- 1Orthopaedic Pathophysiology and Regenerative Medicine Unit, IRCCS Istituto Ortopedico Rizzoli, Bologna, Italy
- 2Department of Biomedical and Neuromotor Sciences, University of Bologna, Bologna, Italy
Bone primary tumors, such as osteosarcoma, are highly aggressive pediatric tumors that in 30% of the cases develop lung metastasis and are characterized by poor prognosis. Bone is also the third most common metastatic site in patients with advanced cancer and once tumor cells become homed to the skeleton, the disease is usually considered incurable, and treatment is only palliative. Bone sarcoma and bone metastasis share the same tissue microenvironment and niches. 3D cultures represent a new promising approach for the study of interactions between tumor cells and other cellular or acellular components of the tumor microenvironment (i.e., fibroblasts, mesenchymal stem cells, bone ECM). Indeed, 3D models can mimic physiological interactions that are crucial to modulate response to soluble paracrine factors, tumor drug resistance and aggressiveness and, in all, these innovative models might be able of bypassing the use of animal-based preclinical cancer models. To date, both static and dynamic 3D cell culture models have been shown to be particularly suited for screening of anticancer agents and might provide accurate information, translating in vitro cell cultures into precision medicine. In this mini-review, we will summarize the current state-of-the-art in the field of bone tumors, both primary and metastatic, illustrating the different methods and techniques employed to realize 3D cell culture systems and new results achieved in a field that paves the way toward personalized medicine.
Introduction
Cancer is a complex disease that thrives in a heterogeneous and adaptive tumor microenvironment (Park et al., 2014). Bone sarcomas and bone metastasis (BM) share the same environment and the niche, where tumor cells can seed and proliferate. Osteosarcoma (OS), chondrosarcoma (CS), and ewing sarcoma (ES) are the most common malignant primary bone tumors, accounting for 70% of all such malignancies. Despite the advent of chemotherapy has widely improved patient survival, sarcomas are still considered deadly and, in a high percentage of cases, incurable diseases (Lewis, 2009). Similarly, BM form when carcinoma cells have homed to the skeleton and, at this stage, the disease is usually considered incurable, treatment with current modalities is only palliative and often associated to uncomfortable side effects (Fornetti et al., 2018).
Bone sarcomas are a disease of mesenchymal origin; they originate in the bone, where the mesenchymal stem cells (MSC) are both ontogenic progenitor tumor cells (Mohseny et al., 2009; Lye et al., 2016) and stromal cells that participates to tumor development (Xiao et al., 2013; Cortini et al., 2017). In the bone, the tumor-supporting stroma is formed by osteoblasts (the bone forming cells deriving from MSC), osteoclasts (the bone resorbing cells), endothelial and immune cells, and MSC. Osteoclasts adhere to bone surface and the spectrum of factors involved in their activation may depend on tumor type. As an example, osteoclasts can be metabolically fueled directly by tumor cells (Lemma et al., 2016, 2017) or also stimulated by tumor-induced osteoblasts (Sousa and Clezardin, 2018). In OS, the presence of osteoclasts in the tumor microenvironment may foster the osteoblastic behavior of tumor cells and increase their aggressiveness (Costa-Rodrigues et al., 2011) and is considered a bad prognostic factor (Salerno et al., 2008). Similarly, in BM, the pathogenic process forms when the delicate balance between bone deposition and resorption is disrupted (Alfranca et al., 2015).
Given the complexity and heterogeneity of bone tumors, the therapeutic strategies aimed at their eradication has exhibited a consistent slow-down to respect to many other carcinomas. Clearly, a better understanding of bone cancer oncogenesis is warranted to overcome drug resistance and improve low survival rate. A number of obstacles impede the study of bone cancers with the current means. These include the physical difficulty of manipulating bone as a tissue, the rarity of the tumors for sarcoma, the difficulties of obtaining tumor tissue fragments from human patients for BM, and the limited number of models that effectively mimic human disease. For all these reasons, the need for new cell models for bone cancers is becoming crucial.
In this review, we focused on the cellular models that are currently available for the study of BM or sarcomas. Such models have long been restricted to the two dimensions (2D) of dishes – an obvious obstacle to investigating structure and organization in cultured cells. However, a variety of 3D cell culture methods have recently emerged and are changing the way that multicellular systems are modeled.
Advances in 2D Systems
For decades, monolayer cultures have been the leading light in wet biology; Harrison (1907) developed the first cell culture from a nerve fiber in 1907 and demonstrated that tissue specimens could live out of the body for as long as a 4 weeks time. Since then, 2D monolayers have been worldwide extended and the culture technique substantially improved. Nowadays, monolayer cultures have been upgraded for the study of single or multiple populations. Co-seeding, transwell membranes and conditioned culture medium are examples of how cells can be easily handled. Cancer cells can be treated with conditioned medium of other cells that play a consistent role in tumor growth (i.e., fibroblasts or MSC) (Sasser et al., 2007; Iser et al., 2016). Transwell allows the culturing of two cell types seeded in separate compartments (Figure 1; Chiovaro et al., 2015; Avnet et al., 2017). Lastly, co-seeding two different cell population in the same compartment is also possible, but discrimination of the studied effect on one of the two populations requires the physical separation of the cells that is expensive and not always possible (Molloy et al., 2009).
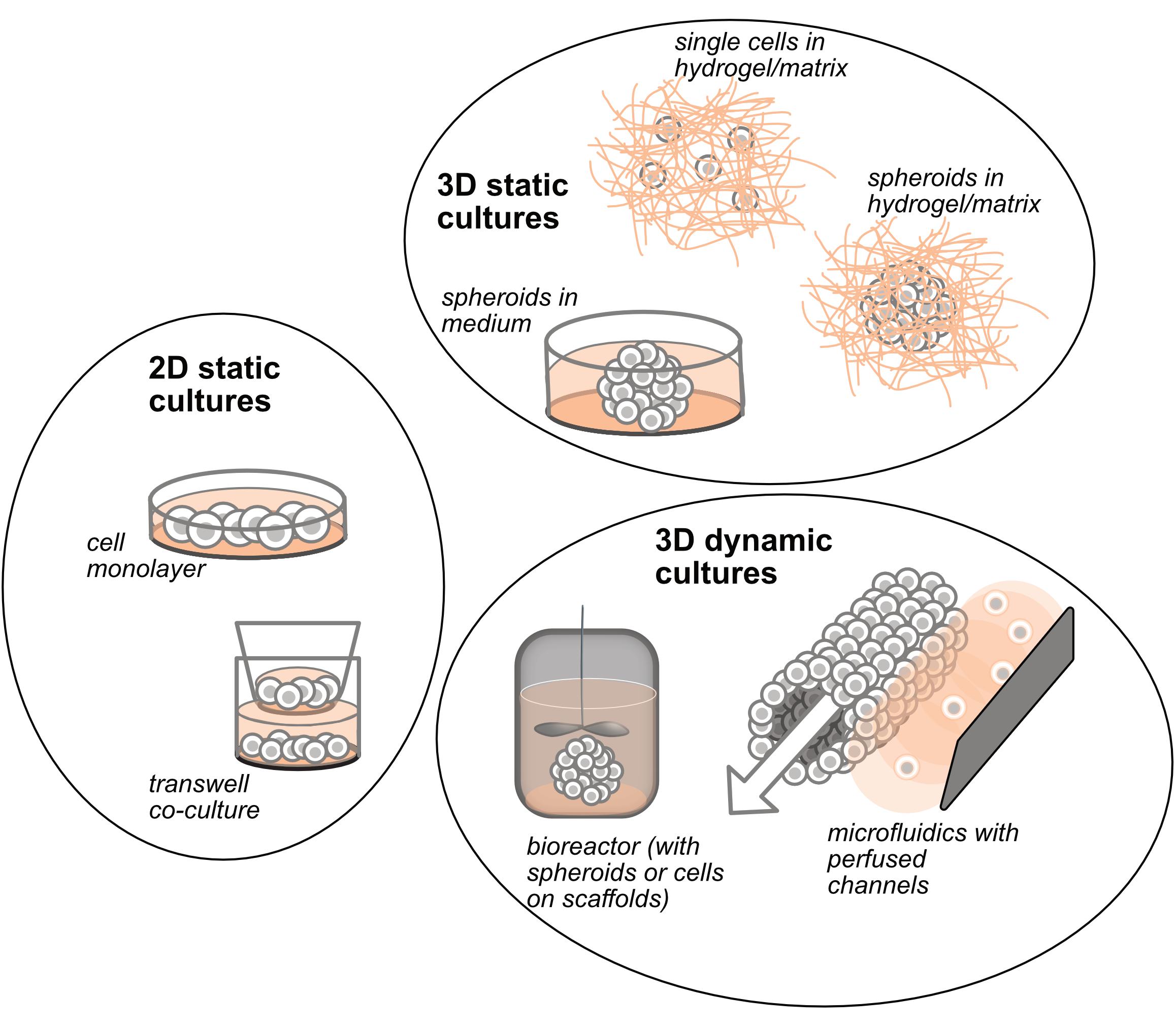
Figure 1. Schematic representation of the different and most used 2D and 3D in vitro culture systems.
Despite this, differences in cell morphology, migration, polarization, interaction with the ECM but also cell metabolism and regional genotypic and phenotypic changes (Dhiman et al., 2005; Chitcholtan et al., 2013; Russell et al., 2017), or chemoresistance (Colella et al., 2018) are often far from being confirmed in 3D models. Altogether, these difference are surely due to the lack of spatial relationships and adequate culture conditions. Among the others, one example is that cells respond differently to hard substrates like lab plastic than they do on softer ones that resemble the ECM (Engler et al., 2006).
The Upgrade to the In Vitro Third Dimension
Three dimensional architecture is one of the main issues at the basis of tissue and organ formation; this level of complexity starts during embryonic development and further enhances with cell-to-cell contacts that pose the basis to intracellular functions (Fitzgerald et al., 2015). Furthermore, cells are surrounded by an ECM that crucially determines cell differentiation, proliferation, and homeostasis (Kinney et al., 2014). An ideal 3D culture model should thus properly mimic not only oncogenesis, and maintenance of tumor cell growth, but also imitate the interactions between cells intermingled within the ECM. To date, with this aim, several technologies have been developed and explored: static 3D cultures include the seeding of cells in spheroid-like structure without the extracellular matrix, and the seeding of cells in matrices or scaffolds, made of natural or synthetic biomaterials (Figure 1); dynamic 3D cultures include either spheroids or scaffolds cultured in bioreactors, and the seeding of cells in microfluidic perfused devices (Figure 1).
Spheroids
One of the pioneer studies that has opened the field to 3D cultures is the work by Sutherland et al. (1971); they were among the first to observe that lung cells grown in suspension would form spheroids that develop with an outer zone containing proliferating cells, a poorly nourished and oxygenated intermediate zone containing few cells in mitosis, and a central zone of necrosis, a typical feature of physiological tumor masses. Forced-floating spheroids are the simplest method to generate spheroids: cells are prevented to attach to the well bottom, resulting in floating aggregates and cell-cell contacts. The hanging-drop method is the most widely used and is a static technology (Kelm et al., 2003). Adversely, rotating cell culture bioreactor, spinner flasks or stirred-tank cultures (Santo et al., 2016) force homogenous spheroid formation by continuous agitation (Breslin and O’Driscoll, 2013). Also in this case, spheroids can be formed by a single cell type or can mimic interactions between multiple cells, such as tumor and stromal cells (Ishiguro et al., 2017). These culture systems are highly reproducible and have low production costs; already in 1971 it was clear that spheroids had the potential to be used for drug screening or to test radiation therapies (Sutherland et al., 1971). Despite the advantages, not all cell lines form spheroids and some form only unpredictable cell aggregates.
Matrices and Scaffolds
As for spheroids, cells seeded into matrices or on scaffolds can be cultured either in static or dynamic cultures using rotating cell culture bioreactors.
A hydrogel-based matrix is a network of physically or chemically cross-linked polymer molecules of hydrophilic nature that allows to retain large amounts of water (Ahmed, 2015) and provide a 3D biomimetic environment supporting cell proliferation and differentiation (Peck and Wang, 2013). The major advantage of hydrogels is their customization according to the specific features of the ECM. As an example, hydrogels can be designed to shrink or swell based on the environmental stimuli that they receive (Ahmed, 2015), and can be easily enriched with specific cell adhesion ligands to mimic soft tissues. Hydrogels are of synthetic or natural origin (Li and Kumacheva, 2018), and are mainly based on matrigel, collagen or fibrin. Matrigel derives from a mouse sarcoma and has the most heterogeneous composition. The chief components are structural proteins such as laminin, nidogen, collagen, and heparan sulfate proteoglycans. Matrigel polymerization depends on temperature. Collagen-based hydrogels rely also on pH and plays a crucial role in cancer progression and is the most common protein of mammalian ECM. However, the pH-dependency makes collagen-based hydrogels unsuitable for the study of the effects of tumor acidosis, a feature that is crucial for the development of bone cancer (Cortini et al., 2017; Avnet et al., 2019), or of cancer-induced bone pain (Yoneda et al., 2015; Di Pompo et al., 2017).
Traditionally described as tools made of polymeric biomaterials, 3D scaffolds have the advantage to provide recapitulation of the ECM by providing, like hydrogels, attachment sites and interstitial space for the cells that can grow and proliferate, forming 3D structures (Breslin and O’Driscoll, 2013). Scaffold stiffness can be tuned to influence cell adhesion, proliferation and activation (Keogh et al., 2010). Materials used for scaffold fabrication must be biocompatible and must induce molecular biorecognition from cells (Carletti et al., 2011). ECM-mimicking biomaterials are made of collagen, hyaluronan, matrigel, elastin, laminin-rich-extracellular matrix, and also alginate, chitosan, silk and are considered as the most biocompatible. Synthetic biomaterials include polyethylen glycol, hyaluronic acid-PEG, polyvinyl alcohol, polycaprolactone, or two-phase systems such as polyethylene glycol-dextran. A number of biomaterials, such as ceramics, can fall in the natural or synthetic category (Thakuri et al., 2018).
Microfluidic Devices
Recent advancements in tissue engineering have led to the development of living multicellular microculture systems, which are maintained in controllable microenvironments and function with organ level complexity [for an extensive review see Huh et al. (2011)]. The applications of these “on-chip” technologies are becoming increasingly popular for cancer studies (Sontheimer-Phelps et al., 2019). Continuous perfusion of media through the microfluidic network is the major innovation in these systems (Chung et al., 2017) since it mimics blood flow and enables exchange of nutrients, oxygen and metabolites with the blood tissue that are crucial for modeling living cancer tissues. Invading cells detaching from a solid tumor are exposed to the novel microenvironment of the circulatory system. Depending on the size of the vessel, the blood flow velocity can reach 0.03–40 cm/s, with arterial hemodynamic shear-force of 4.0–30.0 dyn/cm2 and venous shear-force of 0.5–4.0 dyn/cm2 (McCarty et al., 2016). Therefore, tumor cells must promptly adapt from static growth to fluid shear stress (Mitchell and King, 2013; Phillips et al., 2014), a condition that is far from being taken into account on static cultures. Until few years ago, microplates supported only 2D environments (Wu et al., 2010). More recently, the third dimension has been introduced to support 3D aggregates (Toh et al., 2007; van Duinen et al., 2015; Lanz et al., 2017). Finally, microfluidics has allowed the design and the development of self-organized organ-like cell aggregates that originate from multipotent stem cells, the organoids, and has opened a whole new level of biomimicry to be achieved (Yu et al., 2019). Representative examples are the blood brain barriers, the 3D neuronal networks, the kidney, liver or the intact gut epithelium or, when mentioning cancer tissues, glioma, breast cancer or sarcoma models (Sontheimer-Phelps et al., 2019).
This technology has the power to add multiple cell lines in the same chip. As an example, it is possible to mimic the tumor-endothelial cells interaction that is fundamental for the metastasization process, including angiogenesis, intravasation and cancer cell colonization (Zervantonakis et al., 2012). Likewise, microfluidics have been thoroughly studied to better recapitulate the cancer cell-immune cell interactions, with the ultimate aim of increasing knowledge on cancer immunotherapies (Boussommier-Calleja et al., 2016).
Finally, formation of 3D spheroids by using hanging drops have been combined to microfluidic platforms for drug testing or chemoresponses assays (Marimuthu et al., 2018). The next big challenge is the full validation of these models and subsequently the implementation in drug development pipelines of the pharmaceutical industry and ultimately in personalized medicine applications.
Studying the Pathogenesis of Tumour Niche in 3D In Vitro Systems of Bone Cancers
Many papers have discussed the importance of switching from 2D to 3D cultures in a number of tumor cell lines (Praharaj et al., 2018), including bone sarcoma (De Luca et al., 2018). Novel models have now been acquainted also for bone cancers, and for tumor-related bone microenvironment (Table 1), and that include 3D tumor-resembling structure endothelial or fibroblastic cells in order to develop antiangiogenic therapies and to better understand vasculature expansion (Lee et al., 2006; Reddy et al., 2008; de Nigris et al., 2013). 3D OS cells have been combined with 2D endothelial HUVEC cells to form a well-organized network, including tubule-like structures that infiltrated the tumor spheroids, like new vessels in vivo. In this model, HUVEC proliferation and expression of angiogenesis-associated genes was possible induced by VEGF secretion from quiescent OS cells, embedded in matrix at the center of the spheroid, and stressed by the hypoxic core (Chaddad et al., 2017). The vasculature also seemed to direct the reactivation of dormant disseminated tumor cells. Targeting the vascular niches in such early steps of BM delays or even prevents the metastatic relapse (Kusumbe, 2016). Likewise, a functional tri-culture has been developed for studying metastatic breast cancer that has spread to the bone. This includes a stable vascular networks within a 3D native bone matrix cultured on a microfluidic chip; this niche-on-a-chip is characterized by controlled flow velocities, shear stresses, and oxygen gradients. Interestingly, MSC, which have undergone phenotypical transition toward perivascular cell lineages, support the formation of capillary-like structures lining the vascular lumen (Marturano-Kruik et al., 2018). MSC are associated with the tumor microenviroment since they are recruited by tumor cells from the bloodstream and are a considerable component of the general host response to tissue damage caused by cancer cells (Cortini et al., 2016; Avnet et al., 2017; Cortini et al., 2017). In another breast cancer metastatic model, MSC stimulate tumor extravasation and activation of the cancer cell receptor CXCR2 and the bone-secreted chemokine CXCL5 (Bersini et al., 2014). Chemokines and interleukins are also responsible for chemo-attraction of immune cells that, once recruited to the niche, become part of the tumor bulk and play a fundamental role in the tumor TME. Microfluidic platforms retain also the possibility to monitor immune cell migration and analyze their contribution to the formation of the metastatic niche through spatial compartmentalization (Gopalakrishnan et al., 2015; Boussommier-Calleja et al., 2016).
Microfluidics have been also used to successfully recreate the complex bone marrow TME (Torisawa et al., 2014). The microchip device included two different compartments: one for the specific growth of osteoblasts and one for medium change. Because osteoblastic tissues require long-term cultures, this design was proven successful as it allowed the formation of a thick mineralized osteoblastic tissue in vitro in a 1 month time period (Hao et al., 2018). The bone niche can be also recreated by using bone scaffolds (Marturano-Kruik et al., 2016). In ES, for example, cell growth rate is far slower in vivo than that observed in vitro, thereby more likely reproducing reliable growth conditions (Fong et al., 2013) and mimicking critical signaling cascades, such as the IGF-1R/PI3K7mTOR signaling pathway (Lamhamedi-Cherradi et al., 2014).
Assaying Chemoresistance in 3D In Vitro Systems of Bone Cancers
Arai et al. (2013) found that spheroid cells displayed more chemoresistance to doxorubicin corresponding to higher IC50 values than conventional monolayer cells (Baek et al., 2016a) in more than 11 OS cell lines. Similarly, Baek et al. (2016b) confirmed that OS cells were more chemoresistant in 3D compared to 2D culture. Similar results were obtained also with cisplatin. Likewise, the use of MG-63 spheroids effectively predicted the cytotoxicity of oxidovanadium(IV) in vivo models (Leon et al., 2016). 3D CS cultures are resistant to doxorubicin and mafosfamide, when compared to standard monolayer cultures (Monderer et al., 2013). However, the use of 3D spheroids allowed to reveal that treatment with the ionophore salinomycin, previously uncharacterized for its effects on CS, significantly enhanced the cytotoxic effect to doxorubicin in 3D structures (Perut et al., 2018).
Finally, drug sensitivity of tumor cells might be strongly affected by microenvironmental factors that include the presence of MSC (Avnet et al., 2017; Senthebane et al., 2017), also when co-cultured with cancer cells in 3D structures. As an example, the 3D assembly of ES cells with MSC elicits ligand-mediated activation of the insulin-like growth factor-1 receptor (IGF-1R), thereby mediating resistance to IGF-1R inhibitors (Santoro et al., 2017).
From Preclinical Models to Clinical Validation
The main aim of expanding the knowledge on culture systems is to be able to translate the molecular features of the 3D cell cultures of individual patients using them as a platform for drug screening and to identify biomarkers and new drug targets (Breslin and O’Driscoll, 2013). Findings using 3D models that more accurately reflect human sarcoma biology are likely to translate into improved clinical outcomes (Gao et al., 2017). As an example moving in this direction, Pauli and colleagues have made a great effort in describing a precision-medicine platform that integrates whole-exome sequencing with a living biobank that enables high-throughput drug screens on patient-derived tumor organoids. To date, 56 tumor-derived organoid cultures and 19 patient-derived xenograft models have been established from the 769 patients enrolled in an Institutional Review Board–approved clinical trial (Pauli et al., 2017). These types of approach have the potential not only to select the appropriate therapeutic option, but also to improve the knowledge on the molecular cues that lay at the basis of tumor development.
Conclusion
Three dimensional models offer early promise in establishing robust preclinical platforms for the identification of crucial molecular pathways and for the assessment of clinical efficacy of novel drugs to inhibit cancer development and progression. Despite the perfect model currently does not exist and 3D approaches are characterized by weaknesses, these greatly expand the spectrum of cancer subtypes that might be considered for new drug screening and for the development of personalized medicine. In the field of bone cancers, rare and deadly diseases, this is of paramount importance to improve the clinical outcomes.
Author Contributions
All authors listed have made a substantial, direct and intellectual contribution to the work, and approved it for publication.
Funding
This study was funded by the Italian Association for Cancer Research (AIRC IG# 21403 to NB) and by the financial support for Scientific Research “5xMille 2016 Ministry of Health” (to NB).
Conflict of Interest Statement
The authors declare that the research was conducted in the absence of any commercial or financial relationships that could be construed as a potential conflict of interest.
References
Ahmed, E. M. (2015). Hydrogel: preparation, characterization, and applications: a review. J. Adv. Res. 6, 105–121. doi: 10.1016/j.jare.2013.07.006
Alfranca, A., Martinez-Cruzado, L., Tornin, J., Abarrategi, A., Amaral, T., de Alava, E., et al. (2015). Bone microenvironment signals in osteosarcoma development. Cell Mol. Life Sci. 72, 3097–3113. doi: 10.1007/s00018-015-1918-y
Arai, K., Sakamoto, R., Kubota, D., and Kondo, T. (2013). Proteomic approach toward molecular backgrounds of drug resistance of osteosarcoma cells in spheroid culture system. Proteomics 13, 2351–2360. doi: 10.1002/pmic.201300053
Avnet, S., Di Pompo, G., Chano, T., Errani, C., Ibrahim-Hashim, A., Gillies, R. J., et al. (2017). Cancer-associated mesenchymal stroma fosters the stemness of osteosarcoma cells in response to intratumoral acidosis via NF-kappaB activation. Int. J. Cancer 140, 1331–1345. doi: 10.1002/ijc.30540
Avnet, S., Di Pompo, G., Lemma, S., and Baldini, N. (2019). Cause and effect of microenvironmental acidosis on bone metastases. Cancer Metastasis Rev. doi: 10.1007/s10555-019-09790-9. [Epub ahead of print].
Baek, N., Seo, O. W., Kim, M., Hulme, J., and An, S. S. (2016a). Monitoring the effects of doxorubicin on 3D-spheroid tumor cells in real-time. Onco. Targets Ther. 9, 7207–7218. doi: 10.2147/OTT.S112566
Baek, N., Seo, O. W., Lee, J., Hulme, J., and An, S. S. (2016b). Real-time monitoring of cisplatin cytotoxicity on three-dimensional spheroid tumor cells. Drug Des. Devel. Ther. 10, 2155–2165. doi: 10.2147/DDDT.S108004
Bersini, S., Jeon, J. S., Dubini, G., Arrigoni, C., Chung, S., Charest, J. L., et al. (2014). A microfluidic 3D in vitro model for specificity of breast cancer metastasis to bone. Biomaterials 35, 2454–2461. doi: 10.1016/j.biomaterials.2013.11.050
Boussommier-Calleja, A., Li, R., Chen, M. B., Wong, S. C., and Kamm, R. D. (2016). Microfluidics: a new tool for modeling cancer-immune interactions. Trends Cancer 2, 6–19. doi: 10.1016/j.trecan.2015.12.003
Breslin, S., and O’Driscoll, L. (2013). Three-dimensional cell culture: the missing link in drug discovery. Drug Discov. Today 18, 240–249. doi: 10.1016/j.drudis.2012.10.003
Carletti, E., Motta, A., and Migliaresi, C. (2011). Scaffolds for tissue engineering and 3D cell culture. Methods Mol. Biol. 695, 17–39. doi: 10.1007/978-1-60761-984-0_2
Chaddad, H., Kuchler-Bopp, S., Fuhrmann, G., Gegout, H., Ubeaud-Sequier, G., Schwinte, P., et al. (2017). Combining 2D angiogenesis and 3D osteosarcoma microtissues to improve vascularization. Exp. Cell Res. 360, 138–145. doi: 10.1016/j.yexcr.2017.08.035
Chiovaro, F., Martina, E., Bottos, A., Scherberich, A., Hynes, N. E., and Chiquet-Ehrismann, R. (2015). Transcriptional regulation of tenascin-W by TGF-beta signaling in the bone metastatic niche of breast cancer cells. Int. J. Cancer 137, 1842–1854. doi: 10.1002/ijc.29565
Chitcholtan, K., Asselin, E., Parent, S., Sykes, P. H., and Evans, J. J. (2013). Differences in growth properties of endometrial cancer in three dimensional (3D) culture and 2D cell monolayer. Exp. Cell Res. 319, 75–87. doi: 10.1016/j.yexcr.2012.09.012
Chung, M., Ahn, J., Son, K., Kim, S., and Jeon, N. L. (2017). . Biomimetic Model of Tumor Microenvironment on Microfluidic Platform. Adv. Healthc. Mater. 6:1700196. doi: 10.1002/adhm.201700196
Colella, G., Fazioli, F., Gallo, M., De Chiara, A., Apice, G., Ruosi, C., et al. (2018). Sarcoma spheroids and organoids-promising tools in the era of personalized medicine. Int. J. Mol. Sci. 19:615. doi: 10.3390/ijms19020615
Cortini, M., Avnet, S., and Baldini, N. (2017). Mesenchymal stroma: role in osteosarcoma progression. Cancer Lett. 405, 90–99. doi: 10.1016/j.canlet.2017.07.024
Cortini, M., Massa, A., Avnet, S., Bonuccelli, G., and Baldini, N. (2016). Tumor-activated mesenchymal stromal cells promote osteosarcoma stemness and migratory potential via IL-6 secretion. PLoS One 11:e0166500. doi: 10.1371/journal.pone.0166500
Costa-Rodrigues, J., Fernandes, A., and Fernandes, M. H. (2011). Reciprocal osteoblastic and osteoclastic modulation in co-cultured MG63 osteosarcoma cells and human osteoclast precursors. J. Cell Biochem. 112, 3704–3713. doi: 10.1002/jcb.23295
De Luca, A., Raimondi, L., Salamanna, F., Carina, V., Costa, V., Bellavia, D., et al. (2018). Relevance of 3d culture systems to study osteosarcoma environment. J. Exp. Clin. Cancer Res. 37:2. doi: 10.1186/s13046-017-0663-5
de Nigris, F., Mancini, F. P., Schiano, C., Infante, T., Zullo, A., Minucci, P. B., et al. (2013). Osteosarcoma cells induce endothelial cell proliferation during neo-angiogenesis. J. Cell Physiol. 228, 846–852. doi: 10.1002/jcp.24234
Dhiman, H. K., Ray, A. R., and Panda, A. K. (2005). Three-dimensional chitosan scaffold-based MCF-7 cell culture for the determination of the cytotoxicity of tamoxifen. Biomaterials 26, 979–986. doi: 10.1016/j.biomaterials.2004.04.012
Di Pompo, G., Lemma, S., Canti, L., Rucci, N., Ponzetti, M., Errani, C., et al. (2017). Intratumoral acidosis fosters cancer-induced bone pain through the activation of the mesenchymal tumor-associated stroma in bone metastasis from breast carcinoma. Oncotarget 8, 54478–54496. doi: 10.18632/oncotarget.17091
Engler, A. J., Sen, S., Sweeney, H. L., and Discher, D. E. (2006). Matrix elasticity directs stem cell lineage specification. Cell 126, 677–689. doi: 10.1016/j.cell.2006.06.044
Fitzgerald, K. A., Malhotra, M., Curtin, C. M., O’Brien, F. J., and O’Driscoll, C. M. (2015). Life in 3D is never flat: 3D models to optimise drug delivery. J. Control Release 215, 39–54. doi: 10.1016/j.jconrel.2015.07.020
Fong, E. L., Lamhamedi-Cherradi, S. E., Burdett, E., Ramamoorthy, V., Lazar, A. J., Kasper, F. K., et al. (2013). Modeling ewing sarcoma tumors in vitro with 3D scaffolds. Proc. Natl. Acad Sci. U.S.A. 110, 6500–6505. doi: 10.1073/pnas.1221403110
Fornetti, J., Welm, A. L., and Stewart, S. A. (2018). Understanding the bone in cancer metastasis. J. Bone Miner. Res. 33, 2099–2113. doi: 10.1002/jbmr.3618
Gao, S., Shen, J., Hornicek, F., and Duan, Z. (2017). Three-dimensional (3D) culture in sarcoma research and the clinical significance. Biofabrication 9:032003. doi: 10.1088/1758-5090/aa7fdb
Gopalakrishnan, N., Hannam, R., Casoni, G. P., Barriet, D., Ribe, J. M., Haug, M., et al. (2015). Infection and immunity on a chip: a compartmentalised microfluidic platform to monitor immune cell behaviour in real time. Lab Chip 15, 1481–1487. doi: 10.1039/c4lc01438c
Hao, S., Ha, L., Cheng, G., Wan, Y., Xia, Y., Sosnoski, D. M., et al. (2018). A spontaneous 3D bone-on-a-chip for bone metastasis study of breast cancer cells. Small 14:e1702787. doi: 10.1002/smll.201702787
Huh, D., Hamilton, G. A., and Ingber, D. E. (2011). From 3D cell culture to organs-on-chips. Trends Cell Biol. 21, 745–754. doi: 10.1016/j.tcb.2011.09.005
Iser, I. C., Ceschini, S. M., Onzi, G. R., Bertoni, A. P., Lenz, G., and Wink, M. R. (2016). Conditioned medium from adipose-derived stem cells (ADSCs) promotes epithelial-to-mesenchymal-like transition (EMT-Like) in glioma cells In vitro. Mol. Neurobiol. 53, 7184–7199. doi: 10.1007/s12035-015-9585-4
Ishiguro, T., Ohata, H., Sato, A., Yamawaki, K., Enomoto, T., and Okamoto, K. (2017). Tumor-derived spheroids: relevance to cancer stem cells and clinical applications. Cancer Sci. 108, 283–289. doi: 10.1111/cas.13155
Kelm, J. M., Timmins, N. E., Brown, C. J., Fussenegger, M., and Nielsen, L. K. (2003). Method for generation of homogeneous multicellular tumor spheroids applicable to a wide variety of cell types. Biotechnol. Bioeng. 83, 173–180. doi: 10.1002/bit.10655
Keogh, M. B., O’Brien, F. J., and Daly, J. S. (2010). Substrate stiffness and contractile behaviour modulate the functional maturation of osteoblasts on a collagen-GAG scaffold. Acta Biomater. 6, 4305–4313. doi: 10.1016/j.actbio.2010.06.001
Kinney, M. A., Hookway, T. A., Wang, Y., and McDevitt, T. C. (2014). Engineering three-dimensional stem cell morphogenesis for the development of tissue models and scalable regenerative therapeutics. Ann. Biomed. Eng. 42, 352–367. doi: 10.1007/s10439-013-0953-9
Kusumbe, A. P. (2016). Vascular niches for disseminated tumour cells in bone. J. Bone Oncol. 5, 112–116. doi: 10.1016/j.jbo.2016.04.003
Lamhamedi-Cherradi, S. E., Santoro, M., Ramammoorthy, V., Menegaz, B. A., Bartholomeusz, G., Iles, L. R., et al. (2014). 3D tissue-engineered model of Ewing’s sarcoma. Adv. Drug Deliv. Rev. 7, 155–171. doi: 10.1016/j.addr.2014.07.012
Lanz, H. L., Saleh, A., Kramer, B., Cairns, J., Ng, C. P., Yu, J., et al. (2017). Therapy response testing of breast cancer in a 3D high-throughput perfused microfluidic platform. BMC Cancer 17:709. doi: 10.1186/s12885-017-3709-3
Lee, T. H., Bolontrade, M. F., Worth, L. L., Guan, H., Ellis, L. M., and Kleinerman, E. S. (2006). Production of VEGF165 by Ewing’s sarcoma cells induces vasculogenesis and the incorporation of CD34+ stem cells into the expanding tumor vasculature. Int. J. Cancer 119, 839–846. doi: 10.1002/ijc.21916
Lemma, S., Di Pompo, G., Porporato, P. E., Sboarina, M., Russell, S., Gillies, R. J., et al. (2017). MDA-MB-231 breast cancer cells fuel osteoclast metabolism and activity: a new rationale for the pathogenesis of osteolytic bone metastases. Biochim. Biophys. Acta Mol. Basis Dis. 1863, 3254–3264. doi: 10.1016/j.bbadis.2017.08.030
Lemma, S., Sboarina, M., Porporato, P. E., Zini, N., Sonveaux, P., Di Pompo, G., et al. (2016). Energy metabolism in osteoclast formation and activity. Int. J. Biochem. Cell Biol. 79, 168–180. doi: 10.1016/j.biocel.2016.08.034
Leon, I. E., Cadavid-Vargas, J. F., Resasco, A., Maschi, F., Ayala, M. A., Carbone, C., et al. (2016). In vitro and in vivo antitumor effects of the VO-chrysin complex on a new three-dimensional osteosarcoma spheroids model and a xenograft tumor in mice. J. Biol. Inorg. Chem. 21, 1009–1020. doi: 10.1007/s00775-016-1397-0
Lewis, V. O. (2009). What’s new in musculoskeletal oncology. J. Bone Joint Surg. Am. 91, 1546–1556. doi: 10.2106/JBJS.I.00375
Li, Y., and Kumacheva, E. (2018). Hydrogel microenvironments for cancer spheroid growth and drug screening. Sci. Adv. 4:eaas8998. doi: 10.1126/sciadv.aas8998
Lye, K. L., Nordin, N., Vidyadaran, S., and Thilakavathy, K. (2016). Mesenchymal stem cells: from stem cells to sarcomas. Cell Biol. Int. 40, 610–618. doi: 10.1002/cbin.10603
Marimuthu, M., Rousset, N., St-Georges-Robillard, A., Lateef, M. A., Ferland, M., Mes-Masson, A. M., et al. (2018). Multi-size spheroid formation using microfluidic funnels. Lab Chip 18, 304–314. doi: 10.1039/c7lc00970d
Marturano-Kruik, A., Nava, M. M., Yeager, K., Chramiec, A., Hao, L., Robinson, S., et al. (2018). Human bone perivascular niche-on-a-chip for studying metastatic colonization. Proc. Natl. Acad Sci. U.S.A. 115, 1256–1261. doi: 10.1073/pnas.1714282115
Marturano-Kruik, A., Villasante, A., and Vunjak-Novakovic, G. (2016). Bioengineered models of solid human tumors for cancer research. Methods Mol. Biol. 1502, 203–211. doi: 10.1007/7651_2016_353
McCarty, O. J., Ku, D., Sugimoto, M., King, M. R., Cosemans, J. M., Neeves, K. B., et al. (2016). Dimensional analysis and scaling relevant to flow models of thrombus formation: communication from the SSC of the ISTH. J. Thromb. Haemost. 14, 619–622. doi: 10.1111/jth.13241
Mitchell, M. J., and King, M. R. (2013). Computational and experimental models of cancer cell response to fluid shear stress. Front. Oncol. 3:44. doi: 10.3389/fonc.2013.00044
Mohseny, A. B., Szuhai, K., Romeo, S., Buddingh, E. P., Briaire-de Bruijn, I., de Jong, D., et al. (2009). Osteosarcoma originates from mesenchymal stem cells in consequence of aneuploidization and genomic loss of Cdkn2. J. Pathol. 219, 294–305. doi: 10.1002/path.2603
Molloy, A. P., Martin, F. T., Dwyer, R. M., Griffin, T. P., Murphy, M., Barry, F. P., et al. (2009). Mesenchymal stem cell secretion of chemokines during differentiation into osteoblasts, and their potential role in mediating interactions with breast cancer cells. Int. J. Cancer 124, 326–332. doi: 10.1002/ijc.23939
Monderer, D., Luseau, A., Bellec, A., David, E., Ponsolle, S., Saiagh, S., et al. (2013). New chondrosarcoma cell lines and mouse models to study the link between chondrogenesis and chemoresistance. Lab Invest. 93, 1100–1114. doi: 10.1038/labinvest.2013.101
Park, T. S., Donnenberg, V. S., Donnenberg, A. D., Zambidis, E. T., and Zimmerlin, L. (2014). Dynamic interactions between cancer stem cells and their stromal partners. Curr. Pathobiol. Rep. 2, 41–52. doi: 10.1007/s40139-013-0036-5
Pauli, C., Hopkins, B. D., Prandi, D., Shaw, R., Fedrizzi, T., Sboner, A., et al. (2017). Personalized in vitro and in vivo cancer models to guide precision medicine. Cancer Discov. 7, 462–477. doi: 10.1158/2159-8290.CD-16-1154
Peck, Y., and Wang, D. A. (2013). Three-dimensionally engineered biomimetic tissue models for in vitro drug evaluation: delivery, efficacy and toxicity. Expert Opin. Drug Deliv. 10, 369–383. doi: 10.1517/17425247.2013.751096
Perut, F., Sbrana, F. V., Avnet, S., De Milito, A., and Baldini, N. (2018). Spheroid-based 3D cell cultures identify salinomycin as a promising drug for the treatment of chondrosarcoma. J. Orthop. Res. 36, 2305–2312. doi: 10.1002/jor.23880
Phillips, K. G., Kuhn, P., and McCarty, O. J. (2014). Physical biology in cancer. 2. The physical biology of circulating tumor cells. Am. J. Physiol. Cell. Physiol. 306, C80–C88. doi: 10.1152/ajpcell.00294.2013
Praharaj, P. P., Bhutia, S. K., Nagrath, S., Bitting, R. L., and Deep, G. (2018). Circulating tumor cell-derived organoids: current challenges and promises in medical research and precision medicine. Biochim. Biophys. Acta Rev. Cancer 1869, 117–127. doi: 10.1016/j.bbcan.2017.12.005
Reddy, K., Zhou, Z., Jia, S. F., Lee, T. H., Morales-Arias, J., Cao, Y., et al. (2008). Stromal cell-derived factor-1 stimulates vasculogenesis and enhances Ewing’s sarcoma tumor growth in the absence of vascular endothelial growth factor. Int. J. Cancer 123, 831–837. doi: 10.1002/ijc.23582
Russell, S., Wojtkowiak, J., Neilson, A., and Gillies, R. J. (2017). Metabolic profiling of healthy and cancerous tissues in 2D and 3D. Sci. Rep. 7:15285. doi: 10.1038/s41598-017-15325-5
Salerno, M., Avnet, S., Alberghini, M., Giunti, A., and Baldini, N. (2008). Histogenetic characterization of giant cell tumor of bone. Clin. Orthop. Relat. Res. 466, 2081–2091. doi: 10.1007/s11999-008-0327-z
Santo, V. E., Estrada, M. F., Rebelo, S. P., Abreu, S., Silva, I., Pinto, C., et al. (2016). Adaptable stirred-tank culture strategies for large scale production of multicellular spheroid-based tumor cell models. J. Biotechnol. 221, 118–129. doi: 10.1016/j.jbiotec.2016.01.031
Santoro, M., Menegaz, B. A., Lamhamedi-Cherradi, S. E., Molina, E. R., Wu, D., Priebe, W., et al. (2017). Modeling Stroma-Induced Drug Resistance in a Tissue-Engineered Tumor Model of Ewing Sarcoma. Tissue Eng. Part A 23, 80–89. doi: 10.1089/ten.TEA.2016.0369
Sasser, A. K., Sullivan, N. J., Studebaker, A. W., Hendey, L. F., Axel, A. E., and Hall, B. M. (2007). Interleukin-6 is a potent growth factor for ER-alpha-positive human breast cancer. FASEB J. 21, 3763–3770. doi: 10.1096/fj.07-8832com
Senthebane, D. A., Rowe, A., Thomford, N. E., Shipanga, H., Munro, D., Mazeedi, M., et al. (2017). The role of tumor microenvironment in chemoresistance: to survive, keep your enemies closer. Int. J. Mol. Sci. 18:1580. doi: 10.3390/ijms18071586
Sontheimer-Phelps, A., Hassell, B. A., and Ingber, D. E. (2019). Modelling cancer in microfluidic human organs-on-chips. Nat. Rev. Cancer 19, 65–81. doi: 10.1038/s41568-018-0104-6
Sourla, A., Doillon, C., and Koutsilieris, M. (1996). Three-dimensional type I collagen gel system containing MG-63 osteoblasts-like cells as a model for studying local bone reaction caused by metastatic cancer cells. Anticancer Res. 16, 2773–2780.
Sousa, S., and Clezardin, P. (2018). Bone-targeted therapies in cancer-induced bone disease. Calcif. Tissue Int. 102, 227–250. doi: 10.1007/s00223-017-0353-5
Sutherland, R. M., McCredie, J. A., and Inch, W. R. (1971). Growth of multicell spheroids in tissue culture as a model of nodular carcinomas. J. Natl. Cancer Inst. 46, 113–120.
Thakuri, P. S., Liu, C., Luker, G. D., and Tavana, H. (2018). Biomaterials-based approaches to tumor spheroid and organoid modeling. Adv. Healthc. Mater 7:e1700980. doi: 10.1002/adhm.201700980
Toh, Y. C., Zhang, C., Zhang, J., Khong, Y. M., Chang, S., Samper, V. D., et al. (2007). A novel 3D mammalian cell perfusion-culture system in microfluidic channels. Lab Chip 7, 302–309. doi: 10.1039/b614872g
Torisawa, Y. S., Spina, C. S., Mammoto, T., Mammoto, A., Weaver, J. C., Tat, T., et al. (2014). Bone marrow-on-a-chip replicates hematopoietic niche physiology in vitro. Nat. Methods 11, 663–669. doi: 10.1038/nmeth.2938
van Duinen, V., Trietsch, S. J., Joore, J., Vulto, P., and Hankemeier, T. (2015). Microfluidic 3D cell culture: from tools to tissue models. Curr. Opin. Biotechnol. 35, 118–126. doi: 10.1016/j.copbio.2015.05.002
Wu, M. H., Huang, S. B., and Lee, G. B. (2010). Microfluidic cell culture systems for drug research. Lab Chip 10, 939–956. doi: 10.1039/b921695b
Xiao, W., Mohseny, A. B., Hogendoorn, P. C., and Cleton-Jansen, A. M. (2013). Mesenchymal stem cell transformation and sarcoma genesis. Clin. Sarcoma. Res. 3:10. doi: 10.1186/2045-3329-3-10
Yoneda, T., Hiasa, M., Nagata, Y., Okui, T., and White, F. (2015). Contribution of acidic extracellular microenvironment of cancer-colonized bone to bone pain. Biochim. Biophys. Acta 1848(10 Pt B), 2677–2684. doi: 10.1016/j.bbamem.2015.02.004
Yu, F., Hunziker, W., and Choudhury, D. (2019). Engineering microfluidic organoid-on-a-chip platforms. Micromachines 10:165. doi: 10.3390/mi10030165
Keywords: 3D culture, sarcoma, bone metastasis, tumor niche, microenvironment
Citation: Cortini M, Baldini N and Avnet S (2019) New Advances in the Study of Bone Tumors: A Lesson From the 3D Environment. Front. Physiol. 10:814. doi: 10.3389/fphys.2019.00814
Received: 08 April 2019; Accepted: 11 June 2019;
Published: 26 June 2019.
Edited by:
Thimios Mitsiadis, University of Zurich, SwitzerlandReviewed by:
Nikolaos Dimitrakakis, Wyss Institute for Biologically Inspired Engineering and Harvard Medical School, United StatesCarole Aimé, UMR8640 Processus d’Activation Sélectif par Transfert d’Energie Uni-électronique ou Radiatif (PASTEUR), France
Eumorphia Remboutsika, National and Kapodistrian University of Athens, Greece
Copyright © 2019 Cortini, Baldini and Avnet. This is an open-access article distributed under the terms of the Creative Commons Attribution License (CC BY). The use, distribution or reproduction in other forums is permitted, provided the original author(s) and the copyright owner(s) are credited and that the original publication in this journal is cited, in accordance with accepted academic practice. No use, distribution or reproduction is permitted which does not comply with these terms.
*Correspondence: Sofia Avnet, c29maWEuYXZuZXRAaW9yLml0
†These authors have contributed equally to this work