- 1Department of Physiology, University of Alberta, Edmonton, AB, Canada
- 2Department of Obstetrics and Gynaecology, University of Alberta, Edmonton, AB, Canada
- 3Women and Children’s Health Research Institute, University of Alberta, Edmonton, AB, Canada
- 4Dementia Research Institute, Cardiff University, Cardiff, United Kingdom
- 5Musculoskeletal Research Unit, University of Bristol, Bristol, United Kingdom
Pregnancy complications associated with chronic fetal hypoxia have been linked to the development of adult cardiovascular disease in the offspring. Prenatal hypoxia has been shown to increase placental oxidative stress and impair placental function in a sex-specific manner, thereby affecting fetal development. As oxidative stress is central to placental dysfunction, we developed a placenta-targeted treatment strategy using the antioxidant MitoQ encapsulated into nanoparticles (nMitoQ) to reduce placental oxidative/nitrosative stress and improve placental function without direct drug exposure to the fetus in order to avoid off-target effects during development. We hypothesized that, in a rat model of prenatal hypoxia, nMitoQ prevents hypoxia-induced placental oxidative/nitrosative stress, promotes angiogenesis, improves placental morphology, and ultimately improves fetal oxygenation. Additionally, we assessed whether there were sex differences in the effectiveness of nMitoQ treatment. Pregnant rats were intravenously injected with saline or nMitoQ (100 μl of 125 μM) on gestational day (GD) 15 and exposed to either normoxia (21% O2) or hypoxia (11% O2) from GD15 to 21. On GD21, placentae from both sexes were collected for detection of superoxide, nitrotyrosine, nitric oxide, CD31 (endothelial cell marker), and fetal blood spaces, Vegfa and Igf2 mRNA expression in the placental labyrinth zone. Prenatal hypoxia decreased male fetal weight, which was not changed by nMitoQ treatment; however, placental efficiency (fetal/placental weight ratio) decreased by hypoxia and was increased by nMitoQ in both males and females. nMitoQ treatment reduced the prenatal hypoxia-induced increase in placental superoxide levels in both male and female placentae but improved oxygenation in only female placentae. Nitrotyrosine levels were increased in hypoxic female placentae and were reduced by nMitoQ. Prenatal hypoxia reduced placental Vegfa and Igf2 expression in both sexes, while nMitoQ increased Vegfa and Igf2 expression only in hypoxic female placentae. In summary, our study suggests that nMitoQ treatment could be pursued as a potential preventative strategy against placental oxidative stress and programming of adult cardiovascular disease in offspring exposed to hypoxia in utero. However, sex differences need to be taken into account when developing therapeutic strategies to improve fetal development in complicated pregnancies, as nMitoQ treatment was more effective in placentae from females than males.
Introduction
Chronic fetal hypoxia, a common consequence of pregnancy complications (e.g., placental insufficiency), has been linked to the development of cardiovascular and metabolic diseases in the adult offspring. The placenta serves as feto-maternal interface for the exchange of nutrients and oxygen to the fetus. Placental insufficiency is often associated with placental oxidative and nitrosative stress (i.e., formation of reactive oxygen/nitrogen species; ROS/RNS). An imbalance in ROS/RNS levels caused by excessive generation of ROS and/or a fall in endogenous antioxidants such as superoxide dismutase (SOD) can lead to impaired placental development and altered placental function (reviewed in Al-Gubory et al., 2010; Schoots et al., 2018). One impact of excessive superoxide anions is the resultant scavenging of nitric oxide (NO) to produce RNS (e.g., peroxynitrite; Myatt and Cui, 2004); therefore, increased superoxide levels could reduce NO bioavailability and impair the important contribution of NO in feto-placental angiogenesis (Webster et al., 2008).
Interestingly, the placental response to oxidative stress appears to be different in placentae from males compared with females (reviewed in Rosenfeld, 2015). Human studies have shown that the placental oxidative stress response to adverse maternal environments (such as oxidative/nitrosative stress and reduced levels of antioxidants) in general appears to be more pronounced in male versus female placentae (Stark et al., 2011; Sedlmeier et al., 2014; Muralimanoharan et al., 2015; Evans and Myatt, 2017). In rodents, similar results were found, where adverse maternal environments (such as prenatal hypoxia) altered placental morphology, placental gene expression, and enzymes for epigenetic modifications (e.g., DNA methylation) in a sexually dimorphic manner (Mao et al., 2010; Gabory et al., 2012; Kim et al., 2014; Thompson et al., 2018). Therefore, examining sex-specific differences in the placental responses to adverse maternal environments or placental phenotypes as an outcome of the adverse environments is warranted.
As the placenta lacks both autonomic and cholinergic innervation, placental morphology and function are dependent on locally derived growth factors such as vascular endothelial growth factor A (VEGFA) and insulin-like growth factor 2 (IGF2; Reynolds and Redmer, 2001; Marzioni et al., 2004). Expression of the pro-angiogenic factor VEGFA is regulated by the oxygen sensing family of transcription factors such as hypoxia-inducible factor-1 alpha (HIF-1α; Cuffe et al., 2014). Placental hypoxia has been shown to impair feto-placental vascular development via VEGFA and HIF-1α (Kulandavelu et al., 2013). Decreased IGF2 expression is also associated with intrauterine growth restriction (IUGR) and critical to placental morphology and nutrient transfer to the fetus (Coan et al., 2008). In addition, hypoxia and placental oxidative/nitrosative stress may cause mitochondrial dysfunction, DNA damage, or reduced expression of the antioxidant system, all of which can affect normal placental function and potentially reduce oxygen and nutrient delivery, thus impairing fetal development (Droge, 2002; Lappas et al., 2010; Thompson and Al-Hasan, 2012). Therefore, given that oxidative stress is a central mediator of placental dysfunction in fetal hypoxia, prenatal treatment of placental oxidative stress could potentially prevent the long-term effects of fetal hypoxia on adult offspring.
Mitochondria are a major source of cellular ROS (as reviewed in Holland et al., 2017), and human placental tissues from complicated pregnancies were shown to have increased mitochondrial content, decreased respiratory chain complex expression, and impaired mitochondrial function (as reviewed in Mando et al., 2014; Chiaratti et al., 2015; McCarthy and Kenny, 2016). Therefore, our lab and others have been studying the potential use of the mitochondrial-targeted antioxidant MitoQ to target placental oxidative stress in complicated pregnancies (Phillips et al., 2017; Aljunaidy et al., 2018; Nuzzo et al., 2018). MitoQ consists of a ubiquinone moiety linked to a positively charged lipophilic cation allowing for accumulation on the inner mitochondrial membrane, making it highly effective in preventing mitochondrial oxidative stress (Smith et al., 1999; Ross et al., 2005; Murphy and Smith, 2007). Indeed, in a rat model of prenatal hypoxia, MitoQ treatment prevented the increase in mitochondrial stress markers in the placental labyrinth zone (Nuzzo et al., 2018). Recently, we have shown that MitoQ encapsulated into polymeric nanoparticles (nMitoQ) is a delivery approach to access the placental syncytium without crossing the placental basal membrane to reach the fetus (Aljunaidy et al., 2017; Phillips et al., 2017). With this treatment strategy, we showed that maternal treatment with nMitoQ in a rat model of prenatal hypoxia prevented placental oxidative stress, increased fetal weight in female fetuses, improved neuronal development, and had sex-dependent beneficial effects on in vivo cardiovascular function in prenatally hypoxic adult offspring (Phillips et al., 2017; Aljunaidy et al., 2018). Furthermore, nMitoQ treatment of human preeclamptic placental explants improved neuronal development in vitro (Scott et al., 2018). However, the effect of nMitoQ treatment on placental morphology, the mechanisms via which reduced placental oxidative stress might improve placental function and fetal growth, and any potential sex differences are still under investigation.
In the current study, we aimed to further identify the effect of nMitoQ treatment on placental function and oxidative stress, and the sex-specific effects of the treatment, in a rat model of prenatal hypoxia. We hypothesized that antioxidant nMitoQ treatment would decrease prenatal hypoxia-induced oxidative/nitrosative stress along with increasing VEGFA expression, improving placental morphology, increasing IGF2 expression, and ultimately resulting in improved fetal growth. Moreover, since it has been shown that placentae from male and female fetuses respond to prenatal stress differently, we hypothesized that there is a sex-specific divergence in the placental response to prenatal hypoxia-induced oxidative/nitrosative stress and the effectiveness of nMitoQ treatment.
Materials and Methods
Ethics Approval
All procedures described were approved by the University of Alberta Animal Policy and Welfare Committee and were in accordance with the guidelines of the Canadian Council on Animal Care (AUP #242).
Preparation of Nanoparticle Encapsulated MitoQ (nMitoQ)
MitoQ loaded nanoparticles were synthesized as previously described (Phillips et al., 2017; Aljunaidy et al., 2018). Briefly, an amphiphilic copolymer of poly (γ-glutamic acid) and L-phenylalanine ethyl ester (γ-PGA-Phe) was synthesized as described previously (Kim et al., 2009). γ-PGA-Phe (10 mg/ml) dissolved in dimethyl sulfoxide (DMSO) was added to an equivalent volume of sodium chloride (NaCl; 0.15 M), dialyzed against distilled water using a dialysis membrane, freeze-dried, and resuspended in phosphate-buffered Saline (PBS; 10 mg/ml). Nanoparticles were then measured by dynamic light scattering (Zetasizer Nano ZS, Malvern Instruments, UK) as diameter (180 nm), zeta potential (−20 mV), and polydispersity index (0.12). γ-PGA-Phe nanoparticles (10 mg/ml) were mixed with MitoQ (2 mg/ml) at equivalent volume in NaCl (0.2 M). Nanoparticles were isolated by centrifugation, washed, and resuspended in PBS (10 mg/ml). The amount of MitoQ (278 nm), which was adsorbed to nanoparticles, was evaluated by UV absorption measurement, as previously described (Phillips et al., 2017).
Prenatal Hypoxia Rat Model
Female Sprague-Dawley rats, 3 months of age (weighing 250–275 g), were obtained from Charles River (Quebec, Canada) and housed in a temperature and light controlled room (10:14 h light/dark cycle) with ad libitum access to food and water. Females were housed with Sprague-Dawley males overnight, and pregnancy was confirmed the following morning by the presence of sperm in a vaginal smear, which was defined as gestational day (GD) 0 of pregnancy. On GD15, pregnant dams were randomly assigned into two groups that were exposed to either prenatal hypoxia (11% O2) by placing them in a Plexiglas hypoxic chamber from GD15 to 21 or were housed at atmospheric oxygen (21% O2) as controls. Pregnant dams received an intravenous injection via the tail vein on GD15 with either saline or nMitoQ (100 μl of 125 μM nMitoQ). As nMitoQ is recycled and lasts up to 1 week in vivo, the nMitoQ treatment protocol consisted only of a single injection (Phillips et al., 2017). The dose of nMitoQ was based on our previous studies (Phillips et al., 2017; Aljunaidy et al., 2018). As our study is focused on nMitoQ, as a single entity, we have a saline control rather than a nanoparticle along group as the properties may be different without the MitoQ and, ultimately, nanoparticles alone would never be used in practice. Previous studies have demonstrated that these nanoparticles are inert (Phillips et al., 2017; Scott et al., 2018). At the end of gestation, on GD21, rats were euthanized prior to parturition and fetal and placental weights were measured. Whole placentae (labyrinth and junctional zone) from male and female fetuses (two/sex/litter) were processed and embedded in either paraffin or optimal cutting temperature (OCT) compound for immunofluorescent and other staining procedures, as listed below. In other placentae (two/sex/litter), the placental labyrinth zones were isolated and snap frozen in liquid nitrogen for RNA and DNA methylation analysis.
Dihydroethidium Staining for Superoxide Production and Diaminofluorescein-FM (DAF-FM) for Nitric Oxide Levels
Placental cryostat sections (10 μm) were thawed, washed three times with Hank’s balanced salt solution (HBSS), and incubated with dihydroethidium (DHE) to measure superoxide levels (200 μM, Biotium, Burlington, Canada) or DAF-FM to measure nitric oxide levels (10 μM, Thermo Fisher Scientific, Eugene, OR, USA) in HBSS at 37°C for 30 min. Sections were washed with HBSS (3 × 2 min) and covered with a drop of 4′,6-diamidino-2-phenylindole (DAPI) (Vector Laboratories). Sections were protected from light, and pictures were immediately taken to prevent photobleaching.
Immunofluorescent Nitrotyrosine Staining for Placental Peroxynitrite Levels and CD31 Staining to Assess Placental Labyrinth Feto-Placental Vascular Capillaries
Nitrotyrosine residues are the molecular footprint of peroxynitrite generation and can be used as a marker of peroxynitrite (Webster et al., 2008). CD31 was used as an endothelial marker (Newman, 1997) to assess placental vascularization. Placental cryostat sections (8 μm) were thawed, fixed in ice-cold acetone for 10 min, washed thrice in PBS, and non-specific binding was blocked using 2% bovine serum albumin (BSA) in PBS for 60 min. Sections were incubated overnight at 4°C with a primary antibody for nitrotyrosine (1:10; mouse-anti-tyrosine, NOVUS Biologicals, Oakville, ON, Canada) or CD31 (1:200 mouse-anti-CD31/PECAM-1, NOVUS Biologicals) in 2% BSA/PBS. The next day, sections were washed thrice with PBS and incubated with secondary antibody [1:250 in 2% BSA/PBS; donkey anti-mouse IgG (H + L), AF488, Alexa, Invitrogen] for 1 h at room temperature. Sections were washed with PBS thrice, mounting medium containing DAPI was added (Vector Laboratories; Burlingame, CA, U.S.A) and slides were covered, protected from light and left to dry overnight. Images were taken the next day.
Immunofluorescent Staining for Placental HIF-1α Expression
Placental levels of the transcription factor hypoxia-inducible factor-1α (HIF-1α) were measured as a marker of hypoxia. Placental PFA-fixed sections (8 μm) were dewaxed in xylene and rehydrated in ethanol (100, 95 and 80%). Endogenous peroxidase activity was blocked using 10% H2O2 in distilled water for 10 min and incubated in sodium citrate buffer supplemented with 0.05% Tween 20 at 90°C for 20 min for antigen retrieval. Non-specific staining was blocked with 2% BSA/PBS for 60 min at room temperature and incubated overnight at 4°C with a primary antibody against HIF-1α (1:250; rabbit-anti-HIF1 alpha, NOVUS Biologicals, Oakville, ON, Canada) in 2% BSA/PBS. The next day, sections were washed twice with Tris buffered saline containing 0.05% Tween 20 (TBS-T) and incubated with secondary antibody [1:250; goat-anti-rabbit IgG (H + L)-AF546, Invitrogen, Carlsbad, CA, USA] in TBS-T for 60 min at room temperature. After incubation with secondary antibody, sections were washed twice in TBS-T, once in distilled water and mounted using mounting medium containing DAPI (Vector Laboratories). Slides were protected from light and left to dry overnight. Images were taken the next day.
Immunofluorescent Staining for Placental and Fetal Tissue Oxygenation
In a separate group of dams, tissue oxygenation levels were assessed by intraperitoneal (i.p.) injection of either pimonidazole hydrochloride (60 mg/kg) (Hypoxyprobe™-1, Burlington, USA) or an equivalent volume of vehicle (saline) as a control on GD20. Six hours post injection, dams were euthanized, and placentae, fetal hearts, and fetal liver were collected and snap frozen. Pimonidazole levels in placental tissues and fetal cardiac and hepatic tissues from both sexes were assessed by immunostaining. Pimonidazole hydrochloride (also known as 2-nitroimidazoles) distributes to all tissues but is activated by reduction in cells exposed to oxygen concentration less than 14 micromolar, which is equivalent to a partial pressure pO2 = 10 mmHg at 37°C. The activated intermediate forms stable adducts with proteins containing thiol groups (i.e., reduced pimonidazole, the staining product). Placental cryosections (8 μm) were fixed in acetone (10 min) and washed in PBS thrice, and non-specific staining was blocked using 2% BSA/PBS for 1 h. Sections were incubated overnight at 4°C with monoclonal anti-pimonidazole antibody (1:200; Hypoxyprobe™ Kit) in 2% BSA/PBS. The next day, sections were washed thrice in PBS and incubated with secondary antibody [1:250; donkey anti-mouse IgG (H + L), AF488, Alexa, Invitrogen] in 2% BSA/PBS for 60 min. Sections were washed with PBS three times and mounted using mounting medium containing DAPI (Vector Laboratories). Slides were protected from light and left to dry overnight. Images were taken the next day.
Morphological Analysis of Placenta
Using an established hematoxylin and eosin (H&E) staining protocol, placental PFA-fixed sections (8 μm) were dewaxed in histoclear, rehydrated, stained with filtered Harris’s hematoxylin for 3 min, washed with distilled water, and then put into filtered eosin for 30 s. The placental sections were washed in cold water, covered, and left to dry overnight.
Image Analysis
All images were taken on an Olympus IX81 fluorescence microscope with CellSens Dimensions software (Olympus, Japan) with TRITC at 532 nm (for DHE, HIF-1α staining) or FITC at 488 nm (for nitrotyrosine, DAF-FM and CD31 staining) wavelength, respectively. Three representative pictures of the placental labyrinth zone were randomly taken from each of the tissue section at 20× magnification. All pictures were corrected to the blank controls (i.e., samples without DHE, or samples incubated only with secondary antibodies) to remove background staining. Fluorescent images were analyzed using ImageJ 1.48 software (National Institutes of Health, Bethesda, MD, USA) to determine mean fluorescence intensity (MFI). MFI values from the DHE, nitrotyrosine, and pimonidazole staining were normalized to the nuclei counts per image. The average MFI of the three representative images per experimental group was taken.
For placental morphological analysis, images were taken with a digital camera mounted on a bright field microscope (EVOS XL Core Imaging System, Thermo Fisher Scientific, Canada) at a 2× magnification. For the other assessments, three randomly selected representative fields from each placenta were obtained at a magnification of 40×. Then, using ImageJ software, placental blood space area in each field of view was converted into black and remaining placental tissue into white for quantification. Total area of fetal blood space per field of view was calculated using ImageJ software, and the values were averaged per experimental group. Briefly, images were opened in ImageJ and converted into 16 bit binary images. Following which a threshold was set automatically by the program, which converted the placental blood space area into black and remaining placental tissue into white. Particle count of placental blood space was analyzed on ImageJ using the option of “analyze particles” in the software, which resulted in a surface area value for the black space, i.e., the placental blood space.
Real-Time RT-PCR for Placental Gene Expression of IGF and VEGFA
Total RNA was isolated from the placental labyrinth using RNeasy plus Mini Kit (QIAGEN Inc., Ontario, Canada). Total RNA was reverse transcribed to cDNA using the High Capacity cDNA Reverse Transcription Kit (AB Applied Biosystems, Warrington, UK) according to the manufacturer’s instructions. Using gene-specific primers for Igf2, Igf2P0, Igf1r, Igf2r, and Vegfa, quantitative real time RT-PCR (qPCR) was performed using iQ™ SYBR Green Supermix (Bio-Rad, Hercules, CA, USA; see Table 1 for primers). Briefly, thermal cycling was initiated by a 5 min. Denaturation at 95°C, followed by 40 cycles of 95°C for 30 s, annealing at 60°C for 15 s, and 72°C for 30 s. Samples without reverse transcriptase (RT) using the same PCR primers were done as a control for the presence of genomic DNA. The gene expression levels in each sample (absolute quantification) were calculated from the standard curve (for each primer set) and normalized to rat Cyclophilin A (PPIA) expression.
Statistical Analysis
Statistical analyses were performed using GraphPad Prism 7.04 software (GraphPad Software, U.S.A.). All data are expressed as mean ± SEM. All data were analyzed using a two-way ANOVA, with hypoxia and nMitoQ treatment as the two independent factors, followed by Sidak’s multiple comparison post hoc tests. A value of p < 0.05 was considered significant.
Results
Offspring and Placental Characteristics
In male fetuses, prenatal hypoxia decreased fetal weight and abdominal girth without affecting placental weight (Table 2). nMitoQ treatment increased male abdominal girth in the prenatal hypoxia exposed group, while no effects of nMitoQ treatment were observed on fetal or placental weights in males (Table 2). Prenatal hypoxia reduced placental efficiency and expressed as ratio of fetal weight/placental weight in male fetuses, which was not significantly improved by nMitoQ treatment; however, placental efficiency in the hypoxia/nMitoQ group was no longer significantly different than normoxia/saline controls (Table 2), suggesting an effect of nMitoQ treatment. Crown-to-rump length was similar between all experimental groups in male fetuses (Table 2).
In female fetuses, prenatal hypoxia had no effect on fetal weight or abdominal girth but increased placental weight, which was significantly reduced by nMitoQ treatment (Table 3). nMitoQ treatment had no effect on female fetal weight or abdominal girth (Table 3). Prenatal hypoxia reduced placental efficiency and expressed as ratio of fetal weight/placental weight in female fetuses, which was not significantly improved by nMitoQ treatment; however, placental efficiency in the hypoxia/nMitoQ group was no longer significantly different normoxia/saline controls (Table 3). Crown-to-rump length was similar between all experimental groups in female fetuses (Table 3).
nMitoQ Treatment Improved Placental Oxidative/Nitrosative Stress in Female Offspring
Superoxide and peroxynitrite levels were assessed as markers of oxidative/nitrosative stress. In male offspring, superoxide levels were significantly increased in offspring exposed to prenatal hypoxia compared to normoxic control offspring (Figure 1A). nMitoQ treatment significantly decreased placental superoxide generation in prenatally hypoxic male offspring and had no effect in the control group (Figure 1A). Nitrotyrosine levels (Figure 1B) or nitric oxide levels (Figure 1C) were not affected by prenatal hypoxia or nMitoQ treatment in placentae from male offspring.
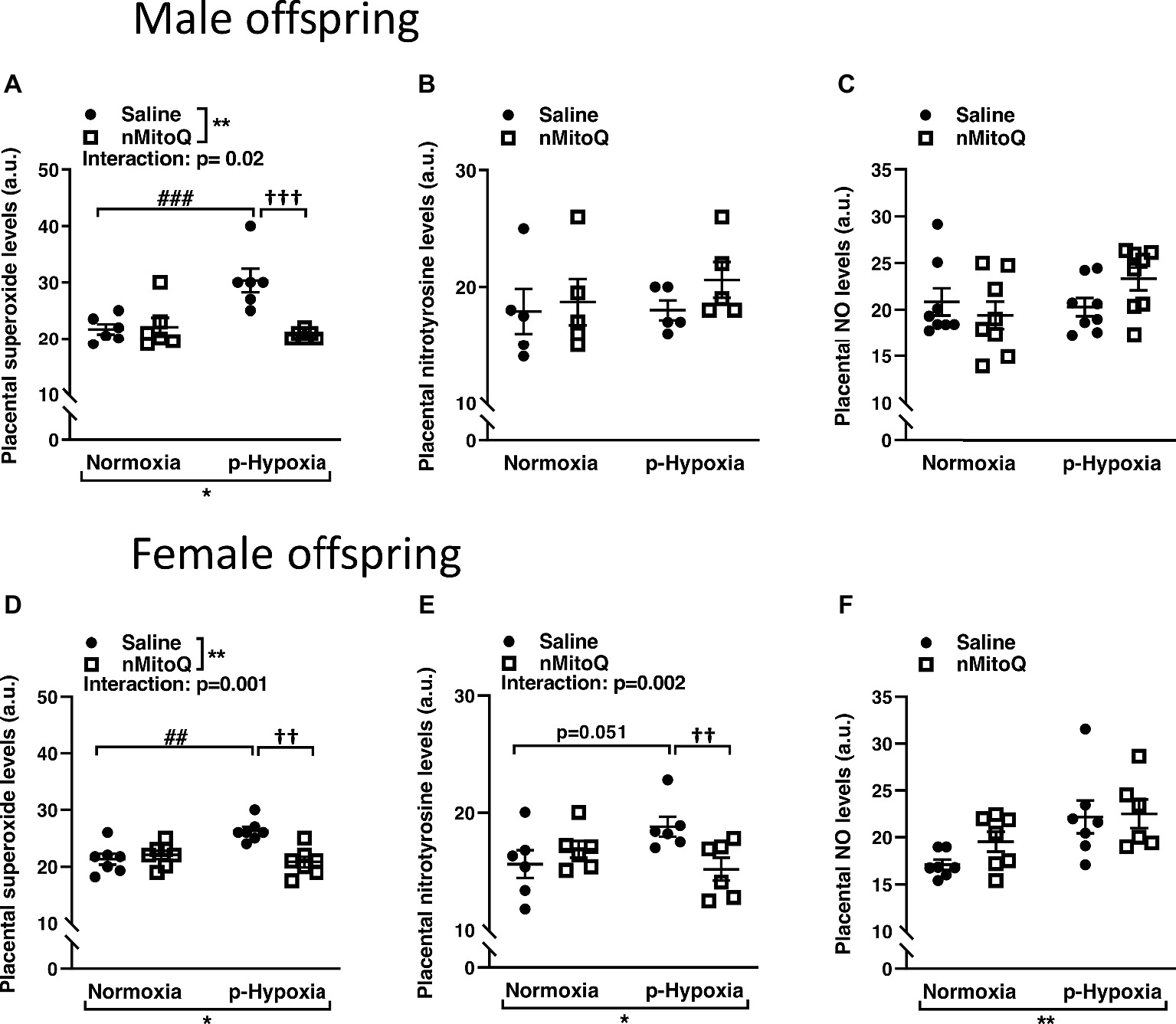
Figure 1. Effects of nMitoQ treatment on placental superoxide, peroxynitrite, and NO levels in normoxic and hypoxic placentae of both male and female offspring. Normoxic and hypoxic dams were treated with nMitoQ or saline, and superoxide levels were assessed by DHE staining in placentae from male (A) and female (D) fetuses; peroxynitrite levels were detected by staining for nitrotyrosine (the footprint of peroxynitrite production) in placentae from male (B) and female (E) fetuses, and NO levels were assessed by DAF-FM staining in placentae from male (C) and female (F) fetuses on GD21. Data are represented as mean ± SEM. a.u.: arbitrary units. All groups were compared using a two-way ANOVA followed by Sidak’s post hoc test (n = 6–7/group). *p < 0.05, **p < 0.01 group effect of prenatal hypoxia or nMitoQ treatment; ##p < 0.01, ###p < 0.001 compared to normoxia-saline; ††p < 0.01, †††p < 0.001 compared to hypoxia-saline group.
In female offspring, superoxide levels significantly increased in offspring exposed to prenatal hypoxia were significantly decreased by nMitoQ treatment in prenatally hypoxic offspring and had no effect in the control group (Figure 1D). Nitrotyrosine levels tended to be increased in the placentae of prenatally hypoxic female offspring (Figure 1E). Moreover, there was a significant interaction between nMitoQ treatment and prenatal hypoxia exposure in which nMitoQ treatment decreased nitrotyrosine levels in placentae of only female hypoxic offspring (Figure 1E). However, hypoxia significantly increased nitric oxide levels in placentae from female offspring, but there was no effect of nMitoQ treatment (Figure 1F).
nMitoQ Treatment Decreased Markers of Placental and Fetal Hypoxia in Female Offspring
We next assessed HIF1-α protein levels as a marker for tissue hypoxia and confirmed placental oxygenation levels by pimonidazole staining. HIF1-α expression was significantly increased in prenatal hypoxia-exposed placentas from male offspring, which was not reduced by nMitoQ treatment (Figure 2A). There was a significant interaction between nMitoQ treatment and prenatal hypoxia in male placentae, whereby nMitoQ increased HIF1-α expression in placentae from normoxic male offspring (Figure 2A). Placental oxygenation was decreased (as shown by increased pimonidazole staining) in placentae of male offspring, which was unaffected by nMitoQ treatment (Figure 2B). Prenatal hypoxia decreased oxygenation in fetal hearts of only male offspring, while nMitoQ increased oxygenation in only prenatally hypoxic hearts of male offspring (Figure 2C). Hepatic oxygenation was reduced in livers of male offspring, but nMitoQ treatment had no effect (Figure 2D).
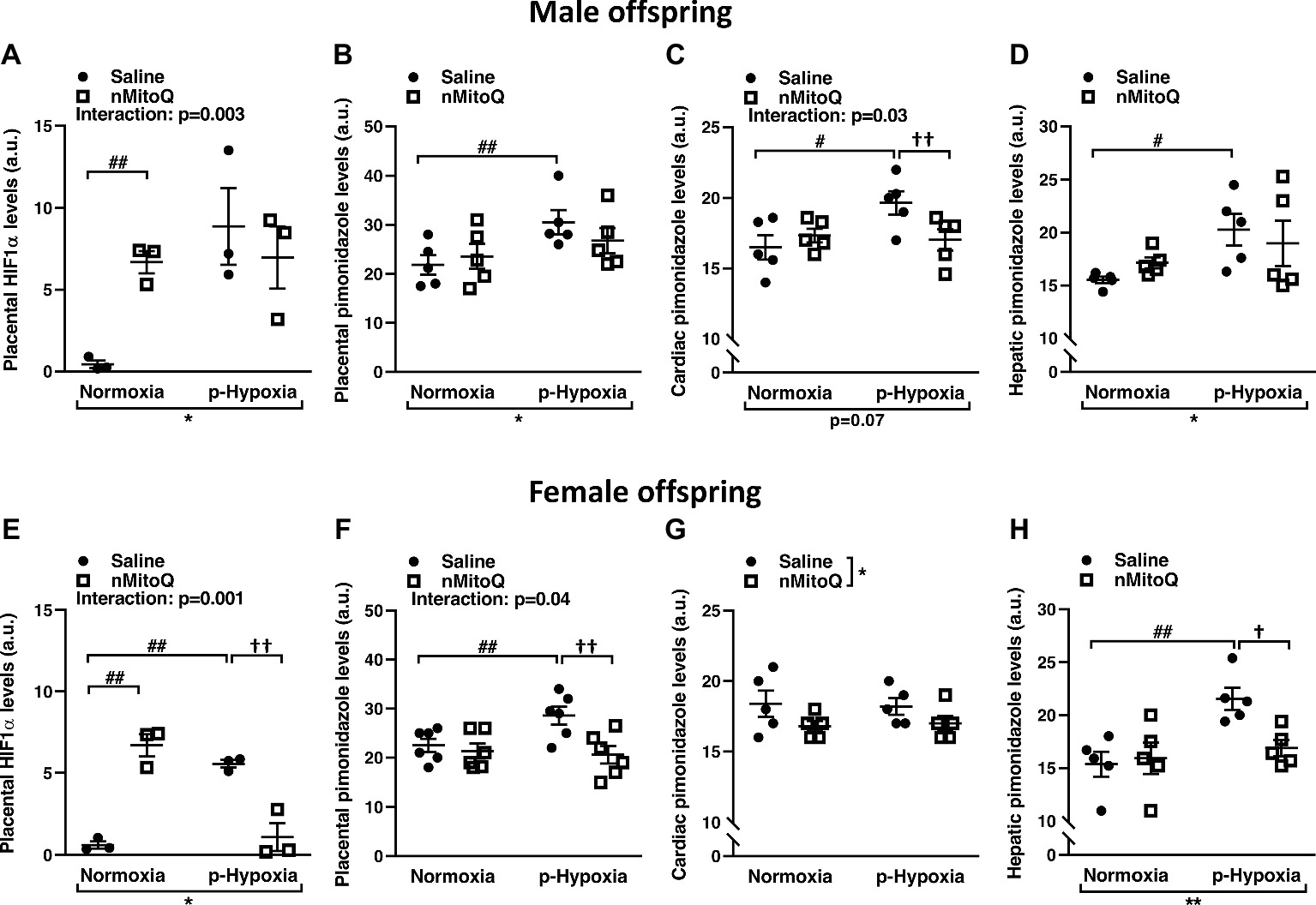
Figure 2. Effects of nMitoQ treatment on placental and fetal hypoxia. Expression of HIF1-α protein, a marker for tissue hypoxia in placentae obtained from male (A) and female (E) fetuses on GD21. Oxygenation levels as assessed by pimonidazole staining in the placentae of male (B) and female (F), the cardiac tissues of male (C) and female (G) and hepatic tissues of male (D) and female (H) fetuses on GD21. Data are represented as mean ± SEM. a.u.: arbitrary units. All groups were compared using a two-way ANOVA followed by Sidak’s post hoc test (n = 5–6/group). *p < 0.05, **p < 0.01 group effect of prenatal hypoxia and nMitoQ treatment; #p < 0.05, ##p < 0.01 compared to normoxia-saline; †p < 0.05, ††p < 0.01 compared to hypoxia-saline group.
Prenatal hypoxia significantly increased placental HIF1-α expression in female offspring (Figure 2E). nMitoQ treatment decreased HIF1-α expression in placentae from female offspring exposed to prenatal hypoxia (Figure 2E). There was a significant interaction between nMitoQ treatment and prenatal hypoxia in female placentae, whereby nMitoQ increased HIF1-α expression in placentae from normoxic female offspring (Figure 2E). Placental oxygenation was also decreased (as shown by increased pimonidazole staining) in placentae of female offspring exposed to prenatal hypoxia (Figure 2F). nMitoQ treatment significantly increased oxygenation in placenta of only female offspring exposed to prenatal hypoxia (Figure 2F). There was no effect of prenatal hypoxia on oxygenation in fetal hearts of female offspring, while nMitoQ increased oxygenation in hearts of female offspring (Figure 2G). Hepatic oxygenation was reduced in livers of prenatally hypoxic female offspring, and nMitoQ treatment increased oxygenation in livers of only female offspring exposed to prenatal hypoxia (Figure 2H).
nMitoQ Treatment Increased Angiogenesis and Vascularization in Placentae of Female Offspring
Placental hypoxia is commonly associated with altered expression of the placental pro-angiogenic peptide vascular endothelial growth factor (VEGF; Lyall et al., 1997; Roh et al., 2005). In male offspring, prenatal hypoxia decreased placental Vegf mRNA expression, which was not altered by nMitoQ treatment (Figure 3A). Prenatal hypoxia also reduced CD31-positive area of staining (i.e., the fetal capillaries; Figures 3B,C). There was a significant interaction between nMitoQ treatment and prenatal hypoxia in male placentae, whereby nMitoQ reduced both Vegf and CD31 staining in placentae from normoxic male offspring (Figures 3A,B).
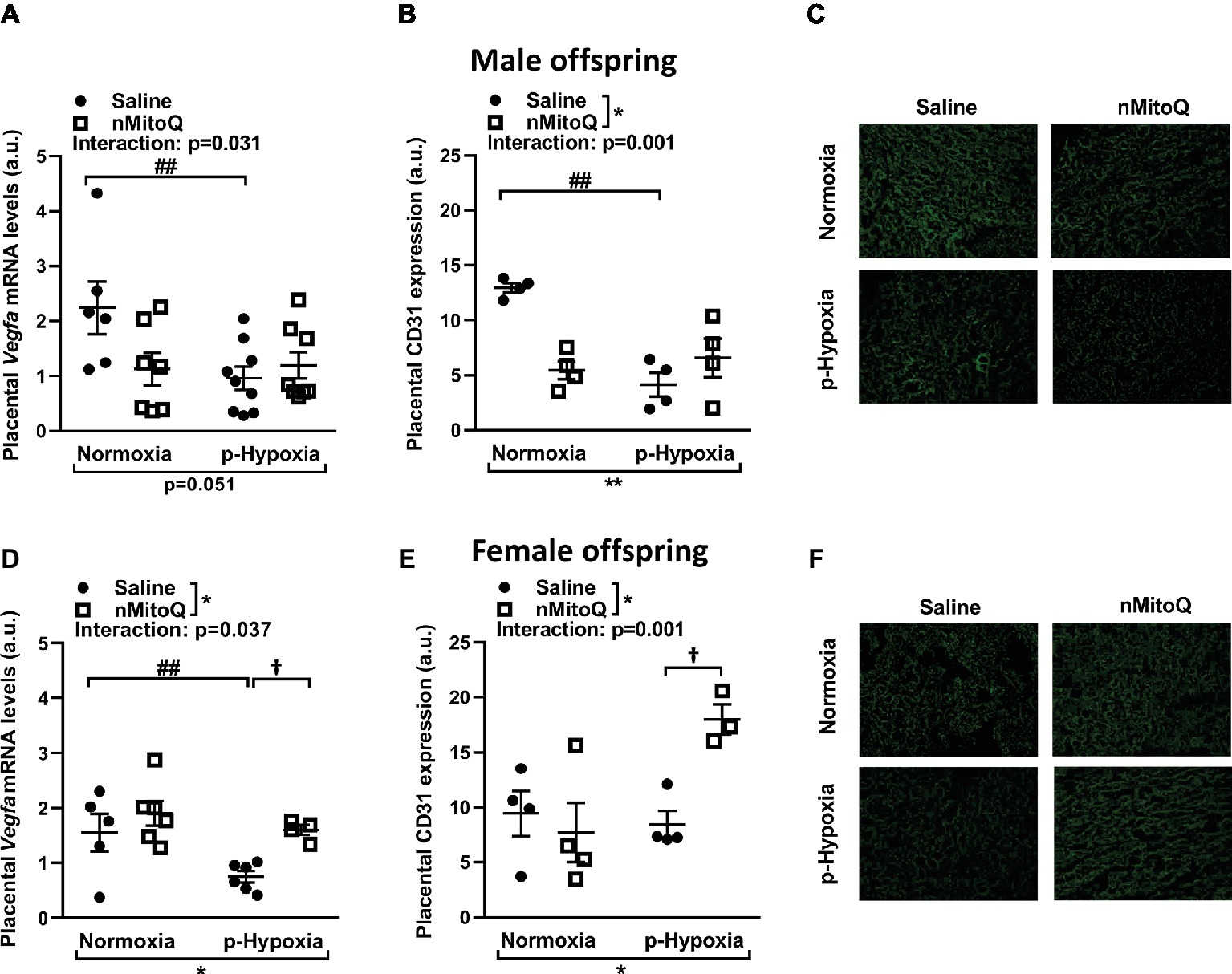
Figure 3. Effects of nMitoQ treatment on markers of angiogenesis and vascularization in prenatally hypoxic placentae of both male female offspring. Proangiogenic factor Vegfa mRNA levels were assessed by qPCR in placental tissue obtained from male (A) and female (D) fetuses on GD21. Feto-placental vascular capillaries as assessed by CD31 staining in placentae obtained from male (B) and female (E) fetuses. Representative images of CD31 stained placental labyrinth sections in placentae of male (C) and female offspring (F). Data are represented as mean ± SEM. a.u.: arbitrary units. All groups were compared using a two-way ANOVA followed by Sidak’s post hoc test (n = 5–9/group). *p < 0.05, **p < 0.01 group effect of prenatal hypoxia and nMitoQ treatment; ##p < 0.01 compared to normoxia-saline; †p < 0.05 compared to hypoxia-saline group.
In female offspring, prenatal hypoxia decreased placental Vegf mRNA expression, which was increased by nMitoQ treatment in placentae from prenatally hypoxic female offspring (Figure 3D). CD31-positive area of staining (i.e., the fetal capillaries) was reduced by prenatal hypoxia in placentae from female offspring (Figures 3E,F). Similar to the Vegf expression pattern, there was a significant interaction between nMitoQ treatment and prenatal hypoxia in female placentae, whereby nMitoQ increased CD31 staining only in the placentae from prenatal hypoxic female offspring (Figure 3E).
Sufficient fetal blood space in the placental labyrinth zone is essential for oxygen and nutrient exchange between the maternal and fetal circulations (Burton and Fowden, 2015). We found that prenatal hypoxia reduced fetal and maternal blood space area in placentae from only male offspring (Figures 4A,B). nMitoQ did not change fetal and maternal blood space area in placentae from males (Figure 4A); however, in female offspring, nMitoQ treatment increased fetal blood space area in placentae exposed to prenatal hypoxia (Figures 4C,D).
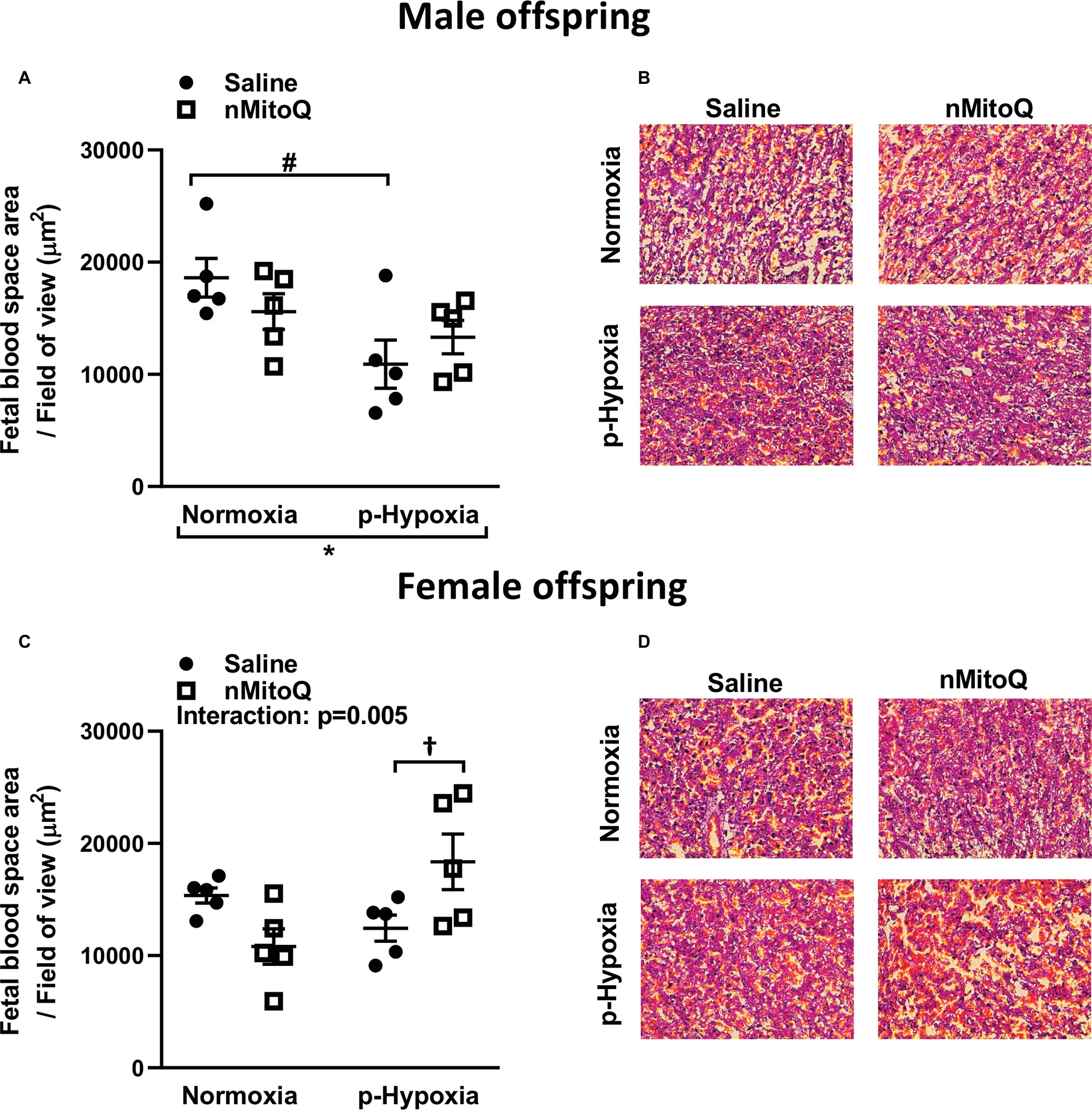
Figure 4. Effects of nMitoQ treatment on fetal blood space area in prenatally hypoxic placentae of both male and female offspring. Fetal blood space area per field of view in labyrinth zones of placenta obtained from male (A) and female (C) fetuses on GD21. Representative images of H&E stained placental labyrinth sections in placentae of males (B) and females (D). Data are represented as mean ± SEM. All groups were compared using a two-way ANOVA followed by Sidak’s post hoc test (n = 5/group). *p < 0.05 group effect of prenatal hypoxia, #p < 0.05 compared to normoxia-saline, †p < 0.05 compared to hypoxia-saline group.
nMitoQ Treatment Increased Placental Igf2 in Female Offspring
IGF2 plays an important role in placental development (Fowden et al., 2006), and total placental Igf2 and Igf2 expressed only in the placental labyrinth region (i.e., Igf2P0) were shown to regulate the nutrient exchange characteristics of the placenta (Sibley et al., 2004). In male offspring, prenatal hypoxia decreased total Igf2 and placental-specific Igf2 expression, which was not affected by nMitoQ treatment (Figures 5A,B). In placentae from female offspring, total Igf2 and placental-specific Igf2 expression were significantly decreased by prenatal hypoxia (Figures 5C,D). Moreover, nMitoQ treatment increased Igf2 expression in the prenatal hypoxia-exposed female placentae only (Figure 5C). Expression levels of the IGF2 receptors, Igf1r and Igf2r, were decreased in placentae of both prenatally hypoxic male and female offspring but were not affected by nMitoQ treatment (Figures 6A–D).
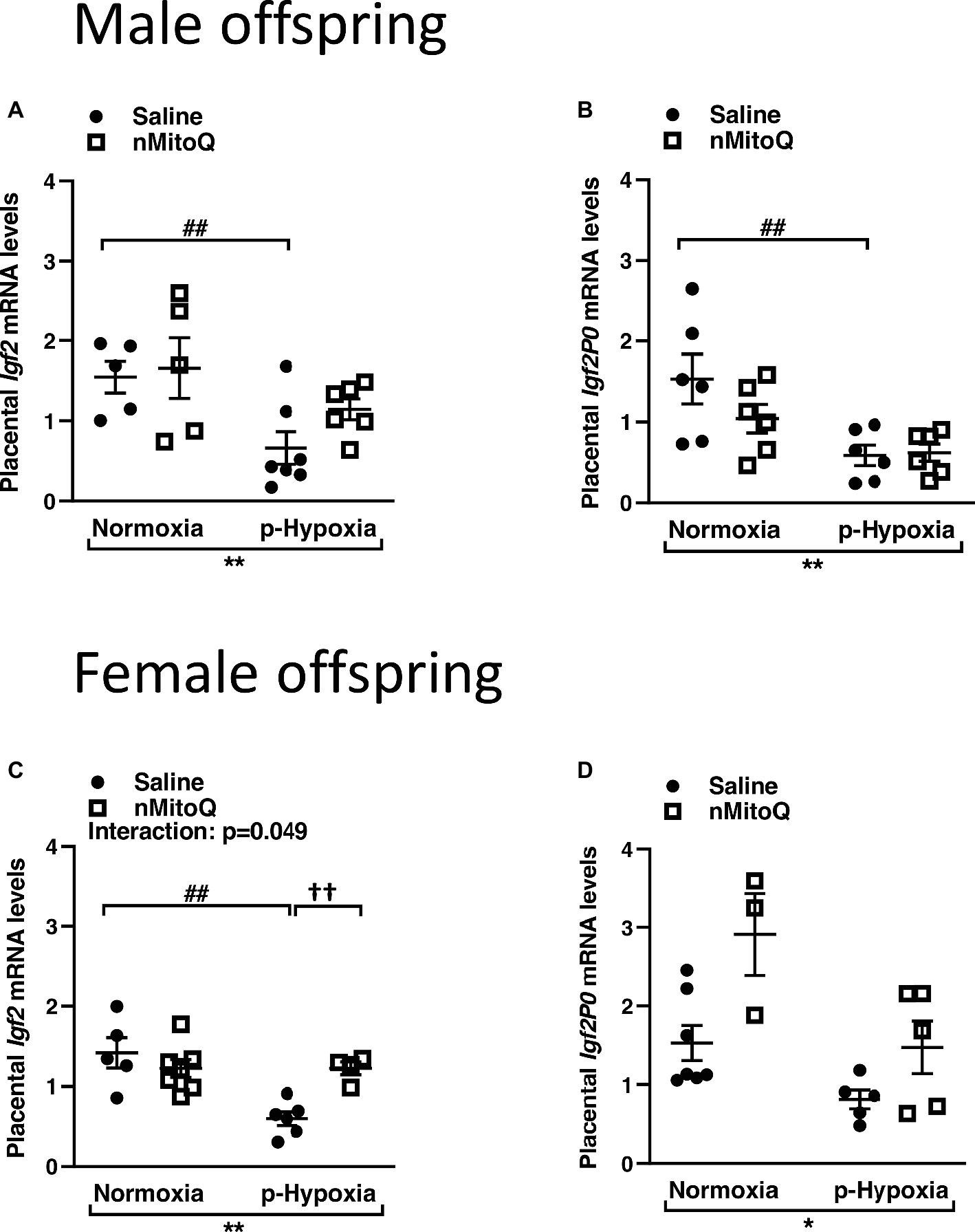
Figure 5. Effects of nMitoQ on placental Igf2 mRNA expression in prenatally hypoxic placentae of both male and female offspring. Growth factor Igf2 (A,C) and Igf2p0 (B,D) mRNA levels as assessed by qPCR in placentae obtained from male (A,B) and female (C,D) fetuses on GD21. Data are represented as mean ± SEM. All groups were compared using a two-way ANOVA followed by Sidak’s post hoc test (n = 5–9/group). *p < 0.05, **p < 0.01 group effect of prenatal hypoxia; ##p < 0.01 compared to normoxia-saline; ††p < 0.01 compared to hypoxia-saline group.
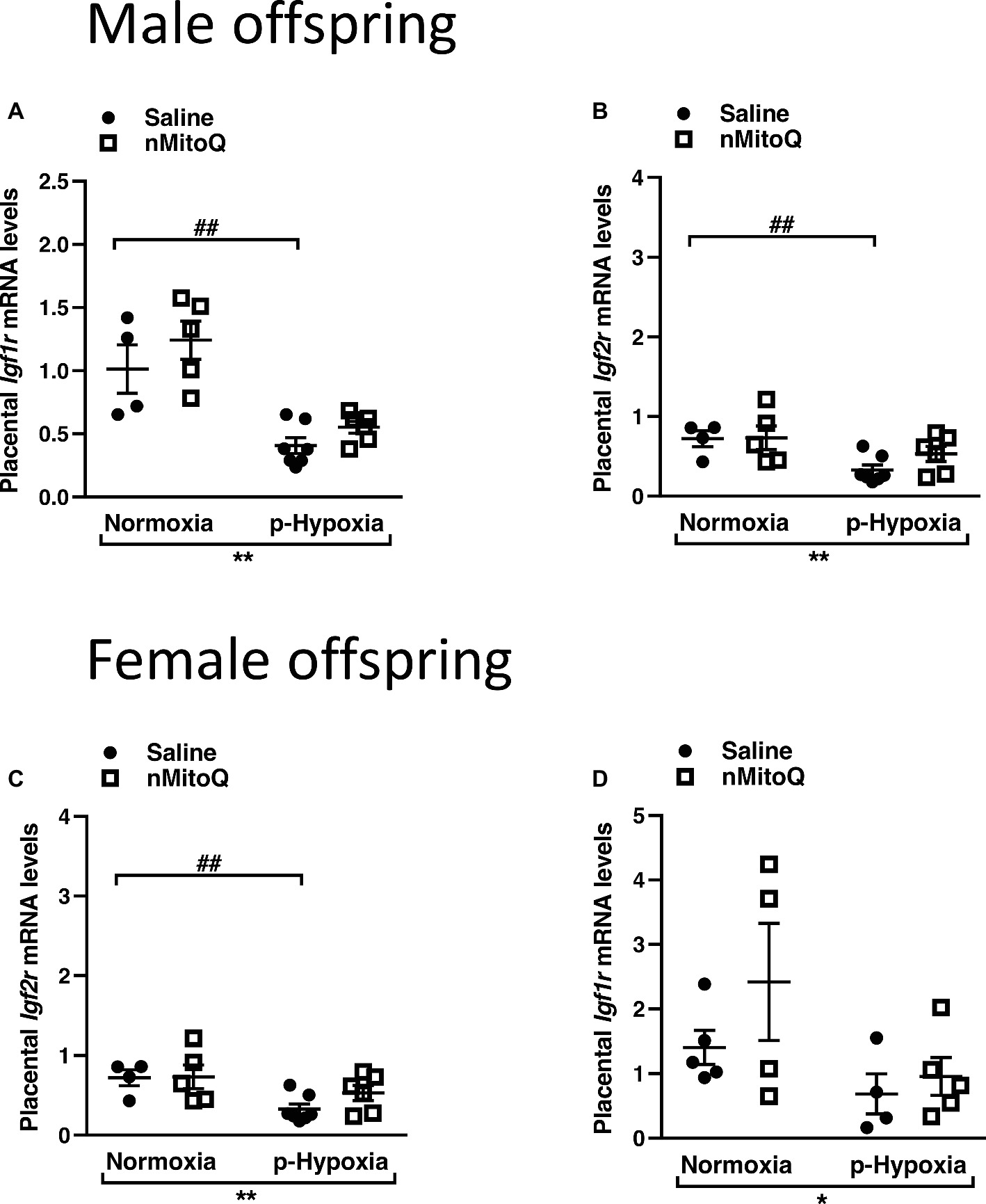
Figure 6. Effects of nMitoQ treatment on Igf1r and Igf2r mRNA expression in prenatally hypoxic placentae of both male and female offspring. Receptors for IGF2, Igf1r (A,C) and Igf2r (B,D) mRNA levels were assessed by qPCR in the labyrinth zones of placentae taken from male (A,B) and female (C,D) fetuses on GD21. Data are represented as mean ± SEM. All groups were compared using a two-way ANOVA followed by Sidak’s post hoc test (n = 4/group). *p < 0.05, **p < 0.01 group effect of prenatal hypoxia or nMitoQ treatment; ## p < 0.01 compared to normoxia-saline.
Discussion
In the current study, we hypothesized the sex-specific effects of preventing placental oxidative/nitrosative stress using nMitoQ, with a specific focus on placental morphology. This is of particular interest because oxidative stress in the placenta has been implicated to play a key role in the etiology of pregnancy complications such as fetal hypoxia and IUGR. Hence, our study was designed to elucidate possible mechanism(s) in the placenta that may account for differences in placental and fetal oxygenation and weights following a hypoxic insult and the sexually dimorphic effects of nMitoQ treatment. We showed that a reduction in placental ROS/RNS by nMitoQ treatment improved oxygenation, increased expression of VEGFA (known to affect placental morphology), and increased expression of Igf2. Moreover, nMitoQ treatment was more effective in placentae of female offspring than males. Our data demonstrated that the placental response to prenatal hypoxia is different in males versus females and that within the same dams, the response of the placentae to nMitoQ treatment is sexually dimorphic, resulting in sex differences in the effect of nMitoQ treatment on placental oxidative stress, vascularization, and placental and fetal growth.
Given that oxidative stress is a central mediator of placental dysfunction, we and others have studied the use of an antioxidant to protect the placenta against oxidative stress (Phillips et al., 2017; Nuzzo et al., 2018). Recently, the antioxidant benefits of MitoQ treatment on placental adaptations from hypoxic pregnancies were extensively studied in male offspring (Nuzzo et al., 2018). Nuzzo et al. showed that MitoQ given daily to hypoxic pregnant dams in drinking water from GD6 to GD20 restored placental efficiency to control levels, increased absolute placental volume, fetal capillary surface area in placental labyrinth zone, and maternal blood spaces related to placentae from normoxic pregnancies (Nuzzo et al., 2018). The placental morphological adaptations (i.e., increased placental weight, increased fetal capillary surface area) to early-onset hypoxia (GD6–GD20) demonstrated in males were contrary to the placental morphological phenotype (i.e., unchanged placental weight and reduced fetal capillary area) observed in our model of late-onset hypoxia (GD15 –GD21) in male offspring. Thus, diverse prenatal hypoxia insults can affect pregnancies differently, which need to be taken into consideration for clinical translation of findings. In our study, using MitoQ encapsulated in nanoparticles (nMitoQ) to target the placenta in order to limit direct drug exposure to the fetus and avoid potential off-target effects during fetal development, we also observed beneficial effects; however, nMitoQ treatment was more effective in placentae of female offspring than males.
In our model of prenatal hypoxia, placental efficiency (fetal weight/placental weight) was reduced in both male and female fetuses, which was partially improved by nMitoQ treatment in both males and females. Interestingly, fetal weight was reduced by prenatal hypoxia only in males but not females, indicating that males may be more severely affected by prenatal hypoxia than females, as has been suggested in other studies (Stark et al., 2011; Muralimanoharan et al., 2015; Evans and Myatt, 2017). This reduction in fetal weight suggests insufficient oxygen and nutrient delivery to the fetus in the hypoxia exposed dams. Interestingly, in females, prenatal hypoxia increased placental weight, which was prevented by nMitoQ treatment. This may suggest that females, in compensation for the hypoxic environment, increased placental growth in order to increase oxygen and nutrient supply to the fetus and prevent growth restriction, which was indeed successful, as fetal weights in the females were not affected by prenatal hypoxia.
We showed that prenatal hypoxia increased oxidative stress (superoxide generation in male and female placentae), as demonstrated previously (Richter et al., 2012; Aljunaidy et al., 2018; Nuzzo et al., 2018), and nitrosative stress (peroxynitrite formation in only female placentae). Oxidative and nitrosative stresses can inhibit normal placental development in various ways (e.g., inhibit mitochondrial electron transport and oxidation of DNA) and potentially reduce oxygen and nutrient delivery, thus impairing fetal development (Droge, 2002; Thompson and Al-Hasan, 2012). The nMitoQ treatment was therefore specifically designed to prevent oxidative stress in the placenta, and indeed, nMitoQ treatment significantly decreased placental superoxide and peroxynitrite levels. This supports our hypothesis that using MitoQ loaded nanoparticles as a targeted delivery system to the placenta is an effective treatment against the generation of reactive oxygen/nitrogen species in the placenta in compromised pregnancies. Interestingly, in female offspring, we observed that the increased superoxide/peroxynitrite levels by prenatal hypoxia coincided with increased NO levels. Under normal pregnancy conditions, NO bioavailability promotes feto-placental vasodilation and induces angiogenesis (Cooke, 2003; Kwon et al., 2004). The placental circulation lacks autonomic and cholinergic innervation, thus feto-placental angiogenesis and placental vascular resistance and function are dependent on locally derived vasoactive factors (e.g., NO) and growth factors (e.g., VEGF and IGF2; Pacher et al., 2007; Krause et al., 2011; Kulandavelu et al., 2013). In the human placenta, all nitric oxide synthase (NOS) isoforms are expressed but differentially within the tissues. Endothelial NOS (eNOS) isoform is predominantly expressed in syncytiotrophoblasts and endothelial cells (reviewed by Aban et al., 2018), while neuronal NOS (nNOS) and inducible NOS (iNOS) isoforms are expressed in the placental smooth muscle cells, syncytiotrophoblasts, extravillous trophoblasts, and hofbauer cells of the villous stroma (reviewed by Krause et al., 2011). Moreover, studies have correlated increased placental vascular resistance and placental dysfunction to decreased eNOS and increased inducible NOS (iNOS) isoform expression, leading to increased nitrosative stress (Casanello and Sobrevia, 2002; Giannubilo et al., 2008). Interestingly, IUGR has been associated with higher levels of placental NO, together with increased nitrosative stress and inadequate feto-placental vascularization (Lyall et al., 1996; Tikvica et al., 2008; Myatt, 2010). Therefore, the increased NO levels in female placentae may be an immediate adaptive mechanism to prenatal hypoxia, which may be associated with placental vascular dysfunction. Although we have not assessed NOS isoforms and the contribution of various cell types to the increased placental NO levels in females, the above studies may suggest that increased iNOS expression and/or activity could be a potential source for increased placental NO observed in our model of prenatal hypoxia.
We found that prenatal hypoxia decreased placental and fetal oxygenation (in males and females), which coincided with an increase in HIF-1α levels in both sexes. Systemic and placental responses to hypoxia are orchestrated by hypoxia-inducible factors (such as HIF-1α), and being a marker of hypoxia, this finding is in accordance with the reduced placental oxygenation we observed. Notably, in only the female prenatally hypoxic placentae, nMitoQ treatment effectively improved placental oxygenation. Moreover, the effect of prenatal hypoxia on oxygenation was more pronounced in the female fetal liver than the fetal heart, as previously reported by our laboratory, but only in male offspring (Rueda-Clausen et al., 2011). Interestingly, nMitoQ treatment improved oxygenation in the hearts of both male and female fetuses, but in the liver, only female fetuses showed improved oxygenation with nMitoQ. Moreover, placental HIF-1α was only decreased by nMitoQ in the female placentae. This may explain why the female fetuses did not show any signs of significant growth restriction, as nMitoQ effectively decreased oxidative stress and HIF-1α and reduced placental weight in the female placentae. The underlying mechanisms for reduced effectiveness of nMitoQ treatment in males are not fully understood and remain to be further studied. A possible explanation might be that males show greater growth rate in utero (Parker et al., 1984); therefore, the higher oxygen and nutrient demand by the male fetus may predispose them to a greater risk of adverse developmental outcomes following the oxygen deprivation (Ozaki et al., 2001).
Oxidative stress is known to contribute to abnormal placental growth, function, and angiogenesis (Burton et al., 2009). As mentioned above, the placenta lacks autonomic innervation, and locally derived NO and growth factors such as the pro-angiogenic factor VEGF play essential roles in placental vascular development and function (Kulandavelu et al., 2013). HIF-1α (in hypoxic conditions) has been shown to increase VEGFA expression, while a reduction in VEGF has been observed in placentae from complicated pregnancies such as preeclampsia (Andraweera et al., 2012; Abe et al., 2013). Therefore, placental angiogenesis is dependent on locally derived VEGFA. Our study showed that prenatal hypoxia decreased Vegfa mRNA expression and the area of fetal blood capillaries (measured by endothelial cell marker CD31) in placentae of both male and female offspring, suggesting decreased angiogenesis and vascularization. The decreased Vegfa mRNA expression could be due to decreased binding of HIF-1α to the hypoxia responsive element (HRE) on the Vegfa gene, which was previously shown by Myatt et al. to decrease Vegfa expression in preeclamptic placentae with increased ROS (Muralimanoharan et al., 2012). Interestingly, nMitoQ treatment only improved Vegfa mRNA expression, the area of fetal blood capillaries, and the placental blood space in the female placentae but not in the male placentae. The specific mechanisms remain to be investigated, but it may be speculated that this could be mediated in part by increased placental NO levels present in the prenatally hypoxic placentae, which were not affected by nMitoQ treatment and could increase VEGFA. However, the effects of NO on VEGFA expression vary for different tissues and cell types (Abe et al., 2013); therefore, NO mediated VEGFA expression within the placenta may be highly dependent on the different cell types. Our data are in accordance with the previous studies, where MitoQ (not bound to nanoparticles) was shown to increase maternal blood space surface area in the placenta in males only (Nuzzo et al., 2018). Taken together, our data could suggest that reduction of superoxide by nMitoQ could lead to enhanced angiogenesis via increased Vegfa expression and fetal capillary area and blood space in placentae of female offspring.
Another important growth factor for placental morphogenesis is IGF2 (Sferruzzi-Perri et al., 2017). In humans, decreased placental IGF2 has been associated with IUGR (Diplas et al., 2009). In mice, genetic knockouts of Igf2 showed impaired placental development and fetal growth with reduced placental transfer of nutrients to the fetus (Constancia et al., 2005; Coan et al., 2008). We observed that prenatal hypoxia decreased expression of Igf2 and Igf2P0 in the labyrinth zone of both male and female offspring. This is in line with the previous studies showing that maternal exposure to hypoxia has direct effects on total Igf2 expression in the placenta: maternal exposure to 10–12% oxygen during late gestation decreased Igf2 expression, which affected the labyrinth layer morphology and decreased maternal blood space (Cuffe et al., 2014; Higgins et al., 2016). Hence, the reduction in placental Igf2 expression could also explain the decreased fetal blood spaces by prenatal hypoxia that we observed. The expression of Igf2 is regulated by methylation of the Igf2 gene at the imprinting control region (ICR) and the differentially methylated region 2 (DMR2). Recent studies suggest that a suboptimal intrauterine environment leads to epigenetic changes in the Igf2 gene and associated Igf2 expression in growth restricted offspring of rats exposed to bilateral uterine artery ligation (Gonzalez-Rodriguez et al., 2016). Interestingly, hypoxic stress responses in general appear to be driven by epigenetic changes (Cerda and Weitzman, 1997). For example, prenatal hypoxia increased placental ROS and DNA methylation enzymes (Gheorghe et al., 2007). Therefore, we speculate that the methylation status at ICR and DMR2 may differ in a sex-specific manner, and changes in DNA methylation could account for the altered Igf2 expression, which will be the focus for our future investigations.
It is well known that the placenta functions and adapts to an adverse intrauterine environment in a sex-specific manner (reviewed by Clifton, 2010). Furthermore, generation of oxidative stress differs in both male and female fetuses and placentae under conditions of adverse prenatal stress (Chaudhari et al., 2014). Our current study further demonstrates a dichotomous sex-specific and nMitoQ-specific effect on the placenta and ultimately fetal development. Overall, our data suggested that nMitoQ treatment decreased oxidative/nitrosative stress, improved oxygenation, was effective in bringing Vegfa and Igf2 expression back to control levels, and increased fetal blood space only in female placentae, thereby showing that nMitoQ treatment may protect offspring from the detrimental effects of a hypoxic insult in a sexually dimorphic manner with increased effectiveness in females. Of note, our data showed that nMitoQ treatment also affected the placenta in the control groups. For example, in normoxic controls, nMitoQ treatment increased HIF-1α expression in both male and female placentae and reduced CD31 area in the male offspring. Previous studies have demonstrated that maternal antioxidant supplementation with ascorbic acid in normal pregnancies was associated with vascular dysfunction and weight gain (Richter et al., 2012). Therefore, continuing these studies we need to keep in mind that this treatment is specifically designed to treat pregnancies complicated by fetal hypoxia and be aware that there might be detrimental effects of maternal antioxidant intervention during normal pregnancy.
To conclude, we demonstrated that nMitoQ treatment reduced placental nitrosative stress and improved oxygenation and placental morphology via increased VEGFA and IGF2 expression in a sex-specific manner, showing more effectiveness in placentae from female offspring. Moreover, our study shows that male fetuses appear to be more susceptible to an adverse in utero environment. Although the exact mechanism(s) remain to be further investigated, a higher pro-oxidant state with reduced antioxidant capacity in the male placentae may explain the increased susceptibility of the male offspring under adverse conditions. In addition, our current study illustrates that the placenta is a contributing factor in the sexual dimorphism that has been observed in fetal programming. Thus, sex differences will need to be taken into account when developing placental-targeted therapeutic interventions to prevent fetal hypoxia and ultimately optimize fetal development in complicated pregnancies.
Data Availability
The datasets generated for this study are available on request to the corresponding author.
Ethics Statement
All procedures described were approved by the University of Alberta Animal Policy and Welfare Committee and were in accordance with the guidelines of the Canadian Council on Animal Care (AUP #242).
Author Contributions
EG, MA, FS, JM, TP, CPC, C-LC, and SD contributed to experimental design. EG, MA, and RK participated in data acquisition. EG, MA, FS, JM, C-LC, and SD analyzed the data. EG, FS, C-LC, and SD were involved in drafting of the manuscript. EG, MA, FS, JM, TP, CPC, C-LC, and SD revised the final version of the manuscript. TP and CPC provided the study materials.
Funding
This research was funded by grants from the Canadian Institutes of Health Research (CIHR FS 154313) and the Women and Children’s Health Research Institute (WCHRI) through the generosity of the Stollery Children’s Hospital Foundation and supporters of the Lois Hole Hospital for Women. SD is a Canada research chair in the Maternal and Perinatal Cardiovascular Health and a distinguished university professor at the University of Alberta. FS was supported by a Postgraduate Fellowship Award from the Alberta Innovates – Health Solutions (AIHS) and a Molly Towell Fellowship Award from the Molly Towell Perinatal Research Foundation. EG was supported by the Faculty of Medicine & Dentistry 75th Anniversary Awards and WCHRI Graduate Studentship Program.
Conflict of Interest Statement
The University of Bristol (TP and CC) has submitted a patent application for the nanoparticle formulation used in this study and its application to preeclampsia and related diseases.
The remaining authors declare that the research was conducted in the absence of any commercial or financial relationships that could be construed as a potential conflict of interest.
Acknowledgments
MitoQ was generously provided to TP and CPC by Dr. Michael Murphy, University of Cambridge, UK.
References
Aban, C. E., Accialini, P. L., Etcheverry, T., Leguizamon, G. F., Martinez, N. A., and Farina, M. G. (2018). Crosstalk between nitric oxide and endocannabinoid signaling pathways in normal and pathological placentation. Front. Physiol. 9:1699. doi: 10.3389/fphys.2018.01699
Abe, H., Ishikawa, W., Kushima, T., Nishimura, T., Mori, C., Onuki, A., et al. (2013). Nitric oxide induces vascular endothelial growth factor expression in the rat placenta in vivo and in vitro. Biosci. Biotechnol. Biochem. 77, 971–976. doi: 10.1271/bbb.120923
Al-Gubory, K. H., Fowler, P. A., and Garrel, C. (2010). The roles of cellular reactive oxygen species, oxidative stress and antioxidants in pregnancy outcomes. Int. J. Biochem. Cell Biol. 42, 1634–1650. doi: 10.1016/j.biocel.2010.06.001
Aljunaidy, M. M., Morton, J. S., Cooke, C. M., and Davidge, S. T. (2017). Prenatal hypoxia and placental oxidative stress: linkages to developmental origins of cardiovascular disease. Am. J. Physiol. Regul. Integr. Comp. Physiol. 313, R395–R399. doi: 10.1152/ajpregu.00245.2017
Aljunaidy, M. M., Morton, J. S., Kirschenman, R., Phillips, T., Case, C. P., Cooke, C. M., et al. (2018). Maternal treatment with a placental-targeted antioxidant (MitoQ) impacts offspring cardiovascular function in a rat model of prenatal hypoxia. Pharmacol. Res. 134, 332–342. doi: 10.1016/j.phrs.2018.05.006
Andraweera, P. H., Dekker, G. A., Laurence, J. A., and Roberts, C. T. (2012). Placental expression of VEGF family mRNA in adverse pregnancy outcomes. Placenta 33, 467–472. doi: 10.1016/j.placenta.2012.02.013
Burton, G. J., and Fowden, A. L. (2015). The placenta: a multifaceted, transient organ. Philos. Trans. R. Soc. Lond. Ser. B Biol. Sci. 370:20140066. doi: 10.1098/rstb.2014.0066
Burton, G. J., Yung, H. W., Cindrova-Davies, T., and Charnock-Jones, D. S. (2009). Placental endoplasmic reticulum stress and oxidative stress in the pathophysiology of unexplained intrauterine growth restriction and early onset preeclampsia. Placenta 30(Suppl. A), S43–S48.
Casanello, P., and Sobrevia, L. (2002). Intrauterine growth retardation is associated with reduced activity and expression of the cationic amino acid transport systems y+/hCAT-1 and y+/hCAT-2B and lower activity of nitric oxide synthase in human umbilical vein endothelial cells. Circ. Res. 91, 127–134. doi: 10.1161/01.RES.0000027813.55750.E7
Cerda, S., and Weitzman, S. A. (1997). Influence of oxygen radical injury on DNA methylation. Mutat. Res. 386, 141–152.
Chaudhari, H. N., Kim, S. W., and Yun, J. W. (2014). Gender-dimorphic regulation of antioxidant proteins in response to high-fat diet and sex steroid hormones in rats. Free Radic. Res. 48, 587–598. doi: 10.3109/10715762.2014.896003
Chiaratti, M. R., Malik, S., Diot, A., Rapa, E., Macleod, L., Morten, K., et al. (2015). Is placental mitochondrial function a regulator that matches fetal and placental growth to maternal nutrient intake in the mouse? PLoS One 10:e0130631. doi: 10.1371/journal.pone.0130631
Clifton, V. L. (2010). Review: sex and the human placenta: mediating differential strategies of fetal growth and survival. Placenta 31(Suppl), S33–S39. doi: 10.1016/j.placenta.2009.11.010
Coan, P. M., Fowden, A. L., Constancia, M., Ferguson-Smith, A. C., Burton, G. J., and Sibley, C. P. (2008). Disproportional effects of Igf2 knockout on placental morphology and diffusional exchange characteristics in the mouse. J. Physiol. 586, 5023–5032. doi: 10.1113/jphysiol.2008.157313
Constancia, M., Angiolini, E., Sandovici, I., Smith, P., Smith, R., Kelsey, G., et al. (2005). Adaptation of nutrient supply to fetal demand in the mouse involves interaction between the Igf2 gene and placental transporter systems. Proc. Natl. Acad. Sci. USA 102, 19219–19224. doi: 10.1073/pnas.0504468103
Cooke, J. P. (2003). NO and angiogenesis. Atheroscler. Suppl. 4, 53–60. doi: 10.1016/S1567-5688(03)00034-5
Cuffe, J. S., Walton, S. L., Singh, R. R., Spiers, J. G., Bielefeldt-Ohmann, H., Wilkinson, L., et al. (2014). Mid- to late term hypoxia in the mouse alters placental morphology, glucocorticoid regulatory pathways and nutrient transporters in a sex-specific manner. J. Physiol. 592, 3127–3141. doi: 10.1113/jphysiol.2014.272856
Diplas, A. I., Lambertini, L., Lee, M. J., Sperling, R., Lee, Y. L., Wetmur, J., et al. (2009). Differential expression of imprinted genes in normal and IUGR human placentas. Epigenetics 4, 235–240. doi: 10.4161/epi.9019
Droge, W. (2002). Free radicals in the physiological control of cell function. Physiol. Rev. 82, 47–95. doi: 10.1152/physrev.00018.2001
Evans, L., and Myatt, L. (2017). Sexual dimorphism in the effect of maternal obesity on antioxidant defense mechanisms in the human placenta. Placenta 51, 64–69. doi: 10.1016/j.placenta.2017.02.004
Fowden, A. L., Sibley, C., Reik, W., and Constancia, M. (2006). Imprinted genes, placental development and fetal growth. Horm. Res. 65(Suppl. 3), 50–58. doi: 10.1159/000091506
Gabory, A., Ferry, L., Fajardy, I., Jouneau, L., Gothie, J. D., Vige, A., et al. (2012). Maternal diets trigger sex-specific divergent trajectories of gene expression and epigenetic systems in mouse placenta. PLoS One 7:e47986. doi: 10.1371/journal.pone.0047986
Gheorghe, C. P., Mohan, S., Oberg, K. C., and Longo, L. D. (2007). Gene expression patterns in the hypoxic murine placenta: a role in epigenesis? Reprod. Sci. 14, 223–233. doi: 10.1177/1933719107302860
Giannubilo, S. R., Menegazzi, M., Tedeschi, E., Bezzeccheri, V., Suzuki, H., and Tranquilli, A. L. (2008). Doppler analysis and placental nitric oxide synthase expression during fetal growth restriction. J. Matern. Fetal. Neonatal. Med. 21, 617–622. doi: 10.1080/14767050802214774
Gonzalez-Rodriguez, P., Cantu, J., O’neil, D., Seferovic, M. D., Goodspeed, D. M., Suter, M. A., et al. (2016). Alterations in expression of imprinted genes from the H19/IGF2 loci in a multigenerational model of intrauterine growth restriction (IUGR). Am. J. Obstet. Gynecol. 214, 625.e621–625.e611. doi: 10.1016/j.ajog.2016.01.194
Higgins, J. S., Vaughan, O. R., Fernandez De Liger, E., Fowden, A. L., and Sferruzzi-Perri, A. N. (2016). Placental phenotype and resource allocation to fetal growth are modified by the timing and degree of hypoxia during mouse pregnancy. J. Physiol. 594, 1341–1356. doi: 10.1113/JP271057
Holland, O., Dekker Nitert, M., Gallo, L. A., Vejzovic, M., Fisher, J. J., and Perkins, A. V. (2017). Review: placental mitochondrial function and structure in gestational disorders. Placenta 54, 2–9. doi: 10.1016/j.placenta.2016.12.012
Kim, H., Akagi, T., and Akashi, M. (2009). Preparation of size tunable amphiphilic poly(amino acid) nanoparticles. Macromol. Biosci. 9, 842–848. doi: 10.1002/mabi.200800367
Kim, D. W., Young, S. L., Grattan, D. R., and Jasoni, C. L. (2014). Obesity during pregnancy disrupts placental morphology, cell proliferation, and inflammation in a sex-specific manner across gestation in the mouse. Biol. Reprod. 90:130. doi: 10.1095/biolreprod.113.117259
Krause, B. J., Hanson, M. A., and Casanello, P. (2011). Role of nitric oxide in placental vascular development and function. Placenta 32, 797–805. doi: 10.1016/j.placenta.2011.06.025
Kulandavelu, S., Whiteley, K. J., Bainbridge, S. A., Qu, D., and Adamson, S. L. (2013). Endothelial NO synthase augments fetoplacental blood flow, placental vascularization, and fetal growth in mice. Hypertension 61, 259–266. doi: 10.1161/HYPERTENSIONAHA.112.201996
Kwon, H., Wu, G., Meininger, C. J., Bazer, F. W., and Spencer, T. E. (2004). Developmental changes in nitric oxide synthesis in the ovine placenta. Biol. Reprod. 70, 679–686. doi: 10.1095/biolreprod.103.023184
Lappas, M., Mitton, A., and Permezel, M. (2010). In response to oxidative stress, the expression of inflammatory cytokines and antioxidant enzymes are impaired in placenta, but not adipose tissue, of women with gestational diabetes. J. Endocrinol. 204, 75–84. doi: 10.1677/JOE-09-0321
Lyall, F., Greer, I. A., Young, A., and Myatt, L. (1996). Nitric oxide concentrations are increased in the feto-placental circulation in intrauterine growth restriction. Placenta 17, 165–168. doi: 10.1016/S0143-4004(96)80009-9
Lyall, F., Young, A., Boswell, F., Kingdom, J. C., and Greer, I. A. (1997). Placental expression of vascular endothelial growth factor in placentae from pregnancies complicated by pre-eclampsia and intrauterine growth restriction does not support placental hypoxia at delivery. Placenta 18, 269–276. doi: 10.1016/S0143-4004(97)80061-6
Mando, C., De Palma, C., Stampalija, T., Anelli, G. M., Figus, M., Novielli, C., et al. (2014). Placental mitochondrial content and function in intrauterine growth restriction and preeclampsia. Am. J. Physiol. Endocrinol. Metab. 306, E404–E413. doi: 10.1152/ajpendo.00426.2013
Mao, J., Zhang, X., Sieli, P. T., Falduto, M. T., Torres, K. E., and Rosenfeld, C. S. (2010). Contrasting effects of different maternal diets on sexually dimorphic gene expression in the murine placenta. Proc. Natl. Acad. Sci. USA 107, 5557–5562. doi: 10.1073/pnas.1000440107
Marzioni, D., Tamagnone, L., Capparuccia, L., Marchini, C., Amici, A., Todros, T., et al. (2004). Restricted innervation of uterus and placenta during pregnancy: evidence for a role of the repelling signal Semaphorin 3A. Dev. Dyn. 231, 839–848. doi: 10.1002/dvdy.20178
McCarthy, C. M., and Kenny, L. C. (2016). Mitochondrial [dys]function; culprit in pre-eclampsia? Clin. Sci. 130, 1179–1184. doi: 10.1042/CS20160103
Muralimanoharan, S., Guo, C., Myatt, L., and Maloyan, A. (2015). Sexual dimorphism in miR-210 expression and mitochondrial dysfunction in the placenta with maternal obesity. Int. J. Obes. 39, 1274–1281. doi: 10.1038/ijo.2015.45
Muralimanoharan, S., Maloyan, A., Mele, J., Guo, C., Myatt, L. G., and Myatt, L. (2012). MIR-210 modulates mitochondrial respiration in placenta with preeclampsia. Placenta 33, 816–823. doi: 10.1016/j.placenta.2012.07.002
Murphy, M. P., and Smith, R. A. (2007). Targeting antioxidants to mitochondria by conjugation to lipophilic cations. Annu. Rev. Pharmacol. Toxicol. 47, 629–656. doi: 10.1146/annurev.pharmtox.47.120505.105110
Myatt, L. (2010). Review: reactive oxygen and nitrogen species and functional adaptation of the placenta. Placenta 31(Suppl), S66–S69. doi: 10.1016/j.placenta.2009.12.021
Myatt, L., and Cui, X. (2004). Oxidative stress in the placenta. Histochem. Cell Biol. 122, 369–382. doi: 10.1007/s00418-004-0677-x
Nuzzo, A. M., Camm, E. J., Sferruzzi-Perri, A. N., Ashmore, T. J., Yung, H. W., Cindrova-Davies, T., et al. (2018). Placental adaptation to early-onset hypoxic pregnancy and mitochondria-targeted antioxidant therapy in a rodent model. Am. J. Pathol. 188, 2704–2716. doi: 10.1016/j.ajpath.2018.07.027
Ozaki, T., Nishina, H., Hanson, M. A., and Poston, L. (2001). Dietary restriction in pregnant rats causes gender-related hypertension and vascular dysfunction in offspring. J. Physiol. 530, 141–152. doi: 10.1111/j.1469-7793.2001.0141m.x
Pacher, P., Beckman, J. S., and Liaudet, L. (2007). Nitric oxide and peroxynitrite in health and disease. Physiol. Rev. 87, 315–424. doi: 10.1152/physrev.00029.2006
Parker, A. J., Davies, P., Mayho, A. M., and Newton, J. R. (1984). The ultrasound estimation of sex-related variations of intrauterine growth. Am. J. Obstet. Gynecol. 149, 665–669. doi: 10.1016/0002-9378(84)90255-2
Phillips, T. J., Scott, H., Menassa, D. A., Bignell, A. L., Sood, A., Morton, J. S., et al. (2017). Treating the placenta to prevent adverse effects of gestational hypoxia on fetal brain development. Sci. Rep. 7:9079. doi: 10.1038/s41598-017-06300-1
Reynolds, L. P., and Redmer, D. A. (2001). Angiogenesis in the placenta. Biol. Reprod. 64, 1033–1040. doi: 10.1095/biolreprod64.4.1033
Richter, H. G., Camm, E. J., Modi, B. N., Naeem, F., Cross, C. M., Cindrova-Davies, T., et al. (2012). Ascorbate prevents placental oxidative stress and enhances birth weight in hypoxic pregnancy in rats. J. Physiol. 590, 1377–1387. doi: 10.1113/jphysiol.2011.226340
Roh, C. R., Budhraja, V., Kim, H. S., Nelson, D. M., and Sadovsky, Y. (2005). Microarray-based identification of differentially expressed genes in hypoxic term human trophoblasts and in placental villi of pregnancies with growth restricted fetuses. Placenta 26, 319–328. doi: 10.1016/j.placenta.2004.06.013
Rosenfeld, C. S. (2015). Sex-specific placental responses in fetal development. Endocrinology 156, 3422–3434. doi: 10.1210/en.2015-1227
Ross, M. F., Kelso, G. F., Blaikie, F. H., James, A. M., Cocheme, H. M., Filipovska, A., et al. (2005). Lipophilic triphenylphosphonium cations as tools in mitochondrial bioenergetics and free radical biology. Biochemistry 70, 222–230. doi: 10.1007/s10541-005-0104-5
Rueda-Clausen, C. F., Morton, J. S., Lopaschuk, G. D., and Davidge, S. T. (2011). Long-term effects of intrauterine growth restriction on cardiac metabolism and susceptibility to ischaemia/reperfusion. Cardiovasc. Res. 90, 285–294. doi: 10.1093/cvr/cvq363
Schoots, M. H., Gordijn, S. J., Scherjon, S. A., Van Goor, H., and Hillebrands, J. L. (2018). Oxidative stress in placental pathology. Placenta 69, 153–161. doi: 10.1016/j.placenta.2018.03.003
Scott, H., Phillips, T. J., Stuart, G. C., Rogers, M. F., Steinkraus, B. R., Grant, S., et al. (2018). Preeclamptic placentae release factors that damage neurons: implications for foetal programming of disease. Neuronal Signaling 2. doi: 10.1042/NS20180139
Sedlmeier, E. M., Brunner, S., Much, D., Pagel, P., Ulbrich, S. E., Meyer, H. H., et al. (2014). Human placental transcriptome shows sexually dimorphic gene expression and responsiveness to maternal dietary n-3 long-chain polyunsaturated fatty acid intervention during pregnancy. BMC Genomics 15:941. doi: 10.1186/1471-2164-15-941
Sferruzzi-Perri, A. N., Sandovici, I., Constancia, M., and Fowden, A. L. (2017). Placental phenotype and the insulin-like growth factors: resource allocation to fetal growth. J. Physiol. 595, 5057–5093. doi: 10.1113/JP273330
Sibley, C. P., Coan, P. M., Ferguson-Smith, A. C., Dean, W., Hughes, J., Smith, P., et al. (2004). Placental-specific insulin-like growth factor 2 (Igf2) regulates the diffusional exchange characteristics of the mouse placenta. Proc. Natl. Acad. Sci. USA 101, 8204–8208. doi: 10.1073/pnas.0402508101
Smith, R. A., Porteous, C. M., Coulter, C. V., and Murphy, M. P. (1999). Selective targeting of an antioxidant to mitochondria. Eur. J. Biochem. 263, 709–716. doi: 10.1046/j.1432-1327.1999.00543.x
Stark, M. J., Hodyl, N. A., Wright, I. M., and Clifton, V. L. (2011). Influence of sex and glucocorticoid exposure on preterm placental pro-oxidant-antioxidant balance. Placenta 32, 865–870. doi: 10.1016/j.placenta.2011.08.010
Thompson, L. P., and Al-Hasan, Y. (2012). Impact of oxidative stress in fetal programming. J. Pregnancy 2012:582748. doi: 10.1155/2012/582748
Thompson, L. P., Chen, L., Polster, B. M., Pinkas, G., and Song, H. (2018). Prenatal hypoxia impairs cardiac mitochondrial and ventricular function in guinea pig offspring in a sex-related manner. Am. J. Physiol. Regul. Integr. Comp. Physiol. 315, R1232–R1241. doi: 10.1152/ajpregu.00224.2018
Tikvica, A., Kusan Jukic, M., Pintaric, I., Medic, M., Hudicek-Martincic, G., Kosec, V., et al. (2008). Nitric oxide synthesis in placenta is increased in intrauterine growth restriction and fetal hypoxia. Coll. Antropol. 32, 565–570.
Keywords: placenta, hypoxia, MitoQ, nanoparticles, sex difference
Citation: Ganguly E, Aljunaidy MM, Kirschenman R, Spaans F, Morton JS, Phillips TEJ, Case CP, Cooke C-LM and Davidge ST (2019) Sex-Specific Effects of Nanoparticle-Encapsulated MitoQ (nMitoQ) Delivery to the Placenta in a Rat Model of Fetal Hypoxia Front. Physiol. 10:562. doi: 10.3389/fphys.2019.00562
Edited by:
Dane Alan Crossley II, University of North Texas System, United StatesReviewed by:
Turk Rhen, University of North Dakota, United StatesJayanth Ramadoss, Texas A&M University, United States
Copyright © 2019 Ganguly, Aljunaidy, Kirschenman, Spaans, Morton, Phillips, Case, Cooke and Davidge. This is an open-access article distributed under the terms of the Creative Commons Attribution License (CC BY). The use, distribution or reproduction in other forums is permitted, provided the original author(s) and the copyright owner(s) are credited and that the original publication in this journal is cited, in accordance with accepted academic practice. No use, distribution or reproduction is permitted which does not comply with these terms.
*Correspondence: Sandra T. Davidge, c2RhdmlkZ2VAdWFsYmVydGEuY2E=