmonary vasoconstriction and high pulmonary vascular resistance, principally owing to a relatively low oxygen tension, are hallmarks of the fetal circulation. The high vascular resistance reduces energy expenditure to an organ that does not serve its primary purpose in utero (Weir et al., 2000). LTH during gestation due to high-altitude living, smoking, maternal anemia, placental insufficiency, or other causes is detrimental to the fetus and causes pulmonary hypertension and other complications in the newborn (Niermeyer, 2007). However, the underlying mechanisms remain largely elusive.
Previously, we demonstrated in sheep that LTH increased norepinephrine-induced contractions in pulmonary arteries and decreased acetylcholine-mediated relaxations in pulmonary veins (Xue et al., 2008). Other studies showed that phenylephrine (PE)-induced contractions were increased in endothelium intact but not denuded, pig pulmonary arteries exposed to hypoxia (Ogata et al., 1992). We also demonstrated that PE-induced cytosolic Ca2+ responses were similar in pulmonary arterial myocytes from fetal and adult sheep (Goyal et al., 2008). Nonetheless, little is known regarding the impact of LTH in the fetal and adult periods on adrenergic-mediated contractions or the underlying receptor-induced Ca2+ signals. The aim of the current studies was to determine the influence of long-term hypoxic stress on α-AR-dependent pulmonary arterial contractility. We tested the hypothesis that LTH in the fetal and adult periods enhance α-AR-dependent pulmonary arterial contractility. This was evaluated in intact arterial segments from fetal and adult sheep that lived at low altitude or were exposed to high-altitude hypoxia for 110+ days.
Animal handling was performed as per our previous studies over the past two decades including numerous studies on vessels of the pulmonary vasculature. Sheep were chosen for study because of their similar developmental profile to human infants, especially with regards to their lung development (Papamatheakis et al., 2013; Ducsay et al., 2018). Secondarily, the changes in lung structure and function are somewhat mild relative to other species and again similar to humans (Papamatheakis et al., 2013). Non-pregnant and pregnant ewes born at low altitudes were purchased from Nebeker Ranch (Lancaster, CA; 720 m) and transported to the Loma Linda University (353 m; arterial PaO2 = 95 ± 5 Torr) or were transported and acclimatized to high altitude (3,801 m, PaO2 = 60 ± 5 Torr) at the Barcroft Laboratory, White Mountain Research Station (Bishop, CA) for approximately 110 days (Kamitomo et al., 1993; Longo et al., 1996). Previous studies show that the PaO2 of fetal animals exposed to this level of hypoxia was roughly 20 Torr (Kamitomo et al., 1993; Papamatheakis et al., 2013). Animals acclimatized to high altitude were transported to the Loma Linda University, and shortly after arrival, a tracheal catheter was placed in the ewe, through which N2 flowed at a rate adjusted to maintain PaO2 at ~60 Torr (Kamitomo et al., 1994) until the time of the experimental study. Arterial blood gasses were monitored in the hypoxic animals several times each day and the N2 flow rate adjusted as needed to regulate maternal PaO2. Within 1–5 days after arriving at the university, anesthesia was induced with Ketamine (10 mg/kg IV) and Midazolam (5 mg/kg IV).The ewe was then placed in the supine position, intubated, and anesthesia maintained by inhalation of 1.5–2.5% Isoflurane in oxygen. Following tissue collection, sheep were sacrificed by intravenous injection of the proprietary euthanasia solution, Euthasol (2 ml/kg; Virbac, Ft. Worth, TX, USA). All tissue bath and calcium experimental procedures were performed as previously described (Goyal et al., 2011; Papamatheakis et al., 2011, 2012; Blum-Johnston et al., 2016; Shen et al., 2018) within the regulations of the Animal Welfare Act, the National Institutes of Health Guide for the Care and Use of Laboratory Animals, “The Guiding Principles in the Care and Use of Animals” approved by the Council of the American Physiological Society, and the Animal Care and Use Committee of the Loma Linda University. Although the animals were housed in a rarified environment at the White Mountain Research Station and then the low PaO2 was maintained at the Loma Linda University by breathing hypoxic gasses, all experimental studies were performed under normoxic conditions at the Loma Linda University. This differs from studies performed by researchers in Chile who use sheep housed at the Putre Research Station, International Center for Andean Studies at 3,600 m above sea level (Herrera et al., 2007, 2010). In comparison to work by researchers performed at Putre on sheep and other high-altitude research stations using various other animal models (Papamatheakis et al., 2013; Ducsay et al., 2018), our studies identify those changes and mechanisms that persist in low-altitude environments following high-altitude living and gestation. Our work using terrestrial high-altitude exposure also differs from studies performed on sheep exposed to hypoxia in chambers that have relatively short exposure periods (Allison et al., 2016; McGillick et al., 2017). When comparing the terrestrial and chamber models of exposure, the long durations of our exposures would restrict the number of sheep that could be examined each year. Tissues from a total of 106 experimental animals were examined for the studies of this report. This includes 22 adult normoxic (AN), 26 adult hypoxic (AH), 36 fetal normoxic (FN), and 25 fetal hypoxic (FH) animals. The long duration of exposure combined with the large numbers of animals used in our studies makes such chamber studies or performing studies at the White Mountain field station largely impractical. Even still, we are also fully aware that other phenomenon and mechanisms could be unmasked by performing studies at high-altitude research laboratories, such as the augmented hypoxic pulmonary vascular pressures we previously reported in 2-week-old newborn lambs born at high altitude (Blood et al., 2013).
Pulmonary arteries were dissected immediately from isolated lungs for contractility experiments from non-pregnant adult ewes or fetuses under normoxic conditions. Fourth and fifth order pulmonary arteries with internal diameters of about 500–700 μm were dissected free of parenchyma and cut into 5 mm long rings in ice-cold phosphate free balanced salt solution of the following composition (mM): 126 NaCl; 5 KCl; 10 HEPES; 1 MgCl2; 2 CaCl2; 10 glucose; pH 7.4 (adjusted with NaOH). To avoid complications from endothelium-mediated effects, the endothelium was disrupted by carefully rotating the artery on a small roughened hypodermic needle or on the mounting wire (Papamatheakis et al., 2011).
Pulmonary arterial contraction studies were performed as previously described at the Loma Linda University at 353 m (Blum-Johnston et al., 2016; Giang et al., 2016). In brief, pulmonary arterial rings on tungsten wires were suspended in organ baths (Radnoti Glass Instruments, Inc. Monrovia, CA) that contained 5 or 10 ml of modified Krebs-Henseleit solution containing in mM: 120 NaCl; 4.8 KCl; 1.2 K2HPO4; 25 NaHCO3; 1.2 MgCl2; 2.5 CaCl2; 10 glucose. The bath solution was maintained at 37°C and aerated with 95% O2 to 5% CO2 (pH = 7.4). The wires were attached to low compliance force transducers (Radnoti Glass Instruments Inc.) for the measurement of isometric force (Blum-Johnston et al., 2016; Giang et al., 2016) that were connected to an analogue to digital data interface (Powerlab 16/30 A/D Instruments, Colorado Springs, CO; or MP100, Biopac, Goleta, CA) attached to a computer. The changes in tension were recorded using Chart 5.5 (AD Instruments, Colorado Springs, CO) or AcqKnowledge 3.9 (Biopac, Systems, Inc., Goleta, CA) and analyzed post hoc. Vessels were equilibrated without tension for a minimum of 30 min and tensioned to approximately 0.75 g (Goyal et al., 2011; Papamatheakis et al., 2011; Blum-Johnston et al., 2016). Arterial tension was normalized to a maximum response obtained with 125 mM KCl (high K+) (%TKmax) or 10 μM serotonin (%T5HTmax). For evaluating dose-response characteristics, arteries were stimulated by applying 1 nM to 100 μM phenylephrine or 100 pM to 10 μM dexmedetomidine (DMT) logarithmically without washing in-between each concentration increase. Figure 1 studies were based on arterial segments isolated from normoxic fetal (9), adult (8) and hypoxic fetal (11), and adult (12) animals, which served as the controls for the pharmacological studies outlined in Figures 2, 4. Figure 2 studies were based on arteries isolated from normoxic fetal prazosine (3) and yohimbine (5); adult prazosine (4) and yohimbine (4); as well as hypoxic fetal prazosine (4) and yohimbine (4); adult prazosine (4) and yohimbine (4). Figure 3 studies were based on arteries isolated from fetal normoxic animals for control (3) and yohimbine (4) studies. Figure 4 studies were based on arteries isolated from normoxic fetal CEC (4), WB (4), and BMY (4); adult CEC (5), WB (4), and BMY (5); as well as hypoxic fetal CEC (4), WB (4), and BMY (4); adult CEC (4), WB (4), and BMY (4). Figure 5 measurements were made in six animals from each group except for the fetal normoxic group, which was based on tissues from seven animals. Figure 6 recordings were made in three adult normoxic, three adult hypoxic, five fetal normoxic, and three fetal hypoxic animals.
Figure 1. Phenylephrine-mediated contraction of pulmonary arteries from sheep is depressed in the adult period and enhanced by LTH. (A) Dose-response curves of pulmonary arterial rings exposed to 1 nM to 100 μM of phenylephrine in an additive manner from normoxic (solid lines) and LTH (dashed lines) fetal (gray lines and boxes) and adult (black lines and circles) sheep. Curves are plotted in relation to the maximal contraction induced by initial stimulation of 125 mM K+ Krebs-Henseleit solution (%TKmax). (B) Maximum contraction relative to %TKmax. (C) Log EC50 for phenylephrine induced contraction. (D) Area under the dose-response curve relative to high K+ contraction. (E) Absolute force developed for 100 μM phenylephrine-induced contraction. Points and bars are mean values and error bars indicate ± S.E.M. Comparisons between groups were made using a two-way ANOVA with a Bonferroni post hoc analysis (*p < 0.05, **p < 0.01, ***p < 0.001). Numbers of animals for each group are provided in the “Methods” section.
Figure 2. Phenylephrine-induced contraction is largely dependent on α1-adrenergic contraction. (a) Dose-response curves of pulmonary arterial rings exposed to 1 nM to 100 μM phenylephrine in an additive manner from normoxic and LTH, fetal (A,B) and adult (C,D) sheep. Solid lines with circles indicate vehicle-control (DMSO) while long dashes and squares indicate 10 nM prazosin and triangles and short dashes indicate 100 nM yohimbine. Log agonist vs. response curves are plotted in relation to the maximal contraction induced by initial stimulation of 125 mM K+ Krebs-Henseleit solution (%TKmax). (b) Log EC50 for phenylephrine-induced contraction. (c) Maximum contraction relative to %TKmax. (d) Area under the dose-response curve relative to high K+ contraction. Points and bars are mean values while error bars indicate ± S.E.M. Comparisons of drug treated arteries to control were made using a one-way ANOVA with a Newman-Keuls multiple comparison test (**p < 0.01, ***p < 0.001). Numbers of animals for each fetal and adult group are provided in the “Methods” section and in (b).
Figure 3. Dexmedetomidine causes contraction and relaxation of normoxic fetal pulmonary arteries. Dose-response curves of pulmonary arterial rings exposed to 100 pM to 10 μM of dexmedetomidine in an additive manner from normoxic fetal sheep in the absence (A) and presence (B) of 10 μM serotonin. Gray lines and triangles are for dexmedetomidine contraction (A) or relaxation (B) in the presence of yohimbine, and black lines and boxes are for dexmedetomidine contraction (A) or relaxation (B) in the presence of vehicle-control (DMSO). Solid lines indicate log agonist vs. response curves that are plotted in relation to the maximal contraction induced by initial stimulation of (A) 125 mM K+ Krebs-Henseleit solution (%TKmax) or (B) 10 μM serotonin. Points and error bars indicate mean ± S.E.M. Numbers of animals for each group are provided in the “Methods” section.
Figure 4. α1A-AR and α1B-AR antagonists preferentially block phenylephrine-induced pulmonary vascular contractility. (a) Dose response curves of pulmonary arterial rings exposed to 1 nM to 100 μM phenylephrine in an additive manner from normoxic and LTH, fetal (A,B) and adult (C,D) sheep. Curves of log agonist vs. response were repeated in the absence (circles and solid lines) or presence of 10 μM CEC (α1B-AR blocker, squares and long dashed lines), 100 nM WB (α1A-AR blocker, triangles and short dashed lines) or 100 nM BMY (α1D-AR blocker, upside down triangles and dashed and dotted lines) for normoxic and LTH, fetal (A,B) and adult (C,D) sheep pulmonary arteries. (b) Log EC50 for phenylephrine induced contraction. (c) Maximum contraction relative to high K+. (d) Area under the dose-response curve relative to high K+ contraction. Points and bars are mean values while error bars indicate ± S.E.M. Comparisons of drug treated arteries to control were made using a one-way ANOVA with a Newman-Keuls Multiple Comparison Test (*p < 0.05, **p < 0.01, ***p < 0.001). Numbers of animals for each fetal and adult group are provided in the “Methods” section and (b).
Figure 5. LTH in the fetal and adult periods have unique impact on mRNA expression of alpha adrenergic receptors. Presented are the raw Cycle threshold (Ct) values from real time quantitative PCR measurements for (A) Alpha 1a AR, (B) Alpha 1b AR, (C) Alpha 1d AR, and (D) GAPDH. Normalization was to input RNA, all PCR reactions used input cDNA derived from 5 ng of total RNA. Bars and error bars represent the mean ± S.E.M. Significant differences between groups were established using a two-way ANOVA with a Bonferroni post hoc analysis (*p < 0.05, **p < 0.01, ***p < 0.001). Numbers of animals for each group are provided in the methods section.
Figure 6. Impact of LTH in the fetal and adult periods on unstimulated and PE-mediated whole-cell Ca2+ responses in pulmonary arterial myocytes. Shown are representative fluorescent images at time points shown by the arrow and the traces of fractional Fluo-4 fluorescence for unstimulated (A) and 10 μM phenylephrine stimulated (B) arteries. (C) Percentage of myocytes in a 1,000 μm2 area that had Ca2+ responses to 10 μM PE under unstimulated (open circles) and stimulated (filled circles) conditions based on the number of runs examined. Colored circles on each image correspond to the placement of regions of interest for the traces of the same color in the tracing. About 10 μM phenylephrine was present during the time period denoted by the horizontal bar. Images were made with a 20× non-immersion objective (NA 0.8). Scale bar is 25 μm. Numbers of animals for each group are provided in the “Methods” section.
To obtain RNA of adequate quality for qPCR analysis from fibrous sheep arteries, a protocol combining the Qiagen® Fibrous Tissue RNeasy kit (Qiagen, Germantown, MD) with a Bullet blender (Next Advance Inc.) was developed for mid-sized pulmonary arteries as described below. Notably, the α-AR subtypes in native tissue were expected to have low expression (Michel et al., 2009; Schonbrunn, 2014), increasing concerns about the accuracy of the qPCR measurements. A 450 μl aliquot of buffer RLT with Mercaptoethanol was placed into the RNAse-free Blue Rhino Bead beating tube at room temperature. RNA later treated vessels that were frozen in liquid N2 and stored at −80°C were then placed into the tube and the bullet blender unit was run for 3 min at maximum setting. Subsequently, 885 μl RNase-free H2O and 15 μl of protease K were added, and samples were then mixed and incubated at 55°C in a water bath for 10–11 min. Sample tubes were then spun at 10,000 × g for 3 min at room temperature and the supernatant transferred to RNase free tubes followed by addition of 0.5 vol. of Mol. Bio grade absolute EtOH. The samples were loaded onto RNeasy columns and the fibrous tissue protocol completed with on-column DNAse treatment and elution using two 30 μl additions of RNAse-free H2O. RNA concentrations were quantified by Nanodrop and RNA integrity demonstrated on bleach agarose gels (Aranda et al., 2012). For all samples, the large RNA subunit was clearly predominant relative to the smaller subunit, presenting the appearance of RNA previously demonstrated to have RIN values between 7 and 8. The final RNA samples were aliquoted, frozen in liquid N2, and stored at −80°C.
The QuantiTect Reverse Transcription Kit (Qiagen), which incorporates a second DNAse treatment, was used according to the manufacturer recommended protocol. This included 400 ng input RNA for each 10 μl RT reaction. All reactions were set up on ice to minimize the risk of RNA degradation. Control experiments without reverse transcriptase confirmed that the cDNA samples did not have any genomic sequence contamination as judged by the absence of target product in trans-intron qPCR reactions.
Analysis of mRNA levels was based on the CFX96 Real-Time PCR Detector and software (Biorad, Hercules CA). All qPCR reactions used cDNA derived from 5 ng of reverse transcribed total RNA. Multiple primer pairs were tested to establish the most reliable primers for each receptor subtype based on PCR efficiency, linearity within the analytic range, and localization within essential coding sequence in the second exon of common subtype isoforms. The best primer pair for each subtype positioned within the essential coding sequence in the second exon of common isoforms (Hawrylyshyn et al., 2004) was used for qPCR quantitation. These primers included those targeted to: adra1a Forward, ATACCCATGCTCCAGTCAAG; Reverse, GTGTTTGGAGGACTGCTTTC. adra1b Forward, TGTTCAAGGTGGTCTTCTGG; Reverse, GCTTGAACTCCTTGCTGGA. adra1d Forward, AGGGCGTCTTCAAGGTTATC; Reverse, CAGGGGTAGATGAGTGGATT. GAPDH, Forward, TGGAAATGTATGGAGGTCGG; Reverse, GGAAGAGAGAGTTCCTCAGC.
Initial experiments included both β-actin and GAPDH as potential internal load corrections; however, all applications of the ∆∆Ct approach produced increased variance consequently, normalization was to input cDNA (derived from 5 ng total RNA), and statistical analysis was based on raw, average Ct values produced by three qPCR quantifications on separate days. For each experiment, singly frozen sub-aliquots of sheep genomic DNA (Amsbio LLC) were used as a reference to estimate transcript copies per cell for each of the three α1-AR subtypes. Quantitative estimates of copy number were based on ∆Ct from 316 double strand copies assuming one cycle represented a two-fold change in copy number. Estimates of mRNA copies per cell are based on the assumption of 20 pg total RNA per cell, which implies 5 ng input total RNA representing ~250 cells.
Cytosolic calcium was measured in pulmonary arterial myocytes in situ using an en face preparation in balanced salt solution at the Loma Linda University, as we have recently described (Goyal et al., 2011; Papamatheakis et al., 2011; Blum-Johnston et al., 2016). In brief, tissues were loaded with the Ca2+ sensitive dye Fluo-4 AM (10 μM, Invitrogen, Carlsbad, CA, USA) with 0.1% pluronic F127 for 1.5–2 h at room temperature in the dark and measurements made with a Zeiss 710 NLO laser scanning confocal imaging workstation (Thornwood, NY) mounted to an inverted microscope (Zeiss AxioObserver A1). Arterial segments were then washed for 30 min, cut into linear strips, pinned to sylgard blocks (Ellsworth Adhesives, Germantown, WI), placed in an open bath imaging chamber (Warner Instruments, Hamden, CT), and mounted en face on the confocal imaging stage. Arteries were perfused at approximately 1 ml/min using a peristaltic pump (Rainin, Oakland, CA) with an electronic pinch valve system (Automate Scientific, Berkeley, CA). Cells were illuminated at 488 nm with a krypton argon laser, and the emitted light was collected using a photomultiplier tube (frames of 512 × 512 pixels) and a prismatic defractor (wavelengths of 493–622 nm). Full frame images were generated every 1 s. The pinhole was adjusted to provide an imaging depth of ~10 μm, which is roughly equivalent to the width of two cells (Goyal et al., 2011; Papamatheakis et al., 2011). Images were acquired at a 16-bit sampling depth using a non-immersion 20× Plan Apochromat (numerical aperture, NA 0.8). Regions of interest were examined post hoc, and although attempts were made to analyze, the oscillations in fluorescent intensity from recordings using LCPro quantification could not be adequately performed due to significant vasomotion during PE stimulation (Francis et al., 2012, 2014). Because of the substantial vessel reactivity, the numbers of arterial myocytes with Ca2+ events due to phenylephrine were recorded and the percentage of active myocytes calculated by comparing to the numbers of cells with observable Flou-4 fluorescence in the region of interest (Goyal et al., 2011; Papamatheakis et al., 2011). The number of observable cells examined for Figure 6C in each 1,000 μm2 region were as follows: Adult Normoxic, AN, 6.6 ± 0.3; Adult Hypoxic, AH, 8.3 ± 0.6; Fetal Normoxic, FN, 7.6 ± 0.4; Fetal Hypoxic, FH, 7.0 ± 0.2.
Most reagents and chemicals were purchased from Sigma-Aldrich (St. Louis, MO) except dexmedetomidine which was purchased from Tocris and other chemicals and reagents as noted.
All time-series recordings were graphed, and statistical analyses were made using GraphPad Prism 5.0 (La Jolla, CA) with the data presented as mean ± S.E.M. Data for bar plots were examined for normality with a D’Agostino and Pearson omnibus normality test. For most contractility, confocal, and PCR studies, comparisons were made within and among groups using either a two-way ANOVA and Bonferroni post hoc analyses or a one-way ANOVA with a with a Newman-Keuls Multiple Comparison Test. Categorical data shown in Figure 6C were analyzed by a chi-square test. Dose-response curves were fitted in Prism 5.0 using a Hill equation (Goyal et al., 2011; Papamatheakis et al., 2011; Blum-Johnston et al., 2016). Comparisons of the non-linear fit data for the influence of yohimbine on serotonin contraction, Figure 3A were examined by an extra sum of squares F-test. The N values reported reflect the total number of regions of interest, arterial segments, and/or total number of sheep tested as delineated elsewhere in the methods, figure legends, and Table 1. p < 0.05 was accepted as statistically significant.
The first series of experiments were designed to compare the effects of LTH in the fetal and adult periods on sympathetic-induced contractions of pulmonary arteries. This was achieved by examining the influence of cumulative doses of the selective α-AR agonist phenylephrine on contractions of pulmonary vascular rings isolated from adult and fetal sheep housed in either normoxic or LTH conditions. Phenylephrine was chosen in lieu of norepinephrine because the latter activates β-ARs that are expressed in pulmonary arteries (Hyman and Kadowitz, 1989; Magnenant et al., 2003). Figure 1 depicts summarized data for dose-response curves that were produced from these experiments. For all groups, arterial contractions in response to PE displayed a sigmoidal dose-response relationship (Figure 1A) and similar EC50’s (Figure 1C), indicating that neither development nor LTH modified the potency of PE. However, the maximum contraction relative to high potassium in the adult period for normoxic sheep was significantly reduced relative to fetus as shown in Figure 1B. While LTH had no effect on fetal pulmonary arterial contractions, it elevated the maximum contraction in the adult period to near fetal levels as illustrated in Figure 1B and in the area under the curve as shown in Figure 1D. Although maximal contraction relative to TKmax was lower in adult compared to fetus (Figure 1B), the absolute contractile force due to phenylephrine was higher in the adult (Figure 1E).
Based on these results and the literature to date (Levy et al., 1995; MacLean and McCulloch, 1998; Magnenant et al., 2003; Oriowo et al., 2003; Gornemann et al., 2007), we performed a series of experiments in order to determine the contributions of α1- and α2-AR to pulmonary arterial contractions. This was achieved by repeating the dose-response curves for each of the four animal groups in the presence or absence of antagonists of α1-AR (10 nM prazosin) or α2-AR (100 nM yohimbine) (Schindler et al., 2004; Gornemann et al., 2009). Data for these studies are shown in Figure 2. Prazosin had a similar effect in all four groups (Figure 2), causing a substantial rightward shift in the dose-response relationships (Figures 2Aa–Da) that translated into a reduced potency for PE in all groups (Figures 2Ab–Db). In comparison, prazosin had little or no effect on efficacy (Figures 2Ac–Dc). Figures 2Ad–Dd show that the area under the contractile curve was not as sensitive to prazosin or yohimbine as compared to the potency. Yohimbine caused a significant rightward shift in the PE-mediated contractility curve only in vessels from the fetal normoxic group (Figure 1Ac) but had no effect on PE-mediated contractions of adult vessels (normoxic and hypoxic) or fetal hypoxic vessels. These results suggest that α2-ARs may contribute to sympathetic contraction before birth and that antenatal hypoxia and the adult period suppress this role.
Because yohimbine blocked PE-mediated contractions only in arteries from the normoxic fetal group, studies were performed to further delineate the potential influence of α2-AR activation on arterial contraction (Shebuski et al., 1987; Jantschak and Pertz, 2012) as well as relaxation (Magnenant et al., 2003) in the fetal normoxic group. Evaluation of vessel reactivity was achieved by stimulating normoxic fetal arteries with the selective α2-AR agonist, dexmedetomidine (Toyama et al., 2009). Figure 3A shows the dose-response curves for normoxic fetal arteries stimulated with increasing doses of dexmedetomidine in the absence or presence of yohimbine. Dexmedetomidine caused modest contractions with a maximal response of approximately one-fifth that induced by PE (Figure 2A). Yohimbine treatment shifted the dose-response curve for dexmedetomidine contraction by one-log to the right (−7.8 ± 0.25 vs. −6.9 ± 0.14, p < 0.05). This confirms that α2-ARs are involved in vasomotor tone in the fetal pulmonary circulation, and this effect can be mitigated by α2-AR antagonists like yohimbine.
We then sought to evaluate the role of α2-AR to cause further contraction as occurs in pre-stimulated pulmonary vasculature of dog (Shebuski et al., 1987) and rat aorta (Ok et al., 2016), or whether the arteries will relax as described in pig pulmonary arteries (Pepke-Zaba et al., 1993) and fetal sheep pulmonary vasculature (Magnenant et al., 2003). Figure 3B shows the dose-response curves of fetal normoxic arteries treated with incremental doses of dexmedetomidine that were pre-stimulated with 10 μM serotonin. Our results illustrate that serotonin precontracted arteries relaxed in response to dexmedetomidine, an effect which was blocked by yohimbine. Taken together, these results indicate that inhibition of α2-AR does not seem to have a significant impact on phenylephrine-induced contraction in vessels from hypoxic animals from the fetal or adult periods but preferentially attenuates contractions in fetal normoxic vessels. However, activation of α2-AR in fetal normoxic vessels can cause vessel relaxation when arteries have significant serotonergic pretone.
The roles of the different α1-AR subtypes to the contraction responses shown in Figures 1, 2 were further delineated by performing PE dose-response contraction studies in the presence or absence of subtype selective α1-AR antagonists. The antagonists were chosen based on previously shown selectivity for the three α1-AR subtypes, namely α1A-AR (100 nM WB 4101), α1B-AR (10 μM CEC), and α1D-AR (100 nM BMY 7378) (Goyal et al., 2010b, 2014). The results of these experiments are illustrated in Figure 4. Vessels from the fetal normoxic period were affected by the selective inhibitors of the three α1 subtypes, where the dose-response curves (Figure 4Aa) and corresponding EC50 (Figure 4Ab) were significantly rightshifted and the areas under the curve were decreased (Figure 4Ad). The efficacy of contraction (Figure 4Ac) was less sensitive than the other parameters, being reduced by inhibitors of α1B-AR and α1D-AR but not α1A-AR. Similarly, Figure 4B shows the influence of inhibitors on vessels from the fetal hypoxic period. All three inhibitors reduced the amplitude of contraction and area under the curve (Figures 4Ba,Bc,Bd). The potency of contraction, however, was rightshifted by inhibitors of α1A-AR and α1B-AR but not α1D-AR (Figure 4Bb).
Vessels from the adult normoxic period were sensitive to α1A-AR and α1B-AR but much less dependent on the α1D-AR. The EC50 was rightshifted by inhibitors of both α1A-AR and α1B-AR (Figures 4Ca,Cb) while efficacy of contraction and area under the curve were each reduced by inhibition of α1B-AR (Figures 4Cc,Cd). In comparison, contractility of arteries from the adult hypoxic period was affected by inhibitors of all three α1-AR receptors, but the effect for the α1D-AR was marginal. Although the amplitude was insensitive to the inhibitors (Figure 4Dc), the potency was rightshifted substantially by inhibitors of α1A-AR and α1B-AR and to a lesser extent by α1D-AR (Figures 4Da,Dc). The area under the curve was reduced by inhibitors of α1A-AR and α1B-AR but not α1D-AR (Figure 4Dd).
Subsequently, qPCR was used to investigate subtype expression to determine if these patterns account for the changes observed in our functional studies of pulmonary arteries (Figure 5 and Table 1). Analysis of α1A-AR expression showed that message levels of this adrenergic receptor subtype did not increase with hypoxia in fetal or adult vessels but did increase in the adult period relative to fetus as shown in Figure 5A by decreased Ct values and by the increase in fold change and mRNA copies per cell (Table 1). Statistical analysis was performed on raw Ct values because during assay development, attempts to apply the ∆∆Ct method using actin and/or GAPDH as internal controls were ineffective, as these corrections substantially increased variance. Further, GAPDH expression increased under hypoxic conditions in the fetus (Figure 5D and Table 1), much as occurs in bovine endothelial cells (Graven et al., 1994; Graven and Farber, 1995). Of note, the tight clustering of GAPDH measurements for the normoxic adult suggest the variability observed in the other sample groupings reflects real differences in subunit expression. As for the α1A-AR, the α1B-AR and α1D-AR increased expression in the adult period relative to the fetus (Figures 5B,C and Table 1). The only significant difference due to hypoxia was an approximately 2.5-fold increase observed for α1D-AR in adult vessels.
The final series of studies examined the ability of PE to cause Ca2+ responses in myocytes that line the arterial wall. These studies were performed using an en face preparation with confocal laser scanning microscopy approaches such as we have used previously to examine serotonergic as well as caffeine-associated stimulation of Ca2+ responses in pulmonary arterial myocytes (Goyal et al., 2011; Papamatheakis et al., 2011; Hadley et al., 2012). In the present study, cytosolic Ca2+ of the pulmonary arterial myocytes was monitored under unstimulated conditions and in response to addition of 10 μM PE. These studies were performed based on our prior experiments where PE caused cytosolic Ca2+ elevations in isolated myocytes from pulmonary arteries of fetal and adult sheep (Goyal et al., 2008). Moreover, these Ca2+ responses are likely coupled to pulmonary arterial contractions (Goyal et al., 2010b, 2011, 2014; Papamatheakis et al., 2011). The Ca2+-signaling behavior of the regions of interest are expressed as the average baseline-subtracted fractional fluorescence intensity tracing (F/Fo) over time and shown in the time series graphs in Figures 6A,B in the presence of extracellular Ca2+. The bar above the tracing in Figure 6B shows when 10 μM PE was applied to the tissue. The images in Figures 6A,B show in situ fluorescence micrographs of myocytes in the arterial wall isolated from normoxic fetal sheep for the time points as shown by the arrows in unstimulated (Figure 6A) and phenylephrine-stimulated (Figure 6B) conditions for the fluorescence intensity tracings in the panels shown on the right. Regions of interest are denoted by the small colored circles in the image panels and represent subcellular regions within individual myocytes that correspond to associated fluorescent time series data traces of the same color in Figures 6A,B. Figure 6A illustrates that under unstimulated conditions, the Ca2+ oscillations are stochastic, occurring randomly during the recording. Figure 6B shows that stimulation with 10 μM PE causes deterministic Ca2+ responses among the arterial myocytes. Figure 6C shows the number of responsive cells in the arterial wall for the data recordings as determined by examining the number of myocytes with calcium responses in 33 μm × 33 μm boxes (1,000 μm2). The figure shows that there is some variability in the percentage of myocytes with Ca2+ oscillations under control conditions and in response to phenylephrine stimulation, though there are no significant differences due to stimulation or in the fetal or adult periods.
The present study provides new information systematically comparing the effects of LTH in the fetal and adult periods on the functional changes for the role of α1- and α2-adrenergic receptors in the pulmonary vasculature. The contractile response to phenylephrine underwent significant changes from the fetal to adult periods and LTH caused further alterations. Of note, α2-ARs appeared to be important to vascular reactivity before birth in the normoxic fetal period; however, these α2-AR effects were not present in the adult period and were lost in fetuses exposed to LTH. We found there were significant roles for α1A- and α1B-ARs in pulmonary arterial contractions of all groups examined. There was also a lesser role for α1D-AR in the fetus that was reduced by hypoxia and largely absent in the adult period. Developmentally, the contractile properties produced by adrenergic stimulation are complex and take place in the context of changing vessel behavior due to LTH exposure.
Developmental changes in adrenergic signaling of pulmonary arteries were expected given the dramatically different role these arteries play during the fetal period where blood flow is restricted as compared to the adult period where robust blood flow is required to exchange gasses. Given the need to maintain oxygenation in stressful situations, it is not surprising that adrenergic contractions during the adult period lessen relative to contractions due to depolarization with high K+. A similar reduction in adrenergic mediated contractions in the adult period occurs in the piglet, which has reduced norepinephrine mediated pulmonary vascular contractions following birth (Schindler et al., 2004). In this regard, our data showed that pulmonary vessels in adult sheep lost the contractile effects from both α2-AR activity and coupling to the α1D-AR, which were observed in the normoxic fetal period. Nevertheless, this relative decrease in contractility must be viewed in context as the absolute contractile force due to PE increased following birth. It is reasonable to suggest that the increased absolute force caused by PE is partly mediated through an increased expression of the α1A- and α1B-ARs. While it appears contradictory for the α1D-AR to play a minimal role in contractions of adult vessels given the 2.5-fold increases in its mRNA expression, α1D-AR levels do not necessarily correlate with arterial reactivity in arteries and instead contribute significantly to vascular remodeling in hypoxia-related pulmonary hypertension (Faber et al., 2007).
Because autonomic innervation of the lung likely increases after birth, our data suggest that postnatal maturation may result in other functional changes (Garcia-Sainz et al., 2001; Thiriet, 2013; Castillo-Badillo et al., 2015). Indeed, the process by which maturity into adulthood affects adrenergic dependent vascular contractility may be complex. One possibility is that during the adult period the increased sympathetic nerve stimulation may cause α adrenergic receptor desensitization and downregulation (Garcia-Sainz et al., 2001; Castillo-Badillo et al., 2015). Another explanation for decrements relative to high K+ during the adult period is that the sympathetic nerve supply may be trimmed. This tenant is supported by the lack of a profound difference in cellular Ca2+ signaling activity in fetal relative to adult pulmonary arterial myocytes.
The LTH-induced enhancement of phenylephrine reactivity in pulmonary arteries of the adult period was expected. Chronic hypoxia in piglets prevents postbirth decreases in adrenergic tone (Schindler et al., 2004) and has been linked to hypoxia-induced pulmonary hypertension in multiple animal species including sheep (Llanos et al., 2011). This tie between adrenergic signaling and hypoxia-related pulmonary hypertension may be due to various factors. Hypertrophy of the smooth muscle layer in the small pulmonary arteries and arterioles appears to be a major determinant of pulmonary vascular responses to hypoxia in humans and other species (Tucker et al., 1975). Potentially, the thickness of the smooth muscle layer may be linked to the adrenergic receptor density and strength of stimulation (Xiao et al., 2003) and LTH in the fetal and adult periods may enhance adrenergic-mediated cellular hypertrophy and proliferation as occurs in cultured rat pulmonary arteries (Faber et al., 2006). The influence of LTH on adrenergic stimulation may be similar to chronic intermittent hypoxia, which increases sympathetic nervous system outflow from the brainstem (Zoccal et al., 2008). Neurohumoral modulatory pathways influenced by sympathetic stimulation include downregulation of nitric oxide (NO) signaling in the brain (Patel et al., 2001) and upregulation of endothelin-1 (Huang et al., 2010). Furthermore, physiological as well as psychological and environmental stresses are known to increase sympathetic nerve activity (Anderson et al., 1991). Chronic intermittent hypoxia also elicits chemosensory plasticity, including increased basal discharge, enhanced hypoxic sensitivity, and sensory long-term facilitation (Peng et al., 2003). Even still, the effect of LTH in the fetal and adult periods in sheep is distinct from the adaptive responses in chick embryos. Chronic hypoxia in 19-day-old chick embryos reduces pulmonary arterial contractions due to high K+, norepinephrine, U46119, and endothelin-1, although there is no difference in the perivascular innervation density before birth and contraction deficits resolve after birth (Villamor et al., 2004). These distinctions in prenatal programming effects between fetal sheep versus chick embryos are not unfounded and follow from studies by us and others in the cardiovascular systems of these models (Crossley et al., 2003; Villamor et al., 2004; Goyal et al., 2011; Blum-Johnston et al., 2016; Giang et al., 2016).
Adrenergic contraction of fetal pulmonary vessels involves all three α1-AR subtypes and was efficiently coupled to the contraction as PE stimulation produced about the same force as high K+. The role for α2-adrenoreceptors in the fetus was unanticipated as PE is fairly selective for the α1-AR; however, α2-ARs appear to be important for sympathetic pulmonary arterial contraction before birth and antenatal LTH attenuates their role in fetal vessels. Prior studies indicated that α2-ARs were expressed in the pulmonary vasculature and involved in arterial contractions, even before birth (Magnenant et al., 2003; Jantschak and Pertz, 2012). Much like our studies, postnatal maturation seems to contribute to downregulation of α2-AR-mediated responses (Starke, 2001).
Previous in vitro studies showed that vasodilation due to α2-AR activation resulted from endothelial NO release. NO-synthase inhibition not only abolished pulmonary vasodilator effects of α2-AR agonists but also unmasked their pulmonary vasoconstrictive function in rabbit pulmonary arteries where norepinephrine induced contractions were augmented through α2-AR-mediated mechanisms (MacLean et al., 1993). As α2-AR agonists may induce pulmonary arterial myocyte contraction (Pepke-Zaba et al., 1993) in addition to relaxation, it is possible that pulmonary vascular responses to α2-AR result from the balance between the activation of α1- and α2-AR-induced smooth muscle cell contraction and endothelial α2-AR-mediated vasodilation (Magnenant et al., 2003). Though further experiments will be required to directly address the α2-AR-mediated dilation we report in the sheep fetal vessels, we do not believe that this is due to incomplete disruption of the endothelium because bradykinin and acetylcholine have little or no effect on pulmonary arterial reactivity (Blum-Johnston et al., 2016; Giang et al., 2016). Rather, preferential α2-AR reactivity in the normoxic fetus and not in other groups suggests that the role for this receptor is labile and greatly influenced by LTH in the fetal period and by postnatal development.
The simplest explanation for α2-AR-induced contractile effects is that stimulation of smooth muscle α2-ARs inhibits cAMP production via Gi coupling resulting in increased arterial reactivity. Indeed, there is precedent for direct α2-AR-dependent contraction in porcine pulmonary arteries where α2c-ARs mediate myocyte contraction when the cytosolic Ca2+ concentration is elevated. In these arteries, norepinephrine activates α2c-AR following α1-AR-stimulation and concomitant myocyte Ca2+ increases (Jantschak and Pertz, 2012). Such findings are not restricted to the pulmonary vasculature. In nasal mucosa blood vessels of pigs, which are richly innervated by sympathetic nerves, neurogenic vasomotor contractility is largely regulated through postjunctional α2-ARs (Corboz et al., 2013). The relaxation of serotonin preconstricted arteries by α2-AR activation may therefore be explained by varied expression of α2-AR subtypes or by postjunctional mechanisms. For example, in the pregnant rat myometrium, α2A- and α2C-AR activation causes myometrial relaxation, while α2B-AR stimulation elicits contraction. Thus, it is quite possible there are multiple α2-AR subtypes expressed in the pulmonary vasculature that serve distinct roles in regulating arterial relaxation and contraction, which are modified differentially by LTH in the fetal and adult periods (Gaspar et al., 2007). Overall, the current experiments support previous studies suggesting that α2-ARs may modulate pulmonary vascular tone and that LTH influences receptor function in sheep vessels (Magnenant et al., 2003; Schindler et al., 2004). Further investigation is required to fully delineate the importance of α2-ARs and the roles of prejunctional receptors on perivascular nerves as well as postjunctional receptors on smooth muscle or endothelium.
The present study evaluating α-adrenergic stimulation of the pulmonary vasculature parallels recent studies from our group focused on the cerebral vasculature that compared the effects of maturation and LTH in the fetal and adult periods (Goyal et al., 2010b, 2014). The present study delineates that all three α1-AR subtypes are important to normoxic fetal sheep pulmonary arterial reactivity. This compares with normoxic fetal sheep cerebral arteries in which only the α1B and α1D-AR regulate arterial contraction (Goyal et al., 2010b). The importance of α1-AR subtypes in sheep adult cerebral vessels is also distinct from adult pulmonary vessels. Adult normoxic cerebral vessels are dependent on all three receptor subtypes, while LTH causes pronounced loss in α1D-AR function. Adult normoxic pulmonary arteries in comparison are reliant on α1A and α1B-AR but not α1D-AR activity, and their relative roles are not greatly influenced by LTH. Overall, LTH appears to cause cerebral vessels to become more pulmonary-like in regards to the importance of α1-adrenergic receptor subtypes to arterial reactivity (Goyal et al., 2014).
Phenylephrine-induced Ca2+ signals in individual myocytes within intact arteries were examined to better understand the cellular mechanisms linking α1-AR stimulation to vascular reactivity. LTH in the fetal and adult periods caused complex changes in the Ca2+ signals. The modest phenylephrine Ca2+ activity responses in myocytes of pulmonary arteries from the fetal and adult periods together with the decrease in arterial reactivity relative to high K+ in the adult period suggest that arterial contractions in the fetal period are more sensitized to changes in the cytosolic Ca2+ concentration. Further, given the increase in arterial reactivity but lack of change in Ca2+ activity of arterial myocytes LTH may enhance the Ca2+ sensitivity in arterial myocytes from the adult period. Although not examined in the current study, previous work from our group illustrates that there are several intracellular signaling pathways that may be important to changes in the Ca2+ sensitivity. Previously, we have shown that rho-kinase is critical to serotonergic and depolarization-induced reactivity in sheep pulmonary arteries (Papamatheakis et al., 2012; Blood et al., 2013). Protein kinase C (PKC) and extracellular regulated kinase (ERK) pathways are also potentially involved as they are controlled by adrenergic receptor activity in cerebral and uterine arteries of sheep (Xiao et al., 2010; Goyal et al., 2010b). In addition, in cerebral arteries from sheep, the different adrenergic receptor isoforms make varied linkages to Ca2+, PKC, and ERK pathways (Longo et al., 1996; Ueno et al., 1997; Goyal et al., 2010a,b), which can be modified in unique ways by LTH in the fetal and adult vessels. Elucidating the nuances associated with the changes due to LTH in the fetal and adult periods for the Ca2+ sensitization pathways was not addressed in these initial series of studies. However, based on our previous comparisons to vessels from other vascular beds in sheep, we anticipate the mechanistic modifications due to postnatal maturity and LTH to be distinct in the pulmonary vasculature.
The molecular and signaling underpinnings of pulmonary vascular disease are complex. The evidence provided supports the vital role of LTH in the development of adrenergic-induced pulmonary hypertension and the variation in responsiveness due to development. Further, the data substantiate early studies suggesting that adrenergic receptor antagonists can be used to manage the disease (Oriowo et al., 2003) in addition to recent porcine and canine studies (Rothman et al., 2015; Zhou et al., 2015) and a human clinical trial (Chen et al., 2015) focusing on histological, hemodynamic, functional, and clinical benefits to denervation of the pulmonary vasculature. The long-term benefits of the present findings relate to the therapeutic potential of targeting adrenergic signaling in ways that curtail detriments in pulmonary vascular function due to high altitude or other mechanisms that enhance sympathetic drive.
The datasets generated for this study are available on request to the corresponding author.
This study was carried out in accordance with the recommendations of the regulations of the Animal Welfare Act, the National Institutes of Health Guide for the Care and Use of Laboratory Animals, “The Guiding Principles in the Care and Use of Animals” approved by the Council of the American Physiological Society. The protocol was approved by the Animal Care and Use Committee of Loma Linda University (LLU).
SW, LL, DP, LZ, and DPM conceived and designed the research. DP, DPM, QB, SM, MoR, and SV performed the experiments. DP, DPM, DM, QB, SM, MaR, MoR, PG, SV, and SL analyzed data. DM, DP, DPM, QB, SM, MaR, MoR, and SL prepared figures. DM, DP, DPM, PG, LL, LZ, and SW drafted the manuscript. All authors interpreted results of experiments, and with the exception of LL, edited, revised, and approved the final version of manuscript.
A portion of this material was performed in the Loma Linda University School of Medicine Advanced Imaging and Microscopy Core that is supported by the National Science Foundation under Major Research Instrumentation, Division of Biological Infrastructure Grant No. 0923559 (SW) and the Loma Linda University School of Medicine. The work was also supported in part by the USPHS Grants NIH R03HD069746 (SW), P01HD031226 and R01HD003807 (LL), and P01HD083132 (LZ).
The authors declare that the research was conducted in the absence of any commercial or financial relationships that could be construed as a potential conflict of interest.
We would like to acknowledge Travis Merritt and Rachael Wilson for expert technical assistance.
Allison, B. J., Brain, K. L., Niu, Y., Kane, A. D., Herrera, E. A., Thakor, A. S., et al. (2016). Fetal in vivo continuous cardiovascular function during chronic hypoxia. J. Physiol. 594, 1247–1264. doi: 10.1113/JP271091
Anderson, E. A., Sinkey, C. A., and Mark, A. L. (1991). Mental stress increases sympathetic nerve activity during sustained baroreceptor stimulation in humans. Hypertension 17, III43–III49. doi: 10.1161/01.hyp.17.4_suppl.iii43
Aranda, P. S., Lajoie, D. M., and Jorcyk, C. L. (2012). Bleach gel: a simple agarose gel for analyzing RNA quality. Electrophoresis 33, 366–369. doi: 10.1002/elps.201100335
Barnes, P. J., and Liu, S. F. (1995). Regulation of pulmonary vascular tone. Pharmacol. Rev. 47, 87–131.
Blood, A. B., Terry, M. H., Merritt, T. A., Papamatheakis, D. G., Blood, Q., Ross, J. M., et al. (2013). Effect of chronic perinatal hypoxia on the role of rho-kinase in pulmonary artery contraction in newborn lambs. Am. J. Physiol. Regul. Integr. Comp. Physiol. 304, R136–R146. doi: 10.1152/ajpregu.00126.2012
Blum-Johnston, C., Thorpe, R. B., Wee, C., Romero, M., Brunelle, A., Blood, Q., et al. (2016). Developmental acceleration of bradykinin-dependent relaxation by prenatal chronic hypoxia impedes normal development after birth. Am. J. Physiol. Lung Cell. Mol. Physiol. 310, L271–L286. doi: 10.1152/ajplung.00340.2015
Castillo-Badillo, J. A., Sanchez-Reyes, O. B., Alfonzo-Mendez, M. A., Romero-Avila, M. T., Reyes-Cruz, G., and Garcia-Sainz, J. A. (2015). Alpha1B-adrenergic receptors differentially associate with Rab proteins during homologous and heterologous desensitization. PLoS One 10:e0121165. doi: 10.1371/journal.pone.0121165
Chen, S. L., Zhang, H., Xie, D. J., Zhang, J., Zhou, L., Rothman, A. M., et al. (2015). Hemodynamic, functional, and clinical responses to pulmonary artery denervation in patients with pulmonary arterial hypertension of different causes: phase II results from the pulmonary artery denervation-1 study. Circ. Cardiovasc. Interv. 8:e002837. doi: 10.1161/CIRCINTERVENTIONS.115.002837
Corboz, M. R., Rivelli, M. A., Shah, H., Boyce, C. W., Mccormick, K. D., Chapman, R. W., et al. (2013). Role of alpha2-adrenoceptors in electrical field stimulation-induced contraction of pig nasal mucosa and pharmacologic characterization of a novel alpha2C-adrenoceptor agonist. Am. J. Rhinol. Allergy 27, 84–90. doi: 10.2500/ajra.2013.27.3842
Crossley, D. A. 2nd, Burggren, W. W., and Altimiras, J. (2003). Cardiovascular regulation during hypoxia in embryos of the domestic chicken Gallus gallus. Am. J. Physiol. Regul. Integr. Comp. Physiol. 284, R219–R226. doi: 10.1152/ajpregu.00654.2001
Daly, I. D. B., and Hebb, C. (1966). Pulmonary and bronchial vascular systems: Their reactions under controlled conditions of ventilation and circulation. London: Edward Arnold.
Ducsay, C. A., Goyal, R., Pearce, W. J., Wilson, S., Hu, X. Q., and Zhang, L. (2018). Gestational hypoxia and developmental plasticity. Physiol. Rev. 98, 1241–1334. doi: 10.1152/physrev.00043.2017
Faber, J. E., Szymeczek, C. L., Cotecchia, S., Thomas, S. A., Tanoue, A., Tsujimoto, G., et al. (2007). Alpha1-adrenoceptor-dependent vascular hypertrophy and remodeling in murine hypoxic pulmonary hypertension. Am. J. Physiol. Heart Circ. Physiol. 292, H2316–H2323. doi: 10.1152/ajpheart.00792.2006
Faber, J. E., Szymeczek, C. L., Salvi, S. S., and Zhang, H. (2006). Enhanced alpha1-adrenergic trophic activity in pulmonary artery of hypoxic pulmonary hypertensive rats. Am. J. Physiol. Heart Circ. Physiol. 291, H2272–H2281. doi: 10.1152/ajpheart.00404.2006
Francis, M., Qian, X., Charbel, C., Ledoux, J., Parker, J. C., and Taylor, M. S. (2012). Automated region of interest analysis of dynamic Ca(2)+ signals in image sequences. Am. J. Physiol. Cell Physiol. 303, C236–C243. doi: 10.1152/ajpcell.00016.2012
Francis, M., Waldrup, J., Qian, X., and Taylor, M. S. (2014). Automated analysis of dynamic Ca2+ signals in image sequences. J. Vis. Exp. e51560. doi: 10.3791/51560
Garcia-Sainz, J. A., Vazquez-Cuevas, F. G., and Romero-Avila, M. T. (2001). Phosphorylation and desensitization of alpha1d-adrenergic receptors. Biochem. J. 353, 603–610. doi: 10.1042/bj3530603
Gaspar, R., Gal, A., Galik, M., Ducza, E., Minorics, R., Kolarovszki-Sipiczki, Z., et al. (2007). Different roles of alpha2-adrenoceptor subtypes in non-pregnant and late-pregnant uterine contractility in vitro in the rat. Neurochem. Int. 51, 311–318. doi: 10.1016/j.neuint.2007.06.029
Giang, M., Papamatheakis, D. G., Nguyen, D., Paez, R., Blum Johnston, C., Kim, J., et al. (2016). Muscarinic receptor activation affects pulmonary artery contractility in sheep: the impact of maturation and chronic hypoxia on endothelium-dependent and endothelium-independent function. High Alt. Med. Biol. 17, 122–132. doi: 10.1089/ham.2015.0116
Gornemann, T., Villalon, C. M., Centurion, D., and Pertz, H. H. (2009). Phenylephrine contracts porcine pulmonary veins via alpha(1B)-, alpha(1D)-, and alpha(2)-adrenoceptors. Eur. J. Pharmacol. 613, 86–92. doi: 10.1016/j.ejphar.2009.04.011
Gornemann, T., Von Wenckstern, H., Kleuser, B., Villalon, C. M., Centurion, D., Jahnichen, S., et al. (2007). Characterization of the postjunctional alpha 2C-adrenoceptor mediating vasoconstriction to UK14304 in porcine pulmonary veins. Br. J. Pharmacol. 151, 186–194. doi: 10.1038/sj.bjp.0707221
Goyal, R., Creel, K. D., Chavis, E., Smith, G. D., Longo, L. D., and Wilson, S. M. (2008). Maturation of intracellular calcium homeostasis in sheep pulmonary arterial smooth muscle cells. Am. J. Physiol. Lung Cell. Mol. Physiol. 295, L905–L914. doi: 10.1152/ajplung.00053.2008
Goyal, R., Goyal, D., Chu, N., Van Wickle, J., and Longo, L. D. (2014). Cerebral artery alpha-1 AR subtypes: high altitude long-term acclimatization responses. PLoS One 9:e112784. doi: 10.1371/journal.pone.0112784
Goyal, R., Mittal, A., Chu, N., Arthur, R. A., Zhang, L., and Longo, L. D. (2010a). Maturation and long-term hypoxia-induced acclimatization responses in PKC-mediated signaling pathways in ovine cerebral arterial contractility. Am. J. Physiol. Regul. Integr. Comp. Physiol. 299, R1377–R1386. doi: 10.1152/ajpregu.00344.2010
Goyal, R., Mittal, A., Chu, N., Zhang, L., and Longo, L. D. (2010b). Alpha(1)-adrenergic receptor subtype function in fetal and adult cerebral arteries. Am. J. Physiol. Heart Circ. Physiol. 298, H1797–H1806. doi: 10.1152/ajpheart.00112.2010
Goyal, R., Papamatheakis, D. G., Loftin, M., Vrancken, K., Dawson, A. S., Osman, N. J., et al. (2011). Long-term maternal hypoxia: the role of extracellular Ca2+ entry during serotonin-mediated contractility in fetal ovine pulmonary arteries. Reprod. Sci. 18, 948–962. doi: 10.1177/1933719111401660
Graven, K. K., Troxler, R. F., Kornfeld, H., Panchenko, M. V., and Farber, H. W. (1994). Regulation of endothelial cell glyceraldehyde-3-phosphate dehydrogenase expression by hypoxia. J. Biol. Chem. 269, 24446–24453.
Hadley, S. R., Blood, Q., Rubalcava, M., Waskel, E., Lumbard, B., Le, P., et al. (2012). Maternal high-altitude hypoxia and suppression of ryanodine receptor-mediated Ca2+ sparks in fetal sheep pulmonary arterial myocytes. Am. J. Physiol. Lung Cell. Mol. Physiol. 303, L799–L813. doi: 10.1152/ajplung.00009.2012
Hawrylyshyn, K. A., Michelotti, G. A., Coge, F., Guenin, S. P., and Schwinn, D. A. (2004). Update on human alpha1-adrenoceptor subtype signaling and genomic organization. Trends Pharmacol. Sci. 25, 449–455. doi: 10.1016/j.tips.2004.06.011
Herrera, E. A., Pulgar, V. M., Riquelme, R. A., Sanhueza, E. M., Reyes, R. V., Ebensperger, G., et al. (2007). High-altitude chronic hypoxia during gestation and after birth modifies cardiovascular responses in newborn sheep. Am. J. Physiol. Regul. Integr. Comp. Physiol. 292, R2234–R2240. doi: 10.1152/ajpregu.00909.2006
Herrera, E. A., Riquelme, R. A., Ebensperger, G., Reyes, R. V., Ulloa, C. E., Cabello, G., et al. (2010). Long-term exposure to high-altitude chronic hypoxia during gestation induces neonatal pulmonary hypertension at sea level. Am. J. Physiol. Regul. Integr. Comp. Physiol. 299, R1676–R1684. doi: 10.1152/ajpregu.00123.2010
Huang, J., Xie, T., Wu, Y., Li, X., Lusina, S., Ji, E. S., et al. (2010). Cyclic intermittent hypoxia enhances renal sympathetic response to ICV ET-1 in conscious rats. Respir. Physiol. Neurobiol. 171, 83–89. doi: 10.1016/j.resp.2010.03.008
Hyman, A. L., and Kadowitz, P. J. (1989). Analysis of responses to sympathetic nerve stimulation in the feline pulmonary vascular bed. J. Appl. Physiol. 67, 371–376. doi: 10.1152/jappl.1989.67.1.371
Jantschak, F., and Pertz, H. H. (2012). Alpha2C-adrenoceptors play a prominent role in sympathetic constriction of porcine pulmonary arteries. Naunyn Schmiedeberg's Arch. Pharmacol. 385, 595–603. doi: 10.1007/s00210-012-0741-3
Kamitomo, M., Alonso, J. G., Okai, T., Longo, L. D., and Gilbert, R. D. (1993). Effects of long-term, high-altitude hypoxemia on ovine fetal cardiac output and blood flow distribution. Am. J. Obstet. Gynecol. 169, 701–707.
Kamitomo, M., Longo, L. D., and Gilbert, R. D. (1994). Cardiac function in fetal sheep during two weeks of hypoxemia. Am. J. Phys. 266, R1778–R1785. doi: 10.1152/ajpregu.1994.266.6.R1778
Levy, M., Tulloh, R. M., Komai, H., Stuart-Smith, K., and Haworth, S. G. (1995). Maturation of the contractile response and its endothelial modulation in newborn porcine intrapulmonary arteries. Pediatr. Res. 38, 25–29. doi: 10.1203/00006450-199507000-00005
Llanos, A. J., Ebensperger, G., Herrera, E. A., Reyes, R. V., Pulgar, V. M., Seron-Ferre, M., et al. (2011). Fetal and postnatal pulmonary circulation in the alto Andino. Placenta 32(Suppl. 2), S100–S103. doi: 10.1016/j.placenta.2011.01.001
Longo, L. D., Ueno, N., Zhao, Y., Zhang, L., and Pearce, W. J. (1996). NE-induced contraction, alpha 1-adrenergic receptors, and ins(1,4,5)P3 responses in cerebral arteries. Am. J. Phys. 270, H915–H923. doi: 10.1152/ajpheart.1996.270.3.H915
MacLean, M. R., and McCulloch, K. M. (1998). Influence of applied tension and nitric oxide on responses to endothelins in rat pulmonary resistance arteries: effect of chronic hypoxia. Br. J. Pharmacol. 123, 991–999. doi: 10.1038/sj.bjp.0701682
MacLean, M. R., McCulloch, K. M., and McGrath, J. C. (1993). Influences of the endothelium and hypoxia on alpha 1- and alpha 2-adrenoceptor-mediated responses in the rabbit isolated pulmonary artery. Br. J. Pharmacol. 108, 155–161. doi: 10.1111/j.1476-5381.1993.tb13456.x
Magnenant, E., Jaillard, S., Deruelle, P., Houfflin-Debarge, V., Riou, Y., Klosowski, S., et al. (2003). Role of the alpha2-adrenoceptors on the pulmonary circulation in the ovine fetus. Pediatr. Res. 54, 44–51. doi: 10.1203/01.PDR.0000065726.43910.91
Mandel, J., and Taichman, D. (2006). Pulmonary vascular disease. Philadelphia, PA: Saunders Elsevier.
McGillick, E. V., Orgeig, S., Allison, B. J., Brain, K. L., Niu, Y., Itani, N., et al. (2017). Maternal chronic hypoxia increases expression of genes regulating lung liquid movement and surfactant maturation in male fetuses in late gestation. J. Physiol. 595, 4329–4350. doi: 10.1113/JP273842
Michel, M. C., Wieland, T., and Tsujimoto, G. (2009). How reliable are G-protein-coupled receptor antibodies? Naunyn Schmiedeberg's Arch. Pharmacol. 379, 385–388. doi: 10.1007/s00210-009-0395-y
Niermeyer, S. (2007). Going to high altitude with a newborn infant. High Alt. Med. Biol. 8, 117–123. doi: 10.1089/ham.2007.1068
Ogata, M., Ohe, M., Katayose, D., and Takishima, T. (1992). Modulatory role of EDRF in hypoxic contraction of isolated porcine pulmonary arteries. Am. J. Phys. 262, H691–H697. doi: 10.1152/ajpheart.1992.262.3.H691
Ok, S. H., Kwon, S. C., Baik, J., Hong, J. M., Oh, J., Han, J. Y., et al. (2016). Dexmedetomidine-induced contraction involves CPI-17 phosphorylation in isolated rat aortas. Int. J. Mol. Sci. 17:1663. doi: 10.3390/ijms17101663
Oriowo, M. A., Chandrasekhar, B., and Kadavil, E. A. (2003). Alpha 1-adrenoceptor subtypes mediating noradrenaline-induced contraction of pulmonary artery from pulmonary hypertensive rats. Eur. J. Pharmacol. 482, 255–263. doi: 10.1016/j.ejphar.2003.10.001
Papamatheakis, D. G., Blood, A. B., Kim, J. H., and Wilson, S. M. (2013). Antenatal hypoxia and pulmonary vascular function and remodeling. Curr. Vasc. Pharmacol. 11, 616–640. doi: 10.2174/1570161111311050006
Papamatheakis, D. G., Patel, J. J., Blood, Q., Merritt, T. T., Longo, L. D., and Wilson, S. M. (2012). Depolarization-dependent contraction increase after birth and preservation following long-term hypoxia in sheep pulmonary arteries. Pulm. Circ. 2, 41–53. doi: 10.4103/2045-8932.94832
Papamatheakis, D. G., Vemulakonda, S., Blood, Q., Goyal, R., Rubalcava, M., Vrancken, K., et al. (2011). Preservation of serotonin-mediated contractility in adult sheep pulmonary arteries following long-term high-altitude hypoxia. High Alt. Med. Biol. 12, 253–264. doi: 10.1089/ham.2010.1076
Patel, K. P., Li, Y. F., and Hirooka, Y. (2001). Role of nitric oxide in central sympathetic outflow. Exp. Biol. Med. 226, 814–824. doi: 10.1177/153537020122600902
Peng, Y. J., Overholt, J. L., Kline, D., Kumar, G. K., and Prabhakar, N. R. (2003). Induction of sensory long-term facilitation in the carotid body by intermittent hypoxia: implications for recurrent apneas. Proc. Natl. Acad. Sci. USA 100, 10073–10078. doi: 10.1073/pnas.1734109100
Pepke-Zaba, J., Higenbottam, T. W., Dinh-Xuan, A. T., Ridden, C., and Kealey, T. (1993). Alpha-adrenoceptor stimulation of porcine pulmonary arteries. Eur. J. Pharmacol. 235, 169–175. doi: 10.1016/0014-2999(93)90133-3
Rothman, A. M., Arnold, N. D., Chang, W., Watson, O., Swift, A. J., Condliffe, R., et al. (2015). Pulmonary artery denervation reduces pulmonary artery pressure and induces histological changes in an acute porcine model of pulmonary hypertension. Circ. Cardiovasc. Interv. 8:e002569. doi: 10.1161/CIRCINTERVENTIONS.115.002569
Salvi, S. S. (1999). Alpha1-adrenergic hypothesis for pulmonary hypertension. Chest 115, 1708–1719. doi: 10.1378/chest.115.6.1708
Schindler, M. B., Hislop, A. A., and Haworth, S. G. (2004). Postnatal changes in response to norepinephrine in the normal and pulmonary hypertensive lung. Am. J. Respir. Crit. Care Med. 170, 641–646. doi: 10.1164/rccm.200311-1551OC
Schonbrunn, A. (2014). Editorial: antibody can get it right: confronting problems of antibody specificity and irreproducibility. Mol. Endocrinol. 28, 1403–1407. doi: 10.1210/me.2014-1230
Shebuski, R. J., Ohlstein, E. H., Smith, J. M. Jr., and Ruffolo, R. R. Jr. (1987). Enhanced pulmonary alpha-2 adrenoceptor responsiveness under conditions of elevated pulmonary vascular tone. J. Pharmacol. Exp. Ther. 242, 158–165.
Shen, C. P., Romero, M., Brunelle, A., Wolfe, C., Dobyns, A., Francis, M., et al. (2018). Long-term high-altitude hypoxia influences pulmonary arterial L-type calcium channel-mediated Ca(2+) signals and contraction in fetal and adult sheep. Am. J. Physiol. Regul. Integr. Comp. Physiol. 314, R433–R446. doi: 10.1152/ajpregu.00154.2017
Starke, K. (2001). Presynaptic autoreceptors in the third decade: focus on alpha2-adrenoceptors. J. Neurochem. 78, 685–693. doi: 10.1046/j.1471-4159.2001.00484.x
Thiriet, M. (2013). Anatomy and physiology of the circulatory and ventilatory systems. New York: Springer.
Toyama, H., Wagatsuma, T., Ejima, Y., Matsubara, M., and Kurosawa, S. (2009). Cesarean section and primary pulmonary hypertension: the role of intravenous dexmedetomidine. Int. J. Obstet. Anesth. 18, 262–267. doi: 10.1016/j.ijoa.2008.08.001
Tucker, A., Mcmurtry, I. F., Reeves, J. T., Alexander, A. F., Will, D. H., and Grover, R. F. (1975). Lung vascular smooth muscle as a determinant of pulmonary hypertension at high altitude. Am. J. Phys. 228, 762–767.
Ueno, N., Zhao, Y., Zhang, L., and Longo, L. D. (1997). High altitude-induced changes in alpha1-adrenergic receptors and ins(1,4,5)P3 responses in cerebral arteries. Am. J. Phys. 272, R669–R674. doi: 10.1152/ajpregu.1997.272.2.R669
Villamor, E., Kessels, C. G., Ruijtenbeek, K., Van Suylen, R. J., Belik, J., De Mey, J. G., et al. (2004). Chronic in ovo hypoxia decreases pulmonary arterial contractile reactivity and induces biventricular cardiac enlargement in the chicken embryo. Am. J. Physiol. Regul. Integr. Comp. Physiol. 287, R642–R651. doi: 10.1152/ajpregu.00611.2003
Weir, E. K., Archer, S. L., and Reeves, J. T. (2000). The fetal and neonatal pulmonary circulations. Armonk, NY: Futura Pub.
Xiao, D., Huang, X., Longo, L. D., and Zhang, L. (2010). PKC regulates alpha(1)-adrenoceptor-mediated contractions and baseline Ca(2+) sensitivity in the uterine arteries of nonpregnant and pregnant sheep acclimatized to high altitude hypoxia. High Alt. Med. Biol. 11, 153–161. doi: 10.1089/ham.2009.1076
Xiao, D., Huang, X., Pearce, W. J., Longo, L. D., and Zhang, L. (2003). Effect of cortisol on norepinephrine-mediated contractions in ovine uterine arteries. Am. J. Physiol. Heart Circ. Physiol. 284, H1142–H1151. doi: 10.1152/ajpheart.00834.2002
Xue, Q., Ducsay, C. A., Longo, L. D., and Zhang, L. (2008). Effect of long-term high-altitude hypoxia on fetal pulmonary vascular contractility. J. Appl. Physiol. 104, 1786–1792. doi: 10.1152/japplphysiol.01314.2007
Zhou, L., Zhang, J., Jiang, X. M., Xie, D. J., Wang, J. S., Li, L., et al. (2015). Pulmonary artery denervation attenuates pulmonary arterial remodeling in dogs with pulmonary arterial hypertension induced by dehydrogenized monocrotaline. JACC Cardiovasc. Interv. 8, 2013–2023. doi: 10.1016/j.jcin.2015.09.015
Zoccal, D. B., Simms, A. E., Bonagamba, L. G., Braga, V. A., Pickering, A. E., Paton, J. F., et al. (2008). Increased sympathetic outflow in juvenile rats submitted to chronic intermittent hypoxia correlates with enhanced expiratory activity. J. Physiol. 586, 3253–3265. doi: 10.1113/jphysiol.2008.154187
Keywords: pulmonary artery, hypoxia, calcium, contraction, adrenergic receptor, fetus, sheep
Citation: Moretta D, Papamatheakis DG, Morris DP, Giri PC, Blood Q, Murray S, Ramzy M, Romero M, Vemulakonda S, Lauw S, Longo LD, Zhang L and Wilson SM (2019) Long-Term High-Altitude Hypoxia and Alpha Adrenoceptor-Dependent Pulmonary Arterial Contractions in Fetal and Adult Sheep. Front. Physiol. 10:1032. doi: 10.3389/fphys.2019.01032
Received: 01 May 2019; Accepted: 26 July 2019;
Published: 28 August 2019.
Edited by:
Francisco C. Villafuerte, Universidad Peruana Cayetano Heredia, PeruReviewed by:
Patricia Siques, Arturo Prat University, ChileCopyright © 2019 Moretta, Papamatheakis, Morris, Giri, Blood, Murray, Ramzy, Romero, Vemulakonda, Lauw, Longo, Zhang and Wilson. This is an open-access article distributed under the terms of the Creative Commons Attribution License (CC BY). The use, distribution or reproduction in other forums is permitted, provided the original author(s) and the copyright owner(s) are credited and that the original publication in this journal is cited, in accordance with accepted academic practice. No use, distribution or reproduction is permitted which does not comply with these terms.
*Correspondence: Sean M. Wilson, c2VhbndpbHNvbkBsbHUuZWR1
†Deceased
Disclaimer: All claims expressed in this article are solely those of the authors and do not necessarily represent those of their affiliated organizations, or those of the publisher, the editors and the reviewers. Any product that may be evaluated in this article or claim that may be made by its manufacturer is not guaranteed or endorsed by the publisher.
Research integrity at Frontiers
Learn more about the work of our research integrity team to safeguard the quality of each article we publish.