- 1Institute of Molecular and Translational Therapeutic Strategies (IMTTS), Hannover Medical School, Hanover, Germany
- 2National Heart and Lung Institute, Imperial College London, London, United Kingdom
During the past decade numerous studies highlighted the importance of long non-coding RNAs (lncRNAs) in orchestrating cardiovascular cell signaling. Classified only by a transcript size of more than 200 nucleotides and their inability to code for proteins, lncRNAs constitute a heterogeneous group of RNA molecules with versatile functions and interaction partners, thus interfering with numerous endogenous signaling pathways. Intrinsic transcriptional regulation of lncRNAs is not only specific for different cell types or developmental stages, but may also change in response to stress factors or under pathological conditions. Regarding the heart, an increasing number of studies described the critical regulation of lncRNAs in multiple cardiac disorders, underlining their key role in the development and progression of cardiac diseases. In this review article, we will summarize functional cardiac lncRNAs with a detailed view on their molecular mode of action in pathological cardiac remodeling and myocardial infarction. In addition, we will discuss the use of circulating lncRNAs as biomarkers for prognostic and diagnostic purposes and highlight the potential of lncRNAs as a novel class of therapeutic targets for therapeutic purpose in heart diseases.
Long Non-Coding RNAs
Although the majority of the human genome is transcribed into RNA molecules, only ∼2% of these transcripts code for proteins, thus, scientists have started to explore the wide universe of non-coding RNAs (ncRNAs) as crucial regulators of physiological and pathological cell function. Numerous studies demonstrated the involvement of small ncRNAs like microRNAs (miRNAs) in cardiovascular disease (Quiat and Olson, 2013; Thum, 2014), whereas the importance of long non-coding RNAs (lncRNAs) as key regulators in the development and progression of cardiac diseases is only beginning to be understood. As all ncRNAs longer than 200 nucleotides in length are arbitrarily classified as lncRNAs, this group of molecules is very heterogeneous and exhibit multifaceted biological functions and interact with a variety of other RNAs or proteins. Depending on their subcellular localization in the nucleus or cytoplasm, lncRNAs can interfere with transcriptional and post-transcriptional gene regulation, as well as mRNA translation, respectively. Nuclear transcripts, for example, can mediate epigenetic gene modifications or transcriptional activation and silencing, whereas cytoplasmic lncRNA often interact with miRNAs to post-transcriptionally regulate gene expression or act as molecular scaffolds for RNA-protein complexes. For further details on lncRNA modes of action we refer to the current literature available (Sun et al., 2017; Noh et al., 2018). Here, we discuss the growing body of evidence depicting a central role of lncRNAs in cellular responses during cardiac disease focusing on pathological hypertrophic cardiac growth and myocardial infarction (MI), and their potential to serve as novel therapeutic targets and diagnostic biomarkers.
LncRNAs in Cardiovascular Disease
The term cardiovascular disease (CVD) comprises a wide range of pathologies. However, in order to adequately address the importance of lncRNAs in distinct diseases and to highlight specific molecular modes of action, we will focus on a selection of lncRNAs associated with cardiac hypertrophy and myocardial infarction. In addition, Table 1 provides an exhaustive list of relevant lncRNAs in CVD. Importantly, lncRNAs have also been shown to play essential roles in embryonic development and cardiac lineage commitment [such as Fendrr and Braveheart (Grote et al., 2013; Klattenhoff et al., 2013)] and are important regulators of accurate heart functionality. More details on lncRNAs in organogenesis and heart development have been extensively reviewed elsewhere; (Devaux et al., 2015; Grote and Herrmann, 2015).
Cardiac Hypertrophy
One of the first studies on functionally relevant lncRNAs in cardiac hypertrophy discovered a cluster of lncRNAs partially overlapping the Myh7 gene locus which, accordingly, were called myosin heavy-chain-associated RNA transcripts (Mhrt) (Han et al., 2014). Mhrt was permanently downregulated after induction of cardiac hypertrophy by transverse aortic construction (TAC) surgery in mice. In addition, the dynamic regulation of this conserved, cardiac-specific lncRNA was accompanied by the TAC-induced isoform switch from Myh6 to Mhy7, a hallmark of developing cardiomyopathy (Miyata et al., 2000; Krenz and Robbins, 2004). Inducible transgenic overexpression of Mhrt resulted in reduced cardiac hypertrophy and fibrosis and improved cardiac function compared to TAC operated mice without reactivated Mhrt (Han et al., 2014). Importantly, this effect was observed when Mhrt expression was induced before TAC surgery as well as 2 weeks after pressure overload initiation, indicating that downregulation of Mhrt is important for the progression of pressure overload induced cardiac remodeling. Mechanistically, Mhrt directly interacts with the chromatin-remodeling factor Brg1 in order to inhibit its own transcriptional silencing at the shared Mhrt/Myh6 bidirectional promoter region under physiological conditions. In contrast, during cardiac stress Brg1 expression exceeds Mhrt abundance, resulting in active Brg1-mediated chromatin remodeling than leads to Mhy6 to Mhy7 isoform switch. This thereby represents an important regulatory circuit in the development and progression of cardiac hypertrophy.
Using a microarray approach to compare the lncRNA transcriptome of TAC versus sham operated mice, Viereck and colleagues identified the conserved lncRNA cardiac hypertrophy-associated transcript (Chast) to be upregulated in hypertrophic cardiomyocytes (Viereck et al., 2016). Chast expression is, at least partially, induced via the pro-hypertrophic transcription factor nuclear factor of activated T cells (NFAT) and acts in cis to regulate Pleckstrin homology domain–containing protein family M member 1 (Plekhm1), resulting in impaired autophagy. Remarkably, adeno-associated virus (AAV)-overexpression of Chast was sufficient to induce hypertrophic growth in vitro and in vivo in the absence of additional hypertrophic stress factors. In contrast, silencing of Chast using GapmeR antisense chemistries (for further details see section below ‘LncRNAs as potential therapeutic targets in CVD’) prevented hypertrophic cardiac growth and preserved cardiac function in TAC operated animals. Of note, silencing Chast was cardio-protective in a preventive approach, as well as in a clinically more relevant therapeutic approach with repeated GapmeR injection starting 4 weeks after induction of pressure overload in mice. Strikingly, the human CHAST transcript was able to induce hypertrophic cell growth in murine cardiomyocytes in vitro, suggesting functional conservation. Furthermore, CHAST was upregulated in hearts of patients with aortic stenosis, where cardiac hypertrophy occurs as a compensatory response to increased afterload, highlighting the therapeutic potential of CHAST for the treatment of cardiac hypertrophy in humans.
Another example of a pro-hypertrophic lncRNA, which emphasizes the importance of lncRNAs to precisely regulate a switch in gene expression upon cardiac stress, is cardiac-hypertrophy-associated epigenetic regulator (Chaer). Following TAC surgery Chaer-knockout mice showed less hypertrophic cardiac growth, reduced fibrosis and preserved cardiac function in comparison to wildtype control animals (Wang et al., 2016). In contrast, overexpression of Chaer induced hypertrophic cell growth in both phenylephrine and vehicle treated cardiomyocytes. The mainly nuclear located Chaer directly interacts with the EZH2 subunit of polycomb repressive complex 2 (PRC2), resulting in reduced H3K27 trimethylation at promoter regions and thereby enhanced expression of the pro-hypertrophic genes Anf, Myh7, and Acta. Furthermore, the authors highlighted the pivotal role of Chaer-PCR2 interaction at the onset of pathological cardiac stress by knocking down Chaer expression either 2 days before or 1 day after TAC surgery. Loss of Chaer at the very beginning of pathological pressure overload reduced hypertrophic heart growth and marker gene expression and improved cardiac function compared to control animals, while Chaer knockdown 24 h post TAC showed no protective effect. This early interaction between PRC2 and Chaer seems to be required for the onset of cardiac epigenetic reprogramming but not progression of hypertrophic remodeling.
Besides hypertrophic growth of cardiomyocytes, pressure overload induced pathological remodeling is accompanied by cardiac fibroblast (CF) activation and rearrangement of the extra cellular matrix (ECM), resulting in fibrosis and impaired cardiac function. By performing lncRNA array analysis in CFs of mice undergoing 13 weeks of TAC, Piccoli et al. identified over 1400 deregulated lncRNAs in CF fractions of hypertrophic mouse hearts (Piccoli et al., 2017). The most abundant and CF-specific lncRNA Maternally expressed gene 3 (Meg3) appeared to be upregulated during the first 4 weeks after TAC followed by a long term repression. Meg3 interaction with p53 facilitates transcriptional activation of matrix metalloproteinase-2 (MMP-2) and subsequent remodeling of ECM composition (Figure 1A). Consecutive injection of GapmeRs targeting Meg3 reduced cardiac fibrosis and ameliorated cardiac performance and diastolic function in TAC operated mice compared to control GapmeR treated animals. Interestingly, knockdown of Meg3 also diminished hypertrophic cardiomyocyte growth, although Meg3 showed no TAC-induced regulation in cardiomyocytes in the initial screen suggesting further paracrine effects of Meg3 silencing in CFs on other cardiac cell types.
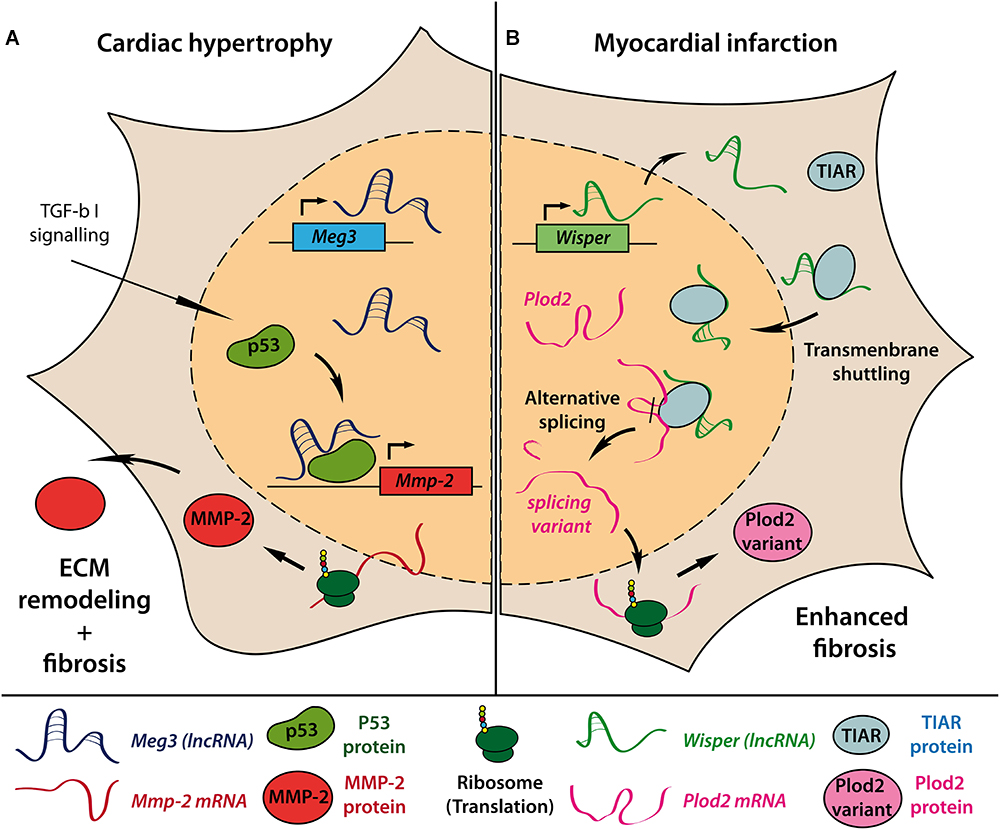
Figure 1. Molecular mechanisms of lncRNAs involved in cardiac fibrosis. Introduction of pressure-overload-induced cardiac hypertrophy in mice causes upregulation of the lncRNA Meg3 in cardiac fibroblasts. Interaction of Meg3 with the transcription factor p53 is required for TGF-β–induced transcription of Mmp-2 gene. Increased extracellular MMP-2 levels subsequently drive enhanced extracellular matrix (ECM) remodeling and cardiac fibrosis (A). Myocardial infarction causes upregulation of lncRNA Wisper in cardiac fibroblasts. Only in the presence of Wisper can TIAR shuttle from the cytoplasm to the nucleus to interfere with RNA processing and induce alternative splicing of Plod2 mRNA. The resulting alternative Plod2 protein variant causes enhanced fibrosis (B).
Myocardial Infarction
The lncRNA myocardial infarction associated transcript (MIAT) was first described in a large scale single nucleotide polymorphism (SNP) association study from a Japanese population in 2006. In this study the presence of six SNPs in the MIAT locus was associated with an increased risk for MI (Ishii et al., 2006). In 2017, Qu and colleagues also identified MIAT as a pro-fibrotic lncRNA after MI in vivo (Qu et al., 2017). CF activation and differentiation, cardiac fibrosis and scar formation represent key events after MI. On the one hand, fibrotic remodeling of the infarct region is crucial for sustaining myocardial integrity and preventing cardiac wall rupture during wound healing processes. On the other hand, sustained fibrosis throughout subsequent cardiac remodeling contributes to increased cardiac stiffness, impaired cardiac function and development of heart failure (Van Den Borne et al., 2010). In regards to MIAT, permanent occlusion of the left anterior descending artery (LAD) in mice resulted in it’s continuously upregulated expression in peri-infarcted tissue (Qu et al., 2017). By targeting several anti-fibrotic miRNAs (including miR-24, miR-29, miR-30, and miR-133), MIAT was shown to promote cardiac fibrosis and adverse remodeling in the infarcted mouse hearts. In contrast, lentiviral-mediated knockdown of MIAT prior to MI reduced infarct size and interstitial fibrosis contributing to preserved cardiac function, via the control of CF proliferation and collagen production. Additionally, two other publications highlighted further potential of MIAT to act as a pro-hypertrophic lncRNA in cardiomyocytes by sponging the anti-hypertrophic miR-150 (Zhu et al., 2016) and miR-93 (Li et al., 2018). Altogether, these findings emphasize the complex regulatory network of MIAT during cardiac disease and strengthen its potential to serve as a therapeutic target.
Recently, the conserved lncRNA Wisp2 super-enhancer–associated RNA (Wisper) has been described to control CF functions in vitro and in vivo. Wisper is transcribed from a cardiac-specific super-enhancer region and was found to be highly upregulated in the border zone after MI in mice (Micheletti et al., 2017). Wisper regulated proliferation, migration, and apoptosis as well as gene expression of pro-fibrotic factors like Col3a1, Fn1, and Tgfb2 in CFs but not fibroblasts from other origin. In vivo, GapmeR-induced knockdown of Wisper after MI resulted in a smaller infarct size, reduced fibrosis and preserved cardiac structure and function. Of note, pre-operative GapmeR treatment also impaired CF function in the acute wound healing process leading to increased mortality due to left ventricular wall rupture. The authors demonstrated that Wisper directly interacts with TIA1-related protein (TIAR) to regulate alternative splicing of lysyl hydroxylase 2 (LH2 or Plod2), which has been associated with fibrosis-related disorders (Yeowell et al., 2009; Figure 1B). Knockdown of Wisper suppressed transmembrane shuttling of TIAR protein to the nucleus and prevented TIAR-Plod2-mRNA interaction. Consequently, reduced Plod2 expression coincided with the described phenotypical changes in CF function in vitro and in vivo.
During MI, oxygen-deficiency primarily serves to induce massive loss in viable cardiomyocytes by apoptotic and necrotic cell death. Several studies have highlighted the regulatory involvement of lncRNAs in apoptotic cell death during MI. For example, the lncRNAs cardiac apoptosis-related lncRNA (Carl) (Wang et al., 2014b) and mitochondrial dynamic related lncRNA (Mdrl) (Wang et al., 2014c) were downregulated after MI. Adenoviral overexpression of Carl or Mdrl was able to inhibit mitochondrial fission and cardiomyocyte apoptosis by inhibiting pro-apoptotic miRNAs miR-539 or miR-361, respectively, and resulted in smaller infarct sizes in vivo. Similar to Carl and Mdrl, the lncRNA five prime to Xist (Ftx) is transcriptionally repressed after ischemia reperfusion injury and showed anti-apoptotic potential by regulating the Bcl2l2 repressor miRNA miR-29b-1-5p (Long et al., 2018) in vitro.
As highlighted above lncRNAs are crucial regulators of pathological cardiomyocyte growth, fibrosis and cell survival in hypertrophic and infarcted hearts (Figure 2). Through interfering with epigenetic gene regulation, transcriptional activation or repression and post-transcriptional modifications by directly interacting with proteins or other ncRNAs such as miRNAs, lncRNAs orchestrate miscellaneous intracellular signaling pathways in a number of cardiac diseases.
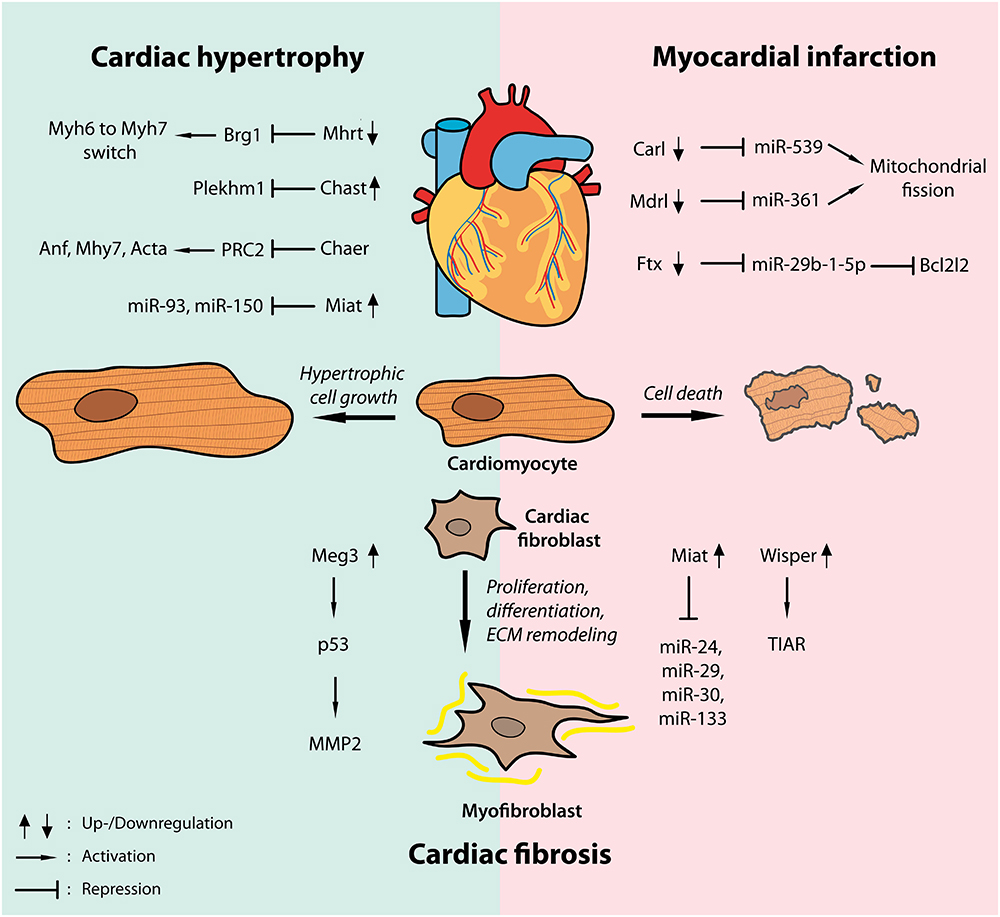
Figure 2. Widespread regulatory functions of lncRNAs in cardiac disease. Dynamic regulation of a number of lncRNAs has been shown in models of cardiac hypertrophy (left, blue shade) as well as myocardial infarction (right, pink shade) driving hypertrophic cardiomyocyte growth and cardiac fibrosis or regulating cardiac cell death by interfering with transcription factors, epigenetic modifiers or different microRNAs.
Besides intracellular signaling, changes in lncRNA expression levels also have the potential to influence intercellular communication by modulating paracrine signaling. The lncRNA myocardial infarction-associated transcript 1 (Mirt1) for example was shown to be involved in the regulation of the acute inflammatory response after MI (Li et al., 2017). In their study Li and colleagues demonstrated that hypoxia-induced upregulation of Mirt1 in neonatal mouse CFs facilitated nuclear transport of NF-κB and expression of the pro-inflammatory cytokines IL-6, IL-1β, and TNF-α. Secretion of these pro-inflammatory cytokines in turn mediated enhanced cardiomyocyte apoptosis and macrophage infiltration into the infarcted tissue.
In addition to the aforementioned modulation of paracrine signaling, first studies have appeared describing that secreted lncRNA display a novel layer of intercellular communication themselves by mediating cell proliferation (Zhang et al., 2018) or cancer progression (Pan et al., 2017). Furthermore, circulating lncRNAs might also have the potential to act in an endocrine manner and provoke systemic responses in CVD. Regardless, further studies need to be performed to reveal the consequences of altered lncRNA levels in the systemic circulation.
LncRNA as Biomarkers
As outlined above, lncRNAs have multifaceted intracellular regulatory functions and the capability to directly or indirectly alter intercellular communication. Furthermore, it has been shown that lncRNAs can be detected in extracellular body fluids such as plasma or urine and display a dynamic alteration upon diseases (Zhou et al., 2015; Martignano et al., 2017; Terracciano et al., 2017). LncRNAs can enter the blood stream encapsulated in exosomes (Li et al., 2015) and extracellular vesicles or inside of apoptotic bodies released from dying cells (Viereck and Thum, 2017). Beside this, association with RNA-binding proteins might also explain the enhanced stability of circulating lncRNAs conferred by their resistance to rapid degradation by RNases. Long term stability of lncRNAs in easily accessible body fluids, in combination with disease specific abundancy patterns, make lncRNAs of particular interest as a novel class of non-invasive prognostic and diagnostic biomarker.
One prominent example of lncRNAs as a potential biomarker is the mitochondria-derived lncRNA long intergenic non-coding RNA predicting cardiac remodeling (LIPCAR), whose plasma levels are associated with left ventricular (LV) remodeling after MI and increased risk of developing heart failure (Kumarswamy et al., 2014). Kumarswamy et al. reported a higher abundance of LIPCAR in the plasma of patients with consecutive heart failure after MI compared to MI patients without LV remodeling. Furthermore, the magnitude of circulating LIPCAR was also associated with an increased risk of cardiovascular death in chronic heart failure patients. In a 2-year follow-up, circulating LIPCAR was further identified as an independent predictor for diastolic dysfunction in well-controlled type 2 diabetes patients (De Gonzalo-Calvo et al., 2016). In addition, two other circulating lncRNAs, MIAT and smooth muscle and endothelial cell-enriched migration/differentiation-associated long non-coding RNA (SENCR), were associated with LV cardiac remodeling in these patients from the same study. Moreover, Zhang et al. reported increased plasma levels of LIPCAR and the paternally-imprinted lncRNA H19 in patients with coronary artery disease (CAD), especially in those subjects with concomitant chronic heart failure (Zhang et al., 2017).
Other recent studies identified the circulating lncRNAs non-coding repressor of NFAT (NRON) and MHRT as further independent predictors for heart failure (Xuan et al., 2017) and associated increased plasma levels of ANRIL with a higher risk for in-stent restenosis (Wang et al., 2017).
The possibility of detecting lncRNAs in extracellular body fluids discloses an enormous pool of molecules to extend the current catalog of prognostic and diagnostic biomarkers for cardiovascular and other diseases (Gutschner et al., 2017; Viereck and Thum, 2017).
LncRNAs as Potential Therapeutic Targets in CVD
CVDs still represent the number one cause of death worldwide (World Health Organization, 2017). As highlighted above, cardiac-specific in vivo modulation of lncRNAs exhibit the potential to ameliorate cardiac dysfunction or diminish pathological progression in the diseased heart (Han et al., 2014; Viereck et al., 2016; Wang et al., 2016; Micheletti et al., 2017; Piccoli et al., 2017; Qu et al., 2017), potentially making them new targets for the treatment of CVDs. LncRNAs may represent therapeutic targets, provided their expression can be modulated in vivo.
Silencing of RNA molecules can be achieved by the use of sequence-specific antisense oligonucleotides (ASO) or RNA interference (RNAi) methods. Antisense drug therapies have already been applied in clinical trials targeting protein-coding mRNAs with one compound already on the market for the treatment of familial hypercholesterolemia [Mipomersen (Rader and Kastelein, 2014; Geary et al., 2015; Santos et al., 2015)] and another approved by the Food and Drug Administration to treat Duchenne muscular dystrophy [Eteplirsen (Mendell et al., 2013, 2016)]. In contrast to protein-coding mRNAs, lncRNAs may exert different functions with respect to their subcellular localization (nucleus or cytoplasm). This needs to be considered for the general targeting strategy. SiRNAs, for example, mainly function in the cytoplasm, therefore may be less effective against nuclear localized lncRNAs (Lennox and Behlke, 2016). In addition to subcellular localization, tissue and/or cell type-specific delivery of antisense therapeutics is crucial for targeted lncRNA modulation in different CVDs. Different delivery strategies to improve targeting of the heart have been reviewed by Lucas and colleagues (Lucas et al., 2018).
Currently, GapmeRs are the most promising class of ASOs used for pharmacological silencing of lncRNAs in vivo, as they are able to enter the nucleus, thus, enable targeting of nuclear transcripts as well. GapmeRs consist of a DNA core flanked by two locked nucleic acids (LNA) sequences complementary to the target mRNA or lncRNA sequence. By chemically ‘locking’ the ribose backbone of the nucleotide structure, LNAs display a higher stability, target specificity and RNase H activation potential resulting in enhanced knockdown efficiency (Swayze et al., 2007). To date, no clinical trials targeting lncRNAs have been performed. This might be due to the relative novelty of lncRNAs been regarded as potential therapeutic targets compared to proteins. However, therapeutic GapmeR injections have successfully been used to modulate lncRNAs in animal models of pressure overload [Chast (Viereck et al., 2016) and Meg3 (Piccoli et al., 2017)] and MI [Wisper (Micheletti et al., 2017)]. In all of the mentioned studies the authors presented remarkably improved cardiac function upon therapeutic intervention, stressing the great potential of antisense drugs therapeutically targeting lncRNAs. Nevertheless, the use of LNA oligonucleotides may be associated with hepatotoxicity (Swayze et al., 2007; Burdick et al., 2014), highlighting the need for further chemical refinement of this novel class of drugs. For detailed information on the pharmacology of antisense drugs, different modification strategies, and current clinical trials in general and CVDs we refer to further literature specifically discussing these topics in depth (Bennett et al., 2017; Lucas et al., 2018; Smith and Zain, 2019).
In contrast to silencing approaches, therapeutic overexpression of lncRNAs in vivo appears to be more challenging and requires the use of viral-mediated gene delivery, nanoparticles, or RNA mimics. Overexpression of lncRNAs using viral gene delivery poses several obstacles including the efficiency of lncRNA upregulation itself. The second challenge is to overexpress the target lncRNA in its endogenous subcellular localization – in other words, to enhance the function of cis regulatory lncRNAs via ectopic overexpression. AAV vectors that are commonly used for gene therapy approaches have relatively low packaging limit. Hence, they cannot be used for lncRNA transcripts longer than 3–4 kb. Furthermore, depending on the pathological context, a transient or stable overexpression may be needed for the therapeutic treatment. Of note, the targeted lncRNA should be overexpressed in a cell type specific manner, as lncRNAs may have varied functions in different cells or organs (Raveh et al., 2015). Despite the aforementioned shortcomings, two independent studies provided proof of principle for cardio protective viral-based overexpression strategies in a preventive therapy approach using the MI mouse model (Wang et al., 2014b,c). Although a pre-MI treatment does not represent a clinically relevant scenario, these two studies provide first evidence for the prospective potential of lncRNA overexpression as a promising therapeutic intervention in cardiovascular diseases.
In summary, a vast number of lncRNAs are dynamically regulated upon initiation and progression of CVDs. Many have important biological functions and/or have the potential to serve as a novel class of circulating biomarkers. Several in vivo experiments have revealed that modulation of lncRNAs offers a promising new therapeutic approach to treat cardiovascular diseases, albeit the silencing or overexpression approaches still require further refinements. Nevertheless, large screening approaches are often performed in animal models of CVD and lncRNAs are not always conserved among species but this is a prerequisite for clinical translation. However, as the field of lncRNAs as potential therapeutic targets is still in its infancy it is not unlikely that in the near future lncRNAs will emerge as valuable new tools for the treatment of numerous diseases, including CVDs.
Author Contributions
LH wrote the manuscript and prepared the display items. CB and TT guided the manuscript preparation and critically revised it.
Funding
This work was supported by Deutsche Forschungsgemeinschaft (KFO311) and by the Federal Ministry of Education and Research (BMBF, Germany, research grant ERA-CVD JTC2016 EXPERT).
Conflict of Interest Statement
TT has filed patents about the diagnostic and therapeutic use of several cardiovascular lncRNAs and is a founder of and holds shares in Cardior Pharmaceuticals.
The remaining authors declare that the research was conducted in the absence of any commercial or financial relationships that could be construed as a potential conflict of interest.
Acknowledgments
We thank Michael Dodsworths for proofreading the manuscript.
References
Bennett, C. F., Baker, B. F., Pham, N., Swayze, E., and Geary, R. S. (2017). Pharmacology of Antisense drugs. Annu. Rev. Pharmacol. Toxicol. 57, 81–105. doi: 10.1146/annurev-pharmtox-010716-104846
Burdick, A. D., Sciabola, S., Mantena, S. R., Hollingshead, B. D., Stanton, R., Warneke, J. A., et al. (2014). Sequence motifs associated with hepatotoxicity of locked nucleic acid-modified antisense oligonucleotides. Nucleic Acids Res. 42, 4882–4891. doi: 10.1093/nar/gku142
Chen, L., Yan, K. P., Liu, X. C., Wang, W., Li, C., Li, M., et al. (2018). Valsartan regulates TGF-β/Smads and TGF-β/p38 pathways through lncRNA CHRF to improve doxorubicin-induced heart failure. Arch. Pharm. Res. 41, 101–109. doi: 10.1007/s12272-017-0980-4
De Gonzalo-Calvo, D., Kenneweg, F., Bang, C., Toro, R., Van Der Meer, R. W., Rijzewijk, L. J., et al. (2016). Circulating long-non coding RNAs as biomarkers of left ventricular diastolic function and remodelling in patients with well-controlled type 2 diabetes. Sci. Rep. 6, 1–12. doi: 10.1038/srep37354
Devaux, Y., Zangrando, J., Schroen, B., Creemers, E. E., Pedrazzini, T., Chang, C. P., et al. (2015). Long noncoding RNAs in cardiac development and ageing. Nat. Rev. Cardiol. 12, 415–425. doi: 10.1038/nrcardio.2015.55
Gast, M., Rauch, B. H., Nakagawa, S., Haghikia, A., Jasina, A., Haas, J., et al. (2018). Immune system-mediated atherosclerosis caused by deficiency of long non-coding RNA MALAT1 in ApoE-/-mice. Cardiovasc. Res. doi: 10.1093/cvr/cvy202 [Epub ahead of print].
Geary, R. S., Baker, B. F., and Crooke, S. T. (2015). Clinical and Preclinical pharmacokinetics and pharmacodynamics of mipomersen (Kynamro®): a Second-generation antisense oligonucleotide inhibitor of apolipoprotein B. Clin. Pharmacokinet. 54, 133–146. doi: 10.1007/s40262-014-0224-4
Grote, P., and Herrmann, B. G. (2015). Long noncoding RNAs in organogenesis: making the difference. Trends Genet. 31, 329–335. doi: 10.1016/j.tig.2015.02.002
Grote, P., Wittler, L., Hendrix, D., Koch, F., Währisch, S., Beisaw, A., et al. (2013). The tissue-specific lncrna fendrr is an essential regulator of heart and body wall development in the mouse. Dev. Cell 24, 206–214. doi: 10.1016/j.devcel.2012.12.012
Gutschner, T., Richtig, G., Haemmerle, M., and Pichler, M. (2017). From biomarkers to therapeutic targets – The promises and perils of long non-coding RNAs in cancer. Cancer Metastasis Rev. 37, 83–105.
Han, P., Li, W., Lin, C. H., Yang, J., Shang, C., Nurnberg, S. T., et al. (2014). A long noncoding RNA protects the heart from pathological hypertrophy. Nature 514, 102–106. doi: 10.1038/nature13596
Huang, S., Zhang, L., Song, J., Wang, Z., Huang, X., Guo, Z., et al. (2018). Long noncoding RNA MALAT1 mediates cardiac fibrosis in experimental postinfarct myocardium mice model. J. Cell. Physiol. 1, 1–10. doi: 10.1002/jcp.27117
Ishii, N., Ozaki, K., Sato, H., Mizuno, H., Saito, S., Takahashi, A., et al. (2006). Identification of a novel non-coding RNA, MIAT, that confers risk of myocardial infarction. J. Hum. Genet. 51, 1087–1099. doi: 10.1007/s10038-006-0070-9
Klattenhoff, C. A., Scheuermann, J. C., Surface, L. E., Bradley, R. K., Fields, P. A., Steinhauser, M. L., et al. (2013). Braveheart, a long noncoding RNA required for cardiovascular lineage commitment. Cell 152, 570–583. doi: 10.1016/j.cell.2013.01.003
Krenz, M., and Robbins, J. (2004). Impact of beta-myosin heavy chain expression on cardiac function during stress. J. Am. Coll. Cardiol. 44, 2390–2397. doi: 10.1016/j.jacc.2004.09.044
Kumarswamy, R., Bauters, C., Volkmann, I., Maury, F., Fetisch, J., Holzmann, A., et al. (2014). Circulating long noncoding RNA, LIPCAR, predicts survival in patients with heart failure. Circ. Res. 114, 1569–1575. doi: 10.1161/CIRCRESAHA.114.303915
Lai, Y., He, S., Ma, L., Lin, H., Ren, B., Ma, J., et al. (2017). HOTAIR functions as a competing endogenous RNA to regulate PTEN expression by inhibiting miR-19 in cardiac hypertrophy. Mol. Cell. Biochem. 432, 179–187. doi: 10.1007/s11010-017-3008-y
Lennox, K. A., and Behlke, M. A. (2016). Cellular localization of long non-coding RNAs affects silencing by RNAi more than by antisense oligonucleotides. Nucleic Acids Res. 44, 863–877. doi: 10.1093/nar/gkv1206
Li, H. Q., Wu, Y. B., Yin, C. S., Chen, L., Zhang, Q., Hu, L. Q. (2016a). Obestatin attenuated doxorubicin-induced cardiomyopathy via enhancing long noncoding Mhrt RNA expression. Biomed. Pharmacother. 81, 474–481. doi: 10.1016/j.biopha.2016.04.017
Li, Q., Shao, Y., Zhang, X., Zheng, T., Miao, M., Qin, L., et al. (2015). Plasma long noncoding RNA protected by exosomes as a potential stable biomarker for gastric cancer. Tumor Biol. 36, 2007–2012. doi: 10.1007/s13277-014-2807-y
Li, X., Wang, H., Yao, B., Xu, W., Chen, J., and Zhou, X. (2016b). LncRNA H19/miR-675 axis regulates cardiomyocyte apoptosis by targeting VDAC1 in diabetic cardiomyopathy. Sci. Rep. 6, 1–9. doi: 10.1038/srep36340
Li, X., Zhou, J., and Huang, K. (2017). Inhibition of the lncRNA Mirt1 attenuates acute myocardial infarction by suppressing NF-κB activation. Cell. Physiol. Biochem. 42, 1153–1164. doi: 10.1159/000478870
Li, Y., Wang, J., Sun, L., and Zhu, S. (2018). LncRNA myocardial infarction-associated transcript (MIAT) contributed to cardiac hypertrophy by regulating TLR4 via miR-93. Eur. J. Pharmacol. 818, 508–517. doi: 10.1016/j.ejphar.2017.11.031
Liu, L., An, X., Li, Z., Song, Y., Li, L., Zuo, S., et al. (2016). The H19 long noncoding RNA is a novel negative regulator of cardiomyocyte hypertrophy. Cardiovasc. Res. 111, 56–65. doi: 10.1093/cvr/cvw078
Long, B., Li, N., Xu, X. X., Li, X. X., Xu, X. J., Guo, D., et al. (2018). Long noncoding RNA FTX regulates cardiomyocyte apoptosis by targeting miR-29b-1-5p and Bcl2l2. Biochem. Biophys. Res. Commun. 495, 312–318. doi: 10.1016/j.bbrc.2017.11.030
Lucas, T., Bonauer, A., and Dimmeler, S. (2018). RNA therapeutics in cardiovascular disease. Circ. Res. 123, 205–220.
Martignano, F., Rossi, L., Maugeri, A., Gallà, V., Conteduca, V., De Giorgi, U., et al. (2017). Urinary RNA-based biomarkers for prostate cancer detection. Clin. Chim. Acta 473, 96–105. doi: 10.1016/j.cca.2017.08.009
Mendell, J. R., Goemans, N., Lowes, L. P., Alfano, L. N., Berry, K., Shao, J., et al. (2016). Longitudinal effect of eteplirsen versus historical control on ambulation in Duchenne muscular dystrophy. Ann. Neurol. 79, 257–271. doi: 10.1002/ana.24555
Mendell, J. R., Rodino-Klapac, L. R., Sahenk, Z., Roush, K., Bird, L., Lowes, L. P., et al. (2013). Eteplirsen for the treatment of Duchenne muscular dystrophy. Ann. Neurol. 37, e109–e111. doi: 10.1002/ana.23982
Micheletti, R., Plaisance, I., Abraham, B. J., Sarre, A., Alexanian, M., Maric, D., et al. (2017). The long noncoding RNA Wisper controls cardiac fibrosis and remodeling. Sci. Transl. Med. 9:eaai9118. doi: 10.1126/scitranslmed.aai9118
Miyata, S., Minobe, W., Bristow, M. R., and Leinwand, L. A. (2000). Myosin Heavy chain isoform expression in the failing and nonfailing human heart. 86, 386–390. doi: 10.1161/01.RES.86.4.386
Noh, J. H., Kim, K. M., Mcclusky, W. G., Abdelmohsen, K., and Gorospe, M. (2018). Cytoplasmic functions of long noncoding RNAs. Wiley Interdiscip. Rev. RNA 9:e1471. doi: 10.1002/wrna.1471
Pan, L., Liang, W., Fu, M., Huang, Z. H., Li, X., Zhang, W., et al. (2017). Exosomes-mediated transfer of long noncoding RNA ZFAS1 promotes gastric cancer progression. J. Cancer Res. Clin. Oncol. 143, 991–1004. doi: 10.1007/s00432-017-2361-2
Piccoli, M. T., Gupta, S. K., Viereck, J., Foinquinos, A., Samolovac, S., Kramer, F. L., et al. (2017). Inhibition of the cardiac fibroblast-enriched lncRNA Meg3 prevents cardiac fibrosis and diastolic dysfunction. Circ. Res. 121, 575–583. doi: 10.1161/CIRCRESAHA.117.310624
Qu, X., Du, Y., Shu, Y., Gao, M., Sun, F., Luo, S., et al. (2017). MIAT is a pro-fibrotic long non-coding RNA governing cardiac fibrosis in post-infarct myocardium. Sci. Rep. 7, 1–11. doi: 10.1038/srep42657
Quiat, D., and Olson, E. E. N. (2013). MicroRNAs in cardiovascular disease: from pathogenesis to prevention and treatment. J. Clin. Invest. 123, 11–18. doi: 10.1172/JCI62876.mature
Rader, D. J., and Kastelein, J. J. P. (2014). Lomitapide and mipomersen: two first-in-class drugs for reducing low-density lipoprotein cholesterol in patients with homozygous familial hypercholesterolemia. Circulation 129, 1022–1032. doi: 10.1161/CIRCULATIONAHA.113.001292
Rahimi, E., Ahmadi, A., Boroumand, M. A., Soltani, B. M., and Behmanesh, M. (2018). Association of ANRIL expression with coronary artery disease in type 2 diabetic patients. Cell J. 20, 41–45. doi: 10.22074/cellj.2018.4821
Raveh, E., Matouk, I. J., Gilon, M., and Hochberg, A. (2015). The H19 long non-coding RNA in cancer initiation, progression and metastasis – A proposed unifying theory. Mol. Cancer 14, 1–14. doi: 10.1186/s12943-015-0458-2
Santos, R. D., Raal, F. J., Catapano, A. L., Witztum, J. L., Steinhagen-Thiessen, E., and Tsimikas, S. (2015). Mipomersen, an Antisense oligonucleotide to apolipoprotein B-100, reduces lipoprotein(a) in Various populations with hypercholesterolemia: results of 4 phase III trials. Arterioscler. Thromb. Vasc. Biol. 35, 689–699. doi: 10.1161/ATVBAHA.114.304549
Smith, C. I. E., and Zain, R. (2019). Therapeutic oligonucleotides: state of the art. Annu. Rev. Pharmacol. Toxicol. 59:annurev-pharmtox-010818-021050. doi: 10.1146/annurev-pharmtox-010818-021050
Sun, Q., Hao, Q., and Prasanth, K. V. (2017). Nuclear long noncoding RNAs: key regulators of gene expression. Trends Genet. 34, 142–157. doi: 10.1016/j.tig.2017.11.005
Swayze, E. E., Siwkowski, A. M., Wancewicz, E. V., Migawa, M. T., Wyrzykiewicz, T. K., Hung, G., et al. (2007). Antisense oligonucleotides containing locked nucleic acid improve potency but cause significant hepatotoxicity in animals. Nucleic Acids Res. 35, 687–700. doi: 10.1093/nar/gkl1071
Terracciano, D., Ferro, M., Terreri, S., Lucarelli, G., D’Elia, C., Musi, G., et al. (2017). Urinary long noncoding RNAs in nonmuscle-invasive bladder cancer: new architects in cancer prognostic biomarkers. Transl. Res. 184, 108–117. doi: 10.1016/j.trsl.2017.03.005
Thum, T. (2014). Noncoding RNAs and myocardial fibrosis. Nat. Rev. Cardiol. 11, 655–663. doi: 10.1038/nrcardio.2014.125
Van Den Borne, S. W. M., Diez, J., Blankesteijn, W. M., Verjans, J., Hofstra, L., and Narula, J. (2010). Myocardial remodeling after infarction: the role of myofibroblasts. Nat. Rev. Cardiol. 7, 30–37. doi: 10.1038/nrcardio.2009.199
Vausort, M., Wagner, D. R., and Devaux, Y. (2014). Long noncoding RNAs in patients with acute myocardial infarction. Circ. Res. 115, 668–677. doi: 10.1161/CIRCRESAHA.115.303836
Viereck, J., Kumarswamy, R., Foinquinos, A., Xiao, K., Avramopoulos, P., Kunz, M., et al. (2016). Long noncoding RNA Chast promotes cardiac remodeling. Sci. Transl. Med. 8, 326ra22–326ra22. doi: 10.1126/scitranslmed.aaf1475
Viereck, J., and Thum, T. (2017). Circulating noncoding RNAs as biomarkers of cardiovascular disease and injury. Circ. Res. 120, 381–399. doi: 10.1161/CIRCRESAHA.116.308434
Wang, F., Su, X., Liu, C., Wu, M., and Li, B. (2017). Prognostic Value of plasma long noncoding RNA ANRIL for in-stent restenosis. Med. Sci. Monit. 23, 4733–4739. doi: 10.12659/MSM.904352
Wang, J. X., Zhang, X. J., Li, Q., Wang, K., Wang, Y., Jiao, J. Q., et al. (2015). MicroRNA-103/107 regulate programmed necrosis and myocardial ischemia/reperfusion injury through targeting FADD. Circ. Res. 117, 352–363. doi: 10.1161/CIRCRESAHA.117.305781
Wang, K., Liu, F., Zhou, L. Y., Long, B., Yuan, S. M., Wang, Y., et al. (2014a). The long noncoding RNA CHRF regulates cardiac hypertrophy by targeting miR-489. Circ. Res. 114, 1377–1388. doi: 10.1161/CIRCRESAHA.114.302476
Wang, K., Long, B., Zhou, L. Y., Liu, F., Zhou, Q. Y., Liu, C. Y., et al. (2014b). CARL lncRNA inhibits anoxia-induced mitochondrial fission and apoptosis in cardiomyocytes by impairing miR-539-dependent PHB2 downregulation. Nat. Commun. 5:3596. doi: 10.1038/ncomms4596
Wang, K., Sun, T., Li, N., Wang, Y., Wang, J. X., Zhou, L. Y., et al. (2014c). MDRL lncRNA Regulates the processing of miR-484 primary transcript by targeting miR-361. PLoS Genet. 10:e1004467. doi: 10.1371/journal.pgen.1004467
Wang, Z., Zhang, X. J., Ji, Y. X., Zhang, P., Deng, K. Q., Gong, J., et al. (2016). The long noncoding RNA Chaer defines an epigenetic checkpoint in cardiac hypertrophy. Nat. Med. 22, 1131–1139. doi: 10.1038/nm.4179
World Health Organization (2017). Fact Sheet Cardiovascular diseases (CVDs). Available at: http://www.who.int/en/news-room/fact-sheets/detail/cardiovascular-diseases-(cvds) [accessed November 28, 2018].
Wu, H., Zhao, Z.-A., Liu, J., Hao, K., Yu, Y., Han, X., et al. (2018). Long noncoding RNA Meg3 regulates cardiomyocyte apoptosis in myocardial infarction. Gene Ther. 25, 511–532. doi: 10.1038/s41434-018-0045-4
Xuan, L., Sun, L., Zhang, Y., Huang, Y., Hou, Y., Li, Q., et al. (2017). Circulating long non-coding RNAs NRON and MHRT as novel predictive biomarkers of heart failure. J. Cell. Mol. Med. 21, 1803–1814. doi: 10.1111/jcmm.13101
Yeowell, H. N., Walker, L. C., Mauger, D. M., Seth, P., and Garcia-Blanco, M. A. (2009). TIA nuclear proteins regulate the alternate splicing of lysyl hydroxylase 2. J. Invest. Dermatol. 129, 1402–1411. doi: 10.1038/jid.2008.386
Zhang, P., Zhou, H., Lu, K., Lu, Y., Wang, Y., and Feng, T. (2018). Exosome-mediated delivery of MALAT1 induces cell proliferation in breast cancer. Onco. Targets. Ther. 11, 291–299. doi: 10.2147/OTT.S155134
Zhang, Z., Gao, W., Long, Q.-Q., Zhang, J., Li, Y.-F., Liu, D.-C., et al. (2017). Increased plasma levels of lncRNA H19 and LIPCAR are associated with increased risk of coronary artery disease in a Chinese population. Sci. Rep. 7:7491. doi: 10.1038/s41598-017-07611-z
Zhou, M., Zou, Y., Xue, Y., Wang, X., and Gao, H. (2018). Long non-coding RNA H19 protects acute myocardial infarction through activating autophagy in mice. Eur. Rev. Med. Pharmacol. Sci. 22, 5647–5651.
Zhou, X., Yin, C., Dang, Y., Ye, F., and Zhang, G. (2015). Identification of the long non-coding RNA H19 in plasma as a novel biomarker for diagnosis of gastric cancer. Sci. Rep. 5, 1–10. doi: 10.1038/srep11516
Zhou, X., Zhang, W., Jin, M., Chen, J., Xu, W., and Kong, X. (2017). lncRNA MIAT functions as a competing endogenous RNA to upregulate DAPK2 by sponging miR-22-3p in diabetic cardiomyopathy. Cell Death Dis. 8:e2929. doi: 10.1038/cddis.2017.321
Keywords: non-coding RNA, cardiac hypertrophy, myocardial infarction, cardiovascular disease, therapy
Citation: Hobuß L, Bär C and Thum T (2019) Long Non-coding RNAs: At the Heart of Cardiac Dysfunction? Front. Physiol. 10:30. doi: 10.3389/fphys.2019.00030
Received: 18 April 2018; Accepted: 11 January 2019;
Published: 29 January 2019.
Edited by:
Lars Maegdefessel, Karolinska Institute (KI), SwedenReviewed by:
Keith W. Vance, University of Bath, United KingdomChristian Schulte, King’s College London, United Kingdom
Copyright © 2019 Hobuß, Bär and Thum. This is an open-access article distributed under the terms of the Creative Commons Attribution License (CC BY). The use, distribution or reproduction in other forums is permitted, provided the original author(s) and the copyright owner(s) are credited and that the original publication in this journal is cited, in accordance with accepted academic practice. No use, distribution or reproduction is permitted which does not comply with these terms.
*Correspondence: Thomas Thum, dGh1bS50aG9tYXNAbWgtaGFubm92ZXIuZGU=