- 1Key Laboratory of Animal Genetics, Breeding and Reproduction of Shaanxi Province, College of Animal Science and Technology, Northwest A&F University, Yangling, China
- 2Laboratory of Reproductive Endocrinology, Graduate School of Biosphere Science, Hiroshima University, Hiroshima, Japan
- 3Livestock Research Institute, Oita Prefectural Agriculture, Forestry and Fisheries Research Center, Oita, Japan
- 4Women’s Clinic Oizumi-Gakuenn, Tokyo, Japan
- 5Department of Animal Breeding of Genetics, Bangabandhu Sheikh Mujibur Rahman Agricultural University, Gazipur, Bangladesh
Sperm motility patterns are continuously changed after ejaculation to fertilization in the female tract. Hyperactivated motility is induced with high glucose medium in vitro or the oviduct fluids in vivo, whereas sperm maintain linear motility in the seminal plasma or the uterine fluids containing low glucose. Therefore, it is estimated that sperm motility patterns are dependent on the energy sources, and the mitochondrial oxidative phosphorylation is activated to produce ATP in low glucose condition. To elucidate these hypotheses, boar sperm was incubated in different energy conditions with the transcription and translation inhibitors in vitro. Sperm motility parameters, mitochondrial activity, ATP level, gene expression and protein synthesis were analyzed. Sperm progressive motility and straight-line velocity were significantly increased with decreasing glucose level in the incubation medium. Moreover, the mitochondrial protein turnover meaning transcription and translation from mitochondrial genome in sperm is activated during incubation. Incubation of sperm with mitochondrial translation inhibitor (D-chloramphenicol) suppressed mitochondrial protein synthesis, mitochondrial activity and ATP level in sperm and consequently reduced the linear motility speed, but not the motility. Thus, it is revealed that the mitochondrial central dogma is active in sperm, and the high-speed linear motility is induced in low glucose condition via activating the mitochondrial activity for ATP generation.
Introduction
Mammalian spermatozoa reside in the female genital tracts for several hours from ejaculation to fertilization process. Linear motility, as defined by low lateral amplitude and high straight-line velocity, is essential for sperm migration from the cervix to the uterus and then to oviduct (Shalgi et al., 1992; Suarez and Pacey, 2006). Just after ovulation, capacitated sperm leave the oviduct epithelial cells and move to the oocyte (Demott and Suarez, 1992; De Lamirande et al., 1997). The capacitated sperm show hyperactivated status (Suarez, 2008), defined as high curvilinear velocity as well as high lateral amplitude, and penetrate the oocyte for successful fertilization (Stauss et al., 1995). Sperm motility patterns are dependent on flagellar motion; the symmetry flagellar motion induces the linear motility pattern, whereas the asymmetry one is associated with hyperactivation (Phillips, 1972; Suarez et al., 1991; Ho et al., 2002; Curtis et al., 2012). The flagellum of mammalian sperm consists of nine fused pairs of microtubule doublets surrounding two central single microtubules (Fawcett, 1970; Woolley and Fawcett, 1973). Moreover, the sliding filament theory is adapted to sperm flagella, namely the sperm motility is induced by ATP production (Huxley and Niedergerke, 1954; Summers and Gibbons, 1971; Brokaw, 1972).
Glycolysis and mitochondrial oxidative phosphorylation (OXPHOS) are two main metabolic pathways for ATP production in mammalian cells. The metabolic pathways of cells are changed depending on the oxygen availability and the composition of metabolic substrates in their environment (Gohil et al., 2010; Potter et al., 2016). When HepG2 cells were cultured in the medium containing a high level of glucose, glycolysis pathway was highly activated; however, the reduction of glucose level in the medium switched off the glycolytic pathway to turn on OXPHOS pathway to generate ATP in the cells (Marroquin et al., 2007). Amino acids and pyruvate are the substrates of TCA cycle in mitochondria present at high concentrations in both seminal plasma and uterus fluids (Marden, 1961; Brown-Woodman and White, 1974; Harris et al., 2005). On the other hand, the high level of glucose is detected in the oviductal fluids, especially after ovulation (Nichol et al., 1992; Vecchio et al., 2007; Umehara et al., 2018). Therefore, for the successful fertilization in vivo, the sperm probably use the different metabolic pathways to produce ATP in each condition as similar to those in somatic cells (Ruiz-Pesini et al., 2007; Storey, 2008). It is generally accepted that the glycolysis pathway is essential for sperm hyperactivation (Du Plessis et al., 2015). However, there is limited information about the roles of mitochondria in producing ATP regarding sperm motility patterns.
Mitochondria, double membrane sub-cellular organelles have their own maternally inherited genome mitochondrial DNA (mtDNA) which encodes 13 polypeptides, 22 tRNAs, and 2 rRNAs. The polypeptides are essential subunits for mitochondrial electron transport chain (ETC) complexes wheras the tRNAs and rRNAs are essential for translation of the polypeptides (Anderson et al., 1981). The mitochondrial mRNA transcription (such as NADPH dehydrogenase subunits 1–6 (mt-Nd1 – mt-Nd6), cytochrome c oxidase subunits 1–3 (CoxI – CoxIII), etc. and their translation processes are activated with increasing the mitochondrial activity and ATP level in serum-stimulated HeLa cells (Xiong et al., 2012). Therefore, we hypothesized that mitochondrial OXPHOS is activated in sperm for ATP supply, and their transcription and translation process become functioning to the sperm motility. Hence, the present study was performed to (1) determine whether sperm motility patterns and metabolic pathways in different glucose levels are changed or not, (2) understand the induction of gene expression and protein synthesis in sperm mitochondria, and (3) elucidate the roles of the mitochondrial transcription and translation in sperm motility.
Materials and Methods
Materials
Routine chemicals and reagents were obtained from Nakarai Chemical Co. (Osaka, Japan) or Sigma Chemical Co. (St. Louis, MO, United States).
Animals and Semen Collection
Five healthy, fertile, and mature boars (between the ages of 2 and 4 years) were used in this study. The boars were housed individually, maintained under natural daylight, fed basal diets and been free access to water. The sperm-rich fraction was collected weekly from each boar using the gloved-hand technique. The sperm-rich fraction was filtered through double gauze.
Ethics Statement
All animals and experimental procedures were treated in accordance with the National Institutes of Health Guide for the Care and Use of Laboratory Animals, approved by the Animal Care and Use Committee at Hiroshima University (approval number: E18-1).
Sperm Incubation
Fresh sperm was diluted with Modena solution composed of 153 mM D-glucose, 26.7 mM trisodium citrate, 11.9 mM sodium hydrogen carbonate, 15.1 mM citric acid, 6.3 mM EDTA-2Na, 46.6 mM Tris, 1000 IU/mL penicillin G potassium and 1 mg/mL amikamycin (Okazaki et al., 2012). The concentration of glucose in Modena solution (153 mM) was defined as 100%. Lactose was used partially with glucose to make the different dose of glucose extender (153, 122.4, 91.8, 61.2, 30.6, and 0 mM; namely 100%, 80%, 60%, 40%, 20%, and 0%) because sperm did not use lactose as an energy substrate. Sperm were incubated for 1 h at 37°C in each media containing different levels of glucose. Some of the sperm were incubated with various concentrations of rotenone (0, 10, 100 nM, an inhibitor of complex I) for 3 h at 37°C in 30.6 mM glucose media (20% glucose). Other sperm were incubated with various concentrations of D-chloramphenicol (a mitochondrial translation inhibitor, CRP: 0, 200, 400, 600, 800 ng/mL), cycloheximide (mRNA translation inhibitor, CHX: 0, 50, 100 ng/mL) and α-amanitin (nuclear transcription inhibitor, AMNT: 0, 10, 50, 100 ng/mL) for 3 h at 37°C in 30.6 mM glucose media (20% glucose). Furthermore, sperm were also incubated in 30.6 mM glucose media (20% glucose) for up to 6 h at 37°C to evaluate whether the transcription and translation in sperm mitochondria is working or not.
Evaluation of Sperm Motility by Computer-Assisted Sperm Analysis (CASA) System
After incubation of sperm in different treatments, a total of 10 μL of sample was placed in a pre-warmed counting chamber. According to our previous study (Umehara et al., 2018), sperm tracks (0.5 s, 45 frames) were captured at 60 Hz using a CASA system (HT CASA-Ceros II; Hamilton Thome, MA, United States). More than 200 individual trajectories were recorded. Non-progressive motility (%) = total motility (%) - progressive motility (%).
Membrane Integrity
Membrane integrity was evaluated using a LIVE/DEAD Sperm Viability Kit (L7011; Thermo Fisher Scientific), as described previously (Zhu et al., 2017). Briefly, samples were stained with SYBR-14/PI. The staining was analyzed in a flow cytometer (FAC-S Calibur, BD Biosciences) with Excitation/Emission = 485/535 nm for SYBR-14 fluorescence, Excitation/Emission = 525/590 nm for PI fluorescence. A total of 50,000 sperm-specific events were analyzed. Data were processed by using the CellQuest program (BD Biosciences).
Mitochondrial Activity
Sperm mitochondrial activity was measured with MitoPT® JC-1 Assay Kit (911, ImmunoChemistry Technologies, llc.) according to Treulen et al. (2016). Briefly, sperm samples were incubated with 500 μL 1x working solution at 37°C for 30 min in dark, the mitochondrial activity was analyzed by flow cytometry using a filter with a bandwidth of 574/26 nm (Attune®NxT Acoustic Focusing Cytometer, Invitrogen) and measured as the mean fluorescence intensity (MFI) of JC-1 orange aggregates. A total of 50,000 sperm events were analyzed.
Measurement of Sperm ATP Level
EnzylightTM ATP Assay Kit (EATP-100, Bioassay System, Hayward, CA, United States) was used to detect sperm ATP level according to the manufacturer’s instruction. Briefly, sperm samples were mixed with assay buffer and substrates, and then the luminescence was measured with a luminometer (2030 Multilabel Reader ARVO X4; PerkinElmer Inc., Waltham, MA, United States). The ATP production was calculated over sperm concentration and expressed as nmol/107 of sperm.
RNA Extraction and Reverse Transcription-Polymerase Chain Reaction (RT-PCR)
Total RNA was obtained from fresh sperm and the granulosa cells (positive control) using the RNAeasy mini kit (QIAGEN Sciences, Germantown, MD, United States), according to the manufacturer’s instructions. Reverse transcription (RT) was performed using 500 ng poly-deoxythymidine and 0.25 U avian myeloblastosis virus reverse transcriptase (Promega, Madison, WI, United States) at 42°C for 75 min and 95°C for 5 min. RT-PCR analyses were performed with KOD FX Neo (TOYOBO Life Science, Osaka, Japan) according to the manufacturer’s instructions. Specific primers pairs used in the RT-PCRs are shown in Table 1; PCR products were resolved on 2% (wt/vol) agarose gels.
Quantitative PCR Analyses
Quantitative real-time PCR analyses were performed as previously (Shimada et al., 2008). Briefly, cDNA and primers shown in Table 1 were added to 15 μL total reaction volume of the Power SYBR Green PCR master mix (Applied Biosystems). PCRs were then performed using the StepOne real-time PCR system (Applied Biosystems).
Immunofluorescence
Sperm cells were washed with PBS and fixed with 4% paraformaldehyde for 5 min. The sperm were spread onto glass slides, then permeabilized by 0.5% Triton X-100 in PBS. Non-specific binding was blocked with PBS contained 10% bovine serum albumin (w/v) (Life Technologies, Grand Island, NY, United States) for 30 min at room temperature. Sperm were proved with a 1:100-diluted NADPH dehydrogenase subunits 1 (MT-ND1) antibody (1973-1-AP, Proteintech, United States), NADPH dehydrogenase subunits 6 (MT-ND6) antibody (bs-3955R; Bioss, Inc., Boston, MA, United States), and NADH-ubiquinone oxidoreductase subunit A7 (NDUFA7) antibody (ab140871; Abcam, United States). The antigens were visualized with biotinylated goat anti-rabbit Cy3-IgG or donkey anti-goat FITC-IgG (1:200). Subsequently, sperm were incubated with 4′,6′-diamidino-2-phenylindole (DAPI; 5 μg/mL; Sigma-Aldrich). Digital images were captured using a BZ-9000 microscope (Keyence Co., Osaka, Japan). Negative control was prepared at the same time without the primary antibody.
Western Blotting
Western blotting analyses were performed according to our previous study (Umehara et al., 2018). Total protein was extracted from sperm in sodium dodecyl sulfate (SDS) sample buffer. The protein was separated by 12.5% SDS-PAGE and transferred to PVDF blotting membrane (GE Bioscience, Newark, NJ, United States). Non-specific binding sites were blocked by incubation in Tris-buffered saline (TBS) containing 0.1% (v/v) Tween-20 and 5% (w/v) bovine serum albumin (Life Technologies, Grand Island, NY, United States). The membranes were immunoblotted with primary antibodies [anti-MT-ND1, anti-MT-ND6, anti-NDUFA7 and anti-α-tubulin (2148; Cell Signaling Technology, Inc.)] diluted in 5% bovine serum albumin in TBS-Tween (1:1000 dilution) overnight at 4°C. Followed by incubation with HRP conjugated secondary [goat anti-rabbit antibody (7074S, Cell Signaling Technology, Inc.) for MT-ND1, MT-ND6, and α-tubulin, donkey anti-goat (ab97110, Abcam, United States) for NDUFA7, 1:5000 dilution]. After washing in TBST, enhanced chemiluminescence (ECL) detection was performed by using the ECLTM Prime Western Blotting Detection Reagents (RPN2235, GE Bioscience) according to the manufacturer’s specifications, and appropriate exposure of blots to Fuji X-ray film (Fujifilm, Tokyo, Japan). Band intensities were analyzed using a Gel-Pro Analyzer (Media Cybernetics, Rockville, MD, United States).
Statistical Analysis
All data were tested for normality and variance homogeneity prior to statistical analysis. Data were transformed by arc-sin square root transformation when it is necessary. Data from three replicates for comparison were performed by either Student’s t-test or one-way analysis of variance followed by Tukey’s post hoc test (Statview; Abacus Concepts, Inc., Berkeley, CA, United States). All the values are presented as the mean ± standard error (SE). Treatments were considered statistically different from one another at p < 0.05.
Results
Low Glucose Condition Increases the Mitochondrial Activity and Motility Patterns of Sperm at 1 h Incubation
The sperm motility tracks generated by CASA revealed that the incubation in high glucose media made circle-like tracks, while low glucose condition made it linear-like tracks (Figure 1A). The sperm total motility wasn’t significantly changed in all glucose concentration [from 30.6 mM (20%) to 153 mM (100%)] in the media (Figure 1B). However, the reduction of glucose level from 153 mM to 30.6 mM significantly increased the sperm progressive motility and straight-line velocity, decreased the non-progressive motility in a dose-dependent manner (Figures 1A,C–E). The high linier motility was observed until 3 h; however, the total motility, progressive motility, and straight-line velocity were significantly decreased at 6 h of incubation (Supplementary Figure 2). Meanwhile, no significant difference was observed in lateral amplitude (Figure 1F), an index for evaluating hyperactivation among the treatment groups. The mitochondrial activity was also significantly increased by the reduction of glucose level in the incubation medium (Figure 1G and Supplementary Figure 1). However, the ATP level showed no significant difference among the treatment groups (Figure 1H).
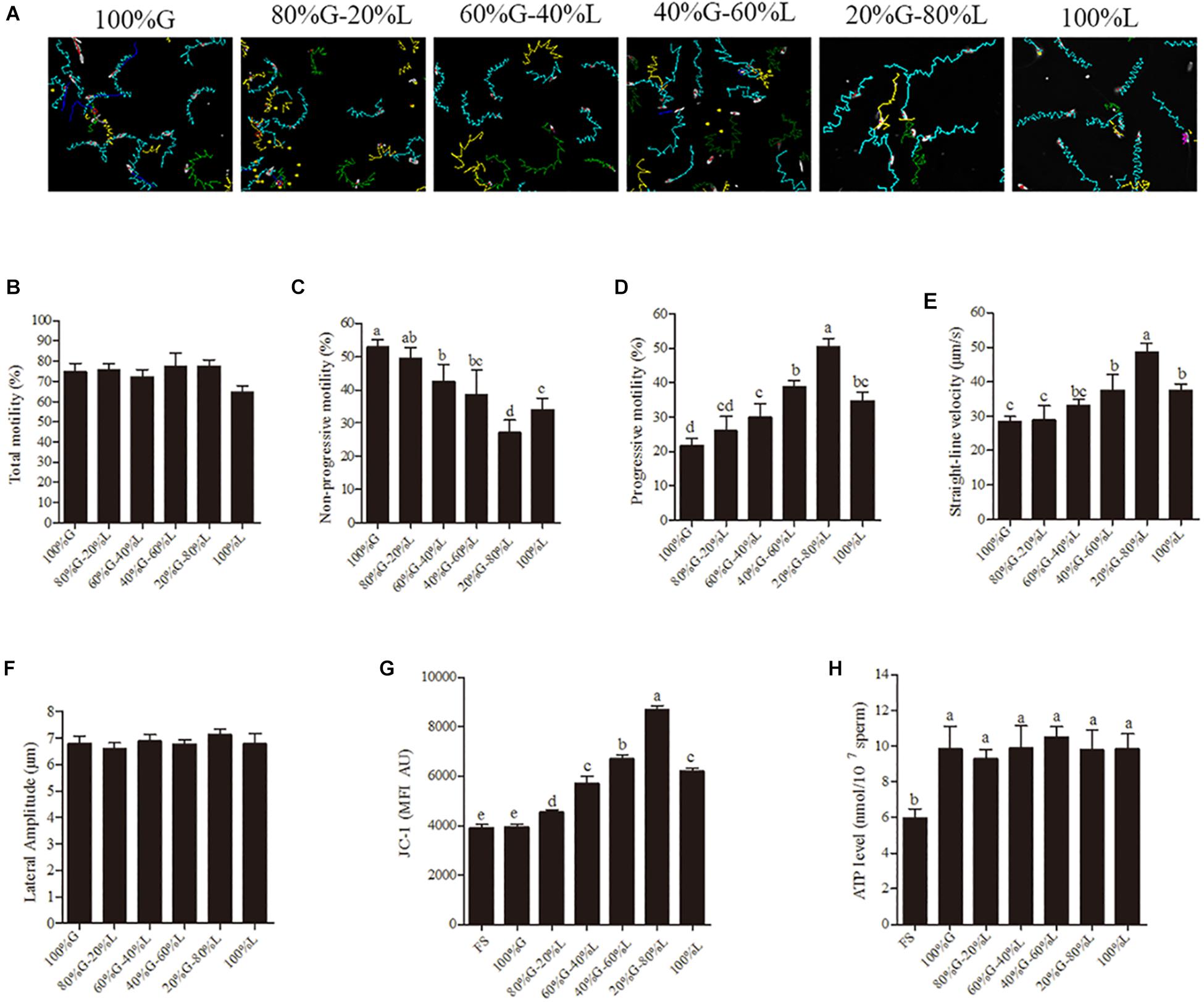
Figure 1. Low glucose condition improves the sperm motility patterns through enhancing the mitochondrial activity at 1 h of incubation. (A) CASA derived changes in the sperm motility track from circular to linear by reducing the glucose level from 153 mM to 0 mM (namely 100–0%). (B–F) Dynamic changes in the sperm parameters: (B) total motility, (C) non-progressive motility (D) progressive motility, (E) straight-line velocity and (F) lateral amplitude. (G) Kinetic changes in the mitochondrial activity. (H) ATP level in the sperm. Values are specified as mean ± standard error of mean (SEM) of three replicates. Columns with different lowercase letters differ significantly (p < 0.05). The letters in X-axis are symbolized as – G, glucose; L, lactose; FS, fresh sperm.
Addition of Rotenone to Incubation Media Reduces the Mitochondria Activity, ATP Level and Kinetic Patterns of Sperm
To understand the relationship between sperm motility pattern and mitochondrial ATP production, rotenone (an inhibitor of complex I) was added to the low glucose medium [30.6 mM (20%) glucose]. The sperm motility tracks and the CASA analytic data revealed that the progressive motility and straight-line velocity were significantly decreased with the treatment with rotenone at 1- and 3-h points in a dose-dependent manner, whereas the non-progressive motility was increased (Figures 2A–D). However, the addition of rotenone did not alter sperm total motility during incubation (Figure 2E). Apart from the motility data, the sperm membrane integrity used for survival evaluation showed no significant difference among the treatment groups (Figure 2F). The mitochondrial activity and ATP levels were significantly reduced by the addition of rotenone in a dose-dependent manner (Figures 2G,H) at 1-h point.
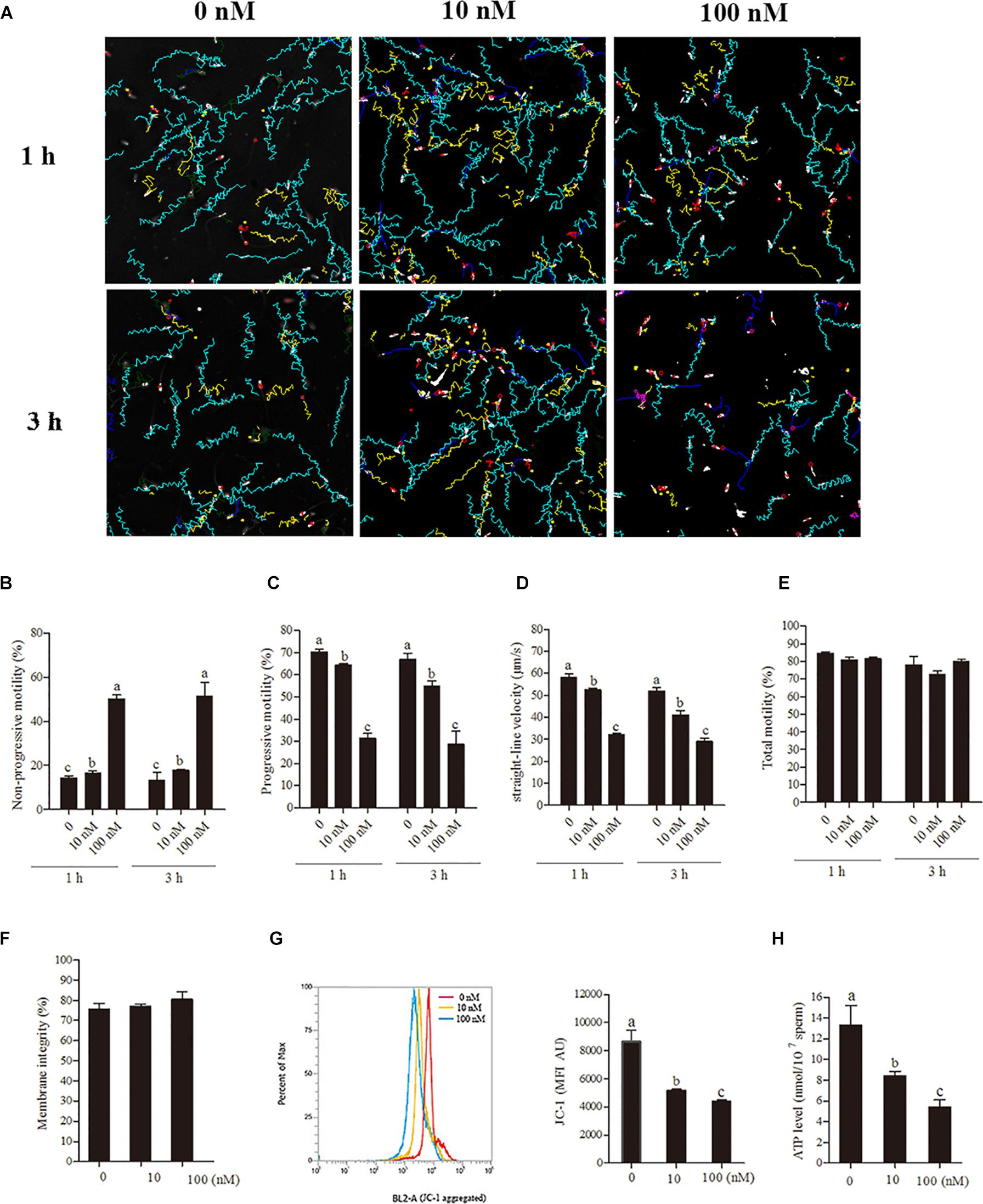
Figure 2. Sperm incubated with rotenone in 30.6 mM glucose media alters the mitochondrial activity, ATP level and kinetic patterns. (A–E) Kinetic changes in the sperm motility patterns at 1 and 3 h of incubation, (A) motility tracks generated from CASA, (B) non-progressive motility, (C) progressive motility, (D) straight-line velocity, (E) total motility. (F–H) Rotenone-induced changes in mitochondrial attributes at 1 h of incubation, (F) membrane integrity, (G) mitochondrial activity, and (H) ATP levels. Values are specified as mean ± SEM of three replicates. Columns with different lowercase letters differ significantly (p < 0.05).
Transcription and Translation in Sperm Mitochondria Is Working During Incubation
To elucidate whether the transcription and translation in sperm mitochondria is working or not, we checked the expression of 13 genes derived from mtDNA and 3 genes derived from nuclear DNA in sperm. Granulosa cells were used as the positive control. We detected all of the 13 mitochondrial-encoded mRNAs in both sperm and granulosa cells. However, the nucleus-encoded mRNAs of NDUFA7, NDUFB10, and NDUFS4 were not detected in sperm by 40-cycles PCR, although strongly expressed in granulosa cell (Figure 3A). Moreover, the expression of genes mt-Nd1 and mt-Nd6 were significantly increased at 3-h and 6-h points, but no change at 1-h point (Figures 3B,C). Similarly, western blot positive bands of MT-ND1 and MT-ND6 showed that the intensities of mitochondrial proteins were significantly increased at 3 h and 6 h but not changed at 1-h point (Figures 3D–F, p < 0.05). Meanwhile, the signal of the nuclear encoded NDUFA7 protein was not changed throughout the 6-h incubation period (Figures 3D,G). We also observed that the immunolocalizations of MT-ND1, MT-ND6, and NDUFA7 were in the sperm midpiece which also the location of mitochondria (Figure 3H).
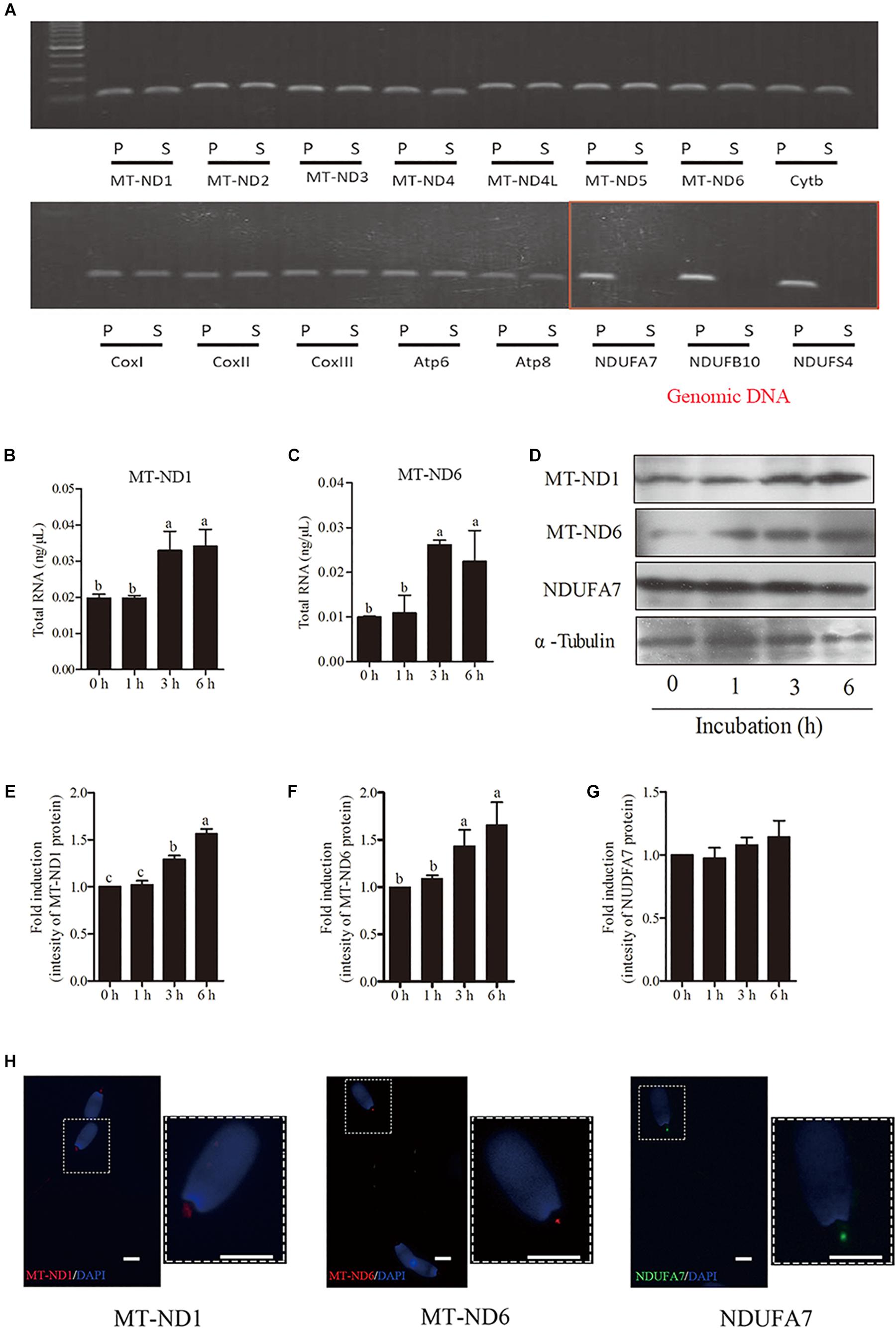
Figure 3. The transcription and translation in sperm mitochondria. (A) RT-PCR derived expression of mitochondrial and nuclear genes, where – S, boar sperm; P, positive control (porcine ovarian granulosa cell). (B,C) Time-dependent changes in the expression of mt-Nd1 (B) and mt-Nd6 (C) genes during 6 h incubation. (D) Western blotting image showing the expression of the mitochondria-encoded protein (MT-ND1 and MT-ND6), nuclear-encoded protein (NDUFA7), and α-tubulin during 6 h incubation. (E–G) Quantitative expression of the MT-ND1, MT-ND6, and NDUFA7 over α-tubulin (control) generated from western blotting. (H) Immunolocalizations of MT-ND1, MT-ND6, and NDUFA7 in boar sperm, scale bar indicates 5 μm. Values are specified as mean ± SEM of three replicates. Columns with different lowercase letters differ significantly (p < 0.05).
Mitochondrial Translational Inhibitor (CRP) Reduces the Mitochondrial Activity, ATP Level, Protein Synthesis, as Well as Motility Patterns of Sperm
Sperm were incubated with various doses (0, 200, 400, 600, 800 ng/mL) of CRP, a specific mitochondrial translational inhibitor for 3 h. At 3 h point, the progressive motility, straight-line velocity, mitochondrial activity and ATP levels were significantly decreased at 400, 600, and 800 ng/mL doses (Figures 4A,C–F) while the non-progressive motility was increased (Figure 4B). Moreover, the intensity of MT-ND1 and MT-ND6 proteins are observed to be significantly decreased only at higher (600 and 800 ng/mL) doses (Figures 4G–J). The sperm membrane integrity showed no significant difference (Figure 4K).
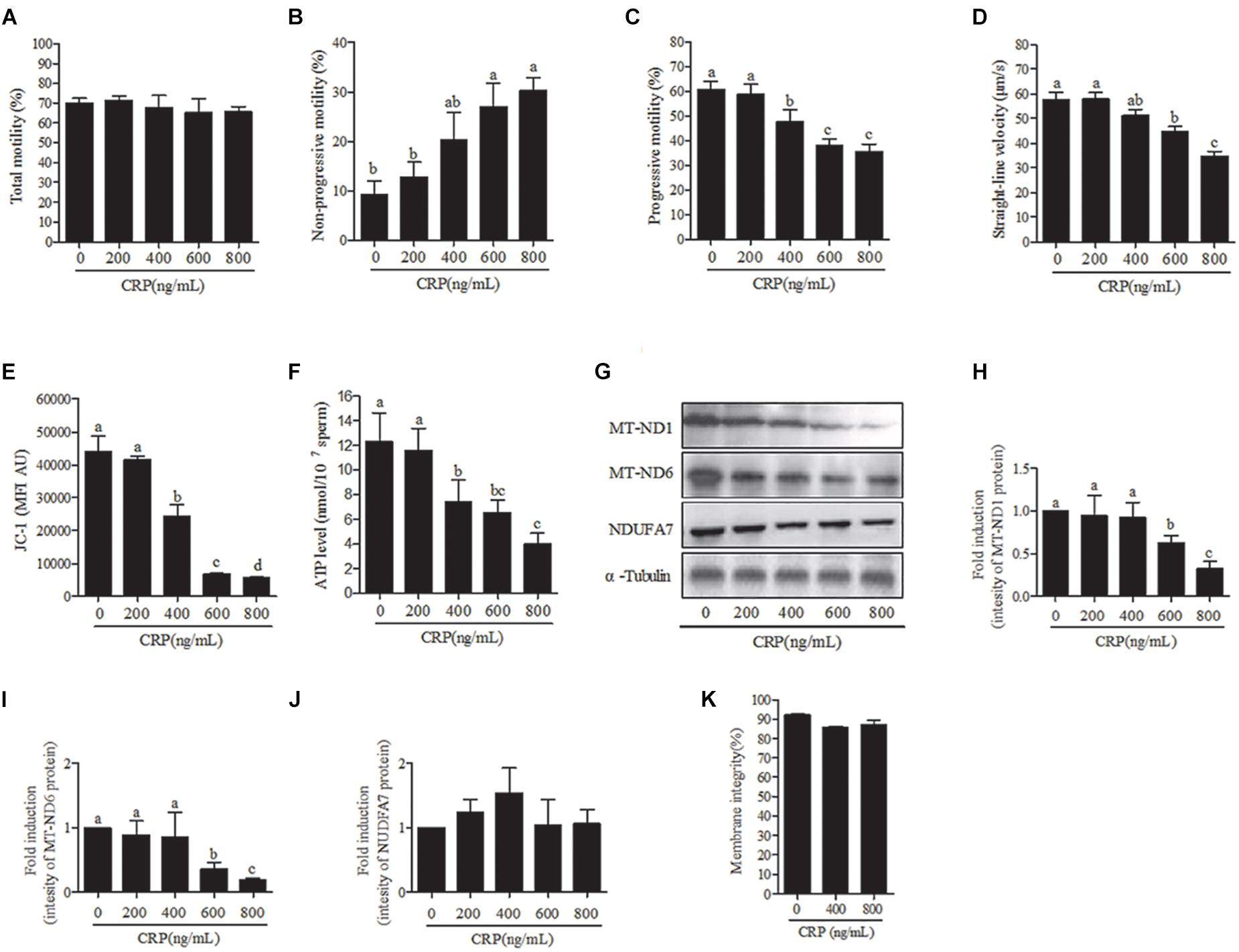
Figure 4. Effect of D-chloramphenicol (CRP) on boar sperm at 3 h incubation with different doses (0, 200, 400, 600, 800 ng/mL). (A–F) Dose-dependent changes in the sperm (A) total motility, (B) non-progressive motility, (C) progressive motility, (D) straight-line velocity, (E) mitochondrial activity, and (F) ATP level. (G) Western blotting image showing the expression of the MT-ND1, MT-ND6, NDUFA7, and α-tubulin. (H–J) Quantitative expression of the MT-ND1, MT-ND6, and NDUFA7 over α-tubulin (control) generated from western blotting. (K) Membrane integrity measured in sperm. Values are means ± SEM of three replicates. Columns with different lowercase letters differ significantly (p < 0.05).
In terms of time-dependent experiment, a significant (p < 0.05) decrease was observed with 600 ng/mL CRP in the sperm progressive motility, straight-line velocity (Figures 5A,D,E), mitochondrial activity and ATP levels (Figures 5F,G) at 3 h of incubation point but not at 1-h point. Moreover, addition of 600 ng/mL CRP significantly increased non-progressive motility at 3-h point incubation (Figure 5C). However, the total motility was not changed with addition of 600 ng/mL CRP both at 1-h and 3-h points (Figure 5B). Furthermore, when sperm were incubated with 600 ng/mL CRP, the intensity of MT-ND1 and MT-ND6 proteins was significantly decreased at 3-h point but not changed at 1-h point (Figures 5H–J, p < 0.05), whereas the NDUFA7 protein signal was not changed during incubation (Figures 5H,K).
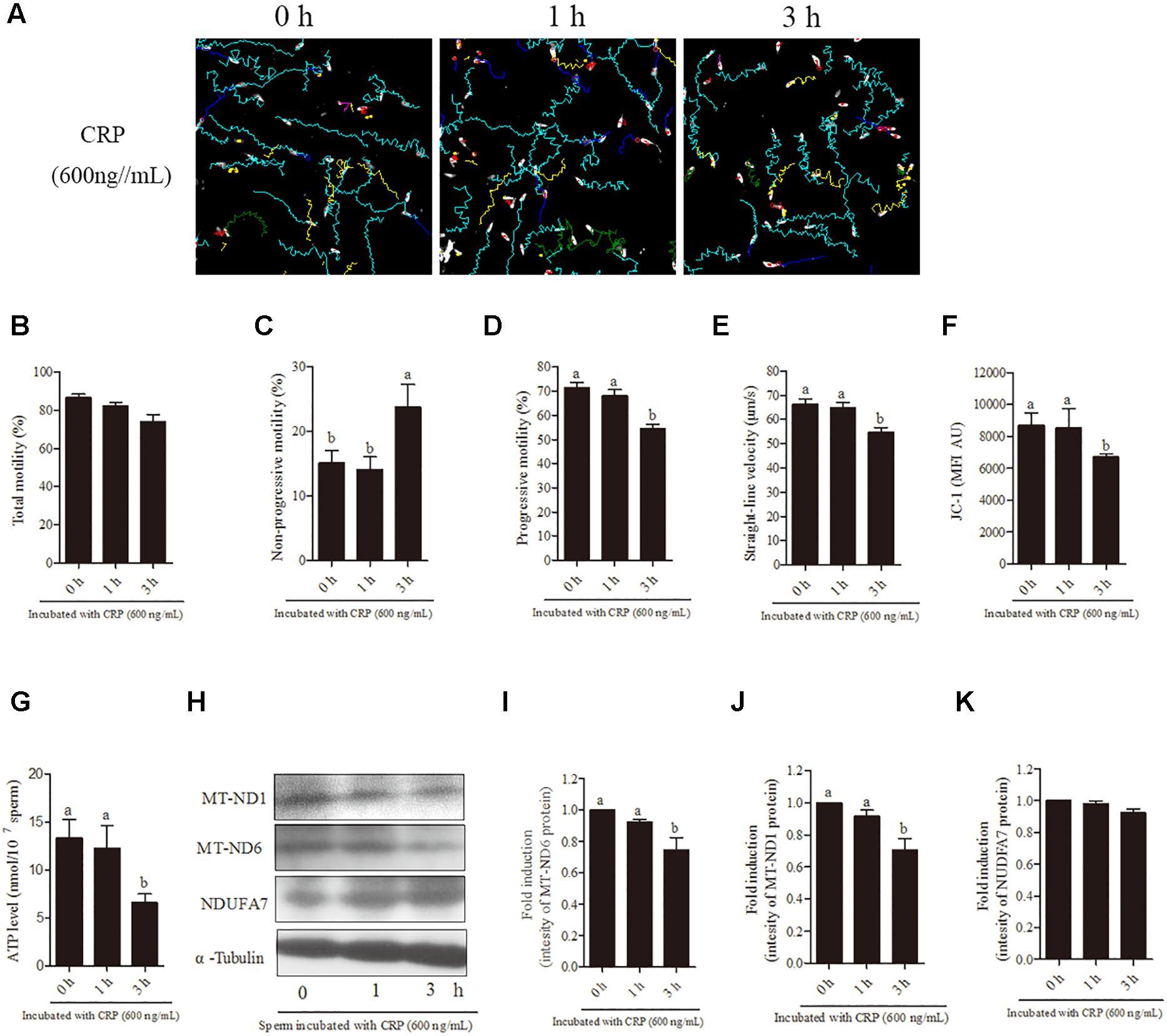
Figure 5. Effect of 600 ng/mL CRP on boar sperm during 3 h incubation in 30.6 mM glucose media. (A) Changes in the motility tracks of sperm generated by CASA system. (B–G) Time-dependent changes in the sperm (B) total motility, (C) non-progressive motility (D) progressive motility, (E) straight-line velocity, (F) mitochondrial activity, and (G) ATP level. (H) Western blotting image showing the expression of the MT-ND1, MT-ND6, NDUFA7, and α-tubulin. (I–K) Quantitative expression of the MT-ND1, MT-ND6, and NDUFA7 over α-tubulin (control) generated from western blotting. Values are means ± SEM of three replicates. Columns with different lowercase letters differ significantly (p < 0.05).
Cytoplasmic Translation Inhibitor (CHX) and Nuclear Transcription Inhibitor (AMNT) Do Not Affect the Sperm Quality
For further confirmation of our hypothesis that sperm linear motility patterns are solely dependent on transcription and translation in mitochondria but not in nucleus and cytoplasm, we incubated sperm with CHX and AMNT for 3 h. However, sperm incubated with different doses (0, 50, 100 ng/mL) of CHX revealed that the sperm motility tracks were unchanged (Figure 6A). Moreover, no significant difference was observed among the treatment doses in motility patterns [total motility, non-progressive motility, progressive motility, and straight-line velocity (Figures 6B–E)], mitochondrial activity (Figure 6F) and the intensity of the proteins (MT-ND1, MT-ND6, and NDUFA7) (Figures 6G–J). Similarly, sperm incubated with AMNT showed a non-significant difference in the total motility, straight-line velocity and mitochondrial activity (Figures 6K–M).
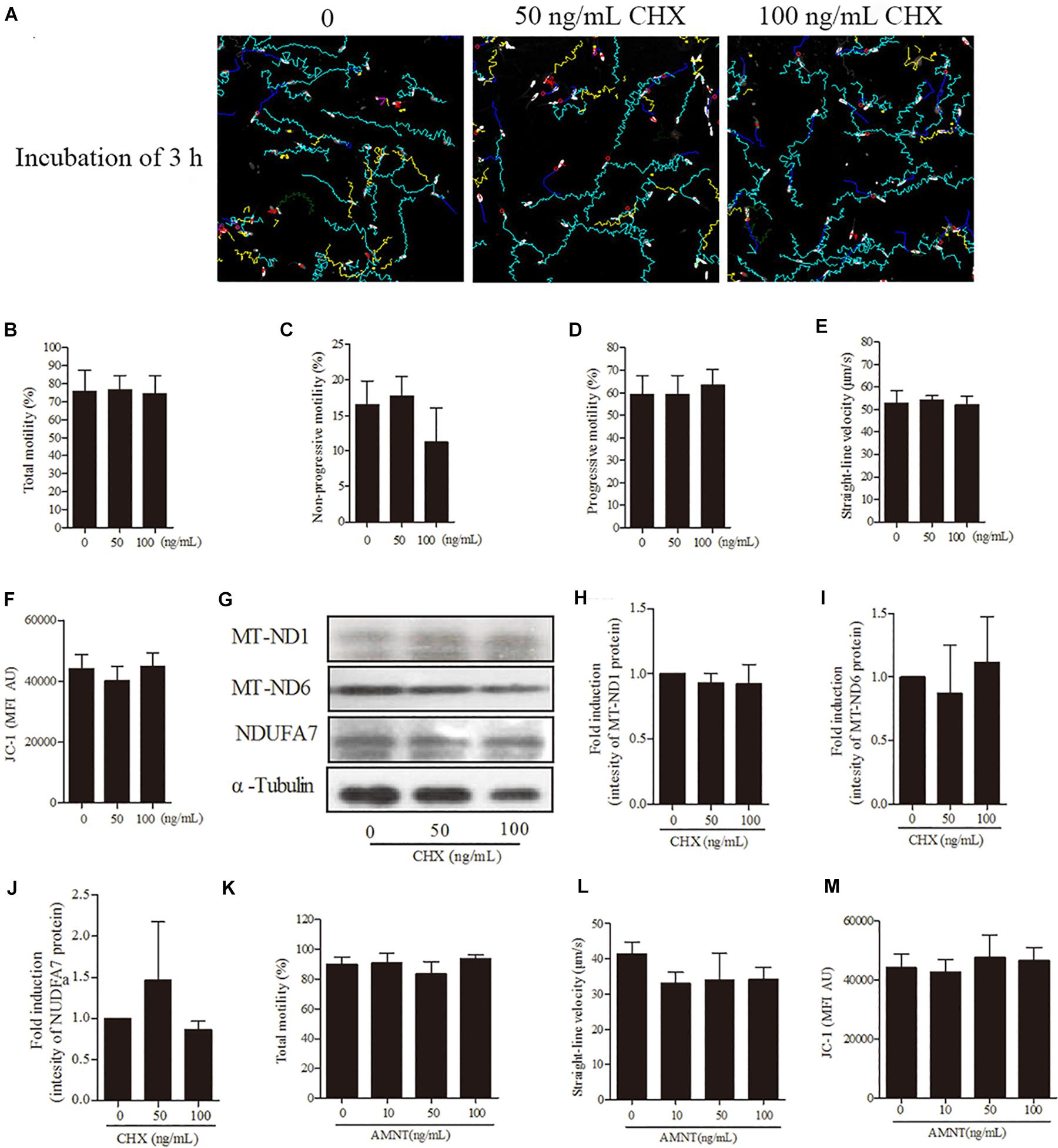
Figure 6. Effects of cycloheximide (CHX) and α-amanitin (AMNT) on boar sperm at 3 h of incubation 30.6 mM glucose media. (A) Tracks of sperm motility incubated with different concentrations of CHX (0, 50, and 100 ng/mL) generated by CASA system. (B–E) Motility patterns: (B) total motility, (C) non-progressive motility, (D) progressive motility, (E) straight-line velocity. (F) Mitochondrial activity. (G) Western blotting image showing the expression of the MT-ND1, MT-ND6, NDUFA7, and α-tubulin. (H–J) Quantitative expression of the MT-ND1, MT-ND6, and NDUFA7 over α-tubulin (control) generated from western blotting. (K) Total motility, (L) straight-line velocity, and (M) mitochondrial activity of sperm were not changed after 3 h of incubation with different concentrations of AMNT (0, 10, 50, and 100 ng/mL). Values are means ± SE of three replicates. Columns with different lowercase letters differ significantly (p < 0.05).
Discussion
In vivo fertilization, sperm are ejaculated to the female reproductive tract with seminal plasma and swim toward oviduct for the fertilization (Suarez and Pacey, 2006). During the journey of sperm in female reproductive tracts, the motility pattern of sperm is altered from linear motility in the uterus to zigzag motility in the oviduct (De Lamirande et al., 1997). It is well known that the sperm motility is regulated by ATP produced either from glycolytic pathway in cytoplasm or OXPHOS in mitochondria or both (Storey, 2008; Mukai and Travis, 2012; Du Plessis et al., 2015). Especially, Odet et al. (2008) demonstrated that the glycolysis was important for hyperactivated motility using Ldh-c knock out mice model. On the other hand, in vitro incubation in low-glucose or glucose-free BWW medium with rotenone, an inhibitor of complex I, decreased the sperm progressive motility and straight-line velocity in stallion (Plaza Davila et al., 2015) and human (Barbonetti et al., 2010), suggesting that mitochondrial ATP production is also important for sperm motility. However, the different roles of metabolomics pathways in sperm motility remain unclear. In this study, using the medium containing different doses of glucose we revealed that mitochondrial activity was increased under low glucose condition, and the ATP produced in mitochondria was associated with a high-speed linear motility in boar sperm.
Mitochondria have several functions, such as cell calcium homoeostasis (Contreras et al., 2010), lipid homoeostasis (Labbe et al., 2014), release of cytochrome c (Wang, 2001) and the ATP synthesis via OXPHOS (Bratic and Trifunovic, 2010), etc. Especially, the production of ATP in mitochondria is essential for cell survival and cell homeostasis (Osellame et al., 2012). However, during the process of ATP production in mitochondria, reactive oxygen species (ROS) are produced as the by-products, and then the excessive accumulation of ROS reduces the production of mitochondrial ATP via enzyme degradation. The mitochondrial (TC) complexes, including NADH dehydrogenase (complex I), succinate dehydrogenase/fumarate reductase (complex II), cytochrome c reductase (complex III), cytochrome c oxidase (complex IV) and ATP synthase (complex V) are required for ATP production in mitochondria (Lenaz and Genova, 2010). In addition, the 13 mtDNA-encoded polypeptides are partly subunits of complex I, III, IV, and V (Chomyn et al., 1985; Amaral et al., 2013). Thus, to keep ATP level in sperm, the gene expression and protein synthesis in mitochondria would be required.
To elucidate the function of mitochondrial transcription and translation in sperm, the expression and protein synthesis in mitochondria were examined in our study. The genes encoding mitochondria were highly expressed in sperm but the genes encoding nuclear genome were not detected during incubation. The protein turnover system in mitochondria was essential for sperm motility when the sperm were incubated in low glucose media. Gur and Breitbart (2006) showed that the addition of D-chloramphenicol, a mitochondrial translation inhibitor, in modified Tyrode’s glucose-free medium suppressed protein synthesis in bull sperm. Moreover, transcripts of all the mitochondrial genes were detected in mouse epididymal sperm (Alcivar et al., 1989). Interestingly, the cycloheximide (CHX), an inhibitor to cytoplasmic translation did not suppress the intensity of proteins encoded by not only mtDNA, but also by nuclear genome in this study, indicating that the nuclear genome encoded protein is more stable and resistant to oxidative stress during incubation. In addition, when HeLa cells were cultured with a ROS generator, the levels of mitochondrial encoded proteins (MT-ND6, MT-ND1, and MT-CYB) were significantly decreased, but the HSP9A levels, a nuclear-encoded and mitochondria-localized protein showed no significant change (Xie et al., 2012). About 92% of proteins in mammals have at least one cysteine residue containing exposed thiol which is free to interact with the aqueous solvent (Miseta and Csutora, 2000). Requejo et al. (2010) reported that mammalian mitochondria had a large number of redox-active exposed thiols on the surface of native proteins, especially the 75-kDa subunit (NDUFS1) complex I that encoded by nucleus (Hurd et al., 2008). Moreover, the cysteine residue of mitochondrial encoded protein is comparatively lower than those of nuclear-encoded protein in the mitochondrial ETC complexes (cysteine residue per protein:1.69 vs. 3.56; cysteine residue% of protein sequence: 0.773 vs. 1.667%; the data were counted from the protein sequence in GeneCards Database1), signifying that the resistant performance for scavenging ROS of mitochondrial-encoded protein is lower than that in nuclear-encoded protein. Additionally, post-translational modifications including the formation of sulfenic acids (Charles et al., 2007) and S-nitrosothiols (Hogg, 2002) or glutathionylation (Cotgreave and Gerdes, 1998) to the protein thiols are important to maintain cellular redox signaling and protein stability. Usually, most of the nuclear-encoded proteins in mitochondria are potentially post-translationally modified in the cytoplasm prior to translocate to the mitochondria (Hofer and Wenz, 2014), suggesting that the mitochondrial-encoded proteins were more sensitive to ROS and unstable than nuclear-encoded proteins in sperm. Therefore, to keep the ATP production and the linear motility pattern, the transcription and translation in mitochondria are activated during incubation and may also be in the uterus for the successful in vivo fertilization.
In this study, the mitochondria activity and high-speed linear motility similar to uterus were up-regulated with the reduction of glucose concentration. Previous study showed that the concentration of glucose in the mice uterus is lower than those in oviduct during fertilization process (Harris et al., 2005). Additionally, sperm linear motility is induced in the semen plasma and uterus (De Lamirande et al., 1997), suggesting that sperm mitochondria would be activated in uterus when it was presented in the low glucose uterus fluid. However, when sperm was injected to uterus in artificial insemination technique, the sperm was diluted with human tubal fluid (HTF) medium that is commonly used for in vitro fertilization in human and with Modena solution that contains a high dose of glucose in pig. The artificial insemination technique is commercially applied worldwide to breed pigs; however, the technique is still not efficient, as the large sperm numbers per sow in estrus is required for getting high reproductive performance as similar to that by natural mating (5–7 × 109 sperm for per sow fertilization) (Knox, 2016). The large sperm numbers are a critical limitation for artificial insemination application not only in pigs but also in human infertility treatment. Because in human artificial insemination, 1 × 107 or more motile sperm are required (Van Voorhis et al., 2001), in vitro fertilization or intracytoplasmic sperm injection (ICSI) are usually selected in the case of oligospermia. Our novel insight in which high-speed linear motility is induced by low glucose medium might provide a new strategy for improving the artificial insemination technique of both livestock animals and human infertility care.
Conclusion
In conclusion, the transcription and translation in sperm mitochondria is active and the low glucose condition improves sperm progressive motility, straight-line velocity and mitochondrial activity during incubation in vitro. Reduction of the glucose level in diluted medium enhances the duration of high-speed linear motility via activating the mitochondrial activity for ATP generation in the present study. Thus, high-speed linear motility induced by low glucose medium is a novel factor that improves the fertilization of artificial insemination in livestock animals and human infertility care. It is possible that diluting sperm with low glucose insemination medium will enhance sperm high-speed linear motility in the female genital tracts to improve fertilization as a simple and low-cost approach.
Data Availability
All datasets generated for this study are included in the manuscript and/or the Supplementary Files.
Author Contributions
ZZ and TU were responsible for experimental design, sample, data analysis, and writing the manuscript. TO, MG, YF, and TK collected the samples and interpreted the data. SH wrote the manuscript and edited it. WZ were responsible for discussion about experimental design, data analysis, and writing the manuscript. MS supervised all aspects of this study and wrote the manuscript.
Funding
This work was supported in part by Livestock Promotional Funds of Japan Racing Association (JRA) grant no. H30-279 and by Hiroshima Cryopreservation Service Co., grant no. H30-1 (to MS). ZZ was supported by China Scholarship Council during a visit of “Zhendong Zhu” to Hiroshima University (#CSC201706300110).
Conflict of Interest Statement
The authors declare that the research was conducted in the absence of any commercial or financial relationships that could be construed as a potential conflict of interest.
Supplementary Material
The Supplementary Material for this article can be found online at: https://www.frontiersin.org/articles/10.3389/fphys.2019.00252/full#supplementary-material
Footnotes
References
Alcivar, A. A., Hake, L. E., Millette, C. F., Trasler, J. M., and Hecht, N. B. (1989). Mitochondrial gene expression in male germ cells of the mouse. Dev. Biol. 135, 263–271. doi: 10.1016/0012-1606(89)90178-4
Amaral, A., Lourenco, B., Marques, M., and Ramalho-Santos, J. (2013). Mitochondria functionality and sperm quality. Reproduction 146, R163–R174. doi: 10.1530/REP-13-0178
Anderson, S., Bankier, A. T., Barrell, B. G., De Bruijn, M. H., Coulson, A. R., Drouin, J., et al. (1981). Sequence and organization of the human mitochondrial genome. Nature 290, 457–465. doi: 10.1038/290457a0
Barbonetti, A., Vassallo, M. R., Fortunato, D., Francavilla, S., Maccarrone, M., and Francavilla, F. (2010). Energetic metabolism and human sperm motility: impact of CB(1) receptor activation. Endocrinology 151, 5882–5892. doi: 10.1210/en.2010-0484
Bratic, I., and Trifunovic, A. (2010). Mitochondrial energy metabolism and ageing. Biochim. Biophys. Acta 1797, 961–967. doi: 10.1016/j.bbabio.2010.01.004
Brokaw, C. J. (1972). Flagellar movement: a sliding filament model. Science 178, 455–462. doi: 10.1126/science.178.4060.455
Brown-Woodman, P. D., and White, I. G. (1974). Amino acid composition of semen and the secretions of the male reproductive tract. Aust. J. Biol. Sci. 27, 415–422. doi: 10.1071/BI9740415
Charles, R. L., Schroder, E., May, G., Free, P., Gaffney, P. R., Wait, R., et al. (2007). Protein sulfenation as a redox sensor: proteomics studies using a novel biotinylated dimedone analogue. Mol. Cell. Proteomics 6, 1473–1484. doi: 10.1074/mcp.M700065-MCP200
Chomyn, A., Mariottini, P., Cleeter, M. W., Ragan, C. I., Matsuno-Yagi, A., Hatefi, Y., et al. (1985). Six unidentified reading frames of human mitochondrial DNA encode components of the respiratory-chain NADH dehydrogenase. Nature 314, 592–597. doi: 10.1038/314592a0
Contreras, L., Drago, I., Zampese, E., and Pozzan, T. (2010). Mitochondria: the calcium connection. Biochim. Biophys. Acta 1797, 607–618. doi: 10.1016/j.bbabio.2010.05.005
Cotgreave, I. A., and Gerdes, R. G. (1998). Recent trends in glutathione biochemistry–glutathione-protein interactions: a molecular link between oxidative stress and cell proliferation? Biochem. Biophys. Res. Commun. 242, 1–9. doi: 10.1006/bbrc.1997.7812
Curtis, M. P., Kirkman-Brown, J. C., Connolly, T. J., and Gaffney, E. A. (2012). Modelling a tethered mammalian sperm cell undergoing hyperactivation. J. Theor. Biol. 309, 1–10. doi: 10.1016/j.jtbi.2012.05.035
De Lamirande, E., Leclerc, P., and Gagnon, C. (1997). Capacitation as a regulatory event that primes spermatozoa for the acrosome reaction and fertilization. Mol. Hum. Reprod. 3, 175–194. doi: 10.1093/molehr/3.3.175
Demott, R. P., and Suarez, S. S. (1992). Hyperactivated sperm progress in the mouse oviduct. Biol. Reprod. 46, 779–785. doi: 10.1095/biolreprod46.5.779
Du Plessis, S. S., Agarwal, A., Mohanty, G., and Van Der Linde, M. (2015). Oxidative phosphorylation versus glycolysis: what fuel do spermatozoa use? Asian J. Androl. 17, 230–235. doi: 10.4103/1008-682X.135123
Fawcett, D. W. (1970). A comparative view of sperm ultrastructure. Biol. Reprod. 2(Suppl. 2), 90–127. doi: 10.1095/biolreprod2.Supplement_2.90
Gohil, V. M., Sheth, S. A., Nilsson, R., Wojtovich, A. P., Lee, J. H., Perocchi, F., et al. (2010). Nutrient-sensitized screening for drugs that shift energy metabolism from mitochondrial respiration to glycolysis. Nat. Biotechnol. 28, 249–255. doi: 10.1038/nbt.1606
Gur, Y., and Breitbart, H. (2006). Mammalian sperm translate nuclear-encoded proteins by mitochondrial-type ribosomes. Genes Dev. 20, 411–416. doi: 10.1101/gad.367606
Harris, S. E., Gopichandran, N., Picton, H. M., Leese, H. J., and Orsi, N. M. (2005). Nutrient concentrations in murine follicular fluid and the female reproductive tract. Theriogenology 64, 992–1006. doi: 10.1016/j.theriogenology.2005.01.004
Ho, H. C., Granish, K. A., and Suarez, S. S. (2002). Hyperactivated motility of bull sperm is triggered at the axoneme by Ca2+ and not cAMP. Dev. Biol. 250, 208–217. doi: 10.1006/dbio.2002.0797
Hofer, A., and Wenz, T. (2014). Post-translational modification of mitochondria as a novel mode of regulation. Exp. Gerontol. 56, 202–220. doi: 10.1016/j.exger.2014.03.006
Hogg, N. (2002). The biochemistry and physiology of S-nitrosothiols. Annu. Rev. Pharmacol. Toxicol. 42, 585–600. doi: 10.1146/annurev.pharmtox.42.092501.104328
Hurd, T. R., Requejo, R., Filipovska, A., Brown, S., Prime, T. A., Robinson, A. J., et al. (2008). Complex I within oxidatively stressed bovine heart mitochondria is glutathionylated on Cys-531 and Cys-704 of the 75-kDa subunit: potential role of CYS residues in decreasing oxidative damage. J. Biol. Chem. 283, 24801–24815. doi: 10.1074/jbc.M803432200
Huxley, A. F., and Niedergerke, R. (1954). Structural changes in muscle during contraction; interference microscopy of living muscle fibres. Nature 173, 971–973. doi: 10.1038/173971a0
Knox, R. V. (2016). Artificial insemination in pigs today. Theriogenology 85, 83–93. doi: 10.1016/j.theriogenology.2015.07.009
Labbe, K., Murley, A., and Nunnari, J. (2014). Determinants and functions of mitochondrial behavior. Annu. Rev. Cell. Dev. Biol. 30, 357–391. doi: 10.1146/annurev-cellbio-101011-155756
Lenaz, G., and Genova, M. L. (2010). Structure and organization of mitochondrial respiratory complexes: a new understanding of an old subject. Antioxid. Redox Signal. 12, 961–1008. doi: 10.1089/ars.2009.2704
Marden, W. G. (1961). Source of endogenous pyruvic acid in bovine seminal fluid and utilization. J. Dairy Sci. 44, 1688–1697. doi: 10.3168/jds.S0022-0302(61)89941-4
Marroquin, L. D., Hynes, J., Dykens, J. A., Jamieson, J. D., and Will, Y. (2007). Circumventing the crabtree effect: replacing media glucose with galactose increases susceptibility of HepG2 cells to mitochondrial toxicants. Toxicol. Sci. 97, 539–547. doi: 10.1093/toxsci/kfm052
Miseta, A., and Csutora, P. (2000). Relationship between the occurrence of cysteine in proteins and the complexity of organisms. Mol. Biol. Evol. 17, 1232–1239. doi: 10.1093/oxfordjournals.molbev.a026406
Mukai, C., and Travis, A. J. (2012). What sperm can teach us about energy production. Reprod. Domest. Anim. 47(Suppl. 4), 164–169. doi: 10.1111/j.1439-0531.2012.02071.x
Nichol, R., Hunter, R. H., Gardner, D. K., Leese, H. J., and Cooke, G. M. (1992). Concentrations of energy substrates in oviductal fluid and blood plasma of pigs during the peri-ovulatory period. J. Reprod. Fertil. 96, 699–707. doi: 10.1530/jrf.0.0960699
Odet, F., Duan, C., Willis, W. D., Goulding, E. H., Kung, A., Eddy, E. M., et al. (2008). Expression of the gene for mouse lactate dehydrogenase C (Ldhc) is required for male fertility. Biol. Reprod. 79, 26–34. doi: 10.1095/biolreprod.108.068353
Okazaki, T., Akiyoshi, T., Kan, M., Mori, M., Teshima, H., and Shimada, M. (2012). Artificial insemination with seminal plasma improves the reproductive performance of frozen-thawed boar epididymal spermatozoa. J. Androl. 33, 990–998. doi: 10.2164/jandrol.111.015115
Osellame, L. D., Blacker, T. S., and Duchen, M. R. (2012). Cellular and molecular mechanisms of mitochondrial function. Best Pract. Res. Clin. Endocrinol. Metab. 26, 711–723. doi: 10.1016/j.beem.2012.05.003
Phillips, D. M. (1972). Comparative analysis of mammalian sperm motility. J. Cell. Biol. 53, 561–573. doi: 10.1083/jcb.53.2.561
Plaza Davila, M., Martin Munoz, P., Tapia, J. A., Ortega Ferrusola, C., Balao Da Silva, C. C., and Pena, F. J. (2015). Inhibition of mitochondrial complex I leads to decreased motility and membrane integrity related to increased hydrogen peroxide and reduced atp production, while the inhibition of glycolysis has less impact on sperm motility. PLoS One 10:e0138777. doi: 10.1371/journal.pone.0138777
Potter, M., Newport, E., and Morten, K. J. (2016). The Warburg effect: 80 years on. Biochem. Soc. Trans. 44, 1499–1505. doi: 10.1042/BST20160094
Requejo, R., Hurd, T. R., Costa, N. J., and Murphy, M. P. (2010). Cysteine residues exposed on protein surfaces are the dominant intramitochondrial thiol and may protect against oxidative damage. FEBS J. 277, 1465–1480. doi: 10.1111/j.1742-4658.2010.07576.x
Ruiz-Pesini, E., Diez-Sanchez, C., Lopez-Perez, M. J., and Enriquez, J. A. (2007). The role of the mitochondrion in sperm function: is there a place for oxidative phosphorylation or is this a purely glycolytic process? Curr. Top Dev. Biol. 77, 3–19. doi: 10.1016/S0070-2153(06)77001-6
Shalgi, R., Smith, T. T., and Yanagimachi, R. (1992). A quantitative comparison of the passage of capacitated and uncapacitated hamster spermatozoa through the uterotubal junction. Biol. Reprod. 46, 419–424. doi: 10.1095/biolreprod46.3.419
Shimada, M., Yanai, Y., Okazaki, T., Noma, N., Kawashima, I., Mori, T., et al. (2008). Hyaluronan fragments generated by sperm-secreted hyaluronidase stimulate cytokine/chemokine production via the TLR2 and TLR4 pathway in cumulus cells of ovulated COCs, which may enhance fertilization. Development 135, 2001–2011. doi: 10.1242/dev.020461
Stauss, C. R., Votta, T. J., and Suarez, S. S. (1995). Sperm motility hyperactivation facilitates penetration of the hamster zona pellucida. Biol. Reprod. 53, 1280–1285. doi: 10.1095/biolreprod53.6.1280
Storey, B. T. (2008). Mammalian sperm metabolism: oxygen and sugar, friend and foe. Int. J. Dev. Biol. 52, 427–437. doi: 10.1387/ijdb.072522bs
Suarez, S. S. (2008). Control of hyperactivation in sperm. Hum. Reprod. Update 14, 647–657. doi: 10.1093/humupd/dmn029
Suarez, S. S., Katz, D. F., Owen, D. H., Andrew, J. B., and Powell, R. L. (1991). Evidence for the function of hyperactivated motility in sperm. Biol. Reprod. 44, 375–381. doi: 10.1095/biolreprod44.2.375
Suarez, S. S., and Pacey, A. A. (2006). Sperm transport in the female reproductive tract. Hum. Reprod. Update 12, 23–37. doi: 10.1093/humupd/dmi047
Summers, K. E., and Gibbons, I. R. (1971). Adenosine triphosphate-induced sliding of tubules in trypsin-treated flagella of sea-urchin sperm. Proc. Natl. Acad. Sci. U.S.A. 68, 3092–3096. doi: 10.1073/pnas.68.12.3092
Treulen, F., Uribe, P., Boguen, R., and Villegas, J. V. (2016). Mitochondrial outer membrane permeabilization increases reactive oxygen species production and decreases mean sperm velocity but is not associated with DNA fragmentation in human sperm. Mol. Hum. Reprod. 22, 83–92. doi: 10.1093/molehr/gav067
Umehara, T., Kawai, T., Goto, M., Richards, J. S., and Shimada, M. (2018). Creatine enhances the duration of sperm capacitation: a novel factor for improving in vitro fertilization with small numbers of sperm. Hum. Reprod. 33, 1117–1129. doi: 10.1093/humrep/dey081
Van Voorhis, B. J., Barnett, M., Sparks, A. E., Syrop, C. H., Rosenthal, G., and Dawson, J. (2001). Effect of the total motile sperm count on the efficacy and cost-effectiveness of intrauterine insemination and in vitro fertilization. Fertil. Steril. 75, 661–668. doi: 10.1016/S0015-0282(00)01783-0
Vecchio, D., Gasparrini, B., Di Palo, R., Iemma, L., Balestrieri, M. L., Killian, J. G., et al. (2007). Preliminary results on the composition of oviductal fluid in buffalo. Ital. J. Anim. Sci. 6, 731–734. doi: 10.4081/ijas.2007.s2.731
Woolley, D. M., and Fawcett, D. W. (1973). The degeneration and disappearance of the centrioles during the development of the rat spermatozoon. Anat. Rec. 177, 289–301. doi: 10.1002/ar.1091770209
Xie, X., Le, L., Fan, Y., Lv, L., and Zhang, J. (2012). Autophagy is induced through the ROS-TP53-DRAM1 pathway in response to mitochondrial protein synthesis inhibition. Autophagy 8, 1071–1084. doi: 10.4161/auto.20250
Xiong, W., Jiao, Y., Huang, W., Ma, M., Yu, M., Cui, Q., et al. (2012). Regulation of the cell cycle via mitochondrial gene expression and energy metabolism in HeLa cells. Acta Biochim. Biophys. Sin 44, 347–358. doi: 10.1093/abbs/gms006
Keywords: metabolic activity, glycolysis, mitochondrial oxidative phosphorylation, sperm motility pattern, energy source
Citation: Zhu Z, Umehara T, Okazaki T, Goto M, Fujita Y, Hoque SAM, Kawai T, Zeng W and Shimada M (2019) Gene Expression and Protein Synthesis in Mitochondria Enhance the Duration of High-Speed Linear Motility in Boar Sperm. Front. Physiol. 10:252. doi: 10.3389/fphys.2019.00252
Received: 10 December 2018; Accepted: 25 February 2019;
Published: 12 March 2019.
Edited by:
Tadashi Kimura, Osaka University Hospital, JapanReviewed by:
Seunghyung Lee, Kangwon National University, South KoreaHao Chen, Guangdong University of Technology, China
Copyright © 2019 Zhu, Umehara, Okazaki, Goto, Fujita, Hoque, Kawai, Zeng and Shimada. This is an open-access article distributed under the terms of the Creative Commons Attribution License (CC BY). The use, distribution or reproduction in other forums is permitted, provided the original author(s) and the copyright owner(s) are credited and that the original publication in this journal is cited, in accordance with accepted academic practice. No use, distribution or reproduction is permitted which does not comply with these terms.
*Correspondence: Wenxian Zeng, emVuZ3dlbnhpYW4yMDEzQDEyNi5jb20= Masayuki Shimada, bWFzaGltYWRAaGlyb3NoaW1hLXUuYWMuanA=
†These authors have contributed equally to this work